- 1Charlottetown Research and Development Centre, Agriculture and Agri-Food Canada, Charlottetown, PE, Canada
- 2Department of Soils and Agri-Food Engineering, Université Laval, Québec, QC, Canada
- 3Quebec Research and Development Centre, Agriculture and Agri-Food Canada, Québec, QC, Canada
- 4Normandin Research and Development Centre, Agriculture and Agri-Food Canada, Normandin, QC, Canada
Extracellular soil enzymes play a key role in soil organic matter decomposition and nutrient cycling. However, it is not fully understood how these enzymes respond to different land use. Long-term research studies were used to evaluate how diversified management practices affect extracellular enzymes driving C cycling [phenol oxidases (PO), peroxidases (PP), α-glucosidase (AG), β-glucosidase (BG), cellobiohydrolase (CB), β-1,-4-Nacetylglucosaminidase (NAG)], N cycling [leucine aminopeptidase (LAP)], and P cycling [phosphomonoesterase (PME)]. The soil pH, contents of total organic C, total N, mehlich-3 P, soil respiration and soil nitrogen supply capacity were also measured. Different land use included tillage frequency, tillage regimes, mineral N fertilization, crop rotations and liquid dairy manure. Compared to medium or high tillage frequency, low tillage frequency increased total organic C and total N and soil respiration as well as NAG and PME activities, whereas it decreased soil nitrogen supply, mehlich-3 P, and soil pH, as well as PO, PP, AG, BG, CB, and LAP activities. Non till was associated with lower PP and PO activities than moldboard plow. Nitrogen fertilization decreased soil pH and PO activity but increased PME activity. Barley (Hordeum vulgare) in rotation with forage increased total organic C, total N, soil nitrogen supply and soil respiration by 31, 21, 44, and 33%, respectively, in comparison with barley in monoculture. The application of liquid dairy manure increased soil pH, total N and soil nitrogen supply and soil enzyme activities (AG, BG, NAG) in comparison to the mineral N fertilizer. When principal component analysis was performed, soil pH, PO, PP, CB, LAP, and PME were grouped in the first component, which explained the highest variance. This is the core group controlling the C, N, and P cycling. The activities of C, N, and P acquiring enzymes, soil nitrogen supply and soil respiration were related to changes in soil total C and N, and extractable P contents across a broad range of management practices. Increased PO and PP activities reflect total C decline.
Introduction
Soil extracellular enzymes control the soil nutrient cycling (1, 2), and their activities have been suggested as important determinant of soil quality (3). Soil quality was defined as “the capacity of a soil to function, within ecosystem and land use boundaries, to sustain productivity, maintain environmental quality, and promote plant and animal health” (4). Soil quality is dynamic and therefore needs to be monitored over time and appropriate indicators depends on the objectives of the users (5). Rapid and consistent means to evaluate soil quality include physical (i.e., aggregation, available water capacity), chemical (i.e., soil pH and extractable nutrients), and biological (i.e., active organic matter, potentially mineralizable N) soil properties (6). Measurement of enzyme activity and carbon dioxide release reflect soil organic matter active fractions (5). A soil quality indicator should be universal and reflect changes under different land use.
Soil enzymes can be used as soil quality indicators and they reflect soil biology, are operationally practical with high throughput and low cost, are easy to quantify and they relate to agricultural management practices (7–9). Changes in soil management can be observed by measuring soil enzyme activities before changes in other soil quality indicators are noticeable (7).
Soil extracellular enzymes are primarily secreted outside the cell by soil microorganisms (10) or plant roots (11), and they can bind to either the cell or soil particles or diffuse freely in soils (11). Soil extracellular enzymes can be divided in two broad groups: (i) oxidative enzymes [such as phenol oxidases (PO), peroxidases (PP)] and (ii) hydrolytic enzymes [such as α-glucosidase (AG) β-glucosidase (BG), cellobiohydrolase (CB), β-1,-4-Nacetylglucosaminidase (NAG), leucine aminopeptidase (LAP) and phosphomonoesterase (PME)]. In soils, these enzymes are central to the degradation of organic compounds, thereby playing an important role in C, N, and P cycling (12).
Phenol oxidases (PO) are known to play a key role in the breakdown of polyphenols compounds in the presence of oxygen, whereas peroxidases (PP) use hydrogen peroxide (H2O2) (3, 12–14). The oxidation of polyphenols by these enzymes results in formation of radicals or quinones when phenolic hydrogen or hydrogens are removed (15). These radicals become stable by linking together, while quinones undergo nucleophilic addition reactions with other aromatic compounds and other compounds such as amino acids and amino sugars, leading to eventual production of humic acid-like polymers (15). Enhanced oxidative enzyme activity under soil management practices associated with soil organic C loss was reported (16). Oxidative enzymes were associated with microbial acquisition of N (17), and thus their activities are generally decreased with increased soil mineral N supply (18, 19). Phenol oxidases and PP activities were found to increase with soil pH (3).
The α-glucosidase (AG), β-glucosidase (BG), and cellobiohydrolase (CB) are enzymes that belong to a group of C-cycling enzymes called glycosidases that contribute to the degradation of cellulose and other α and β-1,4 glucans (3) to produce sugars, which is the main source of energy to soil microorganisms (20). The AG catalyzes the hydrolysis of α-D-glucopyranosides to release α-D glucose, the BG cleaves glucose from cellobiose (a cellulose dimer), and the CB cleaves cellobiose from cellulose molecules (3). Glycosidase activities were positively related to soil pH, and their activities were found to increase with increased soil organic C (21–23). High N availability would increase microbial demand for C, inducing therefore the production of glucosidases (24).
The β-1, 4-N-acetylglucosaminidase (NAG) is another key glycosidase enzyme involved in the degradation of chitin and other β-1,4-linked glucosamine polymers to release simple N-acetyl glucosamine units (25) and is mainly produced by fungi (26). This hydrolysis is reported to be important in C and N cycling, because NAG participates in the processes whereby chitin is converted to amino sugars, a major source of easily mineralizable C and N in soils (13, 20). The increased NAG activity was found to increase with fungal population which contains chitin in their cell walls (27). The activity of NAG has been positively related to soil organic C and total N. High inorganic N availability was reported to decrease NAG activity (28). Soil pH was positively related to NAG activity (21).
Leucine aminopeptidase (LAP) is an exopeptidase, secreted by microbes, and involved in the hydrolytic release of leucine from peptides (29), playing therefore an important role in N cycling. The increased activity of aminopeptidases was associated to the increase in proteins in soils (30, 31). Protease and peptidase activities were reported to highly influence the rate of organic N mineralization (32) as proteinaceous material represents 40% of total N in soil (31). Decreased LAP activity was reported under high inorganic N availability (23). The LAP activity was positively related to soil pH (3).
Phosphomonoesterase (PME) (i.e., acid and alkaline phosphatases) is the most active soil phosphatase (33). Phosphomonoesterase hydrolyzes phosphomonoesters and, in some cases, phosphodiesters to release assimilable phosphates for microbes and plants (3) playing an important role in organic P cycling (20). The production of PME by plant roots and microorganisms increases when P is limiting (26). Phosphatases are significantly affected by soil pH, which also controls phosphorus availability in soil (20). Acid phosphatases were negatively related to soil pH while alkaline phosphatases were positively related to soil pH (22). Also variations in PME activity were linked to soil organic C (13). Increased phosphatase activities under high inorganic N availability (28, 34) were reported. The relationships between substrates and/or end products and extracellular enzyme activities are complex and are not fully understood and may vary depending on the enzyme.
The production of hydrolytic enzymes related to C, N and P cycles (i.e., glycosidases, proteases, phosphatases) is induced by the presence of their substrates (26), and high enzyme activity can indicate nutrient limitation (26). However, the presence of intermediate and end-products of hydrolysis may repress enzyme activity (26), leading mostly to a negative relationship between nutrient concentrations and enzyme synthesis. Besides, there is another hypothesis that when labile carbohydrates molecules are abundant, the decomposition of more recalcitrant organic compounds is inhibited (35, 36). In fact, enzyme production by soil microbes represent a high cost for nutrients and energy, that can be otherwise used for their growth and metabolism (37). However, that expense is only justified when available nutrients are scarce (38), and consequently, soil microbes will release enzymes to mineralize complex components (37). The above explains the “economic theory of microbial metabolism.” To better manage soil nutrient management, we need to improve our understanding of how enzymes respond to different agricultural management practices.
Improving and maintaining soil quality are key drivers to sustain crop yields and environmental quality (39). Soil quality can be improved through diverse management practices including reducing tillage frequency, growing cover crops, crop rotations and organic and inorganic amendments. There is a growing interest in identifying sensitive indicators of the soils' physical-, chemical- and biological properties (40). Soil enzymes were reported to be more sensitive to respond to changes induced by land use than other soil quality indicators (7). Nevertheless, contradicting information exist in the literature on how the enzyme activities respond to land use. For instance, tillage effect was reported to increase biochemical soil activity due to increased surface area by breaking macroaggregates (41, 42) while others reported decreased enzyme activities due to a decreased organic matter content (43, 44). In addition, mineral and organic fertilizer input was associated with increased biochemical soil activity (45, 46) whereas other reported decreased activity (47, 48). While readily available inorganic nutrients may inhibit enzyme synthesis (49), it may also stimulate it through enhanced plant growth and increased enzymes released by roots (50). It is therefore of paramount importance to increase our understanding on the potential of extracellular enzyme activities to respond to a broad range of soil management practices.
Using long-term experimental sites (>20 years), the objectives of this study were to assess the effects of tillage frequency levels, tillage regimes, mineral and organic N fertilization and crop rotation on the potential activity of eight soil extracellular enzymes involved in C cycling (PO, PP, AG, BG, CB, NAG), N cycling (LAP), and P cycling (PME). Total organic C and N, extractible P, soil pH, soil N supply capacity (SNS, the initial nitrate levels plus nitrate released after an incubation of 2 weeks) and soil respiration (CO2 released after 24 h of incubation) were also measured, and their relationship with extracellular enzymes were assessed. The CO2 released following rewetted soil was reported to derive from microbial respiration and the remainder was a consequence of enzyme activity (51) and thus it reflects metabolic microbial activity, whereas the SNS was reported to be a promising N availability index to reflect the mineralizable N pools over a growing season under temperate soils with cold soil in spring (52). The intent is not to compare measured parameters across the experiments as it is expected to have a high range of values given the differences in management practices, cropping histories and soil types but rather to analyze data separately per each experiment in order to better understand nutrients (C, N, P) and enzyme responses to different land use and how they are linked to soil pH, CO2 and SNS. We hypothesized that: (i) oxidative activities of C related enzymes (PO, PP) will be enhanced under land use associated with TOC decline, and with addition of mineral N; (ii) hydrolytic activities of C related enzymes (AG, BG, CB) will be higher under land use that will enhance TOC; (iii) land use that will enhance TOC and TN are expected to enhance bacterial and fungal activities and will enhance NAG activity; (iv) LAP activity will be increased under land use favoring TN increase; (v) PME activity will be enhanced under land use associated with low extractible mehlich-3 P.
Materials and Methods
Description of Experimental Sites and Treatments
Different Tillage Frequency Levels at Field Scale in Prince Edward Island (PEI), Canada
The PEI soil quality monitoring project (SQM) was initiated in 1998 by the PEI Department of Agriculture and Land to identify the trends in nutrients using georeferenced points across the province. Sampling protocol is described in detail by Nyiraneza et al. (53). Briefly, under the SQM project, a total of 232 agricultural-land sampling locations were selected based on 4 × 4 km National Forestry Inventory (NFI) grid (54). Based on archived information on the cropping history, a total of 60 sites (i.e., 30 sites in 2018 and 30 sites in 2019) having different tillage frequency levels were selected for this study (Supplementary Figures 1A,B): low tillage frequency (forests and continuous grasslands), medium (4 yrs of cereal rotations) and high (3 yrs of potato rotation system with potatoes being grown once every 3 yrs). Each year, 10 sites in low-, medium, and high tillage frequency were selected for a total of 30 sites per year, implying that each tillage frequency level is replicated 10 times per each year. Five sampling points were chosen at each site, located at the intersecting point on the grid and 20 m at the north, south, east and west of the intersecting point. In addition, the crops grown were recorded since 1998, and thus it was possible to identify differences in soil tillage frequency across sites.
Tillage Regimes, N and P Fertilizers Application at L'Acadie Experimental Site
This experimental site was established in 1992 at the L'Acadie Experimental Farm of Agriculture and Agri-Food Canada, located in the province of Quebec, Canada (45°18 ′N, 73°21 ′W), on clay loam soil (Orthic Humic Gleysol; 364 g clay, 432 g silt, 204 g sand kg−1 dry soil) under a corn–soybean rotation. The experimental layout was a split-plot design with two tillage practices (conventional vs. no-till) as the main plots and nine fertilizer combinations (0, 80 and 160 kg N ha−1 in a factorial with 0, 17.5 and 35 kg P ha−1) randomized as sub-plots, all replicated in four randomized blocks for a total of 72 plots. The 160 kg N ha−1 and 35 kg P ha−1 corresponded to the local recommendations for grain corn. All details were reported by Ziadi et al. (55). For the purpose of this study, we used only two N application rates (0 and 160 kg N ha−1) as the sub-plots, for a total of 16 experimental units (2 N application rates × 2 tillage regimes × 4 replications = 16). The conventional tillage treatments consisted of mouldboard plow at 20 cm depth after harvest in the fall, followed by disking and harrowing to 10 cm each spring before seeding. For the no-till treatments, the plots were ridge-tilled from 1992 to 1997 and no-tilled since 1998. For all the treatments, crop residues were left on the ground after harvest. Mineral fertilizers were applied only to the corn phase of the rotation, except in spring 2020 due to Covid-19 pandemic. Nitrogen was band-applied as urea at seeding rates of 0 and 40 kg N ha−1, and side-dressed as ammonium nitrate at 0 and 120 kg N ha−1 at the eight-leaf stage. All plots received the same amount of P (35 kg P ha−1) as triple superphosphate at planting.
Crop Rotation, Mineral and Organic Fertilization at Normandin Research Farm
This experimental site was established in the fall of 1989 in the Lac St-Jean region of Quebec, Canada, on a silty clay soil as a fixed-rotation experiment at the Agriculture and Agri-Food Canada research farm in Normandin (48° 50′ N, 72° 33′ W). The experimental design was a factorial split-split plot with four replications with crop rotation as the main plots (barley monoculture vs. underseeded barley with 2-years forage production), tillage practices as the sub-plots (conventional vs. minimum tillage), and nutrient source as sub-sub-plots (mineral vs. organic fertilizers) for a total of 64 plots. All details were reported by Lafond et al. (56). For the purpose of this study we considered only two crop rotation systems (barley monoculture vs. underseeded barley with 2 yrs forage production) and two nutrient sources (mineral vs. organic fertilizers) under the minimum tillage regime for a total of 16 experimental units (2 rotational crops ×2 nutrient sources ×4 replications = 16). The crop rotation types consisted of a continuous spring barley (“Chapais”) monoculture and a 3 yr barley–forage rotation and were established in the spring of 1990. The barley rotation consisted of 1 yr of spring barley underseeded with a mixture of perennial forages, followed by 2 yr of forage production. The forage crops were timothy (Phleum pratense L. “Champ”) and red clover (Trifolium pratense L. “Prosper”) from 1990 to 1999, and thereafter timothy was replaced by orchardgrass (Dactylis glomerata L. “Okay”). Barley was managed as a grain crop. All selected plots were under chisel plow to a depth of 15 cm after harvest in the fall. Mineral fertilizers were broadcasted and incorporated immediately before barley seeding. The mineral fertilizer treatment on the barley plots provided 70 kg N ha−1 as NH4NO3, 40 kg P2O5 ha−1 as triple superphosphate, and 70 kg K2O ha−1 as KCl. The forage plots under mineral fertilizer received, on average, 74 kg total N ha−1yr−1 for the first application and 46 kg total N ha−1yr−1 for the second after the first cut of hay as NH4NO3, 40 kg P2O5 ha−1as triple superphosphate, and 140 kg K2O ha−1 as KCl. Liquid dairy manure, as organic fertilizer, was surface-applied and provided different amounts of macro nutrients. The amount of nutrients applied from liquid dairy manure are illustrated in Table 1.
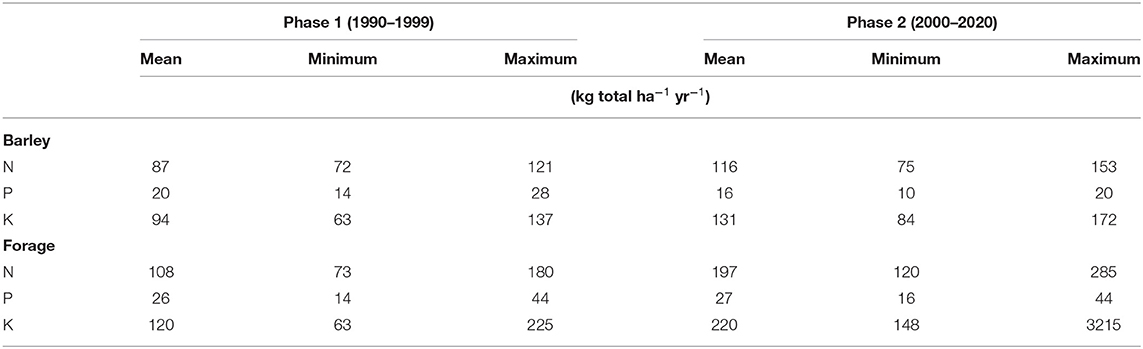
Table 1. Mean, minimum, and maximum amount of nutrients applied from liquid dairy manure at Normandin site.
Soil Properties Analyses and Extracellular Enzyme Assays
Soil samples were collected in May 2018 and 2019 at 0–15 cm soil depth (PEI experimental site), in September 2020 at 0–20 cm soil depth (Normandin experimental site), and in November 2020 at 0–20 cm soil depth (L'Acadie experimental site). Samples from each plot represented a composite of four subsamples taken using a soil probe of 2.0 cm diameter.
Across all experiments, a subset of fresh soil sample was passed through a 4.75-mm sieve immediately after soil sampling, used to determine soil moisture content, and analyzed for mineral N (NO3-N). The second portion of each soil sample was air dried, sieved through a 4.75-mm sieve and analyzed for soil pH and mehlich-3 extractable P (M3-P). The third portion of each soil sample was air dried, ground, sieved through a 500 μm sieve and analyzed for total organic carbon (TOC) and total nitrogen (TN). The fourth portion of each soil sample was air dried, sieved through a 4.75-mm sieve, rewetted and analyzed for mineralized N (flush of NO3-N). A fifth portion of each soil sample was air dried, sieved through a 2.00-mm sieve and analyzed for soil respiration (flush of CO2). A fifth set of each soil samples was frozen at −20°C until enzyme analyses.
Selected soil basic properties and potential activities of selected extracellular enzymes were determined on soil samples from all plots where different land use were implemented. Soil pH was measured using a 1:1 soil to water ratio (57). Total C and N were quantified by dry combustion method (58) using an Elementar analyzer (Vario Max, Elementar Analyzer, Hanau, Germany). Soil extractable P was determined with Mehlich-3 extraction solution (59, 60) followed by inductively coupled plasma optical emission spectroscopy (ICP; VARIAN 820-MS, Varian Inc. Scientific Instruments, Mulgrave, Victoria). To assess soil N supply (SNS) capacity, the extraction of mineral N (NO3-N) was performed using 2 M KCl (61); and the N mineralization potential (NO3-N flush) based on 14-d aerobic incubation of rewetted dried soil at 25°C was measured, as described by Sharifi et al. (62). Both soil filtrates and leachates were analyzed for concentrations of NO3-N colorimetrically using flow injection analysis on a Latchat QuickChem 8500 (Latchat Instruments, Loveland, CO). The SNS was then estimated as initial extracted NO3-N plus NO3-N extracted following a 14-d aerobic incubation. To determine soil respiration, the extraction of CO2 released following a 24-h incubation by rewetting dried soil (CO2 flush) was performed using the capillary method described by Haney and Haney (63). Carbon dioxide was analyzed using a Li-COR gas analyzer (Li-Cor-Li-830-850, Li-COR Biosciences, Lincoln, Nebraska, US) and expressed as μg CO2-C g soil−1 day−1.
We assayed the activities of two oxidase enzymes (PO and PP) following the protocol described by the Center for Dead Plant Studies (2000) with minor modifications. Briefly, soil suspensions were prepared by thoroughly mixing 1.0 g of soil in 50 mL of 50 mmol L−1 sodium acetate buffer solution (pH 5.0). The activities of PO and PP were measured by combining 200 μL of soil suspension with 50 μL of substrate solution (25 mmol L−1 l-3,4-dihydroxyphenylalanine [DOPA, Sigma-Aldrich Canada] into the transparent 96-well plates. Both negative controls (200 μL of sodium acetate buffer + 50 μL of DOPA solution) and blanks (200 μL of soil suspension + 50 μL of 50 mmol L−1 sodium acetate buffer) were added in each microplate. For the PP plates, all wells (including the control wells) also received 10 μL of 0.3% H2O2. The contents of the microplates were incubated at 25°C for 20 h. After incubation, the absorbance was then immediately read using a microplate spectrophotometer reader at 460-nm. Enzyme activities were calculated and expressed as micromoles per hour per gram (μmol h−1 g−1) as described in the protocol by The Center for Dead Plant Studies (2000). Certain studies normalized PO and PP activities per TOC (64, 65) contents and others expressed PO and PP activities per g of soils (2, 16, 64–66). We did not normalize the latter enzymes activities per TOC to be consistent with other hydrolase enzyme activities in this study. Detailed information on enzyme analyses and their substrates are found in Table 2.
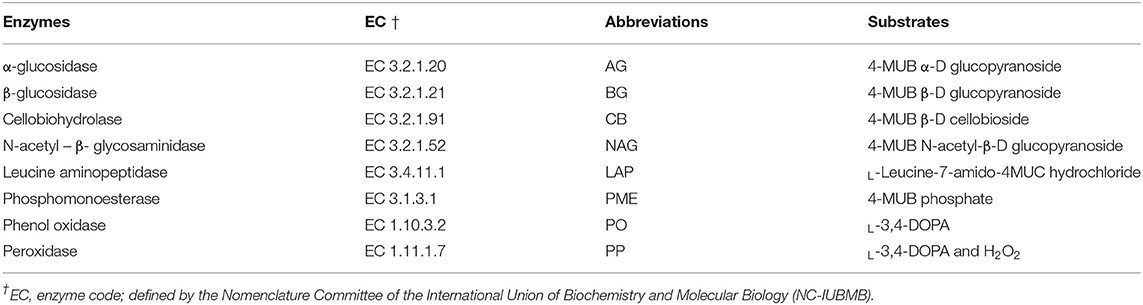
Table 2. Extracellular enzymes assayed in soil collected from three different study sites, their enzyme code (EC), abbreviations used in this study and corresponding substrates.
We also assayed the activities of six hydrolase enzymes (AG, BG, CB, NAG, LAP, and PME) according to the fluorometric microplate protocol by Bell et al. (67). Briefly, soil slurries of each soil sample were prepared by homogenizing 2.75 g of field moist soil and 91 mL of 50 mM sodium acetate buffer solution (pH 5.7) into a 100-mL glass beaker by a stir plate at 350 rpm. A quantity of 800 uL of homogenous soil slurry was added into corresponding wells of MUB (4- MUB: 4 – Methylumbelliferyl) and MUC (4- MUC: 4-Methylcoumarin) standard (i.e., MUB for AG, BG, CB, NAG, PME, and MUC for LAP) and sample plates using an 8-channel pipette. The MUB and MUC sample plates were then inoculated with 200 μL of 200 μM MUB—glucopyranoside L-1, 200 μM—MUB glucopyranoside L-1, 200 μM-MUB cellobioside L-1, 200 μM- MUB N-acetyl-β-D glucopyranoside L-1, 200 μM—MUC hydrochloride, and 200 μM- MUB phosphate that were assumed non-limiting (Table 1). The MUB and MUC standard plates were also prepared by combining 800 μL of soil slurry with 200 μL of MUB or MUC standard solution, respectively, at increasing concentrations (0, 2.5, 5, 10, 25, 50, and 100 μmol L−1). Each assay microplate also contained two columns of blanks (one column of soil homogenate blanks and one column of acetate buffer blanks) for measuring background fluorescence in the substrate. All plates were then sealed and incubated in the absence of light at 25°C for 1.5 h, except for LAP, which was incubated at 4°C for 24 h. After the incubation period, the plates were centrifuged for 3 min at 2,900 rpm; 250 μL from each well of the MUB standard and sample plates and that of MUC standard and sample plates were transferred into corresponding wells of a black flat-bottomed 96-well plate in order to be read. Fluorescence intensity was measured on a microplate reader (Synergy HTX, BioTek Instruments, Inc., Winooski, VT) with 360/40 nm excitation and 460/40 nm emission (center wavelength/bandpass) filters following the instructions from the BioTek® Synergy HTX Multi-Mode Reader (67). From these fluorescence values, we calculated enzyme activity as the rate of substrate converted in nmol activity h−1 g dry soil−1 (nmol h−1g−1). Detailed information on AG, BG, CB, NAG, LAP, and PME enzymes and their substrates are found in Table 2.
Statistical Analysis
To analyze the effect of main factors on selected soil properties and extracellular soil enzyme activities, we used the MIXED procedure of SAS (SAS version 13.2, SAS Institute, North Carolina, 2014) by considering tillage frequency levels, tillage practice types, N fertilization, crop rotation types and N fertilization sources as fixed effects, and blocks or replicates as random effects (68). At the Acadie site, the experimental design was treated as a split- plot design with the main effects being tillage regimes and the sub-plot being mineral N fertilization regimes replicated four times. At the Normandin site, data was analyzed based on a split-plot design with the main plot being crop rotations and the sub-plot being N sources replicated four times. For the study on tillage frequency levels in PEI, statistical analyses were performed considering a completely randomized design with three treatments (low, medium, high) with 10 replicates in 2018 and 2019. Undisturbed sites (forests and continuous grasslands) were used as replicates and so were sites under a 4 year cereal rotation (medium tillage frequency) and sites with a 3 year potato rotation (high tillage frequency). PEI is entirely underlain by a terrestrial sandstone formation with soil derived from glacial till being mainly sandy and well-drained (69) (Supplementary Table 1). Based on the scatter plots using row data for TOC, TN, soil pH, and M3-P (Supplementary Figures 2A,B), and considering that PEI soils are relatively uniform being dominated by Podzolic soil order (Supplementary Figure 1C), we assume that the differences among these sites are more attributed to the tillage frequency than geological differences. All data were tested for normality using the PROC UNIVARIATE of SAS before ANOVA (68). Any variables that were not normally distributed were transformed to meet the assumptions of ANOVA. The DIFF option of SAS was used for treatment means comparisons when the main treatment effect was significant at the probability level of 0.05. Pearson's correlation coefficients between soil properties and extracellular enzyme activities were calculated using the CORR procedure of SAS (68). A principal component analysis (PCA) was performed using correlations of Euclidian distances and data were standardized prior to PCA analysis. All statistical analyses were performed separately per each experimental design. In addition overall means from all experiments were used to analyze correlation among soil enzyme activity and soil properties and to perform PCA. A flow chart summarizing data processing is reported in Supplementary Figure 3.
Results
Effect of Tillage Frequency on Soil Properties and Extracellular Enzymes Activities
Tillage frequency had significant effects on soil pH, TOC, TN, M3-P, SNS, and CO2 (Tables 3, 4). Organic acids released by root exudates in low tillage frequency sites contributed to decrease soil pH. Conversely, liming at medium and high tillage frequency sites contributed to increase soil pH.
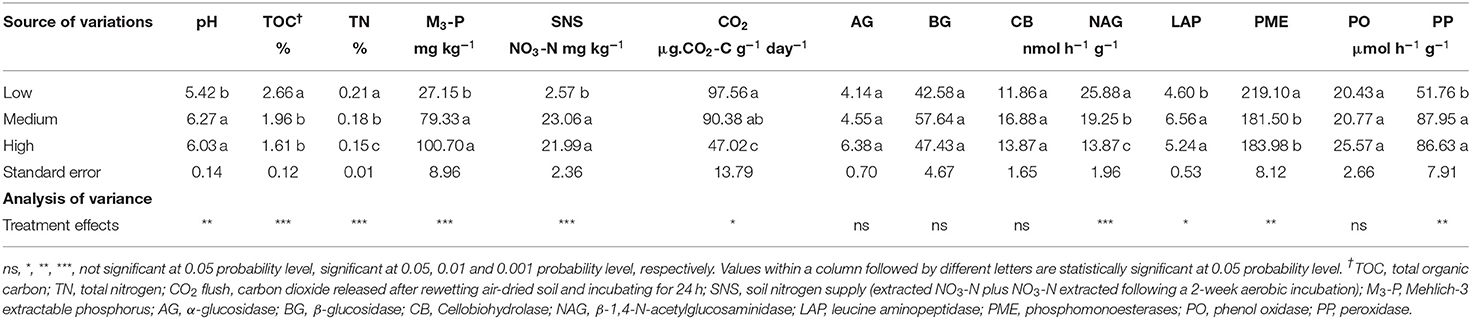
Table 3. Effect of tillage frequency on selected soil properties and extracellular enzyme activities at 0–15 cm soil depth at PEI site in 2018.
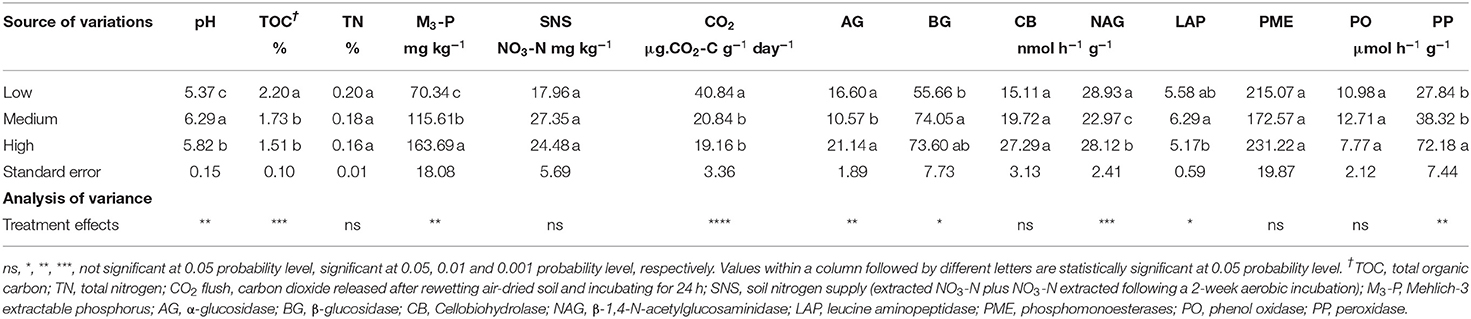
Table 4. Effect of tillage frequency on selected soil properties and extracellular enzyme activities at 0–15 cm soil depth at PEI site in 2019.
The TOC values were significantly lower in medium- and high- tillage frequency sites than low tillage frequency sites in both 2018 and 2019 (Tables 3, 4). Total N content was highest in low- tillage frequency sites (0.21%), followed by medium- (0.18%) and high- (0.15%) tillage frequency in 2018. However, no significant difference was observed in 2019. The concentration of M3-P was significantly higher in medium- and high tillage frequency sites compared to low tillage frequency sites in both sampling years (Tables 3, 4). Soil nitrogen supply estimated as initial NO3-N plus that released after a 14-d incubation of a rewetted soil was higher in medium- and high tillage frequency sites than low tillage frequency sites in 2018, but no significant difference was observed in 2019. Soil respiration estimated as CO2 released after a 24-h incubation on a rewetted soil was higher in low- tillage frequency sites than medium- and high tillage frequency sites in both sampling years (Tables 3, 4).
The activities of oxidative (PO, PP) and hydrolytic (AG, BG, CB, NAG) enzymes related to C cycling were significantly affected by tillage frequency, with some exceptions (Tables 3, 4). The PO activity was comparable between tillage frequency levels in both 2018 and 2019. The activity of PP in medium-and high-tillage frequency sites was comparable but higher than that of low-tillage frequency sites in 2018, while in 2019, the PP activity was higher under high tillage frequency than under medium and low tillage frequency sites (Tables 3, 4).
The activity of AG was comparable between tillage frequency levels in 2018, whereas, in 2019, the AG activity was significantly higher in high- tillage frequency sites than in medium tillage frequency sites. The activity of BG was comparable between tillage frequency levels in 2018, whereas BG activity was highest in medium tillage frequency sites in 2019, followed by high frequency and low frequency sites. There was no statistically significant difference between tillage frequency levels for CB activity in both 2018 and 2019. The activity of NAG was significantly higher in low tillage frequency sites in 2018, followed by medium tillage frequency and high tillage frequency. However, in 2019, NAG showed high activity in low- tillage frequency sites, followed by high frequency and medium frequency sites (Tables 3, 4).
The activity of extracellular enzyme related to N cycling (LAP) was comparable between medium- and high- tillage frequency sites but higher than that of low tillage frequency sites in 2018, while in 2019, the LAP activity was highest in medium-tillage frequency sites, followed by low frequency and then high frequency sites (Tables 3, 4).
The activities of extracellular enzymes involved in the P cycling (PME) were higher in low- tillage frequency sites than medium- and high tillage frequency sites in 2018, whereas, in 2019, PME activities were comparable between tillage frequency levels (Tables 3, 4).
Effect of Tillage Regimes and Mineral N Fertilization on Soil Properties and Extracellular Enzymes Activities
The effect of tillage regimes was not statistically significant on soil pH, whereas N fertilization significantly influenced soil pH, with average values of 6.28 at the rate of 160 N kg ha−1 and 6.71 at zero N fertilization, corresponding to a decrease of 0.43 units (Table 5). Ammonium-based N fertilizer contributed to decrease soil pH. For the remaining measured soil properties (TOC, TN, M3-P, SNS, soil respiration) there were no statistically significant differences between tillage regimes and N fertilization rate (Table 5). The interaction between tillage systems and N fertilization was not statistically significant for all measured soil properties (Table 5).
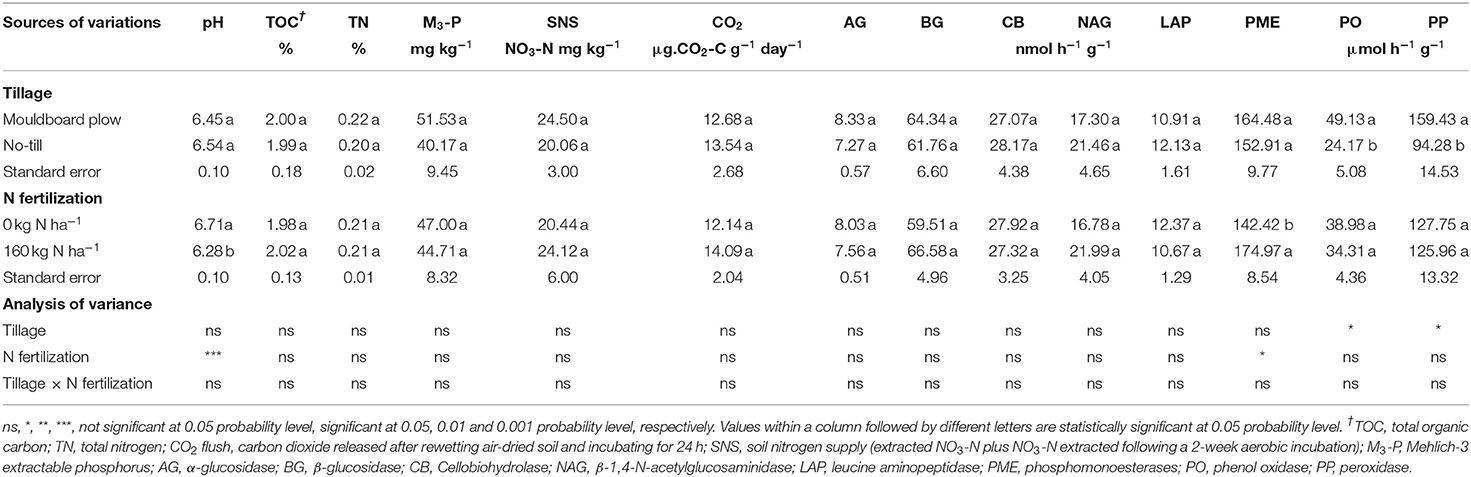
Table 5. Effects of tillage regimes and nitrogen fertilization on selected soil properties and soil extracellular enzyme activities at 0–20 cm soil depth at Acadie site in 2020.
The activities of PO and PP were not affected by N fertilization. In contrast, tillage regimes statistically decreased the PO and PP activities. The PO activity was 49.13 μmol h−1g−1 under moldboard plow plots and 24.71 μmol h−1g−1 under no-till plots, corresponding to a decrease of 50% in no-tilled soils (Table 5). The PP activity was 159.43 μmol h−1g−1 under moldboard plow plots and 94.28 μmol h−1g−1 under no-till plots, corresponding to a decrease of 41% in no-tilled soils (Table 5). The interaction between tillage systems and N fertilization was not statistically significant (Table 5).
The activities of both C cycling (AG, BG, CB, NAG) and N cycling (LAP) hydrolytic enzymes were comparable between tillage and N fertilization treatments, and there were no interaction effects between tillage systems and N fertilization rates for these enzymes (Table 5).
The hydrolytic activity of PME enzyme was not affected by tillage treatments, while it showed significantly higher activity under N fertilization treatment in comparison to zero N application. Activities of PME increased by an average of 23% in N fertilized soils. The interaction between tillage systems and N fertilization was not statistically significant (Table 5).
Effect of Rotation and N Fertilizer Sources on Soil Properties and Extracellular Enzymes Activities
Rotation treatments did not impact all measured soil properties (soil pH, TOC, TN, M3-P, SNS, soil respiration) (Table 6). However, the N fertilizer sources had statistically significant effects on some soil properties including soil pH, TN and SNS (Table 6). There were no interaction effects between rotation types and N fertilizer sources for all measured soil properties (Table 6). Soil pH values, TN content and SNS were significantly higher in liquid dairy manure than in mineral N treatment (Table 6). On average, soil pH value was 6.48 in manure and 6.06 in mineral fertilizer, corresponding to a decrease of 0.42 units in the mineral fertilizer treatment. Total N content was 0.22% in manure and 0.20% in mineral fertilizer, corresponding to 10% of increase in the liquid dairy manure treatment. Soil N supply was 51.50 NO3-N mg kg−1 in manure and 40.46 NO3-N mg kg−1 in mineral fertilizer, corresponding to an average increase of 27% in the liquid dairy manure treatment (Table 6).
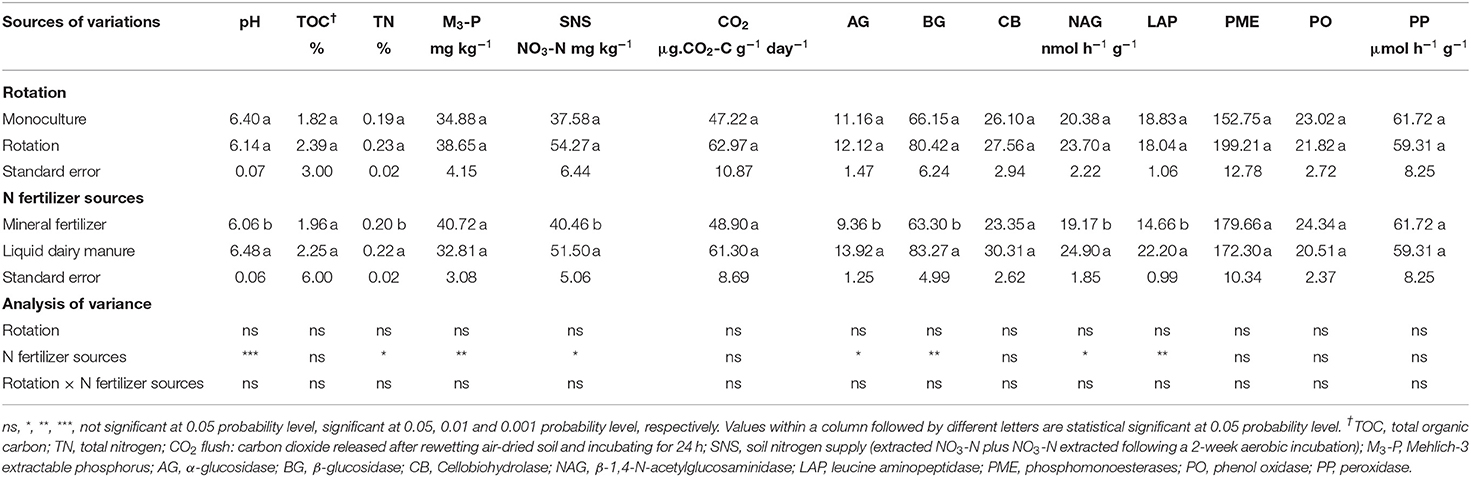
Table 6. Effect of crop rotation types and N fertilizer sources on selected soil properties and soil extracellular enzyme activities at 0–20 cm soil depth at Normandin site.
The activities of PO and PP were not affected by crop rotation and N fertilizer sources (Table 6). The activities of AG, BG, CB, and NAG were comparable between crop rotation treatments. However, these enzymes were significantly affected by N fertilizer sources, except for CB activity. The activities of AG, BG and NAG were significantly higher under liquid dairy manure treatment compared to mineral fertilizer treatment. The AG activity was 13.92 nmol h−1 g−1 (manure) and 9.36 nmol h−1 g−1 (mineral fertilizer), corresponding to an increase of 48%. The BG activity was 83.27 nmol h−1 g−1 (manure) and 63.30 nmol h−1 g−1 (mineral fertilizer), corresponding to an increase of 32%. The NAG activity was 24.90 nmol h−1 g−1 (manure) and 19.17 nmol h−1 g−1 (mineral fertilizer), corresponding to an increase of 30% (Table 6).
The activity of LAP was not affected by crop rotation, whereas it was significantly affected by N fertilizer sources. The LAP activity was 22.20 nmol h−1 g−1 (manure) and 14.66 nmol h−1 g−1 (mineral fertilizer), corresponding to an increase of 51% (Table 6). The activities of PME were not affected by either crop rotation and N fertilizer sources (Table 6).
Correlations Between Soil Properties and Extracellular Enzyme Activities
The Pearson's correlation coefficient was used to assess the relationship between soil pH, TOC, TN, M3-P, SNS, and soil respiration with extracellular enzyme activities. Soil pH was positively related to PO, PP, CB, and LAP activities, and negatively related to NAG and PME activities (Table 7). Total N was positively related to LAP activity, and M3-P was negatively related to LAP. The SNS was positively related to BG, CB, and LAP activities. The soil respiration was negatively correlated with CB activity.
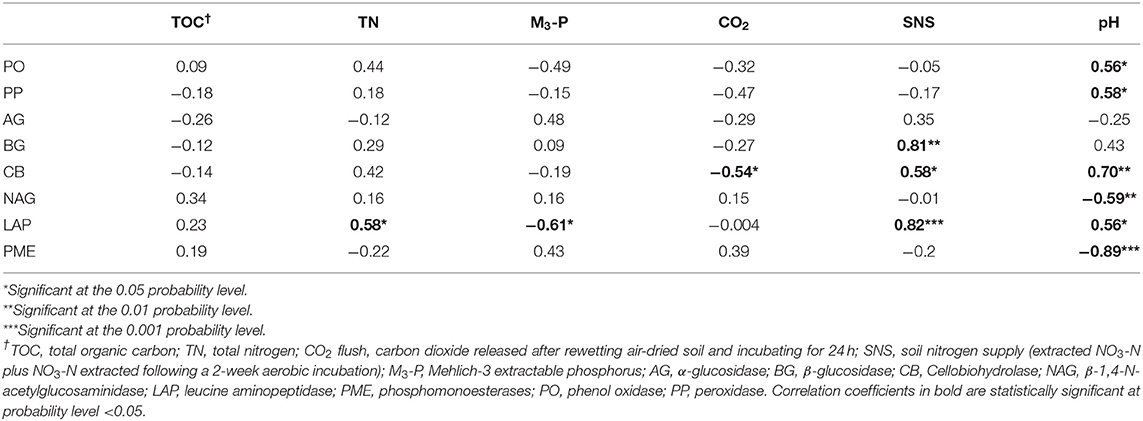
Table 7. Correlation coefficients between extracellular enzyme activities and soil properties using overall means from all study sites.
Principal Component Analysis (PCA)
Multivariate analysis was performed by pooling together overall means from all experiments using 14 parameters including soil pH, TOC, TN, M3-P, SNS, soil respiration, PO, PP, AG, BG, CB, NAG, LAP, PME. Three principal components (PCs) were obtained, which accounted for 83% of the total variance. Examination of the loading values suggests that PC1 (36.22% of the total variance) was associated primarily with pH, CB, LAP, PME, PO, and PP (Table 8). The PC2 (24.86% of the total variance) was associated with SNS, AG, BG and NAG (Table 8). The PC3 (21.55 % of the total variance) was associated with TOC, TN, soil respiration and M3-P (Table 8). A bi-plot (Supplementary Figure 4) showed positive interrelationships between C, N and P contents as well as positive interrelationships between hydrolytic enzymes driving C, N and P cycling. It was also shown positive interrelationships between oxidative enzymes, whereas, negative relationships between hydrolytic and oxidative enzymes were observed.
Discussion
Carbon Cycling Oxidative Extracellular Enzymes (PO, PP) as Impacted by Land Use
The observed PO and PP values are within the range of values reported previously in different studies (2, 12, 64, 66). We hypothesized that oxidative activities of C related enzymes (PO, PP) will be enhanced under land use associated with TOC decline, and with addition of mineral N. This hypothesis was verified. The later enzymes tended to be higher under medium or high tillage frequency in comparison to low tillage frequency. This increase of PO and PP activities under high frequency tilled sites is attributed to the loss of soil organic matter, specifically labile organic C compounds (12) such as carbohydrates, since medium- and- high tillage frequency plots were frequently tilled, while the low-tillage frequency plots had not been tilled for at least 5 years, some much longer or never tilled. The decreased TOC and TN concentrations under medium- and high-tillage frequency likely occurred due to removal of crop residues through cereal and forage crops harvest and increased mineralization of TOC through frequent tillage (70–73). Waldrop et al. (74) also found an increase of PO activity in pineapple plantations in comparison to native tropical forest (74). Other studies also found a positive relationship between PO activity and organic C loss (16).
The same trend with enhanced PO and PP activities was observed under plots without mineral N addition in comparison to mineral N application even though the difference was not statistically significant at 5% probability level. Previous reports suggested that mineral N fertilizer supply stimulates the mineralization of easily decomposable organic C compounds such as carbohydrates and preserves the decomposition of recalcitrant phenolic compounds (65, 74, 75), repressing therefore PO and PP activities (16, 34, 64). Nitrogen fertilization is known to increase TOC and soil N supply, and in this study TOC content and soil N supply capacity tended to be higher in N fertilized soils compared to zero N plots, justifying therefore the low oxidative activity following N addition compared to zero N application. Study by Gallo et al. (64) in northern temperate forest soils showed decreased PO activity by 33 to 73% and PP activity by 5 to 41% after N addition (30 and 80 kg ha−1 yr−1). Increased activities of PO and PP under conventional tilled soils in comparison to no-tilled soils are mostly linked to the loss of soil organic matter (12).
Increased oxidative enzyme activities was observed with barley in monoculture in comparison to barley rotated with the forage legumes. Oxidative enzymes that mineralize C from recalcitrant phenolic compounds are expected to be lower under barley rotation, suggesting the inhibition of recalcitrant phenolic compounds decomposition when abundant labile C compounds are present (35, 36) as predicted by the economic theory of microbial metabolism. The low oxidative activity under barley rotation with legumes is explained by higher TOC content compared to barley in monoculture. Barley in rotation increased TOC by 31% and TN by 21%. Activities of PO and PP were 21 and 17%, respectively, lower under barley in the rotation in comparison with barley in monoculture.
When soil management practices are accompanied with TOC decline and thus low active carbon, the oxidative enzymes which degrade phenolic compounds are increased to satisfy soil microbe energy requirement. Mineral N fertilization contributes to inhibiting oxidative enzyme activities because it may stimulate the soil microbial activity and thus the production of labile organic compounds.
Carbon Cycling Hydrolytic Extracellular Enzymes (AG, BG, CB, NAG) as Impacted by Land Use
Our second hypothesis stated that hydrolytic activities of C related enzymes (AG, BG, CB) will be higher under land use that will enhance TOC was partially verified. Activities of AG, BG and CB were lower under low tillage frequency even if this was associated with higher TOC than medium and high tillage frequency. The presence of intermediate and end-products of hydrolytic reactions catalyzed by these enzymes may have repressed their activities under low tillage frequency sites. However, BG activity was increased under barley in the rotation compared to barley in monoculture.
Liquid dairy manure application increased AG and BG activities which could be attributed to manure-induced changes to soil chemical and physical properties, including increased soil pH and macro-and micronutrient supplies and/or the microbial (76) and enzymes load in manure. Also compared to mineral fertilizers, organic manure was reported to improve TOC content and organic matter status, which in turn could be revealed in higher hydrolytic activity related to C and N cycling (175, 175). This result is supported by greater TOC and TN concentrations in manured than mineral fertilized soils.
The third hypothesis was verified and stated that agricultural practices that will enhance TOC and TN are expected to enhance bacterial and fungal activities and will enhance NAG activity. The activity of NAG was higher under the low tillage frequency sites in comparison to medium and high tillage frequency sites, and under plots that received liquid dairy manure compared to mineral fertilized plots. The presence of substrates (chitin from fungi cell walls) induced the production of NAG. Previous studies also found higher NAG activity in low tillage frequency sites than medium- and high-tillage frequency soils (20, 77). In contrast, the lower NAG activity in medium- and high-tillage frequency sites could be explained by N fertilizer application under intensive cropping systems (26). Increased soil microbial activity under low-tillage frequency sites could be justified by increased concentration of CO2 flush/soil respiration. Franzluebbers et al. (78) and Franzluebbers and Arshad (79) reported a close relationship between flush of CO2 and cumulative C mineralization, potentially mineralizable C and N, and soil microbial biomass C.
Nitrogen Cycling Extracellular Enzyme (LAP) as Impacted by Land Use
We hypothesized that LAP activity will be increased under land use favoring TN increase. This hypothesis was partially verified. LAP activity was lower in low-tillage frequency sites compared with medium- and high-tillage frequency sites. Given that the TN was higher in the low tillage frequency sites than in the high frequency ones, and that proteins can account for 40% of total nitrogen (31), we would expected that proteinaceous compounds are at greater amount under low-sites than high tillage frequency sites. Therefore, LAP could have been inhibited by the presence of proteins or by abundance of end-products (amino acids) in the low tillage frequency sites. In addition, the LAP activity could have been inhibited under low soil pH (5.42) in low tillage frequency sites as it was reported that the optima soil pH for proteolytic enzymes range from 7 to 9 (3). Soil N supply was higher in medium- and high-tillage frequency sites than low-tillage frequency sites suggesting that N was limiting in the former system. Increased soil N supply in intensive cropping systems was more likely caused by mineral N fertilizer addition.
Liquid dairy manure significantly increased LAP activity than mineral N along with TN content. Application of liquid dairy manure can increase soil organic N content, including proteins, peptides, amino acids and urea, which will change the concentration of the substrate as well as the relative ratio of organic N to inorganic N (80). This finding is supported by higher TN content in manured soils compared to mineral fertilized plots. Our results corroborate the findings of other studies, which showed increased LAP activity following manure application (2, 81).
Phosphorus Cycling Extracellular Enzyme (PME) as Impacted by Land Use
The hypothesis that stated that PME activity will be enhanced under land use associated with low M3-P was verified. PME activity showed higher values in low-tillage frequency sites than in medium-and high-tillage frequency sites. This result is supported by low concentration of mehlich-3 extractable P in low tillage frequency sites. Application of inorganic P can inhibit phosphatase activities in soil by repressing the synthesis of phosphatase (28, 82, 83), and thus P was limiting in low tillage frequency sites. In addition, abundance of root biomass as well as different plant species found in low- tillage frequency sites (forests and continuous grasslands) may also have influenced PME activity as PME is also secreted by plants (83, 84). Previous studies showed higher PME activities under low-tillage frequency compared to high-tillage frequency systems (9, 85, 86).
Nitrogen fertilization at a rate of 160 kg N ha−1 increased significantly PME activity by 23% in comparison to zero N addition. This result supports the hypothesis that N fertilization would increase the activity of extracellular enzymes associated with P cycling. Sufficient N supply appears to sustain soil microbial communities to produce more extracellular enzymes associated with hydrolytic P acquisition (28, 34). Mehlich-3 extractable P tended to be lower under N fertilized plots compared to the control, confirming high PME when available P is scarce. Under phosphorus-deficient conditions, there is secretion of acid phosphatase by plant roots (87) and this may also contributed to increased PME activity under N fertilized plots.
Correlation Between Extracellular Enzymes Activities and Soil Properties
A positive relationship between TN and LAP activity was observed implying that LAP is stimulated by the presence of the substrate. In addition, a positive relationship between SNS and BG and CB activities was also observed, suggesting the importance of inorganic nitrogen availability in stimulating the production of these enzymes for cellulose degradation, as reported in previous studies (14, 34).
Soil pH was positively related to PO, PP and CB and negatively related to NAG and PME. Positive relationships between soil pH and PO, PP, and CB implies that the activities of these enzyme are enhanced with liming whereas the activity of NAG and PME is enhanced under acidic to sub-acidic conditions. The soil pH in this study ranged from 5.37 to 6.71 and we could assume that acidic PME was predominant than alkaline PME which is encountered under alkaline soils. A study by Acosta-Martínez and Tabatabai (22) reported positive correlations between soil pH and enzymes involved in C, N, and S cycling but a negative relationship between soil pH and acid phosphatase. The optimum soil pH for acidic phosphomonoesterase and NAG was reported to be between pH 4.0 and pH 5.0 (33). A negative relationship between soil pH and PME confirms severe P limitations for plants and soil microbes in acidic soils, resulting in increased production of PME by both soil microorganisms and plant roots in order to obtain inorganic P from organic P compounds. The relationships between enzymes and soil pH can change across studies depending on the pH range of sampled soils and whether the assay used adjusts the soil pH to match the enzyme pH optima or whether a single buffer is used for multiple enzymes like is done with fluorometric substrates.
When PCA was performed, soil pH, PO, PP, CB, LAP, and PME activities were grouped in the first component and could represent the core group driving C, N and P cycling. The core group highlights the importance of soil pH in affecting enzyme activities and the role of oxidases in degrading phenolic compounds and those enzymes releasing simple sugars (CB) and amino acids (LAP) as well the enzyme releasing assimilable phosphates.
The second group was related to SNS, AG, BG, and NAG and shows the important role of nitrogen in stimulating C and N related hydrolytic enzymes. Increased mineral N availability could increase microbial demand for C, inducing therefore the production of EEs related to C acquisition (25, 34). Also, under C limited conditions, NAG activity may be increased to promote organic C mineralization to help microbes meet their C demands (25).
The third group was composed of TOC, TN, M3-P and CO2 flush, and highlights the linkage between C, N and P cycling. It is well-known that the contents of soil organic C and nutrients (e.g., N and P) are generally related, and increased soil N and P contents following increased accumulation of soil organic C were reported (2, 88). Carbon and nutrient (N, P) cycles are inherently linked via different processes such as photosynthesis, respiration and mineralization (89). Also, soil organic C, nitrogen and phosphorus are stabilized together inside soil organic matter, and during decomposition, the microbial need for C can cause microbial P mineralization when soil microorganisms use organic P compounds as a C source (90). Thus, the C-N-P cycles are linked even though the mechanisms driving biological C and N mineralization, and biochemical P mineralization could be different. The cycles of soil C and P are coupled via microbial and root activity including the release of extracellular phosphatase enzymes that allow the mineralization of P from soil organic matter (91). A diagram summarizing key results of C, N, P and enzymes responses to tested management practices is reported in Figure 1.
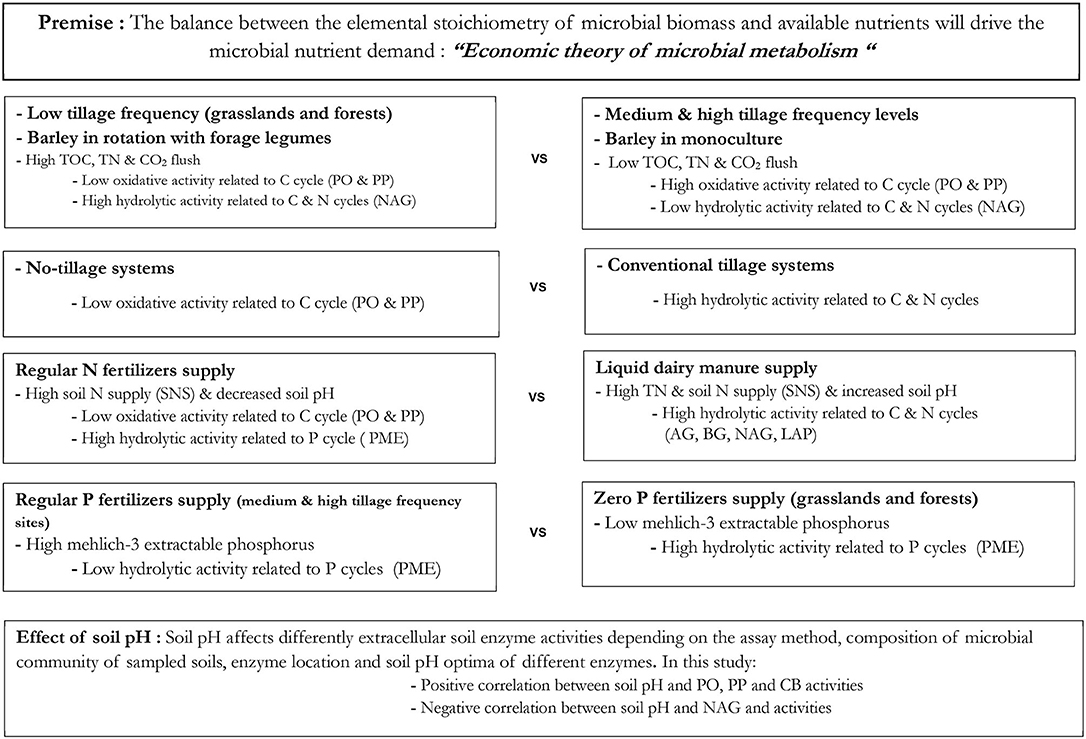
Figure 1. Conceptual diagram summarizing the C, N, and P contents and extracellular enzyme responses to tested land-use. TOC, total organic carbon; TN, total nitrogen; CO2 flush, carbon dioxide released after rewetting air-dried soil and incubating for 24 h; SNS, soil nitrogen supply (extracted NO3-N plus NO3-N extracted following a 2-week aerobic incubation); M3-P, Mehlich-3 extractable phosphorus; AG, α-glucosidase; BG, β-glucosidase; NAG, β-1,4-N-acetylglucosaminidase; LAP, leucine aminopeptidase; PME, phosphomonoesterases; PO, phenol oxidase; PP, peroxidase.
Conclusion
This study assessed how different extracellular enzymes respond to different land use. The activities of C, N, and P acquiring enzymes, soil nitrogen supply and soil respiration were related to changes in soil total C, total N and extractable P across different land use. Hydrolytic C related enzymes (AG, BG, CB) are good indicators of carbon flows, whereas oxidative C enzyme (PO, PP) are good indicators of C concentrations with increased PP and PO activity reflecting total C decline. The NAG activity is an indicator of enhanced fungal activities and increased PME activity reflects low extractible P. Enzyme activities were sensitive to management practices than total C and total N under different land use. The relationship between enzymes activities and C, N and P availability is complex given that C, N and P cycling are linked. In addition, the mechanisms by which enzyme substrates, enzyme end products and nutrient availabilities affect different enzyme activities still need to be elucidated. Soil pH affects differently extracellular soil enzyme activities depending on the assay method, composition of microbial community of sampled soils, enzyme location and soil pH optima of different enzymes.
Data Availability Statement
The datasets analysed in this study are available upon reasonable request directed to the corresponding author JN (anVkaXRoLm55aXJhbmV6YUBhZ3IuZ2MuY2E=).
Author Contributions
JN and JD-R provided advice on the study conceptualization. YU performed sample analyses, data analysis, and manuscript writing. TF, NZ, and JL were the leads of long-term research trials, they managed the sites, and coordinated soil samplings. All authors contributed to data interpretation and provided critical feedbacks on the manuscript.
Funding
This study was funded by Agriculture and Agri-Food Canada.
Conflict of Interest
The authors declare that the research was conducted in the absence of any commercial or financial relationships that could be construed as a potential conflict of interest.
Publisher's Note
All claims expressed in this article are solely those of the authors and do not necessarily represent those of their affiliated organizations, or those of the publisher, the editors and the reviewers. Any product that may be evaluated in this article, or claim that may be made by its manufacturer, is not guaranteed or endorsed by the publisher.
Acknowledgments
The authors acknowledge the field, laboratory, and technical assistance provided by Danielle Murnaghan, Irene Power, Barbara Enman, Gregory Dorothy, Sandy Jenkins, Taylor Main, and Hannah Arseneault. The authors are also thankful to PEI Department of Agriculture and Land, PEI landowners as well as to Kyra Stiles for her guidance in site selection.
Supplementary Material
The Supplementary Material for this article can be found online at: https://www.frontiersin.org/articles/10.3389/fsoil.2022.814554/full#supplementary-material
Abbreviations
AG, α-glucosidase; BG, β-glucosidase; CB, cellobiohydrolase; LAP, leucine aminopeptidase; NAG, β-1,-4-Nacetylglucosaminidase; PME, phosphomonoesterase; PO, phenol oxidases; PP, peroxidases; TN, total nitrogen; TOC, total organic carbon.
References
1. Maharjan M, Sanaullah M, Razavi BS, Kuzyakov Y. Effect of land use and management practices on microbial biomass and enzyme activities in subtropical top-and sub-soils. Appl Soil Ecol. (2017) 113:22–8. doi: 10.1016/j.apsoil.2017.01.008
2. Nyiraneza J, Vernon R, Yvonne U, Fraser TD, Erin S, Fillmore S, et al. Long-term manure application effects on nutrients and selected enzymes involved in their cycling. Soil Sci Soc Am J. (2018) 82:1404–14. doi: 10.2136/sssaj2017.12.0437
3. Sinsabaugh RL, Lauber CL, Weintraub MN, Ahmed B, Allison SD, Crenshaw C, et al. Stoichiometry of soil enzyme activity at global scale. Ecol Lett. (2008) 11:1252–64. doi: 10.1111/j.1461-0248.2008.01245.x
4. Doran JW, Jones AJ. Methods for Assessing Soil Quality. SSSA Spec. Publ. 49. Madison, WI: SSSA (1996).
5. Snapp SS, Morrone VL. Soil quality assessment. In: Logsdon S, Clay D, Moore D, Tsegaye T, editors. Soil Science Step-by-Step Field Analysis. Madison, WI: Soil Science Society of America (2008). p. 79–96.
6. Gugino BK, Abawi GS, Idowu OJ, Schindelbeck RR, van Es HM, Wolfe DW, et al. Cornell Soil Health Assessment Training Manual, Edition 2.0. Geneva, NY: Cornell University (2009).
7. Balota EL, Chaves JCD. Enzymatic activity and mineralization of carbon and nitrogen in soil cultivated with coffee and green manures. Rev Brasil Ciência Solo. (2010) 34:1573–83. doi: 10.1590/S0100-06832010000500010
8. Hussain S, Siddique T, Saleem M, Arshad M, Khalid A. Impact of pesticides on soil microbial diversity, enzymes, and biochemical reactions. Adv Agron. (2009) 102:159–200. doi: 10.1016/S0065-2113(09)01005-0
9. Karaca A, Cetin SC, Turgay OC, Kizilkaya R. Soil enzymes as indication of soil quality. In: Shukla G, Varma A, editors. Soil Enzymology. Heidelberg; Dordrecht; London; New York, NY: Springer (2010). p. 119–48.
10. Skujinš J, Burns RG. Extracellular enzymes in soil. CRC Crit Rev Microbiol. (1976) 4:383–421. doi: 10.3109/10408417609102304
11. Burns RG, DeForest JL, Marxsen J, Sinsabaugh RL, Stromberger ME, Wallenstein MD, et al. Soil enzymes in a changing environment: current knowledge and future directions. Soil Biol Biochem. (2013) 58:216–34. doi: 10.1016/j.soilbio.2012.11.009
12. Sinsabaugh RL. Phenol oxidase, peroxidase and organic matter dynamics of soil. Soil Biol Biochem. (2010) 42:391–404. doi: 10.1016/j.soilbio.2009.10.014
13. Luo L, Meng H, Gu J-D. Microbial extracellular enzymes in biogeochemical cycling of ecosystems. J Environ Manage. (2017) 197:539–49. doi: 10.1016/j.jenvman.2017.04.023
14. Matocha CJ, Haszler GR, Grove JH. Nitrogen fertilization suppresses soil phenol oxidase enzyme activity in no-tillage systems. Soil Sci. (2004) 169:708–14. doi: 10.1097/01.ss.0000146023.53936.6c
15. Martin JP, Haider K. A comparison of the use of phenolase and peroxidase for the synthesis of model humic acid-type polymers. Soil Sci Soc Am J. (1980) 44:983–8. doi: 10.2136/sssaj1980.03615995004400050022x
16. Carreiro MM, Sinsabaugh RL, Repert DA, Parkhurst DF. Microbial enzyme shifts explain litter decay responses to simulated nitrogen deposition. Ecology. (2000) 81:2359–65. doi: 10.1890/0012-9658(2000)081[2359:MESELD]2.0.CO;2
17. Craine JM, Morrow C, Fierer N. Microbial nitrogen limitation increases decomposition. Ecology. (2007) 88:2105–13. doi: 10.1890/06-1847.1
18. Fog K. The effect of added nitrogen on the rate of decomposition of organic matter. Biol Rev. (1988) 63:433–62. doi: 10.1111/j.1469-185X.1988.tb00725.x
19. Kirk TK, Farrell RL. Enzymatic “combustion”: the microbial degradation of lignin. Annu Rev Microbiol. (1987) 41:465–505. doi: 10.1146/annurev.mi.41.100187.002341
20. Acosta-Martinez V, Cruz L, Sotomayor-Ramirez D, Pérez-Alegría L. Enzyme activities as affected by soil properties and land use in a tropical watershed. Appl Soil Ecol. (2007) 35:35–45. doi: 10.1016/j.apsoil.2006.05.012
21. Deng SP, Tabatabai MA. Effect of tillage and residue management on enzyme activities in soils. II Glycosidases. Biol Fertil Soils. (1996) 22:208–13. doi: 10.1007/BF00382514
22. Acosta-Martínez V, Tabatabai MA. Enzyme activities in a limed agricultural soil. Biol Fertil Soils. (2000) 31:85–91. doi: 10.1007/s003740050628
23. Shi Y, Sheng L, Wang Z, Zhang X, He N, Yu Q. Responses of soil enzyme activity and microbial community compositions to nitrogen addition in bulk and microaggregate soil in the temperate steppe of Inner Mongolia. Eurasian Soil Science. (2016) 49:1149–60. doi: 10.1134/S1064229316100124
24. Asmar F, Eiland F, Nielsen NE. Effect of extracellular-enzyme activities on solubilization rate of soil organic nitrogen. Biol Fertil Soils. (1994) 17:32–8. doi: 10.1007/BF00418669
25. Sinsabaugh RL, Gallo ME, Lauber C, Waldrop MP, Zak DR. Extracellular enzyme activities and soil organic matter dynamics for northern hardwood forests receiving simulated nitrogen deposition. Biogeochemistry. (2005) 75:201–15. doi: 10.1007/s10533-004-7112-1
26. Allison SD, Gartner TB, Holland K, Weintraub M, Sinsabaugh RL. Soil enzymes: linking proteomics and ecological processes. In: Manual of Environmental Microbiology, 3rd ed. Washington, DC: ASM Press (2007). p. 704–11.
27. Edwards IP, Zak DR. Fungal community composition and function after long-term exposure of northern forests to elevated atmospheric CO2 and tropospheric O3. Glob Chang Biol. (2011) 17:2184–95. doi: 10.1111/j.1365-2486.2010.02376.x
28. Marklein AR, Houlton BZ. Nitrogen inputs accelerate phosphorus cycling rates across a wide variety of terrestrial ecosystems. New Phytol. (2012) 193:696–704. doi: 10.1111/j.1469-8137.2011.03967.x
29. Stark S, Männistö MK, Eskelinen A. Nutrient availability and pH jointly constrain microbial extracellular enzyme activities in nutrient-poor tundra soils. Plant Soil. (2014) 383:373–85. doi: 10.1007/s11104-014-2181-y
30. Galvez A, Sinicco T, Cayuela ML, Mingorance MD, Fornasier F, Mondini C. Short term effects of bioenergy by-products on soil C and N dynamics, nutrient availability and biochemical properties. Agric Ecosyst Environ. (2012) 160:3–14. doi: 10.1016/j.agee.2011.06.015
31. Schulten HR, Schnitzer M. The chemistry of soil organic nitrogen: a review. Biol Fertil Soils. (1997) 26:1–15. doi: 10.1007/s003740050335
32. Nannipieri P, Badalucco L. Biological processes. In: Bembi DK, Nieder R, editors. Handbook of Processes in the Soil–Plant System: Modelling Concepts and Applications. The Haworth Press: Binghamton NY (2003). pp. 57–76.
33. Turner BL, Romero TE. Stability of hydrolytic enzyme activity and microbial phosphorus during storage of tropical rain forest soils. Soil Biol Biochem. (2010) 42:459–65. doi: 10.1016/j.soilbio.2009.11.029
34. Jian S, Li J, Chen JI, Wang G, Mayes MA, Dzantor KE, et al. Soil extracellular enzyme activities, soil carbon and nitrogen storage under nitrogen fertilization: a meta-analysis. Soil Biol Biochem. (2016) 101:32–43. doi: 10.1016/j.soilbio.2016.07.003
35. Moorhead DL, Linkins AE. Elevated CO2 alters belowground exoenzyme activities in tussock tundra. Plant Soil. (1997) 189:321–9. doi: 10.1023/A:1004246720186
36. Schimel JP, Schimmel JP, Helfer S, Alexander IJ. Effects of starch additions on N turnover in Sitka spruce forest floor. Plant Soil. (1992) 139:139–43. doi: 10.1007/BF00012851
37. Harder W, Dijkhuizen L. Physiological responses to nutrient limitation. Annu Rev Microbiol. (1983) 37:1–23. doi: 10.1146/annurev.mi.37.100183.000245
38. Koch AL. The macroeconomics of bacterial growth. In: Fletcher M, Floodgate GD, editors. Bacteria in Their Natural Environments. London: Academic Press (1985). p. 1–42.
39. Reeves DW. The role of soil organic matter in maintaining soil quality in continuous cropping systems. Soil Tillage Res. (1997) 43:131–67. doi: 10.1016/S0167-1987(97)00038-X
40. Brejda JJ, Moorman TB. Karlen DL, Dao TH. Identification of regional soil quality factors and indicators. I. Central and Southern High Plains. Soil Sci. Soc. Am.J. (2000) 64:2115–24. doi: 10.2136/sssaj2000.6462115
41. Khan AR. Influence of tillage on soil aeration. J Agron Crop Sci. (1996) 177:253–9. doi: 10.1111/j.1439-037X.1996.tb00243.x
42. Latif MA, Mehuys GR, Mackenzie AF, Alli I, Faris MA. Effects of legumes on soil physical quality in a maize crop. Plant Soil. (1992) 140:15–23. doi: 10.1007/BF00012802
43. Dick RP. Soil enzyme activities as indicators of soil quality. Defining Soil Qual Sustain Environ. (1994) 35:107–24. doi: 10.2136/sssaspecpub35.c7
44. Jensen LS, McQueen DJ, Shepherd TG. Effects of soil compaction on N-mineralization and microbial-C and-NI Field measurements. Soil Tillage Res. (1996) 38:175–88. doi: 10.1016/S0167-1987(96)01033-1
45. Dick RP, Rasmussen PE, Kerle EA. Influence of long-term residue management on soil enzyme activities in relation to soil chemical properties of a wheat-fallow system. Biol Fertil Soils. (1988) 6:159–64. doi: 10.1007/BF00257667
46. Kandeler E, Eder G. Effect of cattle slurry in grassland on microbial biomass and on activities of various enzymes. Biol Fertil Soils. (1993) 16:249–54. doi: 10.1007/BF00369300
47. Perucci P, Scarponi L, Businelli M. Enzyme activities in a clay-loam soil amended with various crop residues. Plant Soil. (1984) 81:345–51. doi: 10.1007/BF02323049
48. Schipper LA, Sparling GP. Performance of soil condition indicators across taxonomic groups and land uses. Soil Sci Soc Am J. (2000) 64:300–11. doi: 10.2136/sssaj2000.641300x
49. Dick RP. A review: long-term effects of agricultural systems on soil biochemical and microbial parameters. Agric Ecosyst Environ. (1992) 40:25–36. doi: 10.1016/B978-0-444-89390-1.50005-6
50. Lynch JM, Panting LM. Cultivation and the soil biomass. Soil Biol Biochem. (1980) 12:29–33. doi: 10.1016/0038-0717(80)90099-1
51. Fraser FC, Corstanje R, Deeks LK, Harris JA, Pawlett M, Todman LC, et al. On the origin of carbon dioxide released from rewetted soils. Soil Biol Biochem. (2016) 101:1–5. doi: 10.1016/j.soilbio.2016.06.032
52. Nyiraneza J, Ziadi N, Zebarth BJ, Sharifi M, Burton DL, Drury CF, et al. Prediction of soil nitrogen supply in corn production using soil chemical and biological indices. Soil Sci Soc Am J. (2012) 76:925–35. doi: 10.2136/sssaj2011.0318
53. Nyiraneza J, Thompson B, Geng X, He J, Jiang Y, Fillmore S, et al. Changes in soil organic matter over 18 yr in Prince Edward Island, Canada. Can J Soil Sci. (2017) 97:745–56. doi: 10.1139/CJSS-2017-0033
54. Canadian Forest Inventory Committee. Developing the National Forest Inventory Design. Canadian Forest Inventory Committee, Pacific Forestry Centre, Canadian Forest Service, Victoria, BC, Canada. (1998).
55. Ziadi N, Angers DA, Gagnon B, Lalande R, Morel C, Rochette P, et al. Long-term tillage and synthetic fertilization affect soil functioning and crop yields in a corn–soybean rotation in eastern Canada. Can J Soil Sci. (2014) 94:365–76. doi: 10.4141/cjss2013-067
56. Lafond J, Angers DA, Pageau D, Lajeunesse J. Sustainable cereal and forage production in dairy-based cropping systems. Can J Plant Sci. (2017) 97:473–85. doi: 10.1139/CJPS-2016-0100
57. Hendershot WH, Lalande H, Duquette M. Ion exchange and exchangeable cations. In: Carter MR, Gregorich EG, editors. Soil Sampling and Methods of Analysis. Boca Raton, FL: CRC Press (2008). p. 197–204.
58. Skjemstad J, Baldock JA. Total and organic carbon. In: Carter MR, Gregorich EG, editors. Soil Sampling and Methods of Analysis. Boca Raton, FL: CRC Press (2008). p. 225–38.
59. Mehlich A. Mehlich 3 soil test extractant: a modification of Mehlich 2 extractant. Commun Soil Sci Plant Anal. (1984) 15:1409–16. doi: 10.1080/00103628409367568
60. Ziadi N, Tran TS. Mehlich-3 extractable elements. In: Carter MR, Gregorich EG, editors. Soil Sampling and Methods of Analysis. Boca Raton, FL: CRC Press (2008). p. 81–8.
61. Maynard D, Kalra Y, Crumbaugh J. Nitrate and exchangeable ammonium nitrogen. In: Carter, MR; Gregorich, EG, editors. Chapter 6. Soil Sampling and Methods of Analysis. CRC Press, Boca Raton, FL, Canadian Society of Soil Science (2008). pp. 71–80.
62. Sharifi M, Zebarth BJ, Burton DL, Grant CA, Porter GA, Cooper JM, et al. Evaluation of laboratory-based measures of soil mineral nitrogen and potentially mineralizable nitrogen as predictors of field-based indices of soil nitrogen supply in potato production. Plant Soil. (2007) 301:203–14. doi: 10.1007/s11104-007-9438-7
63. Haney RL, Haney EB. Simple and rapid laboratory method for rewetting dry soil for incubations. Commun Soil Sci Plant Anal. (2010) 41:1493–501. doi: 10.1080/00103624.2010.482171
64. Gallo M, Amonette R, Lauber C, Sinsabaugh RL, Zak DR. Microbial community structure and oxidative enzyme activity in nitrogen-amended north temperate forest soils. Microb Ecol. (2004) 48:218–29. doi: 10.1007/s00248-003-9001-x
65. Saiya-Cork KR, Sinsabaugh RL, Zak DR. The effects of long term nitrogen deposition on extracellular enzyme activity in an Acer saccharum forest soil. Soil Biol Biochem. (2002) 34:1309–15. doi: 10.1016/S0038-0717(02)00074-3
66. Stursova M, Crenshaw CL, Sinsabaugh RL. Microbial responses to long-term N deposition in a semiarid grassland. Microb Ecol. (2006) 51:90–8. doi: 10.1007/s00248-005-5156-y
67. Bell CW, Fricks BE, Rocca JD, Steinweg JM, McMahon SK, Wallenstein MD. High-throughput fluorometric measurement of potential soil extracellular enzyme activities. J Vis Exp. (2013) e50961. doi: 10.3791/50961
69. MacDougall JI, Veer C, Wilson F. Soils of Prince Edward Island: Prince Edward Island Soil Survey No. 83 54. Ministry of Supply and Services, Ottawa, ON (1988).
70. Breuer L, Huisman JA, Keller T, Frede HG. Impact of a conversion from cropland to grassland on C and N storage and related soil properties: analysis of a 60-year chronosequence. Geoderma. (2006) 133:6–18. doi: 10.1016/j.geoderma.2006.03.033
71. Franzluebbers AJ, Stuedemann JA, Schomberg HH, Wilkinson SR. Soil organic C and N pools under long-term pasture management in the Southern Piedmont USA. Soil Biol Biochem. (2000) 32:469–78. doi: 10.1016/S0038-0717(99)00176-5
72. Kopittke PM, Dalal RC, Finn D, Menzies NW. Global changes in soil stocks of carbon, nitrogen, phosphorus, and sulphur as influenced by long-term agricultural production. Glob Chang Biol. (2017) 23:2509–19. doi: 10.1111/gcb.13513
73. Murty D, Kirschbaum MUF, McMurtrie RE, McGilvray H. Does conversion of forest to agricultural land change soil carbon and nitrogen? A review of the literature. Glob Change Biol. (2002) 8:105–23. doi: 10.1046/j.1354-1013.2001.00459.x
74. Waldrop MP, Balser TC, Firestone MK. Linking microbial community composition to function in a tropical soil. Soil Biol Biochem. (2000) 32:1837–46. doi: 10.1016/S0038-0717(00)00157-7
75. Ramirez KS, Craine JM, Fierer N. Consistent effects of nitrogen amendments on soil microbial communities and processes across biomes. Glob Chang Biol. (2012) 18:1918–27. doi: 10.1111/j.1365-2486.2012.02639.x
76. Durso LM, Gilley JE, Marx DB, Woodbury BL. Effects of animal diet, manure application rate, and tillage on transport of microorganisms from manure-amended fields. Appl Environ Microbiol. (2011) 77:6715–7. doi: 10.1128/AEM.02995-10
77. Sotomayor-Ramírez D, Espinoza Y, Acosta-Martínez V. Land use effects on microbial biomass C, β-glucosidase and β-glucosaminidase activities, and availability, storage, and age of organic C in soil. Biol Fertil Soils. (2009) 45:487–97. doi: 10.1007/s00374-009-0359-x
78. Franzluebbers AJ, Haney RL, Honeycutt CW, Schomberg HH, Hons FM. Flush of carbon dioxide following rewetting of dried soil relates to active organic pools. Soil Sci Soc Am J. (2000) 64:613–23. doi: 10.2136/sssaj2000.642613x
79. Frarnzluebbers AJ, Arshad MA. Soil organic matter pools during early adoption of conservation tillage. Soil Sci Soc Am J. (1996) 60:1422–67. doi: 10.2136/sssaj1996.03615995006000050019x
80. Ma Q, Wu L, Wang J, Ma J, Zheng N, Hill PW, et al. Fertilizer regime changes the competitive uptake of organic nitrogen by wheat and soil microorganisms: an in-situ uptake test using 13C, 15N labelling, and 13C-PLFA analysis. Soil Biol Biochem. (2018) 125:319–27. doi: 10.1016/j.soilbio.2018.08.009
81. Ma Q, Wen Y, Wang D, Sun X, Hill PW, Macdonald A, et al. Farmyard manure applications stimulate soil carbon and nitrogen cycling by boosting microbial biomass rather than changing its community composition. Soil Biol Biochem. (2020) 144:107760. doi: 10.1016/j.soilbio.2020.107760
82. Allison SD, Vitousek PM. Responses of extracellular enzymes to simple and complex nutrient inputs. Soil Biol Biochem. (2005) 37:937–44. doi: 10.1016/j.soilbio.2004.09.014
83. Nannipieri P, Giagnoni L, Landi L, Renella G. Role of phosphatase enzymes in soil. In: Bünemann E, Oberson A, Frossard E, editors. Phosphorus in Action: Biological Processes in Soil Phosphorus Cycling. Berlin; Heidelberg: Springer Berlin Heidelberg (2011). p. 215–43.
84. Renella G, Landi L, Ascher J, Ceccherini MT, Pietramellara G, Nannipieri P. Phosphomonoesterase production and persistence and composition of bacterial communities during plant material decomposition in soils with different pH values. Soil Biol Biochem. (2006) 38:795–802. doi: 10.1016/j.soilbio.2005.07.005
85. Gupta V, Germida JJ. Distribution of microbial biomass and its activity in different soil aggregate size classes as affected by cultivation. Soil Biol Biochem. (1988) 20:777–86. doi: 10.1016/0038-0717(88)90082-X
86. Trasar-Cepeda C, Leirós MC, Gil-Sotres F. Hydrolytic enzyme activities in agricultural and forest soils. Some implications for their use as indicators of soil quality. Soil Biol Biochem. (2008) 40:2146–55. doi: 10.1016/j.soilbio.2008.03.015
87. Tadano T, Sakai H. Secretion of acid phosphatase by the roots of several crop species under phosphorus-deficient conditions. Soil Sci Plant Nutr. (1991) 37:129–40. doi: 10.1080/00380768.1991.10415018
88. Hartman WH, Richardson CJ. Differential nutrient limitation of soil microbial biomass and metabolic quotients (q CO2): is there a biological stoichiometry of soil microbes?. PLoS ONE. (2013) 8:e57127. doi: 10.1371/journal.pone.0057127
89. Finzi AC, Austin AT, Cleland EE, Frey SD, Houlton BZ, Wallenstein MD. Responses and feedbacks of coupled biogeochemical cycles to climate change: examples from terrestrial ecosystems. Front Ecol Environ. (2011) 9:61–7. doi: 10.1890/100001
90. Spohn M, Kuzyakov Y. Phosphorus mineralization can be driven by microbial need for carbon. Soil Biol Biochem. (2013) 61:69–75. doi: 10.1016/j.soilbio.2013.02.013
Keywords: crop rotation, cultivation intensity, liquid dairy manure, mineral N fertilizer, tillage regimes
Citation: Uwituze Y, Nyiraneza J, Fraser TD, Dessureaut-Rompré J, Ziadi N and Lafond J (2022) Carbon, Nitrogen, Phosphorus, and Extracellular Soil Enzyme Responses to Different Land Use. Front. Soil Sci. 2:814554. doi: 10.3389/fsoil.2022.814554
Received: 13 November 2021; Accepted: 11 April 2022;
Published: 29 June 2022.
Edited by:
Stephanie Ann Yarwood, University of Maryland, College Park, United StatesReviewed by:
Qingxu Ma, Bangor University, United KingdomAlexander Tischer, Friedrich Schiller University Jena, Germany
Copyright © 2022 Jacynthe Dessureault-Rompré, and Her Majesty the Queen in Right of Canada, as represented by the Minister of Agriculture and Agri-Food for the contribution of Yvonne Uwituze, Judith Nyiraneza, Tandra D. Fraser, Noura Ziadi and Jean Lafond. This is an open-access article distributed under the terms of the Creative Commons Attribution License (CC BY). The use, distribution or reproduction in other forums is permitted, provided the original author(s) and the copyright owner(s) are credited and that the original publication in this journal is cited, in accordance with accepted academic practice. No use, distribution or reproduction is permitted which does not comply with these terms.
*Correspondence: Judith Nyiraneza, anVkaXRoLm55aXJhbmV6YUBhZ3IuZ2MuY2E=