- 1SDG 2 (Zero Hunger) Research Group, Landmark University, Omuaran, Kwara State, Nigeria
- 2SDG 15 (Life on Land) Research Group, Landmark University, Omuaran, Kwara State, Nigeria
- 3Crop and Soil Science Department, Landmark University, Omuaran, Kwara State, Nigeria
- 4Department of Soil Science, Faculty of Agriculture/Institute for Agricultural Research, Ahmadu Bello University, Samaru – Zaria, Nigeria
- 5Department of Crop/Soil Science, Rivers State University, Port Harcourt, Rivers State, Nigeria
- 6Department of Soil Science and Land Management, Federal University of Agriculture, Abeokuta, Ogun State, Nigeria
Soils are polluted by both organic and inorganic substances. Plants growing in polluted soils suffer damages such as leaf rolls, chlorosis, growth inhibition, root tips browning, and death of plant. Soil pollutants such as hydrocarbon and heavy metals are absorbed by crops and such ends up being consumed by human posing health risk like cancer and respiratory abnormally. Conventional methods of remediation such as chemical and physical methods are very expensive and not sustainable. Excavation, which is a type of physical method, merely shifts the pollutant from one site to another. Bioremediation is a biological method of reclaiming polluted soils. Bioremediation is less expensive and more sustainable and safer when compared to the conventional methods of reclamation of polluted environment. This biological method of remediation is an extremely attractive, important, and productive alternative for cleaning, debugging, managing, and rehabilitating and consequently ameliorating contaminated environments via judicious utilization of microbial activities. The rate, at which the waste substances are degraded, is usually dictated by competitiveness among biological agents, sub-optimal supply of essential nutrients, unconducive abiotic conditions (in forms of temperature, aeration, pH, and moisture), and constrained pollutant’s bioavailability. Bioremediation is often effective only under conducive environmental conditions favorable for microbial growth and development. It has been successfully used at various parts of the world. Based on the significance of bioremediation in enhancing the reclamation of polluted environments by decontaminating and degrading heavy metals and xenobiotics, more focused researches would be needed so as to improve contaminated environments in much safer ways and conditions through bioremediation techniques. This research discussed the various types and methods of bioremediation. The mechanisms of actions and strategies of microorganisms in bioremediation were well expatiated. The interaction between bioremediators and the mineral particles in the soil environment was explained.
Introduction
Increase in global industrialization and intensive farming due to the ever increasing human population has resulted into pollution of the ecosystem including the soil (1). Soil pollutants have immunotoxic, carcinogenic, and mutagenic effects which causing changes to soil physical, chemical, and microbiological characteristics (2). These soil pollutants can also find their way into human food and water resulting in serious human, environmental, and soil health issues (3). It, therefore, becomes expedient for polluted soils to be recovered.
Several approaches such as incineration, excavation, and the use of chemicals have been employed to clean up polluted soils but these methods are too expensive and do not provide total cure as some just shifted the contamination from one site to another (4). Most of the times, they also result in production of secondary pollutants, which incur another negative impacts on the environment (5). This, therefore, necessitates the need for a safer and more cost-effective alternative method.
Bioremediation refers to cleaning of polluted lands using biological processes (5). It is an eco-friendly and highly economical method of remediating polluted soils or water (6). Biological method of remediation of polluted sites uses living organisms, which could be plant or microorganisms to abate or clean up pollutants. The use of plant is called phytoremediation. The mechanisms of bioremediation degrade, mineralize, transform, or detoxify the pollutants thereby reducing the concentration of the pollutant to innoxious state (6). Soil pollutants of environmental and health concern include hydrocarbons, heavy metals, chlorinated compounds, pesticides, dyes, and so on (2, 7). Table 1 discusses some of the soil pollutants, the microorganisms that had been used to remove, transform, or reduce their concentration in the soil, and their effect on crop growth and yield.
The growth and metabolism activities of the microbes responsible for bioremediation are enhanced or limited by environmental conditions, which include both soil and climatic conditions.
Several researchers (6, 21) studied and discussed types and methods of bioremediation and their effects on some crop growth and yield but there is little or no records of the impact of soil environment on the efficiency of bioremediation techniques. There is little or no literature on the interaction between bioremediators and the soil environment. The relationship that exists between the bioremediators and the soil minerals in a soil aggregate is scarcely reported. This research was aimed to discuss the various strategies of bioremediation and the interactions between the bioremediator and the mineral particles in the soil environment. To develop an efficient bioremediation system in the future, a good understanding of the factors that influence the natural selection of organisms capable of remediating the pollutant, the genomes, and proteasomes are necessary (22).
This article has, therefore, discussed the various techniques of bioremediation and how they are affected by environmental factors. The strategies and mechanism of actions of microorganisms in remediating polluted soils are extensively explained. The interaction and relationship between the bioremediators and the soil minerals in a soil aggregate were well explained. In addition, the knowledge that is required to develop a more efficient bioremediation system in the future was also highlighted.
Soil pollutants
Pollutants commonly found in soil are classified as organic and inorganic pollutants. Organic pollutants from industries are built up in soil. Anthropogenic activities contribute immensely to the pollution of soil (23). These anthropogenic activities include use of trichloroethylene by drycleaners, pesticides like atrazine, and fuels naphthalene. Some of these organic compounds are highly toxic in the environment (24).
Inorganic pollutants are non-biodegradable substances that may be disposed from industrial chemicals or waste, such as from refineries, pharmaceuticals factories, and even agricultural chemicals such as fertilizers, pesticides, and herbicides. Other inorganic pollutant include heavy metals (arsenic, cadmium, and copper), some trace elements, sulphates, mineral acids, metals, metal compounds, and some inorganic salts (1, 7). These inorganic pollutants persist in the surrounding environment causing health hazards (3, 7).
Bioremediation techniques
Bioremediation can be carried out outside the pollution site (ex-situ) or at the site of pollution (in-situ) (Figure 1). Choice of bioremediation technique depends on some factors that include nature of pollutant, concentration of pollutant, type of environment, cost of remediation technique, depth of contaminant, and environmental policies [1, 3, and 7]. Other abiotic/edaphic factors such as oxygen concentrations, temperature, pH, determine the success of bioremediation processes and hence could also considered (24, 25).
Types of bioremediations
Based on the place of application or where the process is carried out, bioremediation techniques can by grouped into Ex-situ and In-situ Bioremediations (Figure 1).
Ex-situ methods of bioremediation
Polluted soil is removed from site of pollution to another site for treatment. This method is considered when favored by the depth of pollution, concentration of pollutant, cost of treatment, pollutant type, and geographical location of the polluted site (26). The ex-situ technique can be carried using solids with bioremediators or slurry containing bioremediators (25).
Treatment with solids containing bioremediators
Solids containing bioremediators used in this technique include organic waste such as animal manures, leaves, domestic, industrial wastes, and so on. Polluted soil is excavated, placed into piles, and mixed with aforementioned solids containing bioremediators such as bacteria and fungi (26). Air or ventilation is allowed through the piles to enhance microbial respiration. This system of remediation requires large space and clean-up takes longer time to complete compare to the slurry containing bioremediator. Examples of the use of solids containing bioremediators include biopiles, windrows, land farming, composting, and the rest (27).
Treatment with slurry containing bioremediators
In this type of treatment, stones and rubbles in the excavated polluted soil are moved, and then the nutrient and the water are mixed with the polluted soil and oxygen in a bioreactor. This provides an enabling environmental condition for the soil microorganisms to thrive and break down the pollutant contained in the soil (26). After the degradation of the pollutant, soil is removed and dried with the aid of vacuum filters, pressure filters, and centrifuges. The cleaned soil is then deposited, while the resultant fluid is further treated. The rate of remediation by this process is faster compared to the other treatment processes. The soil properties and concentration of the pollutants determine the amount of water to be added to the polluted soil to initiate the degradation process (28).
Biopile
Polluted soil is excavated and parked to another site where they are pilled above ground (26) . The growth and activities of microbes present in the soil are then stimulated by the addition of air and nutrient (6). The biopile type of bioremediation is employed in the treatment of volatile, low molecular weight pollutants, and in extremely very cold environments (29–31). This system is preferred because it is cost effective. The rate of biodegradation is pH, nutrient, temperature, and aeration dependent (26). A heating system can be integrated into the biopile to increase microbial activities and hence increase the rate of biodegradation (32). Organic materials such as, straw, saw dust, bark, or wood chips may be added to enhance remediation process in a biopile site (33). Excessive heating of air may lead to excessive drying of the soil and this will inhibit microbial activities and, therefore, stimulate volatilization rather than biodegradation (26, 33).
Windrows
Piled polluted soil is rotated periodically to improve microbial degradation of pollutant in the soil (26). Windrows treatment when compared to biopile treatment is more effective in the removal of hydrocarbon from the soil (32). The choice of windrows should not be considered in the remediation of toxic volatiles compounds because of the periodic turning associated with windrow treatment (34).
Land farming
In this type of bioremediation technique, aerobic biodegradation of polluted soil is initiated by placing the polluted soils on a fixed layer above the ground surface (35). It could be carried out on the polluted site (in-situ) or at another location (ex-situ). Land farming technique favors large area of polluted soil because it requires low energy and capital and it is a very simple technique with minimal environmental impact (26, 36).
Composting
It can be employed for both ex-situ and in-situ bioremediation techniques. Organic material is added to the contaminated soil, this improves the porosity and air flow through the soil (26, 37). Energy is released during the degradation of the organic material resulting in temperature rise, which stimulate the activities of both mesophilic and thermophilic microorganisms (38). This was supported by AdeOluwa et al. (2015) (39), who reported that compost can have ameliorative effects on petroleum polluted soils and that the effects increased with the increase in the level of amendment.
Biodegradation of contaminants carried out ex-situ especially in a bioreactor is usually more manageable, controllable, and predictable (26). A wide range of soil pollutant can be removed ex-situ. More also, the suitability of ex-situ technique to remove a soil pollutant can be investigated from site data (6). However, ex-situ techniques will not efficiently remove heavy metals or chlorinated hydrocarbons from soil. Additional processing is required to employ ex-situ technique for remediation of contaminant from non-permeable soil. When contaminated soil is extracted and put in a bioreactor, the pollutant in the soil can be stripped off before the soil is placed in the bioreactor (26, 40).
In-Situ bioremediation techniques
With in-situ techniques, soil pollutants are removed right at the site of pollution. The polluted soil is treated right at the site of pollution and is, therefore, more cost-effective than the ex-situ bioremediation techniques (26) . Some in-situ bioremediation techniques are enhanced by microbes present in the polluted soil. In-situ bioremediation techniques have been effectively used to treat chlorinated solvents, heavy metals, dyes, and hydrocarbons polluted sites (17, 18, 41, 42).
Types of In-Situ bioremediation
In-situ bioremediation could be categorized into two types based on whether the bioremediator is within the polluted soil and is introduced from external source. These are intrinsic and engineered bioremediation.
Intrinsic bioremediation
The remediation is carried out by the stimulation of indigenous microbes of the polluted sites. This remediation could be by both aerobic and anaerobic processes. It does not require application of any external force of any sort; hence, the technique is less expensive compared to engineered techniques (26). It has the capacity to remove recalcitrant pollutant because of the anaerobic process that could also be involved (6).
Engineered In-Situ bioremediation
In this system of remediation, an external microorganism that is not indigenous to the polluted sites is introduced. The introduced microorganism improves the chemical and physical properties of the polluted site and, thereby, encouraged the growth of the indigenous microorganisms. This, therefore, accelerates the degradation process of the pollutants (43).
Bioventing
Bioventing is a technique use in in-situ bioremediation of polluted site whereby airflow is controlled to deliver oxygen to unsaturated zone that also called vadose zone. This increases the activities of indigenous microbes, thereby, enhancing biodegradation of pollutants. Nutrients and moisture can also be added to further enhance the activities of the microbes, which are responsible for microbial conversion of the pollutants to a harmless state (26, 44).
Bioslurping
In this technique, bioremediation is achieved by combining the strategies of vacuum-enhanced pumping and soil vapor extraction (45).The technique is commonly used for recovering of products from capillary, light non-aqueous phase liquids (LNAPLs), in saturated and unsaturated zones (26). Volatile and semi-volatile organic compounds can be removed by this technique (45). Via upward movement, the pumping machine transports LNAPLs to the surface. Here, the LNAPL becomes separated from air and water, hence, reduces microbial activities (25). This technique is less suitable for remediation in low-permeable soil, but the cost of operation procedure is lesser compared to some other in-situ techniques (44, 45).
Biosparging
In biosparging technique, microbial activities in polluted sites are improved by injection of air into the soil subsurface (26). This makes biosparging similar to bioventing. In bioventing high air-flow is required for volatilization of pollutant, whereas injected air in biosparging stimulates biodegradation. Soil permeability and pollutant biodegradability are two major factors that determine the success of biosparging technique (26).
Bioaugmentation
This technique is characterized by the increase of the native microbiota by the inoculation of exogenous microorganisms (46). Generally, the microorganisms used are bacteria, filamentous fungi, and yeasts.
Phytoremediation
Phytoremediation is the removal of pollutants from contaminated soil using plants (4). The success of this technique depends on the amount and nature of pollutant. Mechanisms of phytoremediation include phytoextraction, degradation, filtration, accumulation, stabilization, and volatilization. Extraction, transformation, and sequestration will effectively remove pollutants such as heavy metals and radionuclides. With the use of some plants, such as willow and alfalfa organic pollutants, hydrocarbons and chlorinated compounds are mostly removed by degradation and mineralization mechanisms (47, 48). Table 2 shows some plants (phytoremediators) and the pollutants that they removed. Some important characteristics that qualify a plant as a phytoremediator include root system (fibrous or tap), above ground biomass, toxicity of pollutant to plant, plant adaptability to predominant environmental conditions, and plant growth rate. More also, the plant must be resistant to diseases and pests (1, 62). In phytoremediation, removal of pollutant involve translocation from roots to shoots (46). Furthermore, the rate of translocation and accumulation depends on transpiration and partitioning (63). Most often, plants growing in any polluted site are good phytoremediators, hence, great success of phytoremediation is reported by improving the remediation potentials of native plants growing in polluted sites either by bioaugmentation with endogenous or exogenous plant. One of the major advantages of phytoremediation is the possibility to recover plant accumulated metals after remediation through a process called phytomining (46).
Permeable reactive barrier
This technique is sometimes refers to as a physical method for remediating contaminated groundwater. However, biological mechanisms such as precipitation, degradation, and sorption of pollutant are employed in permeable reactive barrier (PRB)method. Hence, this technique can be called biological PRB of bio-enhanced PRB or passive bio-reactive barrier. In general, PRB is an in-situ technique that is used for remediating heavy metals and chlorinated compounds in groundwater pollution (64).
The in-situ methods of bioremediation do not require excavation of the contaminated soil.It treats both dissolved and solid contaminants. The accelerated in-situ method of bioremediation will treat sub-surface pollution faster than the pump and treat processes. Via in-situ techniques, organic contaminants are transformed into innocuous substances such as carbon dioxide, water, and ethane. Since there is minimal site disruption, in-situ techniques are cost-effective (4, 44).
However, in in-situ techniques, some pollutants may not be completely transformed to harmless products depending on site characteristics. If it happens that transformation stops at an intermediate compound, the intermediate may be more toxic and/or mobile than the original compound. Some are recalcitrant contaminants that can no longer be biodegraded. In such an instance, the problem of the contaminated soil remains. In-situ bio-augmentation requires acclimatization of microorganism, which may not develop for pollutants such as oil spills and recalcitrant compounds. Activities of indigenous microorganisms may be inhibited by the concentration of pollutants. If not accurately applied, profuse microbial growth may block injection wells (6, 45).
The soil environment
Soil is the principal facilitator of terrestrial ecosystem and its particulate and colloidal composition, either in organic matter, minerals, or microorganisms form, which are not unconnected beings. They are, rather, continually interacting with one another in affecting the transformation and fate of inorganic and organic pollutants (65). Soil is a term that is understood by virtually everyone but being variously defined based on areas of specialization by chemists, farmers, geologists, engineers, and by laymen. However, Turgut et al. (2004) (51) considered soil as natural bodies that cover parts of the earth’s surface that is known to support plants’ growth and that have properties due to integration of the effects related to climate and organisms acting on parent material as conditioned by relief over a span of time. Soil is, therefore, the unconsolidated upper or superficial layer of the earth’s crust that is transformed by biological, chemical, and physical processes through weathering (66). It is composed of organic matter, mineral particles, air, water, and living beings apportioned in soil horizons. Soil’s health and quality, on the other hand, are the capability of a soil to continuously function as an essential system for conducive life process in a given ecosystem and land-use cycles so as to sustainably promote water and air quality; and biological productivity, thereby, consequently preserving human and even plant’s and animal’s health (67). Soil can, therefore, be put as an essential resource for life (66) as it plays varied roles in the provision of habitat, source of food and other materials, platform for growth, and other environmental interactions.
Soil pollution, on the other hand, generally means the presence of a chemical substance or compound that is foreign and/or at higher concentration in the soil environment that poses adverse threats to un-targeted organisms (68). This menace is often not easily perceived visually or assessed directly, which makes it a hidden but real danger, as exclaimed by Eugenio (2018) (69).
Many governments had, by the late 1970s, banned the production of such chemicals as polychlorinated biphenyls (PCBs), yet a serious environmental pollution and/or contamination still persists (68) consequent to deliberate and/or accidental spills/leaks of solvents or petroleum products following improper disposal, transport, or storage (69, 70). Some of the disposal practices that was acceptable in the past, due to lack of proper understanding of their harmful effects, have also resulted in contaminated soils today. Persistent organic pollutants (POPs) within the environment, for example, will negatively affect food production and human and environmental health as stated by (71). Therefore, soil is the major sink for most persistent pollutants in the environment. Soil environmental changes can, however, change the rates of apportionment of POPs within the soil.
Naturally occurring compounds in soils, such as metals and petroleum seeps, may also be very problematic. Contaminants released at the surface can be transported vertically and laterally into surface and ground waters and eventually ingested by both private and municipal water users, thereby, representing fatal human health risks, among others. Adsorbed contaminants into soil can be directly ingested or inhaled by humans. They can also be taken up by plants, accumulated in animal tissues, and be eventually found in the foods ingested by the human kinds. Literally, there exist thousands of chemicals used in industry and agriculture for human benefit. As such, there are diverse types of compounds that become lethal soil contaminants. Some of such common pollutants and classes of contaminants include the following: Benzene, toluene, ethylbenzene, and xylene (BTEX). Specific components of petroleum and petroleum–related compounds that is carcinogenic and relatively mobile in soil. These compounds can fortunately be microbially degraded both aerobically and anaerobically by adjusting the in-situ conditions to promote BTEX degradation. Methyl tertiary–butyl ether (MTBE) a chemical compound known as oxygenate, which is used as a petrol additive in order to increase octane rating since 1979 as a substitute to the dangerous use of lead (Pb). Aerobic and anaerobic MTBE transformations have recently been identified, although it was initially considered as too recalcitrant for bioremediation. Polycyclic aromatic hydrocarbons (PAHs) present in many petroleum–based compounds, which are less mobile than BTEX compounds and tend to remain near the soil surface. Many PAHs are carcinogenic and more recalcitrant than BTEX. Polychlorinated biphenyls (PCB) commonly occur in mixtures of compounds called congeners, which consist of different arrangements of chlorine on the biphenyl structure resulting in mixtures classified as arocolor, many of which are carcinogenic and also recalcitrant. Chlorinated solvents such as trichloroethylene (TCE) and tetrachloroethylene (PCE), for long being used as cleaning agents, are typically found as groundwater contaminants and pose a serious health threat. The redox potential of affected soil determines the ease of biodegradation of chlorinated solvents. The initial biotransformation steps are usually favored in reduced environments, yet subsequent transformations are often favored by aerobic processes. Other soil contaminants include energetics and explosives, such as 2, 4, 6–trinitrotoluene, and hexahydro–1, 3, 5–trinitro–1, 3, 5–trizine (RDX); metals such as chromium (Cr) and lead (Pb); and radionuclides such as plutonium (Pu) and uranium (U), and inorganic compounds include N and P.
The role of microorganisms in bioremediation
The quality of life on Earth is linked to a large extent to the overall quality of the environment. A large amount of wastes from agriculture, industries, and other activities of man, ranging from raw sewage to nuclear waste, are let out or dumped into the ecosystem (Soil, water and air), further posing serious problems for the survival of mankind itself on Earth (3). Traditional disposal of waste was done by digging a hole and filling it with waste material in the past. This method of waste disposal was difficult to sustain due to lack of new dumpsites each time the former is exhausted. New technologies for waste disposal that use high-temperature incineration and chemical decomposition (e.g., base-catalyzed dichlorination, and ultra-violent oxidation) evolved. Though this can not only be greatly effective at reducing a wide range of contaminants but at the same time have several disadvantages. These methods are complex, uneconomical, and are not acceptable generally. The related disadvantages of these methods have channeled efforts towards harnessing modern-day bioremediation processes as a suitable alternative (72).
In recent times, where the whole world’s ecosystem is faced with the problem of various types of environmental pollution, microorganisms are essential for a solution to overcome these challenges. According to Abatenh (2017) (73), the multiple functions of microorganism’s chemical wastes and physical hazardous materials can be degraded, eradicated, immobilized, or detoxified. Some microorganisms obtain energy by converting, modifying, and utilizing toxic pollutants for biomass production (74).
Mineralization is a type of biodegradation and is defined as the conversion of an organic substance to its inorganic constituents, rendering the original compound harmless. According to (75), bioremediation involves three major interactions between three factors, namely, substrate (pollutant), organisms, and the environment. The interactions of these factors affect biodegradability, bioavailability, and physiological requirements, which determine the success of bioremediation. He opined further that biodegradability of a chemical is determined by the presence or absence of organisms that are able to breakdown a chemical of interest and how widespread these organisms are in that site. The pollutant can interact with its surrounding to change its availability to organisms that can degrade it. For instance, pollutant has low bioavailability if it is tightly bound to soil organic matter or adsorb by soil aggregates. Physiological factors required by organisms to carry out bioremediation in the environment include nutrient availability, optimal pH, and availability of electron acceptors, such as oxygen, nitrate, and conducive, and also climatic environment (1).
Microbial enzyme activity in bioremediation
Enzymes are biological substances also known as catalysts that provide favorable conditions that lower the activation energy of a reaction to enhance the conversion of substrates into products. Enzymes are proteins or glycoproteins. They consist of at least one polypeptide moiety. The regions called the active sites are directly involved in the catalytic process. The detoxification of toxic organic compounds by various bacteria and fungi and higher plants through oxidative reaction is mediated with oxidoreductases. Microbes extract energy through energy-yielding biochemical reactions mediated by these enzymes to cleave chemical bonds and to assist the transfer of electrons from a reduced organic substrate (donor) to another chemical compound (acceptor). The oxidation-reduction reactions oxidize the contaminants to harmless compounds (75).
The oxidoreductases take part in the humification of various phenolic substances that are produced from the breakdown of lignin in a soil environment. Likewise, Dias et al. (2015) (64) reported that toxic xenobiotics, such as phenolic or anilinic compounds, can be detoxify via oxidoreductases by binding to humic substances or by polymerizing and copolymerizing with other substrates. Oxygenases play major roles in the metabolism of organic compounds by increasing their reactiveness, water solubility, or ability to cleave to the aromatic ring. Oxygenases have a wide substrate range and are active against a wide range of compounds, including the chlorinated aliphatics. Generally, the introduction of Oxygen (O2) atoms into the organic molecule by oxygenase results in cleavage of the aromatic rings. According to Chen et al. (2019) (65), the most studied enzymes in bioremediation are bacterial mono- or di-oxygenases. Mono-oxygenases have high region-selectivity and stereoselectivity on wide range of substrates and, therefore, act as biocatalysts in bioremediation process and synthetic chemistry. Most mono-oxygenase has cofactor, but there are certain mono-oxygenases that function independent of a cofactor. These enzymes use the substrate as reducing agent and molecular oxygen for their activities. Multicomponent enzyme systems that introduce molecular oxygen into their substrate are called dioxygenases. Aromatic hydrocarbon dioxygenases belong to a large family of Rieske nonheme iron oxygenases (76).
Hydrolytic enzymes also break major chemical bonds in the toxic molecules causing reduction of their toxicity. Oil spill, organophosphate, and carbamate insecticides are, in most times, effectively degraded by this mechanism. Amylases, proteases, lipases, DNases, pullulanases, and xylanases are examples of extracellular hydrolytic enzymes use for diverse purposes in different areas. The hemicellulase, cellulase, and glycosidase are of much importance due to its application in biomass degradation (75).
Effects of soil environment on bioremediator/bioremediation techniques
Before any bioremediation technique to be successful, the methods of bioremediation to be employed should depend on the right microbes in the right place and with the right environmental factors for a judicious degradation to occur. The right microorganisms are bacteria and/or fungi, which have the physiological and metabolic capabilities to degrade pollutants.
Bioremediation, a scientific and an intensive procedure, must be directed towards a site-specific condition. Observing the site characteristics is necessary to know whether the biodegradation will take place at the site or not (65).
Bioremediation process is influenced according to the activities of heterotrophic microorganisms, both aerobic and anaerobic. The activity of microorganisms in the soil is affected by a number of physical and chemical environmental specifications. Factors that affect bioremediation directly are temperature, electron donors (as source of energy), pH, nutrients electron acceptors, and inhibitory metabolites or substrates (77). One of the most important disparities between the surface soil, groundwater sediments, and vadose zone soil is their organic materials composition. Hence, surface soil usually have a more preponderant organic matter content, which typically receive regular inputs of organic materials from plants, and an advanced presence of microorganisms, vis-à-vis their abundant population diversity. Organic matter stores carbon (C) and energy and is a source of such other macronutrients as nitrogen (N), phosphorous (P), and sulphur (S). The sub-surface soils and ground water sediments, on the other hand, have lower statuses of the organic matter and, consequently, lower levels of microbial population and diversity than their surface soils counterparts (78). This is very attributable to the bacterial ability to utilize alternative electron acceptors other than oxygen. Other established factors controlling the populations of microorganisms are temperature, dissolved oxygen, and moisture content.
Among the most common bioremediation techniques are bioaugmentation (79), biostimulation (80), composting (81) bioventing (82), bioleaching (83), bioreactor (84) and farming (85), phytoremediation, and rhizofiltration (86). Bacteria are most often highly dominant in the community of microbes with an increase in soil depth along the soil profile than the populations of such other organisms such as actinomycetes or fungi, which decrease.
A primary metabolic process in organic compounds is defined as a use of a substrate as C and energy source. The substrate functions as electron donor, which results in the growth of microbes. Hence, the use of co-metabolism to site-specific amelioration of xenobiotics polluted environments is required. Co-metabolism refers to the metabolism of a non-carbon/energy producing compound. Aerobic process is featured by metabolic activities that involve reactant oxygen. Mono-oxygenases and di-oxygenases are two enzymes out of the primary ones employed by aerobic microbes in the process of transforming and mineralizing of xenobiotics (77). The anaerobic microorganisms, therefore, take advantage of an array of electron acceptors, which include Mn, Fe, SO4, CO2, and NO3, depending on prevailing redox conditions and availability.
Interaction between bioremediators and the mineral particles in the soil environment
Bacterial cells (the most populous among the bioremediators) with coat of extracellular polysaccharides (EPS) are enveloped by clay particles. The pore space where clay particles and bacteria interact, bound by silt- and sand particles, is relatively enriched with organic matter including EPS residues as illustrated in Figure 2. Fungal hyphae are attached to the outside surface of an aggregate (87).
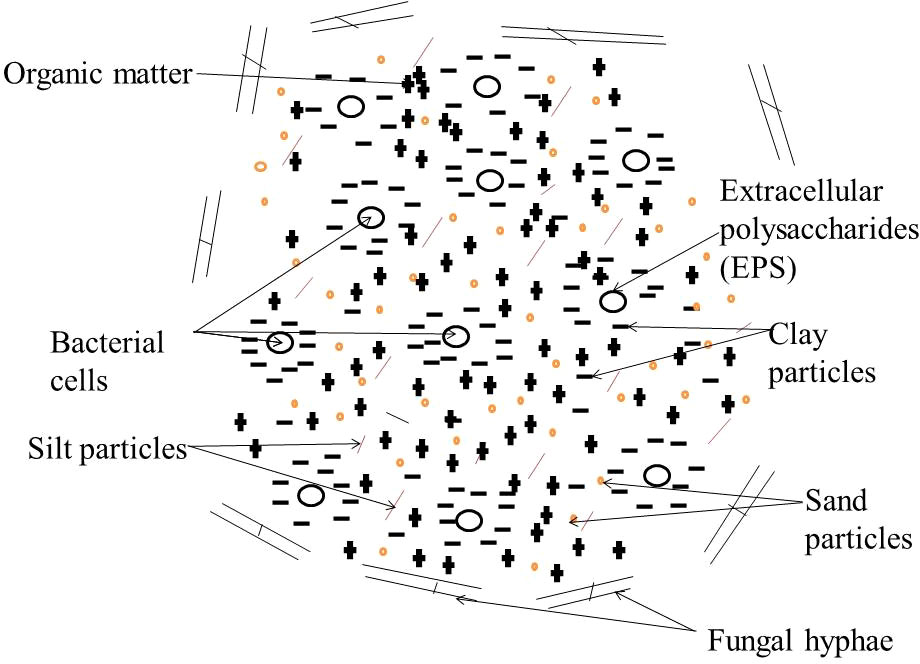
Figure 2 Illustration of the interaction between bioremediators with mineral particles in the soil environment.
Factors affecting bioremediation
Among the major variables that affect microbial activity is the ability of the microbes to reduce organic materials to serve as sources of energy. An average oxidation state of C in a contaminant serves as an effective source of energy for an aerobic heterotrophic organism. Higher oxidation state, therefore, corresponds to a lower energy yield, which, therefore, provides less energy outcome for microbial degradation. The result of any biodegradation practice hinges on microbial (population diversity, enzyme activities, and biomass concentration), substrate (physical and chemical characteristics, molecular concentration, and structure), and an array of environmental factors (pH, moisture content, temperature, EC, availability of electron acceptors and carbon, and energy sources). These parameters influence the adaptation period of the microorganisms to the given substrate. The contaminants concentration and molecular structure are known to strongly influence bioremediation practicability and the type of microbial transfiguration that occurs, and whether or not the compound will serve as a primary, secondary, or co-metabolic substrate (88).
The rate at which a contaminant is taken, metabolized and transferred to the cell (i.e., mass transfer) depends on the rate at which microbial cells can readily convert contaminants during bioremediation process. Boopathy and Manning (1998) (28) explained that an increase in microbial conversion capabilities do not necessarily lead to a higher rate of biotransformation when mass transfer is a limiting factor. Biodegradation is drastically stimulated by mixing the soil rigorously to break up the larger soil particles (89). The bioavailability of a contaminant is controlled by some physical and chemical processes such as sorption and desorption, diffusion, and dissolution. A slow rate of mass transfer from contaminants to the degrading microbes results in reduced bioavailability of contaminants to microbes in soil. When the rate of mass transfer is zero, the contaminants become absolutely unavailable to the microbes (77). The reduction in the bioavailability of pollutants in the course of time is called aging or weathering, and this can occur due to: i) chemical oxidation reactions incorporating contaminants into natural organic matter, ii) slow diffusion into very small pores and absorption into organic matter, and iii) formation of semi-rigid films around non-aqueous-phase liquids (NAPL) with a high resistance towards NAPL-water mass transfer. These bioavailability problems can be defeated in using food-grade surfactants (89), which increase the availability of contaminants for microbial degradation (28).
The term bioactivity is used to indicate the operating state of microbiological processes. Improving bioactivity implies that system conditions are adjusted to optimize biodegradation (90). For example, if the use of bioremediation requires meeting a certain minimum rate, adjustment of conditions to improve biodegradation activity becomes important and a bioremediation configuration that makes this control possible has an advantage over one that does not. In nature, the ability of organisms to transfer contaminants to both simpler and more complex molecules is very diverse. In light of our current limited ability to measure and control biochemical pathways in complex environments, favorable or unfavorable biochemical conversions are evaluated in terms of whether individual or groups of parent compounds are removed, whether increased toxicity is a result of the bioremediation process, and sometimes whether the elements in the parent compound are converted to measurable metabolites. These biochemical activities can be controlled in an in-situ operation when one can control and optimize the conditions to achieve a desirable result.
Future prospects
The future use of genetically engineered microorganisms (GEM) in enhancing bioremediation ability will be a favorable approach. This is because engineering a designer biocatalyst target pollutant, including recalcitrant compounds by combining a novel and efficient metabolic pathways, will widen the substrate range of existing pathways and will increase the stability of catabolic activity (91). More also, using parallel gene transfer and multiplication of GEM will be an encouraging approach. In addition, using derivative pathway of genetically engineered microorganisms with a target polluted compound will increase bioremediation efficiency. Nano materials can prevent microorganisms from the toxicity of pollutant because nano materials increase surface area and lower activation energy, this will reduce the time and cost of bioremediation (92). There is need for further understanding of the relevant genomic and proteomic sequences of bioremediators that exist in nature. The understanding of their diversity and evolutionary relationships existing between the bioremediator will help to develop a more efficient bioremediation system in the future (22).
Conclusion
Bioremediation is uses natural processes to remove, remediate, clean, manage, recover, or solve the problem of polluted soils. The rate of pollutant degradation is determined by competition between the biological agents (such as bacterial, fungi, and algae) nutrient availability, abiotic factors (aeration, moisture, pH, and temperature), and population of biological agents. Choice of bioremediation technique depends on several factors, which include but not limited to cost, site characteristics, type, and concentration of pollutants. The site characteristics help to identify the most suitable and promising bioremediation technique (ex-situ or in-situ). Ex-situ bioremediation techniques tend to be more costly due to excavation and transportation from polluted site. However, they will be wont to treat wider range of pollutants. In contrast, in-situ techniques have no extra cost for excavation; however, the cost of on-site installation of equipment is an additional challenge. More also, the need for effective measure to prevent the contamination of subsurface of polluted site can reduce effectiveness of in-situ bioremediation methods.
Increased knowledge of the response of microbial communities of polluted soil to the pollutant will enhance the identification of the right microbes from the polluted site with which field trials for bioremediation can be conducted and this understanding will improve the capability to biodegrade the pollutant.
Author contributions
All authors listed have made a substantial, direct, and intellectual contribution to the work and approved it for publication.
Conflict of interest
The authors declare that the research was conducted in the absence of any commercial or financial relationships that could be construed as a potential conflict of interest.
Publisher’s Note
All claims expressed in this article are solely those of the authors and do not necessarily represent those of their affiliated organizations, or those of the publisher, the editors and the reviewers. Any product that may be evaluated in this article, or claim that may be made by its manufacturer, is not guaranteed or endorsed by the publisher.
References
1. Alori ET, Fawole OB. Microbial inoculants-assisted phytoremediation for sustainable soil management. In: Ansari AA, Gill R, Gill SS, Lanza GR, Newman L, editors. Phytoremediation: Management of environmental contaminants, vol. 5 . Switzerland: Springer International Publishing (2017). p. 3–17.
2. Sales da Silva IG, Gomes de Almeida FC, Padilha da Rocha e Silva NM, Casazza AA, Converti A, Sarubbo LA. Soil bioremediation: Overview of technologies and trends. Energies (2020) 13:4664. doi: 10.3390/en13184664
3. Alori ET, Babalola OO. Microbial inoculant for improving crop quality and human health. Front Microbiol (2018) 9:2213 1–12. doi: 10.3389/fmicb.2018.02213
4. Alori ET. Phytoremediation using microbial community. In: Ansari AA, Gill SS, Gill R, Lanza GR, Newman L, editors. Phytoremediation: Management of environmental contaminants, vol. 1&2 . Switzerland: Springer International Publishing (2015). p. 183–90.
5. Divya M, Aanand S, Srinivasan A, Ahilan B. Bioremediation – an eco-friendly tool for effluent treatment: A review. Inter J Appl Res (2015) 1(12):530–37. Available at: https://www.allresearchjournal.com/archives/2015/vol1issue12/PartH/1-12-41.pdf
6. Azubuike CC, Chikere CB, Okpokwasili GC. Bioremediation techniques–classification based on site of application: principles, advantages, limitations and prospects. World J Microbiol Biotechnol (2016) 32:180. doi: 10.1007/s11274-016-2137-x
7. Alori ET, Dare MO, Babalola OO. Microbial inoculants for soil quality and plant health. In: Lichtfouse E, editor. Sustainable agriculture review, vol. 22. Switzerland: Springer Cham (2017). p. 281–308.
8. Infante JC, De Arco RD, Angulo ME. Removal of lead, mercury and nickel using the yeast. Rev MVZ Córdoba (2014) 19:4141–49. doi: 10.21897/rmvz.107
9. Thavasi R, Jayalakshmi S, Banat IM. Application of biosurfactant produced from peanut oil cake by Lactobacillus delbrueckii In biodegradation of crude oil. Bioresour Technol (2011) 102:3366–72. doi: 10.1016/j.biortech.2010.11.071
10. Montagnolli RN, Lopes PRM, Bidoia ED. Assessing Bacillus subtilis Biosurfactant effects on the biodegradation of petroleum products. Environ Monit Assess (2015) 187:1–17. doi: 10.1007/s10661-014-4116-8
11. Erika AW, Vivian B, Claudia C, Jorge FG. Biodegradation of phenol in static cultures by Penicillium chrysogenumerk1: catalytic abilities and residual phytotoxicity. Rev Argent Mcrobiol (2013) 44:113–21.
12. Lin C, Gan L, Chen ZL. Biodegradation of naphthalene by strain Bacillus fusiformis (BFN). J Hazard Mater (2010) 182:771–77. doi: 10.1016/j.jhazmat.2010.06.101
13. Hidayat A, Tachibana S. Biodegradation of aliphatic hydrocarbon in three types of crude oil Fusarium by Sp F092 under stress with artificial sea water. J Environ Sci Technol (2012) 5:64–73. doi: 10.3923/jest.2012.64.73
14. Kehinde FO, Isaac SA. Effectiveness of augmented consortia of Bacillus coagulans, citrobacter koseri and serratia ficaria in the degradation of diesel polluted soil supplemented with pig dung. Afr J Microbiol Res (2016) 10:1637–44. doi: 10.5897/AJMR2016.8249
15. Phulpoto H, Qazi MA, Mangi S, Ahmed S, Kanhar NA. Biodegradation of oil-based paint by bacillus species monocultures isolated from the paint warehouses. Int J Environ Sci Technol (2016) 13:125–34. doi: 10.1007/s13762-015-0851-9
16. Maulin P. Microbial degradation of textile dye (Remazol black b) by ETL-2012. J Appl Environ Microbiol (2013) 1:6–11. doi: 10.12691/jaem-1-1-2
17. Chen C, Wang JL. Characteristics of Zn2+ biosorption by Saccharomyces cerevisiae. Biomed Environ Sci (2007) 20(6):478–82.
18. Paranthaman SR, Karthikeyan B. Bioremediation of heavy metal in paper mill effluent using Spp. Int J Microbiol (2015) 1:1–5. Available at: microbiozjournals.com/bioremediation-of-heavy-metal-in-paper-mill-effluent-using-pseudomonas-spp/
19. Mirlahiji SG, Eisazadeh K. Bioremediation of uranium by. J Res Dev (2014) 1:52–8. Available at: https://www.arabianjbmr.com/pdfs/RD_VOL_1_12/3.pdf
20. Niu GL, Zhang JJ, Zhao S, Liu H, Boon N. Ning-yi zhou bioaugmentation of a 4- chloronitrobenzene contaminated soil with Pseudomonas putida ZWL73. Environ pollut (2009) 57:763–71. doi: 10.1016/j.envpol.2008.11.024
21. Popoola LK, Yusuff AS, Adeyi AA, Omotara OO. Bioaugmentation and biostimulation of crude oil contaminated soil: Process parameters influence. South Afr J Chem Engr (2022) 39:12–8 doi: 10.1016/j.sajce.2021.10.003
22. Patel AK, Singhania RR, Albarico FPJB, Pandey A, Chen C-W, Dong C-D. Organic wastes bioremediation and its changing prospects. Sci Total Environ (2022) 824: article153889 doi: 10.1016/j.scitotenv.2022.153889
23. Vidali M. Bioremediation: An overview. J Appl Chem (2001) 73(7):1163–72. doi: 10.1351/pac200173071163
24. Baric M, Pierro L, Pietrangeli B, Papini MP. Polyhydroxyalkanoate (PHB) as a slow-release electron donor for advanced in situ bioremediation of chlorinated solvent-contaminated aquifers. New Biotechnol (2014) 31:377–82. doi: 10.1016/j.nbt.2013.10.008
25. Banitz T, Frank K, Wick LY, Harms H, Johst K. Spatial metrics as indicators of biodegradation benefits from bacterial dispersal networks. Ecol Ind (2016) 60:54–63. doi: 10.1016/j.ecolind.2015.06.021
26. Sharma I. Bioremediation techniques for polluted environment: concept, advantages, limitations, and prospects. In: Murillo-Tovar MA, Saldarriaga-Norena HA, Saeid A, editors. Trace metals in the environment: New approaches and recent advances. London, SW7 2QJ UNITED KINGDOM: IntechOpen, (2020). doi: 10.5772/intechopen.90453
27. Barr D, Finnamore JR, Bardos RP, Weeks JM, Nathaniel CP. Biological methods for assessment and remediation of contaminated land: case studies. London: Construction Industry Research and Information Association (2002) p. 1–24. CIRIA 575.
28. Boopathy R, Manning J. A laboratory study of the bioremediation of 2, 4, 6-trinitrotoluene-contaminated soil using aerobic anaerobic soil slurry reactor. Water Environ Res (1998) 70:80–6. doi: 10.2175/106143098X126919
29. Burgess JE, Parsons SA, Stuetz RM. Developments in odour control and waste gas treatment biotechnology: a review. Biotechnol Adv (2001) 19:35–63. doi: 10.1016/S0734-9750(00)00058-6
30. Besaltatpour A, Hajabbasi M, Khoshgoftarmanesh A, Dorostkar V. Land farming process effects on biochemical properties of petroleum-contaminated soils. Soil Sediment Contam Int J (2011) 20:234–48. doi: 10.1080/15320383.2011.546447
31. Bhattacharya M, Guchhait S, Biswas D, Datta S. Waste lubricating oil removal in a batch reactor by mixed bacterial consortium: a kinetic study. Bioprocess Biosyst Eng (2015) 38:2095–106. doi: 10.1007/s00449-015-1449-9
32. Carniato L, Schoups G, Seuntjens P, Van Nooten T, Simons Q, Bastiaens L. Predicting longevity of iron permeable reactive barriers using multiple iron deactivation models. J Contam Hydrol (2012) 142:93–108. doi: 10.1016/j.jconhyd.2012.08.012
33. Cassidy DP, Srivastava VJ, Dombrowski FJ, Lingle JW. Combining in situ chemical oxidation, stabilization, and anaerobic bioremediation in a single application to reduce contaminant mass and leachability in soil. J Hazard Mater (2015) 297:347–55. doi: 10.1016/j.jhazmat.2015.05.030
34. Cerqueira VS, Peralba MR, Camargo FAO, Bento FM. Comparison of bioremediation strategies for soil impacted with petrochemical oily sludge. Int Biodeterior Biodegradation (2014) 95:338–45. doi: 10.1016/j.ibiod.2014.08.015
35. Chemlal R, Abdi N, Lounici H, Drouiche N, Pauss A, Mameri N. Modeling and qualitative study of diesel biodegradation using biopile process in sandy soil. Int Biodeterior Biodegradation (2013) 78:43–8. doi: 10.1016/j.ibiod.2012.12.014
36. Chen J, Zhou HC, Wang C, Zhu CQ, Tam NF-Y. Short-term enhancement effect of nitrogen addition on microbial degradation and plant uptake of polybrominated diphenyl ethers (PBDEs) in contaminated mangrove soil. J Hazard Mater (2015) 300:84–92. doi: 10.1016/j.jhazmat.2015.06.053
37. Adesodun JK Mbagwu JS. Biodegradation of waste-lubricating petroleum oil in a tropical alfisol as mediated by animal droppings. Bioresour Technol (2008) 99(13):5659–65. doi: 10.1016/j.biortech.2007.10.031
38. Babalola OA, Adesodun JK, Olasantan FO, Adekunle AF. Responses of some soil biological, chemical and physical properties to short-term compost amendment. Int J Soil Sci (2012) 7:28–38. doi: 10.3923/ijss.2012.28.38
39. AdeOluwa OO, Ogundeji AO, Agbeyegbe DO. Remediation of soil contaminated with motor spirit using compost material. Int J Plant Soil Sci (2015) 6(1):16–25. doi: 10.9734/IJPSS/2015/14788
40. Chikere CB, Chikere BO, Okpokwasili GC. Bioreactor-based bioremediation of hydrocarbon-polluted Niger delta marine sediment, Nigeria. 3 Biotech (2012) 2:53–66. doi: 10.1007/s13205-011-0030-8
41. Coulon F, Al Awadi M, Cowie W, Mardlin D, Pollard S, Cunningham C, et al. When is a soil remediated? comparison of biopiled and windrowed soils contaminated with bunker-fuel in a full-scale trial. Environ pollut (2010) 158:3032–40. doi: 10.1016/j.envpol.2010.06.001
42. Chikere CB, Okoye AU, Okpokwasili GC. Microbial community profiling of active oleophilic bacteria involved in bioreactor-based crude-oil polluted sediment treatment. J Appl Environ Microbiol (2016) 4:1–20. doi: 10.12691/jaem-4-1-1
43. Nikolova C, Gutierrez T. Use of microorganisms in the recovery of oil from recalcitrant oil reservoirs: Current state of knowledge, technological advances and future perspectives. Front Microbiol (2020) 10:2996. doi: 10.3389/fmicb.2019.02996
44. Dadrasnia A, Agamuthu P. Diesel fuel degradation from contaminated soil by dracaena reflexa using organic waste supplementation. J Japan Petrol Inst (2013) 56:236–43. doi: 10.1627/jpi.56.236
45. De Pourcq K, Ayora C, García-Gutiérrez M, Missana T, Carrera JA. Clay permeable reactive barrier to remove Cs-137 from groundwater: column experiments. J Environ Radioact (2015) 149:36–42. doi: 10.1016/j.jenvrad.2015.06.029
46. Ahmad AA, Muhammad I, Shah T, Kalwar Q, Zhang J, Liang Z, et al. Remediation Methods of Crude Oil Contaminated Soil. World J Agri & Soil Sci (2021) 4(4): 2020 doi: 10.33552/WJASS.2020.04.000595
47. De-Bashan LE, Hernandez J-P, Bashan Y. The potential contribution of plant growth-promoting bacteria to reduce environmental degradation–a comprehensive evaluation. Appl Soil Ecol (2012) 61:171–89. doi: 10.1016/j.apsoil.2011.09.003
48. Declercq I, Cappuyns V, Duclos Y. Monitored natural attenuation (MNA) of contaminated soils: state of the art in Europe–a critical evaluation. Sci Total Environ (2012) 426:393–405. doi: 10.1016/j.scitotenv.2012.03.040
49. Rissato SR, Mário MS, Fernandes JR, Gerenutti M, Gomes HM, Renata Ribeiro R, et al. Evaluation of Ricinus communis l. for the phytoremediation of polluted soil with organochlorine pesticides. BioMed Res Int (2015) 2015(549863):8. doi: 10.1155/2015/549863
50. Luo J, Qi S, Peng L, Xie X. Phytoremediation potential of cadmium-contaminated soil by eucalyptus globulus under different coppice systems. Bull Environ Contamination Toxicol (2015) 94:321–25. doi: 10.1007/s00128-014-1450-z
51. Turgut C, Katie Pepe M, Cutright TJ. The effect of EDTA andcitric acid on phytoremediation of cd, cr, and Ni from soil using Helianthus annuus. Environ pollut (2004) 131:147–54. doi: 10.1016/j.envpol.2004.01.017
52. Subhasini V, Swamy AVVS. Phytoremediation of cadmium and chromium contaminated soils by cyperus rotundus l. Am Int JRes Sci Technol Eng Math (2014) 6:97–101. doi: 10.1.1.1041.5154&rep=rep1&type=pdf
53. Bani A, Pavlova D, Echevarria G, Mullaj A, Reeves RD, Morel JL, et al. Nickel hyperaccumulation by the species of Alyssum and Thlaspi (Brassicaceae) from the ultramafic soils of the balkans. Bot Serb (2010) 34:3–14.
54. Shehata SM, Badawy RK, Aboulsoud YIE. Phytoremediation of some heavy metals in contaminated soil. Bull Natl Res Cent (2019) 43:189. doi: 10.1186/s42269-019-0214-7
55. Arbaoui S, Campanella B, Rezgui S, Paul R, Bettaieb T. Bioaccumulation and photosynthetic activity response of kenaf (Hibicus cannabinus l.) to cadmium and zinc. Greener J Agri Sci (2014) 4:91–100. doi: 10.15580/GJAS.2014.3.1216131031
56. Herzig R, Nehnevajova E, Pfistner C, Schwitzguebel J-P, Ricci A, Keller C. Feasibility of labile zn phytoextraction using enhanced tobacco and sunflower: results of five-and one-year field-scale experiments in Switzerland. Int J Phytoremediat (2014) 16:735–54. doi: 10.1080/15226514.2013.856846
57. Kalve S, Sarangi BK, Pandey RA, Chakrabarti T. Arsenic and chromium hyperaccumulation by an ecotype of Pteris vittata–prospective for phytoextraction from contaminated water and soil. Curr Sci India (2011) 100:888–94. Available at: https://www.jstor.org/stable/24076481
58. Szalata M, Wróbel T, Luwanska A, Szalata M, Wielgus K. Influence of cadmium on protein profile of flax varieties (Linum usitatissimum l.). Herba Polonica (2014) 2014):60(3). doi: 10.2478/hepo-2014-0017
59. Nizam UM, U-Zzaman W, Rahman MM, Kim JE. Phytoremediation potential of kenaf (Hibiscus cannabinus l.), mesta (Hibiscus sabdariffa l.), and jute (Corchorus capsularis l.) in arsenic-contaminated soil. Korean J Environ Agric (2016) 35:111–20. doi: 10.5338/KJEA.2016.35.2.15
60. Alori E, Fawole O. Phytoremediation of soils contaminated with aluminium and manganese by two arbuscular mycorrhizal fungi. J Agric Sci (2012) 4:246–52. doi: 10.5539/jas.v4n8p246
61. Alori ET, Joseph A, Adebiyi OTV, Aluko AP, Onyekankeya C. Bioremediation potentials of sunflower and Pseudomonas species in soil contaminated with lead and zinc. Afr J Biotechnol (2018) 17(44):1324–30. doi: 10.5897/AJB2018.16523
62. Delforno TP, Moura AGL, Okada DY, Sakamoto IK, Varesche MBA. Microbial diversity and the implications of sulfide levels in an anaerobic reactor used to remove an anionic surfactant from laundry wastewater. Bioresour Technol (2015) 192:37–45. doi: 10.1016/j.biortech.2015.05.050
63. Delille D, Duval A, Pelletier E. Highly efficient pilot biopiles for on-site fertilization treatment of diesel oil-contaminated sub-Antarctic soil. Cold Reg Sci Technol (2008) 54:7–18. doi: 10.1016/j.coldregions.2007.09.003
64. Dias RL, Ruberto L, Calabró A, Balbo AL, Del Panno MT, Mac Cormack WP. Hydrocarbon removal and bacterial community structure in on-site biostimulated biopile systems designed for bioremediation of diesel-contaminated Antarctic soil. Polar Biol (2015) 38:677–87. doi: 10.1007/s00300-014-1630-7
65. Chen XD, Dunfield KE, Fraser TD, Wakelin SA, Richardson AE, Condron LM. Soil biodiversity and biogeochemical function in managed ecosystems. Soil Res (2019) 58:1–20. doi: 10.1071/SR19067
66. Narayanan P. Environmental pollution. In: Principles, analysis and control. India: CBS Publishers and Distributors PVT (2009). p. 671 p.
67. Doran JW, Stamatiadis S, Haberern J. Soil health as an indicator of sustainable management. Agric Ecosys Environ (2002) 88:107–10. doi: 10.1016/S0167-8809(01)00250-X
68. FAO, ITPS. Status of the world’s soil resources (SWSR) - main report. Rome, Italy: Food and Agriculture Organization of the United Nations and Intergovernmental Technical Panel on Soils (2015). Available at: http://www.fao.org/3/a-i5199e.pdf.
69. Rodríguez-Eugenio N, McLaughlin M, Pennock D. Soil pollution: A hidden reality. Rome FAO (2018), 142 p. Available at: http://www.fao.org/3/I9183EN/i9183en.pdfISBN:978-92-5-130505-8
70. Passatore L, Rossetti S, Juwarkar AA, Massacci A. Phytoremediation and bioremediation of polychlorinated biphenyls (PCBs): state of knowledge and research perspectives. J Hazard Mater (2014) 278:189–202. doi: 10.1016/j.jhazmat.2014.05.051
71. Odabasi M, Dumanoglu Y, Ozgunerge FE, Tuna G, Altiok H, Kara M, et al. Investigation of spatial distributions and sources of persistent organic pollutants (POPs) in a heavily polluted industrial region using tree components. Chemosphere (2016) 160:114–25. doi: 10.1016/j.chemosphere.2016.06.076
72. Pal AK, Singh J, Soni R, Tripathi P, Kamle M, Tripathi V, et al. The role of microorganism in bioremediation for sustainable environment management. Bioremediation Pollutants. Elsevier (2020), 227–49. doi: 10.1016/b978-0-12-819025-8.00010-7
73. Abatenh E, Gizaw B, Tsegaye Z, Misgan W. The role of microorganisms in bioremediation- a review. Open J Environ Biol (2017) 2(1):038–46. doi: 10.17352/OJEB.000007
74. Tiedje JM. Bioremediation from an ecological perspective. In: In situ bioremediation: When does it work. Washington, D. C (USA):National Academy of Sciences (1993). p. 110–20.
75. Chandrakant SK, Shwetha SR. Role of microbial enzymes in the bioremediation of pollutants: A review. Enzyme Res (2011) 2011:1–11. doi: 10.4061/2011/80518
76. Arora PK, Srivastava A, Singh VP. “Application of monooxygenases in dehalogenation, desulphurization, denitrification and hydroxylation of aromatic compounds,”. J Bioremediation Biodegradation (2010) 1:1–8. doi: 10.4172/2155-6199.1000112
77. Boopathy R. Factors limiting bioremediation technologies. Bioresource Technol (2000) 74:63–7. doi: 10.1016/S0960-8524(99)00144-3
78. Adriaens P, Hickey WJ. Physiology of Biodegradative Microorganisms. In: Biotechnology for the treatment of hazardous waste. Stone DL. 1st edition. Routledge Taylor and Francis (1994) 39. doi: 10.1201/9781315138442
79. Villaverde J, Láiz L, Lara-Moreno A, Morillo E. Bioaugmentation of PAH-contaminated soils with novel specific degrader strains isolated from a contaminated industrial site. effect of hydroxypropyl -β-cyclodextrin as PAH bioavailability enhancer. Front Microbiol (2019) 10:2588. doi: 10.3389/fmicb.2019.02588
80. Kanissery RG, Sims GK. Biostimulation for the enhanced degradation of herbicides in soil. Appl Environ Soil Sci (2011) 2011(843450):10. doi: 10.1155/2011/843450
81. Shukla KP, Singh NK Sharma S. Bioremediation: Developments, current practices and perspectives. Genet Eng Biotechnol J (2010) 3:1–20. doi: 10.1.1.473.9479&rep=rep1&type=pdf
82. Li YY, Li B. Study on fungi-bacteria consortium bioremediation of petroleum contaminated mangrove sediments amended with mixed biosurfactants. Adv Mater Res (2011) 183-185:1163–67. doi: 10.4028/www.scientific.net/AMR.183-185.1163
83. Baniasadi M, Vakilchap F, Bahaloo-Horeh N, Mousavi SM, Farnaud S. Advances in bioleaching as a sustainable method for metal recovery from e-waste: A review. J Ind Engineer Chem (2019) 76:75–90. doi: 10.1016/j.jiec.2019.03.047
84. Narayanan CM, Narayan V. Biological wastewater treatment and bioreactor design: a review. Sust Environ Res (2019) 29:33. doi: 10.1186/s42834-019-0036-1
85. Brown DM, Okoro S, van Gils J, van Spanning R, Bonte M, Hutchings T, et al. Comparison of land farming amendments to improve bioremediation of petroleum hydrocarbons in Niger delta soils. Sci Total Environ (2017) 296-297:284–92. doi: 10.1016/j.scitotenv.2017.04.072
86. Panesar AS, Kumar A, Kalpana A. Phytoremediation: An ecofriendly tool for in-situ remediation of contaminated soil. J Pharmacognosy Phytochem (2019) 8:311–16. Available at: https://www.phytojournal.com/archives/2019/vol8issue1S/PartH/Sp-8-1-94-389.pdf
87. Theng BKG, Orchard VA. Interactions of clays with microorganisms and bacterial survival in soil: a physicochemical perspective. In: Huang PM, Berthelin J, Bollag J-M, McGill WB, Page AL, editors. Environmental impact of soil component interactions, vol. Vol. 2 . Boca Raton, US: CRC Press/Lewis (1995). p. 123–43.
88. Salilh FZ, Tarekegn MM. Cleaning polluted environments by bioremediation: Review. IJESC (2020) 10(8):27112–21. Available at: https://ijesc.org/upload/36172711a56684478405d1ee977ff36a.Cleaning%20Polluted%20Environments%20by%20Bioremediation-Review.pdf
89. Manning J, Boopathy R, Kulpa CF. A laboratory study in support of the pilot demonstration of a biological soil slurry reactor. report no. SFIM-AEC-TS-CR-94038. Aberdeen Proving Ground, M: US Army Environmental Center (1995).
90. Blackburn JW, Hafker WR. The impact of biochemistry, bioavailability, and bioactivity on the selection of bioremediation technologies. TIB Tech (1993) 11:328–33. doi: 10.1016/0167-7799(93)90155-3
91. Fodelianakis S, Antoniou E, Mapelli F, Magagnini M, Nikolopoulou M, Marasco R, et al. Allochthonous bioaugmentation in ex situ treatment of crude oil-polluted sediments in the presence of an effective degrading indigenous microbiome. J Hazard Mater (2015) 287:78–86. doi: 10.1016/j.jhazmat.2015.01.038
Keywords: bioremediation, biostimulant, soil environment, soil pollution, bioaugumentation,
Citation: Alori ET, Gabasawa AI, Elenwo CE and Agbeyegbe OO (2022) Bioremediation techniques as affected by limiting factors in soil environment. Front. Soil Sci. 2:937186. doi: 10.3389/fsoil.2022.937186
Received: 05 May 2022; Accepted: 14 July 2022;
Published: 04 August 2022.
Edited by:
Christos Damalas, Democritus University of Thrace, GreeceReviewed by:
Sunita Varjani, Gujarat Pollution Control Board (GPCB), IndiaRam Naresh Bharagava, Babasaheb Bhimrao Ambedkar University, India
Copyright © 2022 Alori, Gabasawa, Elenwo and Agbeyegbe. This is an open-access article distributed under the terms of the Creative Commons Attribution License (CC BY). The use, distribution or reproduction in other forums is permitted, provided the original author(s) and the copyright owner(s) are credited and that the original publication in this journal is cited, in accordance with accepted academic practice. No use, distribution or reproduction is permitted which does not comply with these terms.
*Correspondence: Elizabeth Temitope Alori, YWxvcml0b3BlQHlhaG9vLmNvbQ==