- 1Institute of Soil Science, Leibniz Universität Hannover, Hannover, Germany
- 2Institute of Bio- and Geosciences, IBG-3: Agrosphere, Forschungszentrum Jülich GmbH, Jülich, Germany
- 3Institute of Physiology and Cell Biology, Tierärztliche Hochschule Hannover, Hannover, Germany
Many natural and anthropogenic soils are phosphorus (P) limited often due to larger P stocks sequestered in forms of low bioavailability. One of the strategies to overcome this shortage lies in the symbiosis of plants with mycorrhizal fungi, increasing the plant P uptake of these hardly accessible sources. However, little is known about mycorrhizal fungal mediated partitioning of differently available P forms, which could contribute to more efficient use of P by plants and, thereby, reduce competition for soil P. This study aimed to investigate the uptake of P from differently bioavailable P sources by ectomycorrhiza. For that, we conducted a rhizotrone study using Populus x canescens and its compatible ectomycorrhizal fungus Paxillus involutus. Four different P sources [ortho-phosphate (oP), adenosine monophosphate (AMP), hydroxyapatite (HAP), and oP bound to goethite (gP)] or only HAP as 1P control were supplied in separate compartments, where only the fungal partner had access to the P sources. The amount of the specific P sources was increased according to their decreasing bioavailability. In order to distinguish between the P sources, we applied 33P to track its incorporation in plants by a non-destructive analysis via digital autoradiography. Our results show that an ectomycorrhizal plant is able to utilize all provided P sources via its mycorrhizal fungal associate. The acquisition timing was determined by the most bioavailable P sources, with oP and AMP over HAP and gP, and a mixed P pool over a single P source. In contrast, the magnitude was defined by the by the amount of supplied P source provision of additional nitrogen, hence AMP over oP and gP, as well as by P source complexity, with gP as the least favorable P form. Nevertheless, the results of the present study provide evidence that an ectomycorrhiza has the potential to occupy fundamental niches of various P sources differing in their bioavailability, indicating that being a generalist in P nutrition can facilitate adaptation to various nutritional settings in soil.
Introduction
Phosphorus is an essential element for the plant net primary productivity (1), but it is also limited in soils in many ecosystems [e.g., (2)], as more than 90% of P in the soil is present in different chemical forms that are not readily available to plants (3). Plants are able to take up P in the form of free phosphate (Pi) in soil solution (4). However, Pi is poorly mobile, as it interacts strongly with soil constituents and can be adsorbed onto metal oxides and clay minerals, and precipitate as (apatite-like) minerals (5, 6). In addition, P is immobilized in diverse organic forms. In young soils with parent material high in P, plants and microorganisms acquire P from primary minerals (7). However, with increasing soil development, primary minerals are getting depleted, and the plants and soil organisms recycle P from organic matter in the upper soil horizons (7, 8) or pedogenic Fe and Al oxides mainly in the subsoil (9, 10). Therefore, with increasing soil succession and associated depletion in readily available P sources, more efficient plant P uptake and recycling (8) are necessary.
The association of plants with mycorrhizal fungi defines a strategy to cope with P limitation (1). With their extraradical hyphae, the mycorrhizal symbionts are able to increase the volume of soil explored for nutrients beyond the rhizosphere (11, 12). Furthermore, mycorrhizal fungi actively contribute to P mobilization through exudation of low-molecular-weight organic anions (LMWOAs) to release P from primary and secondary minerals and release phosphatases to cleave P from organic P sources [reviewed by (1)]. The mycorrhiza associated P uptake is thereby more cost-effective, as less carbon (C) units are needed for the uptake of one unit P, while the hyphae provide a higher adsorbing length for the same infrastructural investment as compared to roots (13). Furthermore, depending on the inherent P acquiring mechanisms, mycorrhizal fungi are able to mobilize P from mineral and/or organic P sources (14, 15) and could, therefore, contribute to the necessary efficient use of different forms of P in soil. Also, ectomycorrhizal fungi showed an adaptation to a high variety of P sources such as inorganic phosphate (Pi), as well as different mineral (16) and organic P sources (17). Furthermore, the study of Zavičić et al. (18) has shown that fertilization of soils with triple phosphate negatively affected the P uptake efficiency of mycorrhizal beech compared to P uptake efficiency in P deficient soils.
According to the framework of Turner (19), the different chemical forms of P are partitioned by plants with different P acquiring adaptations to P limitation. This framework highlights the fact that mycorrhizal fungi are the key players to reduce competition for P and facilitate the co-existence of plants. Plant communities developing on soils with limited bioavailabilities of P are globally some of the most biodiverse compared with ecosystems limited in other nutrients (20, 21). Therefore, besides affecting the net primary productivity of terrestrial ecosystems, P limitation influences also the diversity and persistence of different plant species (20–22).
Progress in studying P partitioning for soil P has been achieved by examining plant responses to single P sources (23, 24) or a mixed pool of different P sources (25). The study of Andrino et al. (26, 27) investigated the mycorrhizal fungal acquisition of differently available P sources, which was determined by different amounts of C invested by the plant into the association with a mycorrhizal fungus that had access to differently available P sources. Nevertheless, the P sources were supplied as a single P source. The demonstration of the uptake of P with different bioavailabilities is challenging due to the need to differentiate one particular P source from a mixture of various P sources. Starting with Pearson and Jacobsen's (28) groundbreaking study on P uptake via mycorrhiza, recently, a few studies have used radioisotope labeling with 33P to demonstrate differences in plant P uptake from different chemical forms of P offered as a sole P source (21) and as a mixture of different P sources (29). However, the sole contribution of ectomycorrhizal fungi in resource partitioning for P remains unclear. Hence, the aim of our study was to investigate whether an ectomycorrhizal fungus shows preferences to specific P sources of different bioavailability when offered in a mixed pool. This should help understand how the belowground nutrient P diversity shapes the mycorrhizal mediated aboveground plant biomass and P uptake. The present study addressed the following hypotheses: as the mycorrhizal fungi are essential in plant P acquisition and are known to be able to access organic and sorbed P (1, 30), we hypothesized (H1) that all P sources within the diverse P pool are available to mycorrhizal fungi, and therefore to plants, but the order and magnitude of acquisition depend on the P source complexity. We expected that the mycorrhizae would favor the readily available Pi over organic or mineral P sources, whereby the amount of each P source applied into the system was increased according to its complexity. Furthermore, in order to support the general assumption that a minor diversity in belowground resources, which can occur as a side effect of fertilization (31, 32), result in less efficient uptake of P (18), we hypothesize (H2) that mineral source (HAP) as a single P source is less available than within a pool of diverse P sources.
Materials and Methods
Plant and Mycorrhizal Material
Poplar species Populus x canescens clone “Schleswig I” was used as a model plant and its functional ectomycorrhizal fungal strain MAJ of Paxillus involutus (33) was used as a symbiotic partner. Both were identified as functional associates which provide valuable model systems for a more robust test of nutrient acquisition and exchange models (33). The poplar plantlets were propagated on Woody Plant Medium [WPM (34)] without hormones (dephyte e.K., Langenberg, Germany) by establishing explants from the first two lateral meristems with one leaf and bud. The apical buds with 1.5 cm height were used for rooting on WPM medium but with one-third amount of sucrose and vitamins in Microboxes (O118/80, by Sac O2, Deinze, Belgium) with a membrane for sterile gas exchange (81.35 GE day−1). P. involutus was propagated in liquid culture of 0.2 L of modified Melin-Norkrans (MMN; ready to use salts, dephyte e.K., Langenberg, Germany) medium (33) in 0.5 L glass bottles under shaking and proliferated on 0.8 L Perlite as a carrier with 0.2 L MMN.
Preparation of the Phosphorus Sources and the Containers
To investigate mycorrhizal mediated plant P uptake from a pool of different P sources, the following chemical forms of P were tested: free ortho-phosphate (oP), adenosine monophosphate (AMP), hydroxyapatite (HAP), beside phosphate bound to goethite (gP) as a P adsorption complex with the Fe oxide, which is one of the most profuse and naturally occurring in soils (35). To differentiate the uptake between the different P sources, the use of radio-labeling with 33P in combination with the non-destructive digital autoradiography was made. As 33P labeled compounds [P33] phosphoric acid (FF-1) and [alpha-P33] AMP (FF-217) were purchased from Hartmann Analytic GmbH (Braunschweig, Germany).
To obtain the P sources oP and AMP in concentrations of 0.9 and 3.8 mg P ml−1, respectively, NaH2PO4 · 2H2O (for analysis, EMSURE®, Merck KGaA, Darmstadt, Germany) and adenosine 5′-monophosphate sodium salt (from yeast, >99%, SigmaAldrich, Merck KGaA, Darmstadt, Germany) were dissolved in deionized H2O (dH2O) and the pH adjusted to 5.2 using 1 M Na2HPO4 for oP and 1 M HCl for AMP. For labeling with 33P, an aliquot of the oP and AMP solutions was spiked with 0.20 ml 74 MBq PO4 and 0.20 ml 74 MBq AM33P, respectively. The oP and AMP were filtered using sterile syringe filter (Filtropur S, PES, 0.2 μm pore size, Sarstedt AG & Co. KG, Nümbrecht, Germany).
The HAP was produced as multistep synthesis at 25°C according to the protocol described by Wolf et al. (36). The only modification of this protocol was performed in the second step for the application of 33P label, in which the 2 M H3PO4 solution was spiked with 0.5 ml 185 MBq PO4. The HAP pellet was ground with mortar and pestle and passed through a 0.20 mm sieve. The resulting 33P activity of HAP accounted for 117.8 MBq g−1.
To prepare the adsorption complexes of oP to goethite (gP), the procedure described by Andrino et al. (26, 27) was used with following modifications: for the loading of goethite with oP, 1 M NaH2PO4 solution was used. To prepare the 33P labeled gP (gP-33P), 24.5 ml of 1 M NaH2PO4 solution were spiked with 0.5 ml 185 MBq PO4. Always 6.2 ml 1 M NaH2PO4 (non-labeled or 33P-labeled) and 5 g of synthetic goethite (α-FeOOH < 0.045 mm, Bayferrox® 920 Z, Lanxess Deutschland GmbH, Cologne, Germany) were mixed and filled up to 220 ml with dH2O in centrifuge bottles (250 ml, NalgeneTM, Thermo ScientificTM, Waltham, Massachusetts, USA). Further steps are described in detail by Andrino et al. (26, 27) up to the step of drying the gP-33P pellet. Due to the labeling with 33P, gP had to be dried at 50°C in a drying oven without ventilation. After cooling down, the gP pellet was sieved through a 0.63 mm sieve. The resulting 33P activity of gP accounted for 245.6 kBq g−1.
Both gP and HAP (labeled and non-labeled) were sintered three times at 105°C for 30 min in a drying oven, including a subsequent incubation period at 30°C for 2 h the first two times. This step is called tyndallization and is used for fractionated sterilization without changing the mineral structure. The Raman spectrum of HAP with its phosphate typical bands (Supplementary Figure 1) is aligned with the spectra obtained by Wolf et al. (36), confirming the main characteristics of HAP.
To determine the P content of gP and HAP, 102 mg of each were solubilized in 10 ml 32% HCl (AnalaR NORMAPUR® for analysis, VWR International GmbH, Darmstadt, Germany), shaken on an overhead shaker for 24 h, and filtered using filter papers (thickness of 0.14 mm, a pore size of 2–3 μm; LABSOLUTE®, Th. Geyer GmbH & Co. KG, Renningen, Germany). P analysis was performed by Inductively Coupled Plasma-Optical Emission Spectrometry (ICP-OES, Varian 725-ES, City, State). In order to determine the easy desorbable P from gP and HAP, 55 mg gP or 101 mg HAP were suspended in 10 ml dH2O, shaken on an overhead shaker for 24 h, and filtered through a filter paper (as described above). The analysis of P in the filtered solution was performed via ICP-OES. The P content of gP accounted to 1.04 ± 0.06 mg g−1 goethite (n = 7), of which 0.17 ± 0.01 mg P g−1 gP were easy desorbable, so that maximum of 16% P from P bound to goethite could be mobilized without any action of the mycorrhizal fungus. The P content of HAP accounted for 197.5 ± 5.3 mg g−1 HAP (n = 3), of which 7.1 ± 0.1 mg P g−1 HAP (3.6% P in HAP) were easy desorbable. According to the dissolution studies of HAP conducted by Wolf et al. (36), HAP is (independent of the synthesis temperature) otherwise stable in aqueous systems at pH values above 3.8.
All P sources were supplied in separate containers made of 5 ml SafeSeal reaction tubes. At around 2 ml height, a hole of 1 cm in diameter was burned into the tube and sealed with a combination of two types of membranes, allowing only the mycorrhizal partner to access the P sources in the P containers as described in more detail by Andrino et al. (26, 27). Briefly, a hydrophobic PTFE membrane (5 μm, Pieper Filter GmbH, Bad Zwischenahn, Germany) was installed at the P source side and a nylon mesh (20 μm, Franz Eckert GmbH, Waldkirch, Germany) at the plant root side. The containers were autoclaved (121°C, 20 min) and filled with the different labeled and non-labeled P sources under sterile conditions in a laminar flow cabinet. All containers were filled up to 3 ml with dH2O.
Preparation of the Rhizotrone Culture Systems
A small-scaled, continuously closed rhizotrone culture system with four separate containers for the simultaneous supply of four different P sources (oP, AMP, HAP, and gP) was established to test hypothesis H1. In this 4P-33P experiment, only one P source was labeled with 33P before installing into a rhizotrone culture system. The overall P amount per system accounted for 13.2 mg P and was considered sufficient to sustain the system for 3 months. The specific P sources were supplied as 0.9 mg P in the form of oP, 3.8 mg P in the form of AMP, 6.2 ± 0.1 mg P in the form of HAP, and 2.3 ± 0.1 mg P as gP, whereby 223 and 368 μg P from mineral P sources HAP and gP (respectively) were easy desorbable (Table 1). The supplied P amounts increased with the increasing complexity of the P sources. The activity of 33P (Table 1) accounted for 7,452 kBq per P container for oP and AMP and 2,538 and 410 kBq per P container for HAP and gP (respectively) at 7.5 days post inoculation (dpi).

Table 1. Amount of P [mg], 33P activity [kBq], and the calculated specific 33P activity [Bq mg−1 P] of the different P sources applied in the P compartments in the rhizotrone systems.
To test hypothesis H2, a 1HAP-33P experiment was established to test the synthesized, 33P labeled hydroxyapatite (1HAP-33P) as a sole P source as a control to the 4P-33P experiment. The P containers contained 19.9 ± 0.4 mg P in the form of HAP to provide a complex P source in surplus to ensure uptake. The activity of 33P in HAP accounted for 8,080 kBq per P container at 3 dpi.
The rhizotrone culture systems used in the present study were made of square Petri dishes (10 × 10 × 2 cm, Sarstedt AG & Co. KG, Nümbrecht, Germany). The P containers were inserted 1 cm from the bottom into the rizotrones in a randomized order. Then, the rhizotrones were filled with Perlite (washed with dH2O; autoclaved at 121°C for 20 min two times; Perligran® classic, Knauf Aquapanel GmbH, Dortmund, Germany) as nutrient-free substrate. To obtain mycorrhizal plant treatments, the substrate was inoculated with 10 vol.% of P. involutus-Perlite carrier. For the non-mycorrhizal plant treatments, the inoculated substrate was autoclaved one more time before use. The rooted poplar plantlets were placed on the top of the P containers and covered with an additional thin layer of Perlite. Next, the rhizotrone was covered with a thin, sterile PVC foil (10 × 10 × 0.02 cm, Modulor GmbH, Germany) to minimize radio-active shielding and afterwards sealed with hot glue. The skip for the plant stem at the top and the opening to release the excess of nutrient solution at 2 cm height from the bottom of the rhizotrone were closed with sterile cotton wool.
To keep the system sterile during the watering, the rhizotrones were equipped with a sterile syringe filter (Filtropur S, PES, 0.2 μm pore size, Sarstedt AG & Co. KG, Nümbrecht, Germany), which was connected to the rhizotrone through an autoclaved PVC pipe. From 0 dpi, the mesocosms were supplied with a Woody Plant (WPM) nutrient solution containing macro- and micro-elements without P and vitamins (WPM –P; dephyte e.K., Langenberg, Germany), which is a modification from a recipe described by Müller et al. (34) and which was balanced with KNO3 and (NH4)2SO4 to adjust the desired concentration of K.
For each 33P labeled P source, four replicates were prepared as mycorrhizal treatments and three replicates as non-mycorrhizal treatments. To obtain no P controls with P compartments containing H2O only, two mycorrhizal and two non-mycorrhizal rhizotrone culture systems were prepared. The plantlets were kept under a plastic cover that included moisturized protecting paper plugs for the first 2 weeks for acclimatization and, thus, for protection from lower ambient air humidity and higher UV radiation. The rhizotrones were kept in a climate chamber set at an 18/6 h day/night cycle with 20/18°C and 80% ambient humidity.
Autoradiography and Harvest of Rhizotrones and Plant Material
In the time course of the experiment, the rhizotrones were regularly exposed to phospho-imaging screens for 4 h (at 7, 21, 34, 52, 70, and 80 dpi) and 72 h (at 42 and 94 dpi) in order to monitor the transfer of 33P through the ectomycorrhizal fungal partner from the P containers into the plant shoots. To do this, the whole rhizotrone surfaces, including the above-ground plants, were overlaid by phospho-imaging screens (20 × 40 cm and 35 × 43 cm; Dürr NDT GmbH & Co. KG, Germany). They were wrapped into an extra thin cover foil to protect the screens from contamination with the 33P label and damage by an outflow from the rhizotrone. To ensure possibly tight contact between the plant leaves and stems and the phospho-imaging screen, a foam of a suitable thickness was placed under the plant shoots. Another foam and a heavier plate were placed from the phospho-imaging screens' backside to ensure tight contact between the rhizotrone and screen from the top side. As reference to the 33P label, a set of 14C polymer standards (IPcal test source array; Elysia-raytest, Straubenhardt, Germany) were used with different activities (in dpm cm−2): 1,107,000, 424,000, 112,000, 41500, 13,350, and 3,950, supplemented by an activity free background. Thereby, each activity had a surface area of 1 cm2. The exposition of rhizotrones to the phosphor-imaging screens must be in the dark. The phospho-image screens were read out immediately using an image plate scanner (CR-35 BIO, Elysia-raytest, Liège, Belgium) in sensitive mode with 100 μm resolution. Each scan is saved as a set of two raw data files which can be converted subsequently into one (e.g., JPG) data file using the Aida Image Analyser v5.0 (Elysia-raytest, Straubenhardt, Germany).
The mesocosms from 1HAP-33P and 4P-33P experiments were harvested at 108 dpi and between 110 and 112 dpi, respectively. The plant roots were separated from the substrate, washed, and dried with paper clothes. Fine root aliquots of up to 0.15 g of each plant were conserved in 70% ethanol to assess mycorrhization grade. The freshly harvested plant material was immediately imaged first for 4 h and afterwards for 72 h (as described above). The same imaging procedure was performed with the plant material after drying at 40°C overnight. After the last imaging, the plant material was separated into leaves, stem, and roots, and the biomass of the different plant parts was recorded.
The substrate was dried at 40°C for 96 h. The liquids in the P containers were collected in 2 ml SafeSeal reaction tubes, weighed, and controlled for the pH using pH-indicator strips (Neutralit® pH5-10, Merck KGaA, Darmstadt, Germany; pH-Fix 0-6, Macherey-Nagel GmbH & Co. KG, Düren, Germany; and pH-Fix 0-14, Carl Roth GmbH & Co. KG, Karlsruhe, Germany). The pH-indicator stripes may not provide the most accurate result, but it offers reasonable control of changes in pH attributable to P uptake.
Quantification of 33P and P and Calculation of Related Parameters
In order to quantitatively analyse the activity of 33P in plant material, all parts of plant material (leaves, stem, roots) and substrates underwent thermal digestion at 480°C for a minimum of 6 h. 33P, P, and nutrients were extracted using 3.6 M HNO3. After 10 min incubation, the extracts were filtered using a folded filter (SartoriusTM FT-4-303-185, Grade 3hw, ø150 mm, 65 g m−2, Sartorius AG, Goettingen, Germany). Thereafter, an aliquot of plant/substrate extracts (diluted to obtain a 2.3 M HNO3 solution and prevent any phase separation) was mixed with 10 ml scintillation cocktail (Ultima GoldTM, PerkinElmer Inc., USA) and analyzed for 33P activity (dpm single program under blank correction) by liquid scintillation counting (LSC, Tri-Carb 3110TR, PerkinElmer Inc., Waltham, USA). The obtained 33P activity was corrected by subtracting the background value, obtained as a blank undergoing the same extraction and preparation procedures as the plant material. The calibration/normalization of the LSC was performed daily with instrument inherent external standards. The reliability and accuracy of the 33P activity data were ensured by a serial dilution of a sample extract with a high 33P activity at 260 Bq at the day of measurement (Supplementary Figure 2). The reproducibility of measured values down to 1.5 Bq did not deviate significantly from the theoretical values. The measured values starting from 1.0 to 0.5 Bq were with 8–5% (respectively) less reproducible. 33P activity values below 0.2 Bq were with 16% standard deviation less trustful, and as a consequence, excluded from data analysis.
The 33P activity incorporated in different plant parts (leaves, stem, and roots) was determined as follows:
The correction of 33P activity in plant material from LSC measuring date and experiment starting date was calculated as follows:
Since not only the activity but also the P amounts applied with different P sources varied, we had to determine the specific activity in each P compartments containing 33P labeled P source (Table 1) by using the following equation:
The recovery of 33P (5) in plant material and substrate as well as the total P uptake in plant material (6) was calculated as follows:
The analyses of total P in plant material and substrate were performed via ICP-OES (Varian 725-ES, Agilent Technologies, Santa Clara, United States). The standards for the calibration were prepared using the same matrix.
Assessment of Mycorrhization Grade of Mycorrhizal Plant Roots
In order to record the plants' response to mycorrhizal inoculation and the P source dependency and/or diversity, we determined the mycorrhization grade in mycorrhizal plant roots. Characteristically for successful mycorrhization of poplar roots by P. involutus (MAJ) is the change in root morphology, e.g., specific branching of root tips and no development of root hairs. The grade of mycorrhization of plant roots was determined using the gridline intersection method (37) as modified and described in detail by Brundrett et al. (38). Thereby, aliquots of 82 ± 27 cm of mycorrhizal and 28 ± 7 cm of non-mycorrhizal fine roots were inspected for the mycorrhizal root tips and the intersections of roots with the gridlines in triplicates (by rearranging the same root sample after each counting) using a stereo zoom microscope (45x magnification; KERN OZM-5, KERN & SOHN GmbH, Balingen, Deutschland). Mycorrhization
grade was expressed as number of mycorrhizal root tips per cm−1 analyzed root length:
As expected, the fine roots of mycorrhizal plants were abundantly mycorrhized at 108 dpi (Supplementary Figure 3; Supplementary Table 1), while the non-mycorrhizal controls were not mycorrhized.
Statistical Analysis
All statical tests were performed with SPSS® Statistics 26.0 [IBM® Corporation, USA (39)] at the probability level of 0.05. All data were tested for normal distribution using the Shapiro-Wilk test and homogeneity of variances using Levene's test, where the P-value was calculated based on the mean. To test for significant differences in data of plant biomass, P content, and pH of P liquids (normally distributed) between mycorrhizal treatments (with two independent groups), the independent samples t-test was performed. To test for significant differences in data of plant biomass, plant P content, 33P activity, 33P recovery, and plant P uptake between the different P and mycorrhizal treatments (with more than three independent groups), the following statistical procedure was applied: a one-factorial analysis of the variances (ANOVA) with replicates was performed on normally distributed data. In case of significant differences, the Scheffé procedure (for data sets with no equal variances, as it is insensitive to violation of homogeneity of variances) or the Tukey-HSD test (for data sets with equal variances) as a post-hoc test were used. In case the data were not normally distributed, the Kruskal-Walis-H-test was used. It is a non-parametric equivalent of the One-Way ANOVA to test for significant differences between more than two groups using medians. In case of significant differences between the groups, the Mann-Witney-U-test was performed.
Results
Distribution of Biomass and Absolute P Content in Plant Parts Differ Between Mycorrhizal and P Treatments
At the end of the experiment at 108 dpi, we determined in both experiments (4P-33P and HAP-33P) the biomass (Figure 1A) of, and the absolute P content (Figure 1B) in, different plant parts and substrate in order to investigate, whether these two parameters differ between the different plant parts and P pools applied into the system.
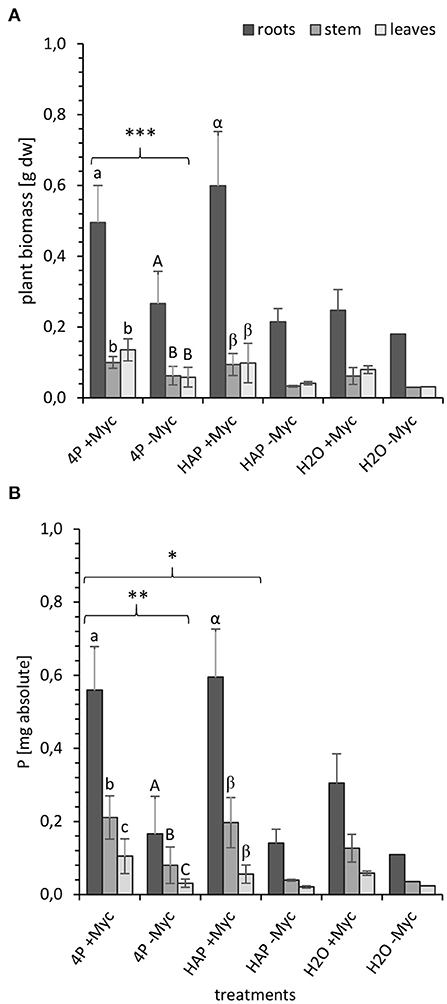
Figure 1. (A) Biomass [g dry weight] and (B) P content [mg absolute] in different parts of mycorrhizal (+Myc) and non-mycorrhizal (-Myc) poplar plant at 108 dpi. 4P experiment with simultaneous supply of four different P sources: oP, ortho-phosphate; AMP, adenosine monophosphate; gP, oP bound to goethite; HAP, synthesized hydroxyapatite; and 1P control experiment supplied with HAP. The error bars show the standard deviation (4P: +Myc: n = 16; -Myc: n = 12; HAP: +Myc: n = 4; -Myc: n = 2; H2O: +Myc: n = 2; -Myc: n = 1). The letters (a, A, α) indicate significant differences between the plant parts: (A) a-b and A-B: P < 0.001; α-β: P < 0.003; (B) a-c: P < 0.001; A-C and α-β: P < 0.005. Marking with (*) indicates significant differences between mycorrhizal and non-mycorrhizal treatments: (A) ***P < 0.001 for whole plant and for leaves, stem, and roots); (B) **P < 0.02 for the whole plant; P < 0.001 for leaves, stem, and roots; *P < 0.04 for the whole plant; P < 0.001 for leaves and stem; P < 0.01 for roots.
In both experiments, mycorrhizal plants developed significantly higher biomass at 108 dpi (Figure 1A) compared with the non-mycorrhizal plants (P < 0.0005). In addition, both treatments (mycorrhizal and non-mycorrhizal) had significantly (P < 0.00001) higher root biomass compared with the shoot biomass. Unfortunately, only two non-mycorrhizal 1HAP-33P and mycorrhizal no P (H2O) controls and only one non-mycorrhizal no P control could be harvested at the end of the experiment so that no statics could be done on these treatments.
The absolute P content (Figure 1B) follows almost the same trend as the plant biomass, as independent of the P species and mycorrhizal treatment, the absolute P content in plant correlated positively with the plant biomass (R2 = 0.64, P < 0.001; Supplementary Figure 4). The absolute P content in mycorrhizal plants from 4P-33P systems was significantly higher (P < 0.001) than in their non-mycorrhized counterparts. Mycorrhizal and non-mycorrhizal plants incorporated significantly more P (P < 0.005) in roots than in their stems and leaves. Furthermore, the P content in mycorrhizal 4P-33P plants was significantly higher (P < 0.04) than in mycorrhizal 1HAP-33P plants.
The plant biomass and the P content in plants from mycorrhizal no P control systems are almost 2-fold lower than the mycorrhizal P treatments. This gain in biomass and P content of mycorrhizal P treatments accounted for 0.35 ± 0.15 g biomass and 0.38 ± 0.17 mg P. This is the gain alone from the P uptake from the P compartments.
33P Incorporation in Plants and Its Distribution Between Plant Parts Differ Between P Treatments
In order to differentiate the plant P uptake from the different P sources and its partitioning in the plant, we have exposed the rhizotrone culture systems in the time course of the experiment (Supplementary Figure 5) as well as the harvested plants (Figure 2) to phospho-imaging screens for 4 and 72 h. Further, for the same reason, we determined the 33P activity in harvested plants (leaves, stem, and roots) and substrate (Figure 3; Supplementary Table 3).
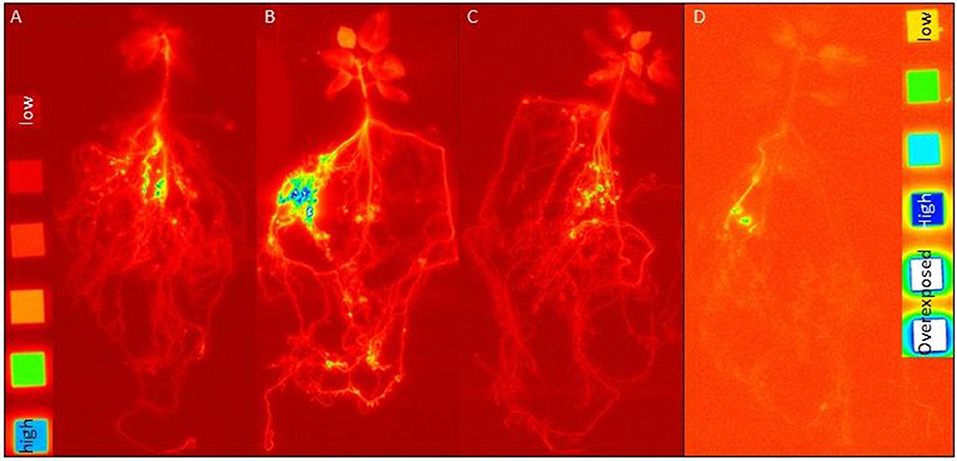
Figure 2. Images of harvested plants from rhizotrone systems labeled with (A) 33P-oP, (B) -AMP, and (C) -HAP (4 h exposing time to imaging plates; images developed at factor 2.3) as well as (D) 33P-gP (72 h exposing time to imaging plates; images developed at factor 3.6) after 108 dpi. As reference, C-14 polymer sources were used with following activities [dpm cm−2]: 1,107,000, 424,000, 112,000, 41,500, 13,350, and 3,950, supplemented by an activity free background (each activity had a surface area of 1 cm2). The blue color indicates areas with high 33P activity.
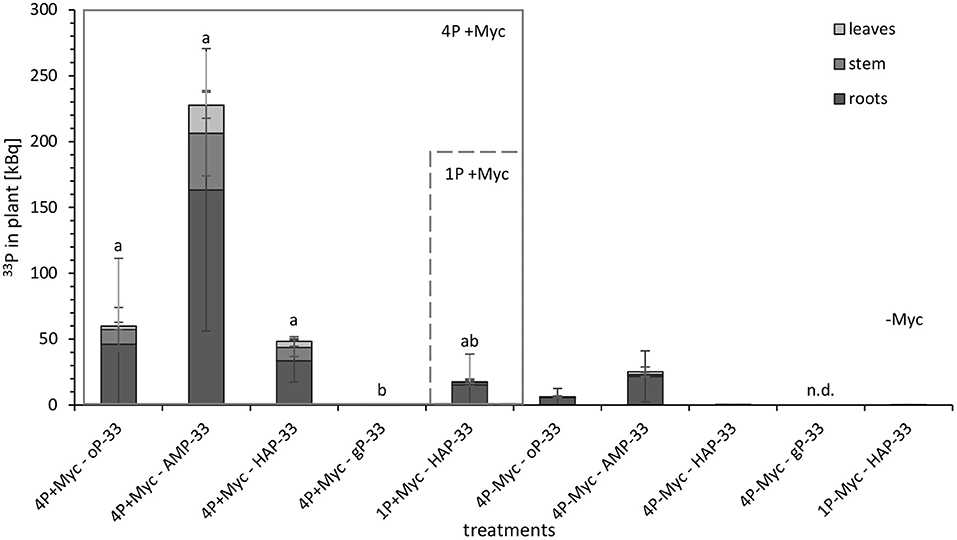
Figure 3. 33P activity [kBq] in different parts of mycorrhizal (+Myc) and non-mycorrhizal (-Myc) poplar plants at 108 dpi. 4P experiment with simultaneous supply of four different P sources: oP, ortho-phosphate; AMP, adenosine monophosphate; gP, oP bound to goethite; HAP, synthesized hydroxyapatite; and 1P control experiment supplied with HAP. The error bars show the standard deviation (4P+Myc: n = 4; 4P-Myc: n = 3; 1P+Myc: n = 4; 1P-Myc: n = 2). Data replaced by n.d. was below detection limit. The letters (a, b, c) indicate significant differences in 33P activity in plant between 4p-33P and 1HAP-33P treatments: P < 0.03 (Kruskal-Wallis-H-test and subsequent Mann-Witney-U-test; see Supplementary Table 3 for detailed information on statistical analysis).
No incorporation of 33P label in plant material was detected at 21 dpi. In contrast, the images of rhizotrone culture systems at 34 dpi revealed that the mycorrhizal fungus started to acquire the P sources oP and AMP (Supplementary Figures 5A,B, respectively) almost simultaneously. From HAP supplied in a pool of four different P sources (Supplementary Figure 5C), incorporation of 33P label in plant shoots was visible for the first time at 42 dpi, while 33P from HAP supplied as a single P source (Supplementary Figure 5D) was detectable for the first time in the plant at 94 dpi, when the rhizotrone culture systems were imaged for 72 h. No incorporation of 33P label could be detected in the plant from HAP supplied as a single P source, nor from gP supplied in a pool of four different P sources at that time point.
The images of harvested plants of 4P systems with 33P-labeled oP, AMP, and HAP (Figure 3) showed a trend in 33P activity incorporated in plant: After 4 h exposing time of harvested plant material to the phospho-imaging screens, the highest 33P activity was detected in 4P systems with 33P-labeled AMP (Figure 2B), followed by oP (Figure 2A), and HAP (Figure 2C). The blue color indicates areas with high 33P activity—these are the areas of roots with high intensity in the branching of the root tips (Supplementary Figure 2). As the main proportion of 33P label in the plant is incorporated in roots, the 33P incorporated in the plant from gP (Figure 2D) could be detected the first time in harvested plant material by exposing the dried plant to the phosphor-imaging plate for 72 h.
The quantitative analysis of 33P activity (Figure 3) in plants showed the same trend as the images of harvested plant material. Moreover, the 33P activity was significantly lower in plants from mycorrhizal gP treatments compared to the other mycorrhizal P treatments. The non-mycorrhizal P treatments were evidently lower enriched in 33P than the mycorrhized counterparts, and the 33P activity for the non-mycorrhizal 4P+gP-33P and 1P+HAP-33P treatments was even under the detection limit. When comparing different plant parts, the leaves of 4P+AMP-33P treatment incorporated significantly (P < 0.03) more 33P compared with the 4P+oP-33P treatment and significantly higher amounts of 33P in leaves, stem, and substrate compared with 1P+HAP-33P treatment. The main proportion of 33P was found in the plant roots (>57%).
33P Recovery in Plant and Plant P Uptake Differ Between the Specific P Sources From a Mixed P Pool
In order to relate the 33P activity incorporated in plant material to the 33P activity of the initially applied P sources, we investigated the 33P recovery [%] in the harvested plant (Figure 4A; Supplementary Table 4). Furthermore, in order to standardize the different 33P activity added with different P amounts applied with each P source, we used the 33P activity [Bq] incorporated in plant material, and the specific 33P activity [kBq mg−1] of the initially applied P sources to determine the plant P uptake [μg] (Figure 4B; Supplementary Table 4) from the specific 33P labeled P source.
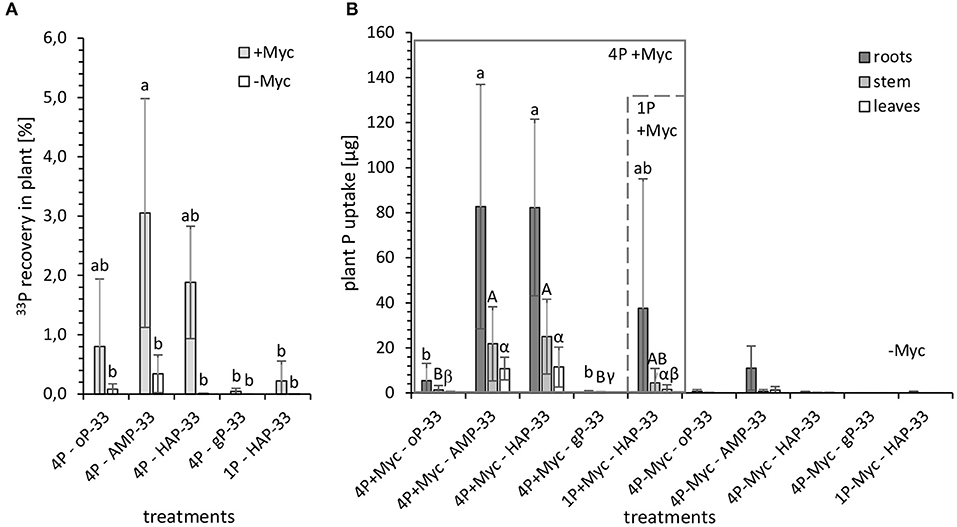
Figure 4. (A) 33P recovery [%] in plant and (B) plant P uptake [μg] from 33P labeled P sources from mycorrhizal (+Myc, n = 4) and non-mycorrhizal (-Myc, n = 3 for 4P-33P; and n = 2 for 1HAP-33P) rhizotrones equipped with P containers with different P sources: 4P experiment with simultaneous supply of four different P sources: oP, ortho-phosphate; AMP, adenosine monophosphate; gP, oP bound to goethite; HAP, synthesized hydroxyapatite; and 1P control experiment supplied with HAP. The error bars show the standard deviation. The letters (a, A, α) indicate significant differences between the P treatments of 4P-33P and 1HAP-33P experiments: P < 0.003 (see Supplementary Table 4 for more details).
The recovery of initially applied 33P in plant (Figure 4A) from the 33P-labeled P sources accounted to a maximum of 5%. Significantly (P < 0.03) higher 33P recovery could be observed in mycorrhizal plants grown in 4P rhizotrones with labeled AMP-33P (followed by HAP-33P and oP-33P) compared with gP-33P and single HAP-33P and non-mycorrhizal treatments. Although the initial P content of 1P rhizotrone system containing labeled HAP-33P as a single P source was 32% higher compared with the 4P system, the recovery of 33P in plant shoots (leaves and stems) was significantly lower (P < 0.03) from the former as compared to the latter.
Looking at the plant 33P uptake [μg] (Figure 4B) standardized by the initially applied P content, the uptake from HAP-33P as a single P source (44 ± 66 μg P) tended to be lower than from HAP-33P with the 4P system (119 ± 59 μg P), which is possibly due to the higher standard deviation in data from 1P+HAP-33P treatment. Furthermore, P taken up from AMP-33P (115 ± 73 μg P) and HAP-33P from a mixed P pool were similar. However, the P uptake from the readily available oP-33P (7.1 ± 1.6 μg P) and the more complex gP-33P (0.61 ± 0.76 μg P) was significantly lower (P < 0.03) compared with other P treatments. Furthermore, the calculated plant P uptake from the mineral P sources HAP and gP (max. 168 μg P and 1.6 μg P, respectively) was lower compared to the easy desorbable P (max. 224 μg P from HAP and 369 μg P from gP).
Discussion
The present study tested the suggestion highlighted in the framework of Turner (19) that the partitioning of different chemical P forms in the soil by plants is mediated by mycorrhizal fungi. For this purpose, we performed an experiment using an axenic rhizotrone culture system and the mycorrhizal associates of the poplar plant P. x canescens and the ectomycorrhizal fungus P. involutus. The system was supplied simultaneously with four different chemical forms of P in separate compartments so that only the mycorrhizal fungus had access to the P sources. To differentiate the mycorrhizal mediated plant P uptake between the different P sources, labeling with the radio-isotope 33P was applied. Finally, the obtained results from this experiment were compared with the mycorrhizal mediated plant P uptake from a mineral P form supplied as a single P source in the system to support the general assumption that a minor diversity in belowground resources, e.g., due to fertilization, resulting in a reduced uptake efficiency (18). We can confirm the previous findings of Andrino et al. (26, 27), as our compartmental system using nylon and hydrophobic membranes to separate the P sources from plant roots was a good choice to test mycorrhizal mediated plant P uptake. The small and negligible quantities of 33P activity detected in non-mycorrhizal treatments possibly result from “leakiness” due to the process of autoclaving of the P compartments to sterilize them and create axenic conditions inside the system. After the autoclaving, the membranes could not adhere so tight anymore at some weaker spots of the P compartment, which we did not examine in this study. The autoclaving of the P compartments was chosen, as it was possible to sterilize a vast amount of P compartments simultaneously and thereby avoid the use of any chemicals and safe time of the preparation of the culture systems.
Our results confirm a successful mycorrhizal mediated plant P uptake from the P compartments, independent of the P form taken up from a pool of differently available P sources or a single P source. The mycorrhizal mediated plant P uptake from the P compartments of the P treatments resulted in the gain in plant biomass of a similar magnitude as absolute plant P content compared to the mycorrhizal no P control treatment. Also, the incorporation of the 33P label (Figures 2–4) in mycorrhizal plants indicates a P uptake from all supplied P sources. Due to the axenic conditions prevalent in our system, we have excluded any competition with other microorganisms inhabiting the plant rhizosphere (40) and the fungal hyphosphere (41), which consume nutrients released by hyphae and root or also by feeding on hyphae (40, 42, 43), and which could reduce the control on the mycorrhizal fungal supply of P to plant. The images of harvested plants (Figure 2) showed areas with the highest 33P label incorporated in roots with high intensity in the branching of the root tips (Supplementary Figure 3), indicating that a high proportion of incorporated 33P label in the plant was detectable at the mycorrhizal fungus-root interface. This observation could result from a higher shielding of 33P by roots and shoots compared to the hyphal mantel around the root tips. In contrast to this assumption, the microradiographic localization of 33P in ectomycorrhizal poplar roots performed by Bücking and Heyser (44) has revealed that P taken up by hyphae accumulates rapidly in the hyphal mantel around the root tip and is slowly allocated through the Hartig net to the root cortical cells. Also, previous studies using excised roots detected an accumulation of P in the fungal sheet around the root tips and linked it to mycorrhizal fungal control on the amounts of P translocated to its host plant (11, 30). Nevertheless, results from experiments using excised plant roots should be handled with care, as they do not provide the same extent of a whole plant controlling its P demand and the P translocation by ECM fungus. The first successful attempt to describe the molecular processes of P transfer through the ectomycorrhizal Hartig net to plant roots under controlled conditions were made by the study of Becquer et al. (45). The H+:Pi transporter, HcPT2, of Hebeloma cylindrosporum was determined not only in the extra-radical hyphae, serving for the Pi entry into the mycelium, but also in the Hartig net, aiding for the transfer of Pi to the host plant Pinus pinaster. Thereby, the host plant was also found to induce the expression of HcPT2, revealing that the host plant is regulating its P supply by the mycorrhizal fungus.
Nevertheless, mycorrhizal fungal P uptake preferences in an axenic system should more closely reflect the potential capabilities (niches) (46, 47) in acquiring differently available P sources. The higher incorporation of the 33P label and especially the significantly higher plant P uptake from AMP compared to oP was surprising. We have also expected that the fungus would start the P acquisition from oP, but within a week (27–34 dpi), we have detected a simultaneous uptake of oP and AMP. Nevertheless, our results cannot approve the assumption that the mycorrhizae prefer or would start to acquire the readily available oP. Free Pi has to be just taken up from the solution via the plasma membrane phosphate transporters at fungal hyphae (40), whereby the mycorrhizal fungus would not need to apply any acquiring mechanisms, causing no costs for the P mining. In contrast, the AMP is assumed to require a phosphomonoesterase to release the phosphate ion (Pi) (19). Previous studies provide evidence that the ectomycorrhizal fungal species P. involutus can produce phosphatases, which are largely surface-bound (48, 49). The phosphatase activity is considered to be induced by the substrate or a low concentration of Pi (7). Furthermore, the results of Scheerer et al. (50) have proposed that AMP (and/or ADP) or at least the nucleoside adenosine consisting of ribose and adenine could be taken up by excised roots of poplar cuttings and beech seedlings via some nucleotide transporters of the root, which were not indicated to date. Their assumption was built due to the uptake rates of 13C and 15N but also of 33P of ATP tested as a P source for plant P uptake. We could not find experimental evidence for the uptake of the whole molecule of AMP or adenosine by mycorrhizal mycelium in the present literature. Still, it was already suggested by Rennenberg and Herschbach (51) that ectomycorrhizal fungi could take up organic P sources as a whole molecule. Many mycorrhizal species, including P. involutus, possess the ortholog of the yeast Porg transporter, ScGit1p (52). This transporter of Saccharomyces cerevisiae is upregulated under P limiting conditions and can import phospho-diesters (53). The activity of these transporters in ectomycorrhizal fungi/symbiosis was not identified to date and present a knowledge gap in the biochemistry of mycorrhizal plant P uptake. Nevertheless, this hypothesized mechanism could probably explain the mycorrhizal favoritism of AMP over oP in our study. The adenine could serve as an additional C and N source to the mycorrhizal fungal associate, reducing the dependency on C supply from the host plant.
However, the plasma membrane Pi transporters of ectomycorrhizal fungi of Basidiomecete are coupled with H+ symporter (54). Also, the Git1p was shown to function as an H+ symporter (55). Hence, with each imported Pi/Porg molecule, the P solution would lose one H+, by which the pH in the P solution would increase. Also, the performance of Git1p was shown to drop with increasing pH (56). Likewise, in our study, the pH (Supplementary Figure 6B) increased significantly in harvested AMP solution of mycorrhizal treatments compared to the non-mycorrhizal treatments but also compared to the pH of 5.2 set initially. Considering the higher incorporation of the 33P label from AMP, followed by oP and HAP, the pH increase in these P solutions in mycorrhizal treatments should be a consequent outcome.
On the one hand, if the activity of the Pi or Git1p transporters decreases with increasing pH, the similar plant P uptake from AMP and HAP could be explained by the pH increase in the AMP solution. We could detect the first incorporation of the 33P label in the plant from HAP only 1 week later compared to AMP and oP in the 4P system. On the other hand, HAP was supplied with a 2-fold higher P amount initially, which could indicate that besides the P source availability also the P amount of a P source is additionally essential for the choice of P uptake from a pool with different P sources by mycorrhizal fungus and its delivery to the plant. To underpin this assumption, shifts in nutritional strategies from acquisition (weathering of P rich primary minerals) to recycling (of accumulated P stocks in organic or secondary mineral P forms) were already observed from P-rich to P-poor temperate beech forests (7), suggesting that the most profuse P sources in an ecosystem are (of course depending on their bioavailability) the most favorable for the acquisition.
Inorganic P bound to metal oxides is considered not or only hardly accessible for P uptake by mycorrhizal plants and accumulating in the Lüss subsoil of forests limited in P (10). This was indebted to the high abundance of Fe and Al oxides in the Bw horizon and comprehensive adsorption of P to the mineral surface. Thus, it might not be surprising that the mycorrhizal mediated plant P uptake from gP was of the lowest magnitude compared to the other P sources supplied in the mixed pool. The desorption of Pi from goethite in H2O is affected by sorption-desorption equilibrium and diffusion transport (35). Further, low molecular organic anions (LMWOAs) increase the release and availability of Pi sorbed to goethite via ligand exchange. The ectomycorrhizal fungal genera Paxillus were described to release extensive amounts of LMWOAs (57, 58). P. involutus was also shown to produce and exude oxalate (59–61), which is suggested to be also a key component in apatite dissolution for the P uptake by ectomycorrhizal fungi and immobilization of Ca into Ca-oxalate (62). Furthermore, it was shown for arbuscular mycorrhizae that if the P source occurs in a not dissolved form, the P is more uniformly shared between the mycorrhizal associates (26). The higher retention of P in hyphae was considered to be a response to P bioavailability (63) and enabled the arbuscular mycorrhizal fungus to invest in the growth of its hyphae to reach the not soluble P sources (64, 65). However, the plant P uptake from gP (Figure 4B; Supplementary Table 4) was lower compared to the easy desorbable P from the same P source. Nevertheless, this parameter represents the maximal desorption rate of P bound to goethite supplied in dH2O suspended form, which was obtained by shaking the gP suspension for 24 h. In our study, the filled P compartments and the rhizotrone systems were handled with care and did not experience extensive shaking. We could instead attribute the lower P uptake from gP to its voluminous but at the same time compact consistency, which indirectly categorizes gP as a more complex P source within the mixed pool in our study. It has been suggested that ectomycorrhizal fungi can function as “biosensors” searching for nutrients from mineral sources and differentiate the mineralogy and grain sizes (61, 62). This could have lead P. invollutus to favor HAP over gP in our study. Nevertheless, for all these reasons, we can conclude that the later and lower P incorporation in the P. involutus ectomycorrhizal plant could be due to the higher complexity of gP as a P source and the lower 33P activity applied initially compared with other P sources.
The absolute P content in the plant and leaves of 4P treatments was significantly higher compared to the 1P treatment. This indicates that the fungus can supply the plant with more P either due to the presence of specific P forms in a mixed P pool or due to a general effect of P source diversity. To explain the latter effect of P source diversity with more favorable and readily available P sources besides the more complex P sources, we could assume that a mixed P pool might work as a “spiking” instrument to ensure an easier and faster familiarization of ectomycorrhiza to P sources with different bioavailabilities. Nevertheless, both statements are strongly supported by the significantly higher incorporation of the 33P label [kBq] from AMP and its significantly higher 33P recovery [%] in plant leaves compared to the 1P-HAP treatment. Higher P content in leaves of 4P plant treatments presumably allowed the earlier acquisition of HAP in 4P systems compared to the 1P system, as a higher C translocation to the HAP compartments is required to release Pi through exudation of organic anions (62). In contrast, photosynthesis is restricted under P limiting conditions (66), reducing at the same time the translocation of C to the below-ground infrastructure in order to mine the HAP patches. The lower P content in leaves of 1P treatment could result in lower photosynthetic fixation of CO2 that consequently could result in a lower C allocation to the mycorrhizal partner for the acquisition of HAP supplied as a single P source and could explain its later uptake. Nevertheless, there was no difference in plant P uptake from HAP supplied as a single P source or with different P sources, even though the amount of P supplied with HAP as a single P source was 1/3-fold higher compared with the sum of P amounts supplied with the four different P sources in the 4P system.
Conclusion
In the conducted rhizotrone experiment under axenic conditions, our results indicate that the representative ectomycorrhizal fungus P. x involutus was able to access all P sources (oP, AMP, HAP, and gP) we have supplied with the P pool, suggesting that this ectomycorrhizal fungus can act as a generalist in P acquisition. We assume a different weighting between the ectomycorrhizal species offering a playground for further research. Nevertheless, we confirm that specialization to the acquisition of one or a few specific P sources while having access to many in order to find a niche of an appropriate resource occur as an option or need due to competition for limited soil resources (67). Ectomycorrhizal fungi, compared with other P acquiring strategies of plants, could be unequally capable of occupying a broad variety of niches (68), which could explain the dominating occurrence of ectomycorrhizal fungi in many boreal (69–71) and temperate (70, 72, 73) forest ecosystems limited in P, controlling the occurrence of other species. Our results indicate that nutrient niching is possible in one species of ectomycorrhiza and that P resource diversity improves plant nutrition, providing supporting evidence for higher functional diversity by nutrient source partitioning.
In our study, from the mixed P pool, the sources AMP and HAP (over oP) were the most favorable to ectomycorrhizal mediated plant P uptake. We conclude that the magnitude of plant P uptake from a mixed pool containing P sources of different availabilities depends on the P species itself, additional nutrients coming with the specific P sources, and the complexity of the P source. In contrast, the order of uptake of different P forms depended on their complexity (oP and AMP over HAP and gP) and supplied diversity (HAP in a pool over HAP alone).
Even though we have used one mycorrhizal fungus and one plant only, to our knowledge, we are the first in showing that the ectomycorrhizal P. x canescens can occupy simultaneously fundamental niches of various P sources through the mediation of its mycorrhizal fungal partner P. involutus, indicating that the acquisition of differently available P sources by an ectomycorrhiza can facilitate niche plasticity and adaptation to specific nutrient limiting conditions.
Data Availability Statement
The original contributions presented in the study are included in the article, further inquiries can be directed to the corresponding author.
Author Contributions
JB, KS, AA, GG, AF, and LS designed the experiment. KS prepared the plant, fungal material, everything for the rhizotrone culture systems, performed the experiment, sample, data analysis, and wrote the paper. DH synthesized the hydroxyapatite and irrigated and imaged the systems from day 10 to 88 as well as performed LSC analysis of plant extracts. JB and GG supervised the research. All authors contributed to the article and approved the submitted version.
Funding
We want to thank the German Federal Ministry of Education and Research for the funding of this project in the framework of the DFG-RTG 1798 Signaling at the Plant-Soil Interface. The publication of this article was funded by the Open Access fund of Leibniz Universität Hannover.
Conflict of Interest
DH was employed by Institute of Bio- and Geosciences, IBG-3: Agrosphere, Forschungszentrum Jülich GmbH.
The remaining authors declare that the research was conducted in the absence of any commercial or financial relationships that could be construed as a potential conflict of interest.
Publisher's Note
All claims expressed in this article are solely those of the authors and do not necessarily represent those of their affiliated organizations, or those of the publisher, the editors and the reviewers. Any product that may be evaluated in this article, or claim that may be made by its manufacturer, is not guaranteed or endorsed by the publisher.
Acknowledgments
The authors are thankful for the great help and guidance received by Traud Winkelmann, Maximillian Koch, Stephan Köppchen, Nina Siebers, Jens Kruse, Anne Herwig, and Viola Rünzi. The plant material (P. x canescens clone Schleswig I) was kindly provided by Andreas Meier-Dinkel from the Nordwestdeutsche Versuchsanstalt (Germany). The ecto-mycorrhizal fungal material, P. involutus, was kindly provided by Andrea Polle, Abteilung Forstbotanik und Baumphysiologie (Büsgen-Institut, Georg-August Universität Göttingen, Germany).
Supplementary Material
The Supplementary Material for this article can be found online at: https://www.frontiersin.org/articles/10.3389/fsoil.2022.865517/full#supplementary-material
References
1. Plassard C, Dell B. Phosphorus nutrition of mycorrhizal trees. Invited review: part of an invited issue on tree nutrition. Tree Physiol. (2010) 30:1129–39. doi: 10.1093/treephys/tpq063
2. Elser JJ, Bracken MES, Cleland EE, Gruner DS, Harpole WS, Hillebrand H. Global analysis on nitrogen and phosphorus limitation of primary producers in freshwaters, marine, and terrestrial ecosystems. Ecol Lett. (2007) 10:1135–42. doi: 10.1111/j.1461-0248.2007.01113.x
3. Mengel K, Kirkby EA. Phosphorus. In: K Mengel, EA Kirkby, H Kosegarten, T Appel, editors, Principles of Plant Nutrition. Dordrecht: Springer (2001). p. 453479. doi: 10.1007/978-94-010-1009-2_9
4. Hinsinger P. Bioavailability of soil inorganic P in the rhizosphere as affected by root-induced chemical changes: a review. Plant Soil. (2001) 237:173–95. doi: 10.1023/A:1013351617532
5. Holford ICR. Soil phosphorus: its measurement, and its uptake by plants. Austr J Soil Res. (1997) 35:227–40. doi: 10.1071/S96047
6. Hinsinger P, Betencourt E, Bernard L, Brauman A, Plassard C, Shen J, et al. P for two, sharing a scarce resource: soil phosphorus acquisition in the rhizosphere of intercropped species. Plant Physiol. (2011) 156:1078–86. doi: 10.1104/pp.111.175331
7. Lang F, Krüger J, Amelung W, Willbold S, Frossard E, Bünemann E, et al. Soil phosphorus supply controls P nutrition strategies of beech forest ecosystems in Central Europe. Biogeochem. (2017) 136:5–29. doi: 10.1007/s10533-017-0375-0
8. Odum EP. The strategy of ecosystem development. Science (1969) 164:267–70. doi: 10.1126/science.164.3877.262
9. Walker TW, Syers JK. The fate of phosphorus during pedogenesis. Geoderma. (1976) 15:1–19. doi: 10.1016/0016-7061(76)90066-5
10. Klotzbücher A, Schunck F, Klotzbücher T, Kaiser K, Glaser B, Spohn M. Goethite-bound phosphorus in an acidic subsoil is not available to beech (Fagus sylvatica L.). Fron Forests Glob Change. (2020) 3:94. doi: 10.3389/ffgc.2020.00094
12. Owen D, Williams AP, Griffith GW, Withers PJA. Use of commercial bio-inoculants to increase agricultural production through improved phosphrous acquisition. Appl Soil Ecol. (2014) 86:41–54. doi: 10.1016/j.apsoil.2014.09.012
13. Jones MD, Durall DM, Tinker PB. A comparison of arbuscular and ectomycorrhizal Eucalyptus coccifera: growth response, phosphorus uptake efficiency and external hyphal production. New Phytol. (1998) 140:125–34. doi: 10.1046/j.1469-8137.1998.00253.x
14. Plassard C, Louche J, Ali MA, Duchemin M, Legname E, Cloutier-Hurteau B. Diversity in phosphorus mobilisation and uptake in ectomycorrhizal fungi. Ann Forest Sci. (2011) 68:33–43. doi: 10.1007/s13595-010-0005-7
15. Smith FA, Smith SE. What is the significance of the arbuscular mycorrhizal colonisation of many economically important crop plants? Plant Soil. (2011) 348:63–79. doi: 10.1007/s11104-011-0865-0
16. Landeweert R, Hoffland E, Finlay RD, Kuyper TW, van Breemen N. Linking plants to rock: ectomycorrhizal fungi mobilise nutrients from minerals. Trends Ecol Evol. (2001) 16:248–54. doi: 10.1016/S0169-5347(01)02122-X
17. Antibus RK, Sinsabaugh RL, Linkins AE. Phosphatase activities and phosphorus uptake from inositol phosphate by ectornycorrhizal fungi. Canad J Botany. (1992) 70:794–802. doi: 10.1139/b92-101
18. Zavišić A, Yang N, Marhan S, Kandeler E, Polle A. Forest soil phosphorus resources and fertilization affect ectomycorrhizal community composition, beech p uptake efficiency, and photosynthesis. Front Plant Sci. (2018) 9:463. doi: 10.3389/fpls.2018.00463
19. Turner BL. Resource partitioning for soil phosphorus: a hypothesis. J Ecol. (2008) 96:698–702. doi: 10.1111/j.1365-2745.2008.01384.x
20. Wassen MJ, Venterink HO, Lapshina ED, Tanneberger F. Endangered plants persist under phosphorus limitation. Nature. (2005) 437:547–50. doi: 10.1038/nature03950
21. Ceuleman T, Stevens CJ, Duchateau L, Jacquemyn H, Gowing DJG, Merck R, et al. Soil phosphorus constrains biodiversity across European grasslands. Glob Change Biol. (2014) 20:3814–22. doi: 10.1111/gcb.12650
22. Cleveland CC, Townsend AR, Taylor P, Alvarez-Clare S, Bustamante M, Chuyong G, et al. Relationships among net primary productivity, nutrients and climate in tropical rain forest: a pan-tropical analysis. Ecol Lett. (2011) 14:939–47. doi: 10.1111/j.1461-0248.2011.01658.x
23. Ahmad-Ramli MF, Cornulier T, Johnson D. Partitioning of soil phosphorus regulates competition between Vaccinium vitis-idaea and Deschampsia cespitosa. Ecol Evol. (2013) 3:4243–52. doi: 10.1002/ece3.771
24. Steidinger BS, Turner BL, Corrales A, Dalling JW. Variability in potential to exploit different organic phosphorus compounds among tropical montane tree species. Funct Ecol. (2014) 29:121–30. doi: 10.1111/1365-2435.12325
25. Liu X, Burslem DFRP, Taylor JD, Taylor AFS, Khoo E, Majalap-Lee N, et al. Partitioning of soil phosphorus among arbuscular and ectomycorrhizal trees in tropical and subtropical forests. Ecol Lett. (2018) 21:713–23. doi: 10.1111/ele.12939
26. Andrino A, Boy J, Mikutta R, Sauheitl L, Guggenberger G. Carbon investment required for the mobilization of inorganic and organic phosphorus bound to goethite by an arbuscular mycorrhiza (Solanum lycopersicum x Rhizophagus irregularis). Fron Environm Sci. (2019) 7:26. doi: 10.3389/fenvs.2019.00026
27. Andrino A, Guggenberger G, Sauheitl L, Burkart S, Boy J. Carbon investment into mobilization of mineral and organic phosphorus by arbuscular mycorrhiza. Biol Fertil Soils. (2021) 57:47–64. doi: 10.1007/s00374-020-01505-5
28. Pearson JN, Jakobsen I. The relative contribution of hyphae and roots to phosphorus uptake by arbuscular mycorrhizal plants, measured by dual labelling with 32P and 33P. New Phytol. (1993) 124:489–94. doi: 10.1111/j.1469-8137.1993.tb03840.x
29. Phoenix GK, Johnson DA, Muddimer SP, Leake JR, Cameron DD. Niche differentiation and plasticity in soil phosphorus acquisition among co-occuring plants. Nature Plants. (2020) 6:349–54. doi: 10.1038/s41477-020-0624-4
30. Hodge A. Accessibility of inorganic and organic nutrients for mycorrhizas. In: Johnson NC, Gehring C, Jansa J, editors. Mycorrhizal Mediation of Soil: Fertility, Structure, and Carbon Storage. Amsterdam: Elsevier (2017). p. 129–48.
31. Rajaniemi TK. Why does fertilization reduce plant species diversity? Testing three competition-based hypotheses. J Ecol. (2002) 90:316–24. doi: 10.1046/j.1365-2745.2001.00662.x
32. Rieger I, Kowarik I, Ziche D, Wellbrock N, Cierjacks A. Linkages between phosphorus and plant diversity in Central European Forest Ecosystems – complementarity or competition? Forests. (2019) 10:1156. doi: 10.3390/f10121156
33. Gafur A, Schutzendubel A, Langenfeld-Heyser R, Fritz E, Polle A. Compatible and incompetent Paxillus involutus isolates for ectomycorrhiza formation in vitro with poplar (Populus × canescens) differ in H2O2 production. Plant Biol. (2003) 6:91–9. doi: 10.1055/s-2003-44718
34. Müller A, Volmer K, Mishra-Knyrim M, Polle A. Growing poplars for research with and without mycorrhizas. Fron Plant Sci. (2013) 4:332. doi: 10.3389/fpls.2013.00332
35. Yan Y, Koopal LK, Liu F, Huang Q, Feng X. Desorption of myo-inositol hexakisphosphate and phosphate from goethite by different reagents. J Plant Nutrit Soil Sci. (2015) 2015:jpln.201500254. doi: 10.1002/jpln.201500254
36. Wolff J, Hofmann D, Amelung W, Lewandowski H, Kaiser K, Bol R. Rapid wet chemical synthesis for 33P-labelled hydroxyapatite – an approach for environmental research. Appl Geochem. (2018) 97:181–6. doi: 10.1016/j.apgeochem.2018.08.010
37. Giovannetti M, Mosse B. An evaluation of techniques for measuring vesicular arbuscular mycorrhizal infection in roots. New Phytolog. (1980) 84:489–500. doi: 10.1111/j.1469-8137.1980.tb04556.x
38. Brundrett M, Bougher N, Dell B, Grove T, Malajczuk N. Working with Mycorrhizas in Forestry and Agriculture. AClAR Monograph. Canberra, ACT: Australian Centre for International Agricultural (1996). p. 32.
39. IBM Corporation. IBM SPSS Statistics for Windows, Versions 24.0.2016 and 28.0.1.0. Armonk, NY: IBM Corporation (2016). p. 142.
40. Becquer A, Trap J, Irshad U, Ali MA, Claude P. From soil to plant, the journey of P through trophic relationships and ectomycorrhizal association. Fron Plant Sci. (2014) 5:548. doi: 10.3389/fpls.2014.00548
41. Warmink JA, Nazir R, van Elsas JD. Universal and species-specific bacterial “fungiphiles” in the mycospheres of different basidiomycetous fungi. Environm Microbiol. (2009) 11:300–12. doi: 10.1111/j.1462-2920.2008.01767.x
42. Nazir R, Warmink JA, Boersma H, van Elsas JD. Mechanisms that promote bacterial fitness in fungal-affected soil microhabitats. FEMS Microbiol Ecol. (2010) 71:169–85. doi: 10.1111/j.1574-6941.2009.00807.x
43. Ballhausen MB, Vandamme P, de Boer W. Trait differentiation within the fungus-feeding (mycophagous) bacterial genus Collimonas. PLoS ONE. (2016) 11:e0157552. doi: 10.1371/journal.pone.0157552
44. Bücking H, Heyser W. Microautoradiographic localization of phosphate and carbohydrates in mycorrhizal roots of Populus tremula x Populus alba and the implications for transfer processes in ectomycorrhizal associations. Tree Physiol. (2001) 21:101–7. doi: 10.1093/treephys/21.2-3.101
45. Becquer A, Garcia K, Amenc L, Rivard C, Dore J, Trives-Segura C, et al. The Hebeloma cylindrosporum HcPT2 Pi transporter plays a key role in ectomycorrhizal symbiosis. New Phytol. (2018) 220:1185–99. doi: 10.1111/nph.15281
46. McKane RB, Johnson LC, Shaver GR, Nadelhoffer KJ, Rastetter EB, Fry B, et al. Resource-based niches provide a basis for plant species diversity and dominance in arctic tundra. Nature. (2002) 415:68–71. doi: 10.1038/415068a
47. Hutchinson GE. Homage to Santa Rosalia, or why are there so many kinds of animals? Am Nat. (1959) 93:145–59. doi: 10.1086/282070
48. Alvarez M, Godoy R, Heyser W, Härtel S. Surface bound phosphatase activity in living hyphae of ectomycorrhizal fungi of Nothofagus obliqua. Mycologia. (2004) 96:479–87. doi: 10.2307/3762168
49. McElhinney C, Mitchell DT. Phosphatase activity of four ectomycorrhizal fungi found in a Sitka spruce–Japanese larch plantation in Ireland. Mycol Res. (1993) 97:725–32. doi: 10.1016/S0953-7562(09)80154-8
50. Scheerer U, Trube N, Netzer F, Rennenberg H, Herschbach C. ATP as phosphorus and nitrogen source for nutrient uptake by Fagus sylvatica and Populus x canescens Roots. Front Plant Sci. (2019) 10:378. doi: 10.3389/fpls.2019.00378
51. Rennenberg H, Herschbach C. Phosphorus nutrition of woody plants: many questions - few answers. Plant Biol. (2013) 15:785–8. doi: 10.1111/plb.12078
52. Plassard C, Becquer A, Garcia K. Phosphorus transport in mycorrhiza: how far are we? Trends Plant Sci. (2019) 24:794–801. doi: 10.1016/j.tplants.2019.06.004
53. Patton-Vogt J. Transport and metabolism of glycerophosphodiesters produced through phospholipid deacylation. Biochim Biophys Acta. (2007) 1771:337–42. doi: 10.1016/j.bbalip.2006.04.013
54. Nels U, Plassard C. Nitrogen and phosphate metabolism in ectomycorrhizas. New Phytol. (2018) 220:1047–58. doi: 10.1111/nph.15257
55. Pao SS, Paulsen IT, Saier MH. Major facilitator superfamily. Microbiol Mol Biol Rev. (1998) 62:1–34. doi: 10.1128/MMBR.62.1.1-34.1998
56. Almaguer C, Cheng W, Nolder C, Patton-Vogt J. Glycerophosphoinositol, a novel phosphate source whose transport is regulated by multiple factors in Saccharomyces cerevisiae. J Biol Chem. (2004) 279:31937–42. doi: 10.1074/jbc.M403648200
57. Courty PE, Franc A, Garbaye J. Temporal and functional pattern of secreted enzyme activities in an ectomycorrhizal community. Soil Biol Biochem. (2010) 42:2022–5. doi: 10.1016/j.soilbio.2010.07.014
58. Smith SE, Anderson IC, Smith FA. Mycorrhizal association and phosphorus acquisition: from cell to ecosystems. Ann Plant Rev. (2015) 48:409–40. doi: 10.1002/9781118958841.ch14
59. Lapeyrie F, Chilvers GA, Bhem CA. Oxalic acid synthesis by the mycorrhizal fungus Paxillus involutus (Batsch EX FR) FR. New Phytol. (1987) 106:139–46. doi: 10.1111/j.1469-8137.1987.tb04797.x
60. Lapeyrie F, Ranger J, Vairelles D. Phosphate-solubilizing activity of ectomycorrhizal fungi in vitro. Can J Bot. (1991) 69:342–6. doi: 10.1139/b91-046
61. Schmalenberger A, Duran AL, Bray AW, Bridge J, Bonneville S, Benning LG, et al. Oxalate secretion by ectomycorrhizal Paxillus involutus is mineral-specific and controls calcium weathering from minerals. Sci Rep. (2015) 5:12187. doi: 10.1038/srep12187
62. Leake JR, Duran AL, Hardy KE, Johnson I, Beerling DJ, Banwart SA, et al. Biological weathering in soil: the role of symbiotic root-associated fungi biosensing minerals and directing photosynthate-energy into grain-scale mineral weathering. Mineral Mag. (2008) 72:85–9. doi: 10.1180/minmag.2008.072.1.85
63. Ezawa T, Smith SE, Smith FAP. P metabolism and transport in AM fungi. Plant Soil. (2002) 244:221–30. doi: 10.1023/A:1020258325010
64. Olsson PA, Hammer EC, Wallander H, Pallon J. Phosphorus availability influences elemental uptake in the mycorrhizal fungus Glomus intraradices, as revealed by particle-induced X-ray emission analysis. Appl Environ Microbiol. (2008) 74:4144–8. doi: 10.1128/AEM.00376-08
65. Hammer EC, Pallon J, Wallander H, Olsson PA. Tit for tat? A mycorrhizal fungus accumulates phosphorus under low plant carbon availability. FEMS Microbiol Ecol. (2011) 76:236–44. doi: 10.1111/j.1574-6941.2011.01043.x
66. Carstensen A, Herdean A, Birkelund Schmidt S, Sharma A, Spetea C, Pribil M, et al. The impacts of phosphorus deficiency on the photosynthetic electron transport chain. Plant Physiol. (2018) 177:271–84. doi: 10.1104/pp.17.01624
67. Dickie IA. Host preference, niches and fungal diversity. New Phytol. (2007) 174:230–3. doi: 10.1111/j.1469-8137.2007.02055.x
68. McNaughton SJ, Wolf LL. Dominance and the Niche in ecological systems. Science. (1970) 167:131–9. doi: 10.1126/science.167.3915.131
69. Singer R, Morello JH. Ectotrophic forest tree mycorrhiza and forest communities. Ecol. (1960) 41:549. doi: 10.2307/1933331
70. Meyer FH. Distribution of ectomycorrhizae in native and manmade forests. In: GC Marks, TT Kozlowski, editors, Ectomycorrhizae: Their Ecology and Physiology. New York, NY: Academic Press (1973). p. 79–105. doi: 10.1016/B978-0-12-472850-9.50009-8
72. Moser M. Die ectotrophe Ernährungsweise an der Waldgrenze. Mitt Forstl Bundesversuchsanst Wien. (1967) 75:357.
Keywords: adenosine monophosphate, ectomycorrhizal fungi, goethite P complex, hydroxyapatite, P availability, P diversity, radioactive labeling, resource partitioning
Citation: Schreider K, Hofmann D, Boy J, Andrino A, Fernandes Figueiredo A, Sauheitl L and Guggenberger G (2022) Mycorrhizal Mediated Partitioning of Phosphorus: Ectomycorrhizal (Populus x canescens x Paxillus involutus) Potential to Exploit Simultaneously Organic and Mineral Phosphorus Sources. Front. Soil Sci. 2:865517. doi: 10.3389/fsoil.2022.865517
Received: 30 January 2022; Accepted: 25 February 2022;
Published: 04 April 2022.
Edited by:
Maqshoof Ahmad, The Islamia University of Bahawalpur, PakistanReviewed by:
Rui S. Oliveira, University of Coimbra, PortugalFarhan Hafeez, COMSATS University, Islamabad, Pakistan
Copyright © 2022 Schreider, Hofmann, Boy, Andrino, Fernandes Figueiredo, Sauheitl and Guggenberger. This is an open-access article distributed under the terms of the Creative Commons Attribution License (CC BY). The use, distribution or reproduction in other forums is permitted, provided the original author(s) and the copyright owner(s) are credited and that the original publication in this journal is cited, in accordance with accepted academic practice. No use, distribution or reproduction is permitted which does not comply with these terms.
*Correspondence: Katharina Schreider, c2NocmVpZGVyQGlmYmsudW5pLWhhbm5vdmVyLmRl