- 1Global Academy of Agriculture and Food Systems, University of Edinburgh, Midlothian, United Kingdom
- 2Global Maize Program, International Maize and Wheat Improvement Center (CIMMYT), Harare, Zimbabwe
- 3Sustainable Agrifood Systems, International Maize and Wheat Improvement Center (CIMMYT), Harare, Zimbabwe
- 4Genetic Resources Program, International Maize and Wheat Improvement Center (CIMMYT), México-Veracruz, Texcoco, Mexico
- 5Sustainable Agrifood Systems, International Maize and Wheat Improvement Center (CIMMYT), Nairobi, Kenya
- 6Global Wheat Program, International Maize and Wheat Improvement Center (CIMMYT), México-Veracruz, Texcoco, Mexico
- 7Donor Relations, International Maize and Wheat Improvement Center (CIMMYT), México-Veracruz, Texcoco, Mexico
- 8Ecological Sciences, The James Hutton Institute, Aberdeen, United Kingdom
- 9Global Maize Program, International Maize and Wheat Improvement Center (CIMMYT), Nairobi, Kenya
Challenges of soil degradation and changing climate pose major threats to food security in many parts of the world, and new approaches are required to close yield and nutrition gaps through enhanced agronomic efficiency. Combined use of mineral fertilizers, organic inputs, improved germplasm and adaptation of these practices to local contexts through improved agronomy can promote efficiency whilst building stocks of soil organic matter (SOM). Within this framework, recent attention has turned to the nature of plant-soil interactions to increase response to mineral fertilizer inputs through utilisation of nutrients from SOM that are replenished through management. This utilisation has been shown in barley and maize to vary with genotype and to be related to root physiological traits associated with rhizodeposition. The identification of candidate genes associated with rhizodeposition takes this a step closer towards the possibility of breeding for sustainability. Here we discuss this potential and feasibility in the context of maize cropping systems, and explore the potential for a combined approach that optimises utilisation of SOM nutrients together with enhanced biological nitrification inhibition to further improve agronomic efficiency.
1 Introduction
Ongoing population growth and climate change represent major pressures on global food security (1, 2), necessitating the development of cropping systems that are productive (yield and nutritional value), are resilient to extreme and variable weather patterns, and that minimise feedbacks to climate forcing via greenhouse gas (GHG) emissions. Sub-Saharan Africa (SSA) is on the frontline of climate change, and technologies are urgently needed for this region that couple sustainable crop production combined with greater soil fertility and continuous protection of the soil resource, with enhanced resilience to climate induced changes in local conditions (3). Soils supporting food production in this region are often degraded, with 25% (350 million ha) of the total land area falling under this category (4), and characterised by poor structure, light texture and low (and declining) soil organic matter (SOM) content, often resulting from continuous conventional agriculture practices (tillage, crop residue removal, mono-cropping) (5, 6).
Increased inorganic fertilizer, in combination with organic inputs and adaptive management, is generally seen as a core enabler of sustainable productivity increases (7). Yet current rates of nitrogen fertilizer usage are very low in most parts of SSA (8). This has been attributed to low average (and highly heterogeneous) agronomic and economic returns to fertilizer usage by smallholders in these systems (9). Such low returns reflect multiple factors: high fertilizer prices and last-mile transportation costs, low output prices, and/or poor agronomic use efficiency. Under current conditions, in many parts of the region, increased inorganic fertilizer use may not be an economically viable way to raise yields (10). This evidence suggests that there would be large and widespread potential economic (and environmental) gains from crop varieties with improved capacity for nutrient utilization from SOM.
Fundamentally, the resources most limiting crop growth are nutrients and water (11), each acquired by roots from soil, while the aboveground resources of light and carbon (C) are effectively non-limiting. Consequently, while it is possible to improve plant water and nutrient use efficiencies to promote grain yields (12, 13), it is logical that there should also be a specific focus on root-soil interactions that mediate the acquisition and availability of these resources (14). This emphasis is particularly relevant for smallholder rain-fed maize systems in SSA, where increased return on investment to fertilizer application alongside improved soil management practices could sustainably increase yields whilst minimizing increased soil N2O emissions (15, 16). Here we explore the feasibility for approaches that optimise utilisation of nutrients from soil, together with enhanced biological nitrification inhibition (BNI) and restorative soil management, to further improve agronomic efficiency tailored to local contexts in maize-based systems (Figure 1); namely (a) harnessing root-soil interactions, (b) advances in genetic approaches underpinning a root trait approach to breeding, and (c) deploying root-soil interactions into breeding programs.
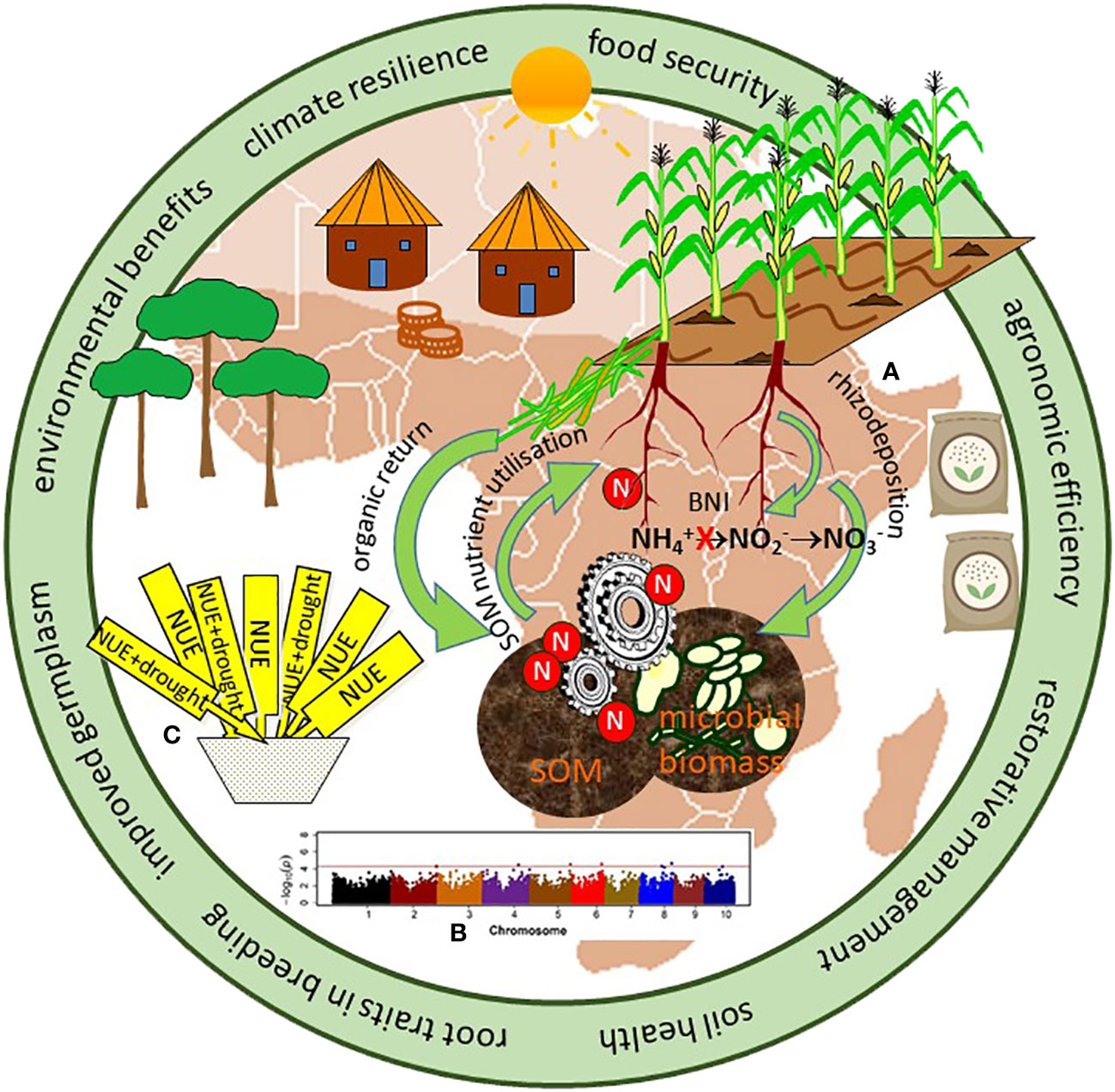
Figure 1 Conceptual representation of our proposed circular nutrient economy and root trait approach to inform breeding for germplasm to enhance agronomic efficiency, climate resilience, and food security. Discussion highlighted in this article is indicated as (A) harnessing root-soil interactions, (B) advances in genetic approaches underpinning a root trait approach to breeding, and (C) deploying root-soil interactions into breeding programs.
2 Harnessing root-soil interactions
2.1 The rhizosphere - a dynamic environment for nutrient supply
The rhizosphere, soil surrounding and under direct influence of roots, has long been recognised as a dynamic environment for microbial communities and the biogeochemical processes that they mediate. In the last decade our understanding has extended to recognition that impacts on microbial communities are plant species and genotype specific, giving rise to the concept of the plant microbiome, where the root-associated microbial community is an intrinsic component of plant interactions with the soil environment (17). Maize is known for its remarkable phenotypic diversity, for example in root traits such as root length, root diameter, and root mass that are influenced by genotype (14, 18); therefore, we expect differences within maize, in terms of influence on the soil microbiome.
This coupling of root and microbial processes (rhizodeposition and biogeochemical fluxes) is critically important in the context of soil nutrient cycling and plant productivity, particularly in low-input agroecosystems prevalent in SSA, as plant nutrient supply is dependent on microbial transformation of SOM into forms available for root uptake (14, 19). Selecting maize varieties that are best equipped to source N from SOM mineralization, whilst also lowering the loss of N through BNI, could help ensure reliable and timely N supply and reduce the low response to fertilizer inputs, a key barrier to reducing the yield gap in SSA (Figure 1).
2.2 Impacts of rhizodeposition on microbial processes
A consequence of the rhizodeposition flux from roots is that it shifts microbial communities in the rhizosphere from a state of C-limitation that is dominant in bulk soil, to a condition where nutrient elements are often limiting to their growth (Figure 2A). Therefore, this can alter microbial community structure (20) and activities (e.g., exo-enzyme production) directed to mobilisation of nutrients from SOM (21). Such plant-mediated rhizosphere priming effects (RPE, 22) function to couple plant growth with microbial nutrient cycling (19). Several studies, across different crop species, have demonstrated that the magnitude of RPE varies with plant genotype (14, 23–26), and this variation is linked to the magnitude and composition of rhizodeposition fluxes, and consequently impacts on microbiome selection and activities (27). Importantly, for barley and maize, this genotype variability in RPE has been identified as representing traits that are heritable, and consequently potential targets for plant breeding (28, 29). In this context, Gowda et al. (28) showed root length, root diameter and plant-derived C flux through soil to be strong predictors of SOM-C mineralization (Figure 2C) and identified two candidate genes in maize associated with enhanced SOM-C mineralization rates. Similarly, it has been demonstrated that root branching, root hair formation, and mucilage production (each under genetic control) are key to rhizosheath development around roots (30), underpinning drought tolerance and improved nutrient acquisition of cultivars under drought conditions (23, 31; Figure 2B). Although mucilage plays an important role in rhizosphere functions, our understanding of how mucilage amounts and polysaccharide composition are affected by maize genotypes is still limited (32). There is potential to target genes for such beneficial root-soil interactions, with the specific aim of improving the sustainability of maize cropping systems in SSA.
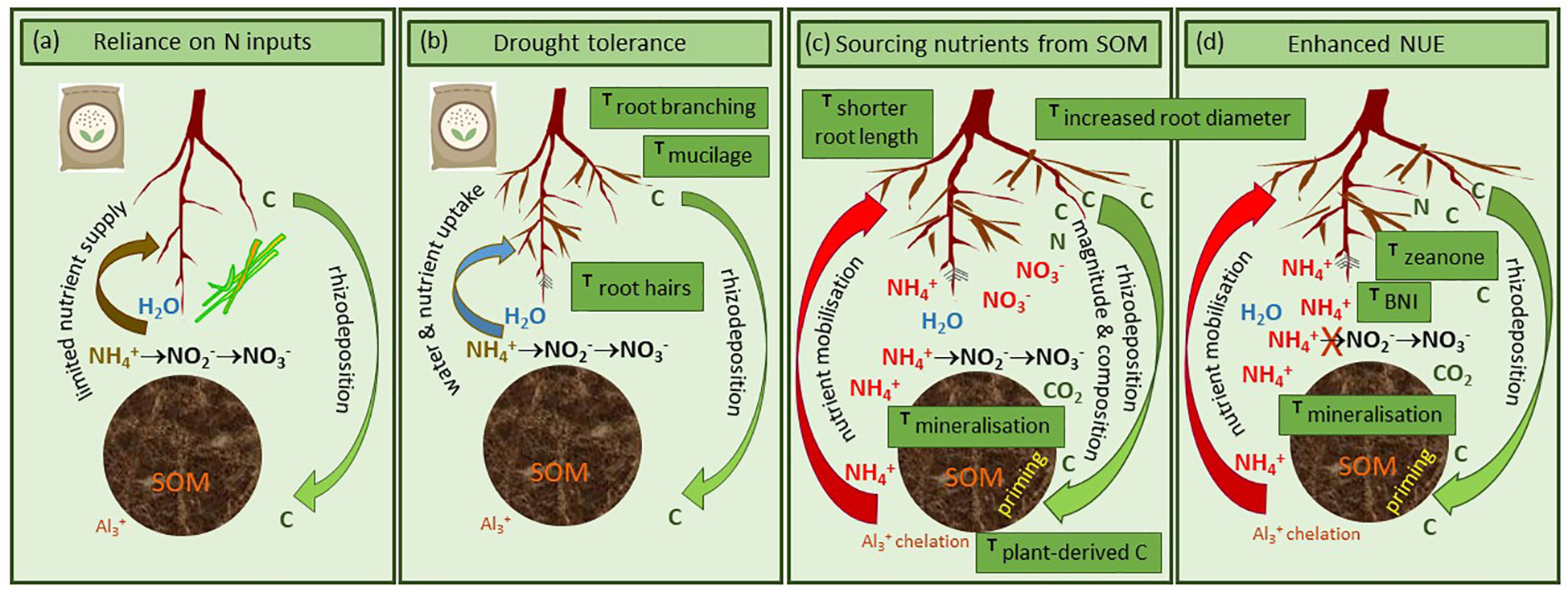
Figure 2 Theoretical representation of processes and associated traits of different maize genotypes exhibiting (A) reliance on mineral and organic inputs to alleviate nutrient limitation, (B) drought tolerance for enhanced water and nutrient uptake, (C) ability to source nutrients released from priming of soil organic matter (SOM), and (D) ability to enhance biological nitrification inhibition (BNI) to retain these SOM-derived nutrients. T indicates the maize traits we highlight in our text as potential targets for breeding.
The identification of potential candidate genes for traits influencing rhizosphere processes paves the way for breeding to achieve more resilient and sustainable maize production. As our understanding of the functional roles and genetic bases of secondary metabolite exudation from roots develops, it is likely that these traits will be exploitable to increase crop resistances to abiotic and biotic stresses (33). Also important is the reorientation of maize breeding to leverage microbial-maize interactions, for example, arbuscular mycorrhizal fungi (AMF)-maize and dark septate endophyte symbioses, which improve nutrient uptake from the rhizosphere (34, 35). This can be achieved by breeding for maize genotypes that exude more strigolactones that initiate root colonization by AMF, and hence improving nutrient acquisition (36). However, nutrients from SOM need to be replenished through management, otherwise there is a risk of soil N mining (37), especially in maize monoculture cropping systems that lack N return and result in a negative N budget/balance of the system. Introducing maize varieties with higher N uptake potential is therefore a promising sustainable solution when combined with strategies to maintain soil N status and improve plant N-use efficiency (NUE), such as BNI and appropriate crop rotations, or intercropping.
2.3 Biological nitrification inhibition
SOM mineralization mobilises ammonium, which is subject to subsequent nitrification. Whilst this supplies N for crop uptake, nitrate can be readily lost through leaching and emission of nitrous oxide. Secondary root-derived substances can also directly regulate key soil N processes through BNI (38–40). BNI is triggered by ammonium concentrations and favoured by low soil pH (41, 42). It can lead to a more balanced plant N uptake, higher yields (43), mitigate nitrous oxide emissions (44) and reduce soil nitrate leaching (45).
BNI has been shown to have a positive impact on maize yields due to a residual BNI effect by a previous Brachiaria humidicola pasture under a rotated cropping system (46). Apart from rotating crops with BNI grasses, another strategy is to further screen promising genotypes of cereals for the BNI trait. Recently an active BNI compound called “zeanone” has been identified in a maize line. This isolated hydrophobic inhibitor has been shown to have a major BNI effect on Nitrosomonas europaea (47). Furthermore, zeanone was present in a set of maize lines characterized for hydrophobic BNI capacity, where there was a large phenotypic variation for both zeanone-intensity, and hydrophobic-BNI-activity (CIMMYT, unpublished results). It is very likely that BNI plays a key role within maize for improved NUE (Figure 2D). However, it is unknown how widespread the phenomenon is among maize lines used in SSA. It is likely that the BNI trait survived the selection process in maize and that it even plays a key role for maize lines that perform well under low soil pH and low N input systems. Since BNI activity has been identified for some genotypes of Sorghum bicolor and Brachiaria humidicola, it is probably an evolutionary strategy for survival in low fertile soils in SSA (48).
Maize selection for southern African countries has been conducted under low N and low pH soils. Such soils have a high ammonium-to-nitrate ratio and genotypes that perform well are likely to be adapted to ammonium-N nutrition and even benefit from it (49). These edaphic factors represent the perfect environment where sourcing nutrients from SOM and BNI achieve beneficial effects in terms of plant N nutrition.
The prevalence of acidic soils in SSA represents an important constraint on crop productivity (50). Low soil pH (< 5.0) promotes the solubilisation of aluminium (Al) into Al3+ ionic forms that are highly rhizotoxic, strongly inhibiting root elongation and development (51). Consequently, root traits conferring tolerance to soluble Al in soil are required, in parallel with those mediating efficient use of soil nutrient sources. Resistance mechanisms, underlying substantial variation in Al tolerance (52, 53), include root exudation of organic acids as a means of chelating Al3+ in soil solution, preventing entry into roots. As this mechanism is understood to be regulated by specific genes encoding malate and citrate membrane transporters, there is strong potential to incorporate this exclusion-based resistance into breeding programmes (54).
3 Advances in genetic approaches underpinning a root trait approach to breeding
To date, maize genetic improvement strategies in SSA have focused on yield potential and yield defending traits. Over the last decade advances have been made in conventional and molecular breeding in SSA, targeting traits for abiotic and biotic stress tolerances such as nitrogen use efficiency, maize streak virus and drought tolerance (55). Successful adoption of improved maize cultivars has already demonstrated the strong potential of breeding approaches to address specific constraints on productivity in SSA (56).
Root traits to improve crop stress tolerance and yield potential have been extensively studied over the past century (57). Under rapidly changing climatic scenarios, plant root traits which have a critical role in improving crop nutrition and root-soil interactions are gaining importance in the development of more sustainable and resilient varieties. Improving root ideotypes through architectural, anatomical and physiological traits is a best optimal way to access rhizosphere resources, which paves the way to develop more resilient varieties. Difficulty in phenotyping belowground root traits and lack of high throughput phenotyping tools has often been cited for the absence of selection for root traits in breeding programs (58). Given the scale (in terms of geographic and pipeline size) that breeding programs work on, even with improved low-cost, high throughput phenotyping methods, routine selection for root traits within a conventional breeding program is unlikely in the near future. For example, in CIMMYT’s southern Africa maize breeding pipeline the number of entries in stages 1, 2, 3 and 4 are 1,800, 800, 220 and 30, respectively (59). To obtain robust estimates of the genetic value of each entry, particularly for low heritable traits, multi-location data is required. Thus, even high throughput phenotyping options for specific root traits would require a significant budget. Breeding programs operate within fixed, and generally limited, budgets. Within a conventional breeding scheme, this would require a reallocation of budget from current selection targets towards root phenotyping and/or a reduction in population size which would reduce overall genetic gain in key traits required by farmers.
Modern tools offer new opportunities to deploy complex traits within a breeding program. Several studies have been conducted to dissect the genetic basis of root-soil interactions. Nested association mapping comprising 5000 maize lines derived from 27 founder parents has extensively been used to dissect multiple agronomic traits. Peiffer et al. (60) used these 27 founder lines to study the rhizosphere microbiome and observed substantial variation in bacterial richness, diversity and relative abundances of taxa between bulk soil and the maize rhizosphere. The rhizospheres of maize inbreds exhibited both a small but significant proportion of heritable variation in total bacterial diversity across fields, and substantially more heritable variation between replicates of the inbreds within each field. The association between maize genotype and microbial diversity using 16S rRNA gene microarrays supports bacterial diversity being related to plant genotype (61, 62). Variation in rhizosphere microbiome composition is likely controlled by plant genetic factors (i.e. heritable) in maize (60), and sorghum (63). However, it remains unclear to what extent these heritable microbes are affected by the plant host and contribute to variation in the crop phenotype. Like any other trait under heritable genetic control, rhizosphere microbiome traits can be targeted in selective breeding experiments. This potential has been exemplified in barley and maize, for which genome wide association studies and quantitative trait loci analyses have revealed several, small effect single nucleotide polymorphisms (SNPs) and potential candidate genes associated with traits related to increased microbial mineralization of SOM, root-derived microbial biomass C and dissolved organic C (28, 29).
Genome-wide association studies with 196 world-wide accessions of Arabidopsis thaliana successfully revealed associations between plant genes and rhizosphere microbiome traits at high-level measures of rhizosphere community (64), or at order level (derived from operational taxonomic units [OTUs]) in a sorghum diversity panel (63). A genome-wide association study with 230 diverse maize lines was conducted using 150 microbial groups which are abundant and consistently reproducible (65). These microbial groups were used as rhizobiome traits and identified 622 plant loci that are linked to 104 microbial groups in the maize rhizosphere. Among these 104 microbial groups as traits, 62 were correlated with variation in plant vigour indicators derived from high throughput phenotyping of the same field experiment. This study revealed that microbial communities are actively and vigorously formed by host plant genetic variation which can be used as the foundation for future research on plant-microbe interactions and ultimately to increase crop productivity and resilience to abiotic stress.
Genome editing technologies are used to target accurately any gene of interest and efficiently introduce them into a new population (66). Genome editing is successfully used on root traits in different crops like in rice for confirmation of DRO1-related genes which are associated with root growth angle (67). Through CRISPR/Cas9 technology, gene/s responsible for more root hairs which improves nodule formation and nitrogen fixation were introduced in to cultivated soyabean (68). In cotton, arginase genes (GhARG) were knocked out to enhance the lateral root formation (69). Nevertheless, CRISPR is more effective on traits controlled by a single or few genes but most of the root traits are polygenic in nature which limits its application on root traits improvement. Since the target genes must be known prior to CRISPR application, it is necessary to improve our understanding of genes affecting root traits, to enable effective use of genome editing technologies in this respect.
4 Deploying root-soil interactions into breeding programs
Genotypic information can be deployed within a breeding pipeline in several ways, primarily depending on the trait architecture and genotyping cost. Forward breeding is a simple form of population enrichment where markers tightly linked to genomic regions of importance are selected prior to field phenotyping. Early research suggests root traits associated with root-soil interactions are polygenic, controlled by many small effect loci and are therefore not amenable to forward breeding. Genomic selection offers the ability to select lines for advancement based on the genomic estimated breeding value (GEBV) of each line and has been successfully used to select for complex traits in animal and plant breeding over the past decade (70). Genomic selection is already deployed in maize breeding in SSA for grain yield under drought, heat tolerance and optimal conditions. All stage 1 entries are genotyped with mid-density makers and advancing individuals to the next stage of testing is based on GEBVs and GBLUPs. In theory, this genomic selection could be extended to traits associated with SOM mineralization and BNI if high quality phenotypic data is available to train models. To date, most phenotyping for SOM mineralization and BNI has been conducted in semi-controlled environments and/or under a limited range of environments. Further work is required to dissect the genotype x environment x management interactions of traits associated with SOM mineralization and BNI before deployment.
5 Discussion
Here we present the potential for consideration of plant-soil interactions as a frontier approach to plant breeding for enhanced agronomic efficiency. Specifically we outline the possibility of selection for enhanced nutrient utilisation in maize, through sourcing of nutrients from SOM and BNI to lower loss of this mineralized N. We highlight the potential of modern breeding approaches to enrich populations for markers linked with key traits associated with SOM mineralization and BNI prior to field phenotyping. Together with the development of improved agricultural practices to promote a circular nutrient economy with replenishment of SOM, this approach promises to contribute to food security in SSA through alleviating low nutrient availability in degraded soils and enhancing the return from mineral fertilizer inputs. Acquisition of nutrients is equally important to reduce their loss, and coupling traits associated with SOM mineralization and BNI with nutrient acquisition traits is an important advancement in breeding programs. Breeding for traits that improve plant-microbial interactions such as maize-AMF associations will serve to improve the acquisition of mineralized N. The example we give here is focused on maize cropping systems in SSA, but there is potential for this approach of harnessing plant-soil interactions to be applied to other crops and for other local contexts as a new frontier within integrated soil fertility management. Molecular breeding approaches are now being deployed in SSA maize breeding pipelines for improving complex traits, opening up new opportunities to expand breeding target traits beyond the current focus on yield to increase traits associated with the acquisition of mineral N, and ultimately make fertilizer use more viable. Improvement of SOM mineralization and BNI with nutrient acquisition traits has significant positive impact on both host plant performance and soil health. Therefore, although phenotyping these traits is challenging and may be slow in terms of demonstrable impact on the environment, their improvement provides immediate benefits for underpinning sustainable production, justifying prioritisation.
Data availability statement
The original contributions presented in the study are included in the article. Further inquiries can be directed to the corresponding author.
Author contributions
The conception of this manuscript was led by EB, JC, MG, EP and CT, and all authors contributed to writing the text. All authors contributed to the article and approved the submitted version.
Funding
The authors are grateful for funding from the UK Global Challenges Research Fund administered by the Biotechnology and Biological Sciences Research Council [BB/P022936/1] and CGIAR Research Program on Maize (MAIZE), which receives support from the Governments of Australia, Belgium, Canada, China, France, India, Japan, Korea, Mexico, Netherlands, New Zealand, Norway, Sweden, Switzerland, U.K., U.S.A, and the World Bank and the Bill & Melinda Gates Foundation, USAID and FFAR project AG2MW (Accelerating Genetic Gains in Maize and Wheat for Improved Livelihoods, B&MGF Investment ID INV-003439). This work was carried out with support from the CGIAR research initiatives Accelerated Breeding Initiative and the Ukama/Ustawi Regional Initiative. We would like to thank all funders who supported this research through their contributions to the CGIAR Trust Fund. The James Hutton Institute receives funding support from the Rural and Environment Science and Analytical Services Division of the Scottish Government. For the purpose of open access, the authors have applied a CC-BY public copyright license to any Author Accepted Manuscript version arising from this submission.
Conflict of interest
The authors declare that the research was conducted in the absence of any commercial or financial relationships that could be construed as a potential conflict of interest.
Publisher’s note
All claims expressed in this article are solely those of the authors and do not necessarily represent those of their affiliated organizations, or those of the publisher, the editors and the reviewers. Any product that may be evaluated in this article, or claim that may be made by its manufacturer, is not guaranteed or endorsed by the publisher.
References
1. Godfray HCJ, Beddington JR, Crute IR, Haddad L, Lawrence D, Muir JF, et al. Food security: The challenge of feeding 9 billion people. Science (2010) 327:812–8. doi: 10.1126/science.1185383
2. Wheeler T, von Braun J. Climate change impacts on global food security. Science (2013) 341 (6145):508–13. doi: 10.1126/science.1239402
3. Komarek AM, Thierfelder C, Steward PR. Conservation agriculture improves adaptive capacity of cropping systems to climate stress in Malawi. Agric Syst (2021) 190:103117. doi: 10.1016/j.agsy.2021.103117
4. Zingore S, Mutegi JK, Agesa B, Tamene L, Kihara J. Soil degradation in sub-Saharan Africa and crop production options for soil rehabilitation. Better Crops (2015) 99:24–6.
5. Morris ML, Kelly VA, Kopicki RJ, Byerlee D. Fertilizer use in African agriculture: Lessons learned and good practice guidelines. World Bank Publications Rep (2007) 39037:1–162. doi: 10.1596/978-0-8213-6880-0
6. Thierfelder C, Paterson E, Mwafulirwa L, Daniell TJ, Cairns JE, Mhlanga B, et al. Toward greater sustainability: How investing in soil health may enhance maize productivity in southern Africa. Renew. Agric Food Syst (2021) 37:1–12. doi: 10.1017/S1742170521000442
7. Vanlauwe B, Wendt J, Giller KE, Corbeels M, Gerard B, Nolte C. A fourth principle is required to define conservation agriculture in sub-Saharan Africa: The appropriate use of fertilizer to enhance crop productivity. Field Crops Res (2014) 155:10–3. doi: 10.1016/j.fcr.2013.10.002
8. Sheahan M, Barrett CB. Ten striking facts about agricultural input use in Sub-Saharan Africa. Food Policy (2017) 67:12–25. doi: 10.1016/j.foodpol.2016.09.010
9. Holden ST. Fertilizer and sustainable intensification in Sub-Saharan Africa. Glob Food Sec. (2018) 18:20–6. doi: 10.1016/j.gfs.2018.07.001
10. Bonilla Cedrez C, Chamberlin J, Guo Z, Hijmans RJ. Spatial variation in fertilizer prices in Sub-Saharan Africa. PloS One (2020) 15(1):e0227764. doi: 10.1371/journal.pone.0227764
11. Li S-X, Wang Z-H, Malhi SS, Li S-Q, Gao Y-J, Tian X-H. Chapter 7: Nutrient and water management effects on crop production, and nutrient and water use efficiency in dryland areas of China. Adv Agron (2009) 102:223–65. doi: 10.1016/S0065-2113(09)01007-4
12. Waraich EA, Ahmad R, Ashraf MY, Saifullah, Ahmad M. Improving agricultural water use efficiency by nutrient management in crop plants. Acta Agric Scand Sect. B-Soil Plant Sci (2011) 61:291–304. doi: 10.1080/09064710.2010.491954
13. Hatfield JL, Dold C. Water-use efficiency: Advances and challenges in a changing climate. Front Plant Sci (2019) 10:1–14. doi: 10.3389/fpls.2019.00103
14. Mwafulirwa L, Paterson E, Cairns JE, Daniell TJ, Thierfelder C, Baggs EM. Genotypic variation in maize (Zea mays) influences rates of soil organic matter mineralisation and gross nitrification. New Phytol (2021) 231:2015–28. doi: 10.1111/nph.17537
15. Leitner S, Pelster DE, Werner C, Merbold L, Baggs EM, Mapanda F, et al. Closing maize yield gaps in sub-Saharan Africa will boost soil N2O emissions. Curr Opin Environ Sustain (2020) 47:95–105. doi: 10.1016/j.cosust.2020.08.018
16. Cairns JE, Chamberlin J, Rutsaert P, Voss RC, Ndhlela T, Magorokosho C. Challenges for sustainable maize production of smallholder farmers in sub-Saharan Africa. J Cereal Sci (2021) 101:103274. doi: 10.1016/j.jcs.2021.103274
17. Angel R, Conrad R, Dvorsky M, Kopecky M, Kotilínek M, Hiiesalu I, et al. The root-associated microbial community of the world’s highest growing vascular plants. Microb Ecol (2016) 72:394–406. doi: 10.1007/s00248-016-0779-8
18. Zhang F, Niu X, Zhang Y, Xie R, Liu X, Li S, et al. Studies on the root characteristics of maize varieties of different eras. J Integr Agric (2013) 12:426–35. doi: 10.1016/S2095-3119(13)60243-9
19. Paterson E. Importance of rhizodeposition in the coupling of plant and microbial productivity. Eur J Soil Sci (2003) 54:741–50. doi: 10.1046/j.1351-0754.2003.0557.x
20. Paterson E, Gebbing T, Abel C, Sim A, Telfer G. Rhizodeposition shapes rhizosphere microbial community structure in organic soil. New Phytol (2007) 173:600–10. doi: 10.1111/j.1469-8137.2006.01931.x
21. Dennis PG, Miller AJ, Hirsch PR. Are root exudates more important than other sources of rhizodeposits in structuring rhizosphere bacterial communities? FEMS Microbiol Ecol (2010) 72:313–27. doi: 10.1111/j.1574-6941.2010.00860.x
22. Kuzyakov Y, Friedel JK, Stahr K. Review of mechanisms and quantification of priming effects. Soil Biol Biochem (2000) 32:1485–98. doi: 10.1016/S0038-0717(00)00084-5
23. George TS, Hawes C, Newton AC, McKenzie BM, Hallett PD, Valentine TA. Field phenotyping and long-term platforms to characterise how crop genotypes interact with soil processes and the environment. Agronomy (2014) 4:242–78. doi: 10.3390/agronomy4020242
24. Delhaize E, Rathjen TM, Cavanagh CR. The genetics of rhizosheath size in a multiparent mapping population of wheat. J Exp Bot (2015) 66:4527–36. doi: 10.1093/jxb/erv223
25. Mwafulirwa L, Baggs EM, Russell J, George T, Morley N, Sim A, et al. Barley genotype influences stabilization of rhizodeposition-derived C and soil organic matter mineralization. Soil Biol Biochem (2016) 95:60–9. doi: 10.1016/j.soilbio.2015.12.011
26. Yin L, Corneo PE, Richter A, Wang P, Cheng W, Dijkstra FA. Variation in rhizosphere priming and microbial growth and carbon use efficiency caused by wheat genotypes and temperatures. Soil Biol Biochem (2019) 134:54–61. doi: 10.1016/j.soilbio.2019.03.019
27. Dijkstra F, Carrillo Y, Pendall E, Morgan J. Rhizosphere priming: a nutrient perspective. Front Microbiol (2013) 4:216. doi: 10.3389/fmicb.2013.00216
28. Gowda M, Cairns JE, Mwafulirwa L, Paterson E, Thierfelder C, Daniell TJ, et al. Maize root traits to influence the rate of soil organic matter mineralization and gross nitrification can be incorporated in breeding. Soil Biol Biochem (2021) 161:108402. doi: 10.1016/j.soilbio.2021.108402
29. Mwafulirwa L, Baggs EM, Russell J, Hackett CA, Morley N, de la Fuente Cantó C, et al. Identification of barley genetic regions influencing plant–microbe interactions and carbon cycling in soil. Plant Soil (2021) 468:165–82. doi: 10.1007/s11104-021-05113-6
30. Brown LK, George TS, Neugebauer K, White PJ. The rhizosheath – a potential trait for future agricultural sustainability occurs in orders throughout the angiosperms. Plant Soil (2017) 418:115–28. doi: 10.1007/s11104-017-3220-2
31. Comas L, Becker S, Cruz VM, Byrne PF, Dierig DA. Root traits contributing to plant productivity under drought. Front Plant Sci (2013) 4. doi: 10.3389/fpls.2013.00442
32. Nazari M, Riebeling S, Banfield CC, Akale A, Crosta M, Mason-Jones K, et al. Mucilage polysaccharide composition and exudation in maize from contrasting climatic regions. Front Plant Sci (2020) 11:587610. doi: 10.3389/fpls.2020.587610
33. Rasmann S, Hiltpold I. Root exudation of specialized molecules for plant-environmental interaction. Chimia (2022) 76:922–7. doi: 10.2533/chimia.2022.922
34. Xie L, Bi Y, Ma S, Shang J, Hu Q, Christie P. Combined inoculation with dark septate endophytes and arbuscular mycorrhizal fungi: Synergistic or competitive growth effects on maize? BMC Plant Biol (2021) 21:498. doi: 10.1186/s12870-021-03267-0
35. Badji A, Diedhiou I, Fall AF. Climate-smart maize breeding: The potential of arbuscular mycorrhizal symbiosis in improving yield, biotic and abiotic stress resistance, and carbon and nitrogen sink efficiency. In: El-Esawi MA, editor. Maize genetic resources. Rijeka: IntechOpen (2022). p. 1–32.
36. Bonfante P, Genre A. Mechanisms underlying beneficial plant–fungus interactions in mycorrhizal symbiosis. Nat Commun (2010) 1:48. doi: 10.1038/ncomms1046
37. Pasley HR, Camberato JJ, Cairns JE, Zaman-Allah M, Das B, Vyn TJ. Nitrogen rate impacts on tropical maize nitrogen use efficiency and soil nitrogen depletion in eastern and southern Africa. Nutr Cycling Agroecosyst. (2020) 116:397–408. doi: 10.1007/s10705-020-10049-x
38. Coskun D, Britto DT, Shi W, Kronzucker HJ. Nitrogen transformations in modern agriculture and the role of biological nitrification inhibition. Nat Plants (2017) 3(6):1–10. doi: 10.1038/nplants.2017.74
39. Coskun D, Britto DT, Shi W, Kronzucker HJ. How plant root exudates shape the nitrogen cycle. Trends Plant Sci (2017) 22(8):661–73. doi: 10.1016/j.tplants.2017.05.004
40. Sasse J, Martinoia E, Northen T. Feed your friends: do plant exudates shape the root microbiome? Trends Plant Sci (2018) 23:25–41. doi: 10.1016/j.tplants.2017.09.003
41. Subbarao GV, Nakahara K, Ishikawa T, Ono H, Yoshida M, Yoshihashi T, et al. Biological nitrification inhibition (BNI) activity in sorghum and its characterization. Plant Soil (2013) 366:243–59. doi: 10.1007/s11104-012-1419-9
42. Egenolf K, Verma S, Schöne J, Klaiber I, Arango J, Cadisch G, et al. Rhizosphere pH and cation-anion balance determine the exudation of nitrification inhibitor 3-epi-brachialactone suggesting release via secondary transport. Physiol Plant (2021) 172(1):116–23. doi: 10.1111/ppl.13300
43. Subbarao GV, Kishii M, Bozal-Leorri A, Ortiz-Monasterio I, Gao X, Ibba MI, et al. Enlisting wild grass genes to combat nitrification in wheat farming: A nature-based solution. PNAS (2021) 118(35):e2106595118. doi: 10.1073/pnas.2106595118
44. Byrnes RC, Nùñez J, Arenas L, Rao I, Trujillo C, Alvarez C, et al. Biological nitrification inhibition by brachiaria grasses mitigates soil nitrous oxide emissions from bovine urine patches. Soil Biol Biochem (2017) 107:156–63. doi: 10.1016/j.soilbio.2016.12.029
45. Karwat H, Egenolf K, Nuñez J, Rao I, Rasche F, Arango J, et al. Low 15N natural abundance in shoot tissue of Brachiaria humidicola is an indicator of reduced N losses due to biological nitrification inhibition (BNI). Front Microbiol (2018) 22:2383. doi: 10.3389/fmicb.2018.02383
46. Karwat H, Moreta D, Arango J, Núñez J, Rao I, Rincón A, et al. Residual effect of BNI by brachiaria humidicola pasture on nitrogen recovery and grain yield of subsequent maize. Plant Soil (2017) 420:389–406. doi: 10.1007/s11104-017-3381-z
47. Otaka J, Subbarao GV, Ono H, Yoshihashi T. Biological nitrification inhibition in maize–isolation and identification of hydrophobic inhibitors from root exudates. Biol Fertil. Soils (2021) 58:251–64. doi: 10.1007/s00374-021-01577-x
48. Lata JC, Le Roux X, Koffi KF, Yé L, Srikanthasamy T, Konaré S, et al. The causes of the selection of biological nitrification inhibition (BNI) in relation to ecosystem functioning and a research agenda to explore them. Biol Fertil. Soils (2022) 58:207–24. doi: 10.1007/s00374-022-01630-3
49. Subbarao GV, Searchinger TD. Opinion: A “more ammonium solution” to mitigate nitrogen pollution and boost crop yields. PNAS (2021) 118(22). doi: 10.1073/pnas.2107576118
50. Pauw EFD. The management of acid soils in Africa. Outlook Agric (1994) 23:11–6. doi: 10.1177/003072709402300104
51. Barros VA, Chandnani R, de Sousa SM, Maciel LS, Tokizawa M, Guimareas CT, et al. Root adaptation via common genetic factors conditioning tolerance to multiple stresses for crops cultivated on acidic tropical soils. Front Plant Sci (2020) 11:565339. doi: 10.3389/fpls.2020.565339
52. Rao IM, Miles JW, Beebe SE, Horst WJ. Root adaptations to soils with low fertility and aluminium toxicity. Ann Bot (2016) 118:593–605. doi: 10.1093/aob/mcw073
53. Ding J, Yang W, Hu X, Yan W, Hu W, Li H, et al. Large-Scale evaluation of aluminium tolerance in maize (Zea mays l.) accessions. Theor Exp Plant Physiol (2022) 34:155–69. doi: 10.1007/s40626-022-00238-0
54. Du H, Raman H, Kawasaki A, Perera G, Diffey S, Snowdon R, et al. A genome-wide association study (GWAS) identifies multiple loci linked with the natural variation for Al3+ resistance in Brassica napus. Func. Plant Biol (2022) 49:845–60. doi: 10.1071/FP22073
55. Prasanna BM, Cairns JE, Zaidi PH, Beyene Y, Makumbi D, Gowda M, et al. Beat the stress: Breeding for climate resilience in maize for the tropical rainfed environments. Theor Appl Genet (2021) 134:1729–52. doi: 10.1007/s00122-021-03773-7
56. Chivasa W, Worku M, Teklewold A, Setimela P, Gethi J, Magorokosho C, et al. Maize varietal replacement in Eastern and southern Africa: Bottlenecks, drivers and strategies for improvement. Glob Food Sec. (2022) 32:100589. doi: 10.1016/j.gfs.2021.100589
57. Ober ES, Alahmad S, Cockram J, Forestan C, Hickey LT, Kant J, et al. Wheat root systems as a breeding target for climate resilience. Theor Appl Genet (2021) 134:1645–62. doi: 10.1007/s00122-021-03819-w
58. Fradgley N, Evans G, Biernaskie J, Cockram J, Marr EC, Oliver AG, et al. Effects of breeding history and crop management on the root architecture of wheat. Plant Soil (2020) 452:587–600. doi: 10.1007/s11104-020-04585-2
59. Prasanna BM, Burgueño J, Beyene Y, Makumbi D, Asea G, Woyengo V, et al. Genetic trends in CIMMYT’s tropical maize breeding pipelines. Sci Rep (2022) 12:20110. doi: 10.1038/s41598-022-24536-4
60. Peiffer JA, Spor A, Koren O, Jin Z, Tringe SG, Dangl JL, et al. Diversity and heritability of the maize rhizosphere microbiome under field conditions. PNAS (2013) 110:6548–53. doi: 10.1073/pnas.1302837110
61. Aira M, Gómez-Brandón M, Lazcano C, Bååth E, Domínguez J. Plant genotype strongly modifies the structure and growth of maize rhizosphere microbial communities. Soil Biol Biochem (2010) 42:2276–81. doi: 10.1016/j.soilbio.2010.08.029
62. Bouffaud M-L, Kyselková M, Gouesnard B, Grundmann G, Muller D, Moënne-Loccoz Y. Is diversification history of maize influencing selection of soil bacteria by roots? Mol Ecol (2012) 21:195–206. doi: 10.1111/j.1365-294X.2011.05359.x
63. Deng S, Caddell DF, Xu G, Dahlen L, Washington L, Yang J, et al. Genome wide association study reveals plant loci controlling heritability of the rhizosphere microbiome. ISME J (2021) 15:3181–94. doi: 10.1038/s41396-021-00993-z
64. Bergelson J, Mittelstrass J, Horton MW. Characterizing both bacteria and fungi improves understanding of the arabidopsis root microbiome. Sci Rep (2019) 9:24. doi: 10.1038/s41598-018-37208-z
65. Meier MA, Xu G, Lopez-Guerrero MG, Li G, Smith C, Sigmon B, et al. Association analyses of host genetics, root-colonizing microbes, and plant phenotypes under different nitrogen conditions in maize. eLife (2022) 11:e75790. doi: 10.7554/eLife.75790.sa2
66. Ahmar S, Saeed S, Khan MHU, Ullah Khan S, Mora-Poblete F, Kamran M, et al. A revolution toward gene-editing technology and its application to crop improvement. IJMS (2020) 21:5665. doi: 10.3390/ijms21165665
67. Huang X, Hilscher J, Stoger E, Christou P, Zhu C. Modification of cereal plant architecture by genome editing to improve yields. Plant Cell Rep (2021) 40:953–78. doi: 10.1007/s00299-021-02668-7
68. Michno JM, Wang X, Liu J, Curtin SJ, Kono TJ, Stupar RM. CRISPR/Cas mutagenesis of soybean and Medicago truncatula using a new web-tool and a modified Cas9 enzyme. GM Crops Food (2015) 6:243–52. doi: 10.1080/21645698.2015.1106063
69. Wang Y, Meng Z, Liang C, Meng Z, Wang Y, Sun G, et al. Increased lateral root formation by CRISPR/Cas9-mediated editing of arginase genes in cotton. Sci China. Life Sci (2017) 60:524–7. doi: 10.1007/s11427-017-9031-y
Keywords: rhizodeposition, biological nitrification inhibition, maize breeding, root traits, plant-soil interactions, sub-Saharan Africa
Citation: Baggs EM, Cairns JE, Mhlanga B, Petroli CD, Chamberlin J, Karwat H, Kommerell V, Thierfelder C, Paterson E and Gowda MS (2023) Exploiting crop genotype-specific root-soil interactions to enhance agronomic efficiency. Front. Soil Sci. 3:1125604. doi: 10.3389/fsoil.2023.1125604
Received: 16 December 2022; Accepted: 16 March 2023;
Published: 30 March 2023.
Edited by:
Amanda Black, Lincoln University, New ZealandReviewed by:
Davey Jones, Bangor University, United KingdomCopyright © 2023 Baggs, Cairns, Mhlanga, Petroli, Chamberlin, Karwat, Kommerell, Thierfelder, Paterson and Gowda. This is an open-access article distributed under the terms of the Creative Commons Attribution License (CC BY). The use, distribution or reproduction in other forums is permitted, provided the original author(s) and the copyright owner(s) are credited and that the original publication in this journal is cited, in accordance with accepted academic practice. No use, distribution or reproduction is permitted which does not comply with these terms.
*Correspondence: Elizabeth M. Baggs, bGl6LmJhZ2dzQGVkLmFjLnVr