- 1College of Agriculture, Henan University of Science and Technology, Luoyang, China
- 2College of Natural Resources and Environment, Northwest A&F University, Yangling, China
- 3Key Laboratory of Plant Nutrition and the Agri-environment in Northwest China, Ministry of Agriculture, Yangling, China
The application of biochar in conjunction with fertilizer in agricultural production is one of the most promising types of management to improve soil quality. However, the effects on the soil microbial community and methane (CH4) emissions from the interactive mechanisms of biochar combined with fertilizer are unclear. In this study, soil column trial was conducted to monitor the surface water nitrogen, dissolved organic carbon (DOC) and CH4 emission dynamics during the process of leaching. Additionally, bacterial and archaeal communities of the soil (0-10 cm) amended with biochar derived from different pyrolysis temperatures (300°C, 500°C, and 700°C) were also analyzed. High-throughput sequencing revealed that the soil archaeal and bacterial community diversities increased under the biochar amendments. The CH4 emission flux of all the treatments in the whole leaching period ranged from 0.0001 to 2.04 μg m-2 h-1, and the DOC ranged from 1.86 to 24.4 mg L-1. Our results showed that biochar amendments significantly increase the soil pH, total nitrogen (TN), and DOC contents, while inhibiting the loss of N during leaching. In addition, biochar addition increased the paddy soil CH4 emissions, which ascribed to the increasing ratio of the abundances of methanogens to methanotrophs. Consequently, the higher CH4 emissions were probably caused by the stimulation of methanogenic archaea under the biochar amendments. Thus, the results obtained in this study can be applied to guide the application of biochar on greenhouse gas emissions in paddy soil.
Introduction
Greenhouse gas (GHG) emissions from paddy fields are one of the major factors that stimulate global warming (1). Methane (CH4) emissions are the second largest factor that significantly contributes to global warming, which has s 45 times that of carbon dioxide (CO2) on a mass basis over 100-year time horizon. (2, 3). Paddy soil is deemed to be a major source of CH4 with the annual paddy CH4 emission ranging from 25 to 54 Tg, which is 4-9% of the total CH4 emissions of 598 Tg (4). Unfortunately, the CH4 contribution ranges between 5.3-19% of the total emissions in China (5). Therefore, effective strategies should be utilized to decrease the CH4 emissions from the paddy soils to relieve global warming.
Methanogenic archaea are known to play a vital role in regulating the biological processes of CH4 production in flooded paddy soils. The organic matter in paddy soils is anaerobically degraded by various bacteria to methanogenic materials (e.g., acetate, H2, CO2 and methyl compounds) during the methanogenesis process, which results in the production of CH4 by the methanogenic archaea (6). However, methanotrophic Proteobacteria consume a greater portion of the CH4 as a carbon source to regulate its net production. A previous study demonstrated that the methanotrophic Proteobacteria oxidized more than 90% of the total CH4 produced under an anaerobic soil environment (7). Therefore, to decrease the CH4 emissions in anoxic paddy soil, the ratio of methanogens to methanotrophs should be reduced rather than focusing on single types of bacteria or archaea (8).
In recent years, crop straw and its derived application has increased due to its renewability and potential value in the agricultural environment. Biochar, a carbon-rich solid, produced through the pyrolysis of the plant biomass under relative high temperature and oxygen-limited conditions, ubiquitously exists in arable soils (9). Due to its specific characteristics, such as a high pH and surface area, it has been used as a beneficial soil amendment to improve the soil fertility and grain yields by enhancing the retention of soil nutrients (10, 11). In addition, biochar amendments can affect the individual soil organic matter (SOM) through releasing carbon-containing compound, such as the dissolved organic carbon (DOC) content, microbial biomass carbon (MBC), compared with unamended soil (12). Liang etal. (13) reported that the soil MBC was 43-125% higher in soil amended with biochar than in soil that lacks biochar during a 532-day incubation experiment. In addition, previous studies revealed that the addition of biochar can alter the use efficiency of C sources and the patterns of microorganisms by regulating soil physicochemical properties, as well as those attributed to the characteristics of biochar (e.g., aeration and sorption) (14). However, the results of biochar mineralization and its influence on SOM content remained controversial viewpoints. For example, the added sugarcane C does not increase the mineralization of aged black C in Anthrosols in the Central Amazon, but the well effect exists in red soil southern China (13).
In addition, with its superior properties, biochar could serve as an appealing habitat to protect soil microorganisms from predators (15), as well as indirectly affecting CH4 emissions (16). However, the effect of the application of biochar amendments on CH4 emissions has varied in previous studies. Although several researchers indicated no impacts (17), others showed that biochar amendments to the soil could increase the CH4 emissions (9, 18) or decrease the CH4 flux (8, 19). Thus, the lack of clarity on the CH4 emissions from paddy soils amended with biochar should be addressed to better understand its underlying mechanism.
In this study, we conducted a soil column experiment supplied with biochars produced at different pyrolysis temperatures (300°C, 500°C, and 700°C) of 0-10 cm soils amended with wheat straw-derived biochar and treated with chemical fertilizer (Urea, Calcium superphosphate, Potassium sulfate) to simulate and explore the paddy soil nutrient leaching factors and CH4 emission dynamics, as well as the variation in the soil microbial community. We hypothesis biochar amendment can regulate soil microbial structures and community, and then promote or restrain CH4 emission flux. The objectives of this study were to (1) evaluate whether wheat straw-derived biochar amendment would decrease CH4 emissions, (2) understand the influence of pyrolysis temperature (300°C, 500°C, and 700°C) in the production of biochar on CH4 emissions and soil and surface water qualities, and (3) assess the difference of the soil microbial community among the various treatments.
Materials and Methods
Soil Sampling and Biochar Preparation
Soil was collected on September 25, 2017 from a test site located in Xinji County, Hanzhong City (33°0′16’’N, 108°48′44’’E) after the rice harvest. Wheat straw was used to produce the biochar. After air-drying and crushing, the crushed wheat straw was placed in crucibles sealed with lids to prevent the oxygen from entering and pyrolyzed in a muffle furnace heating at a rate of 10°C/min and holding at 300, 500, and 700°C for 2 h, respectively. The biochars produced with low (300°C), medium (500°C), and high (700°C) temperatures were designated BC300, BC500, and BC700, respectively. The process ended when no external smoke was released from the reactor at the target temperature. The biochar obtained was ground using a mortar and pestle before passing through a 1 mm mesh for further use. The biochar characteristics were examined. The concentrations of elemental C and N were determined using an elemental analyzer (Vario EL, Elementar, Germany). The biochar pH was measured using a pH probe with a 1:5 (w/w) suspension of biochar in deionized water. The basic properties of the soil test were as follows: pH: 7.38, soil organic carbon: 32.22 g kg-1, available potassium: 134.4 mg kg-1, available phosphorus: 20.12 mg kg-1, cation exchange capacity: 18.62 cmol kg-1, and total nitrogen: 1.21 g kg-1. The characteristics of different pyrolysis temperature biochars are presented in Table 1.

Table 1 Basic characteristics of biochar produced through pyrolysis of wheat straw at 300°C (BC300), 500°C (BC500), and 700°C (BC700).
Soil Column Leaching Experimental Design
To simulate wheat straw biochar amendment in flooded paddy soil, a series of soil column experiments were conducted in the Yangling District, Shaanxi Province, China (34°15′N, 108°34′E). The soil used in this column study was collected from four different soil layers (0-10, 10-20, 20-30, and 30-40 cm) of the local farmland dominated by a system of wheat and rice. Measurements of the basic physicochemical properties of the soil tested were conducted using standard analytical methods, and the details are described in Table 2.

Table 2 Basical characteristics of the profile (0-40cm) soils filled in column in the present study.
Approximately 1 kg of air-dried soil was placed in a soil column at a height of every 10 cm (Figure 1), and the 0-10 cm layer was mixed with 3% (w/w) biochar obtained at different temperature (referred as to soil to biochar) at an identical fertilizer level (i.e., CK: control treatment without biochar and fertilizer; F: only fertilizer applied; FBC300: 300°C biochar combined with fertilizer; FBC500: 500°C biochar combined with fertilizer, and FBC700: 700°C biochar combined with fertilizer) (21). In field rice season, the amounts of fertilizer were 180 kg N and 90 kg P2O5, respectively. Hence, the fertilizer nitrogen and phosphorus sources were urea (0.32 g/column) and calcium superphosphate (3.92 g/column), and mixed with 0-10 cm soil before leaching. A total of 1400 mL deionized water was added to saturate the dried soil in the column. On days 2, 3, 6, 10, 20, 35 and 55, the soil leachates were sampled from the four layers, and changes in the DOC concentrations were quantified. Deionized water was added to keep the surface water volume at 300 mL during the whole experiment.
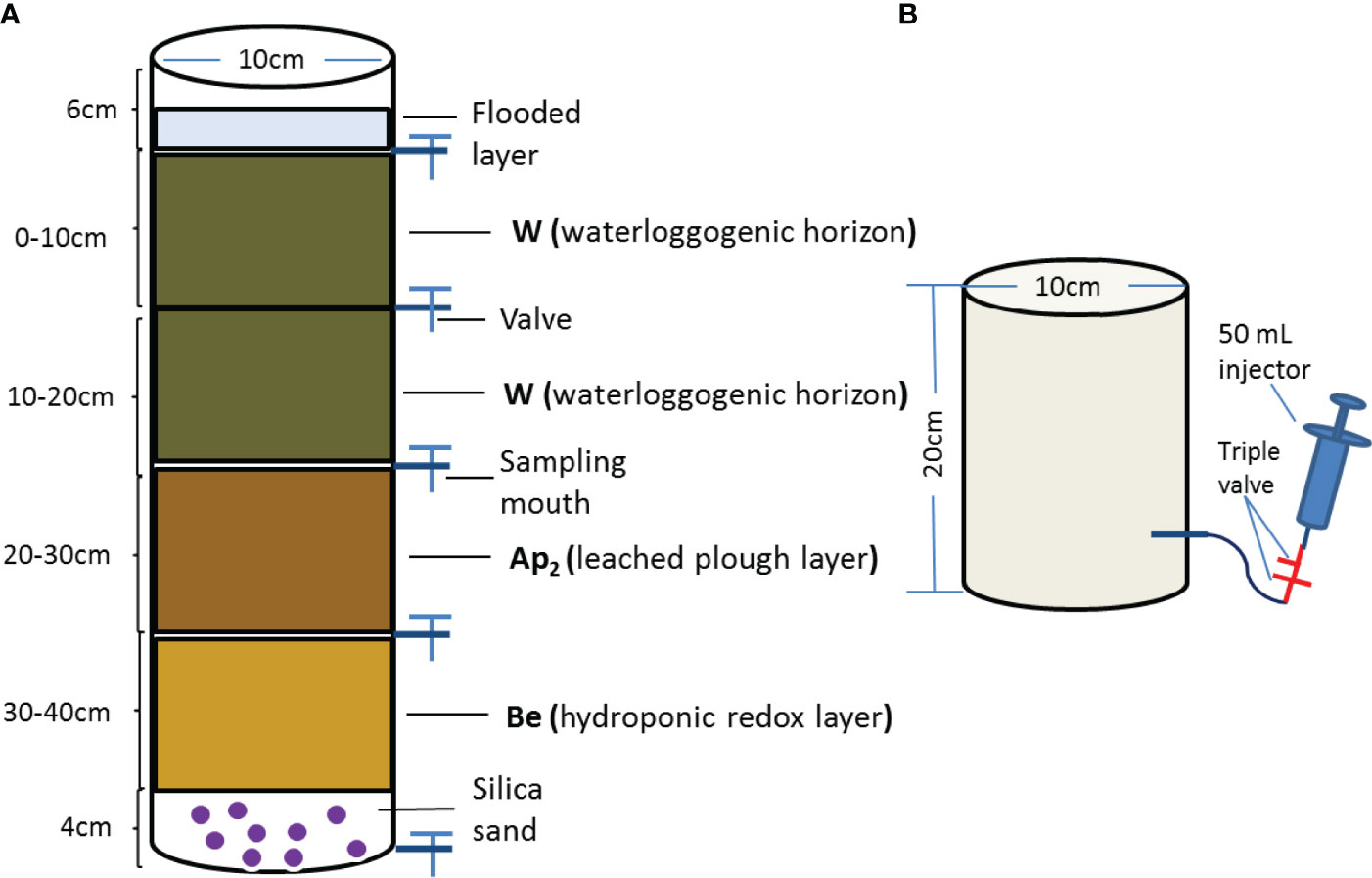
Figure 1 (A) Diagram of soil column experimental design (Column height: 50cm, Inner diameter: 10cm). A 3% w/w different pyrolysis wheat straw biochar was mixed with 0-10cm soil layer (referred as to soil to biochar) along with an identical fertilizer level (i.e. CK, Control treatment without biochar and fertilizer; F, Only fertilizer added; FBC300, 300°C biochar combined with fertilizer; FBC500, 500°C biochar combined with fertilizer; FBC700, 700°C biochar combined with fertilizer). Silica sands were placed at the bottom to keep column aeration, and unwoven was twined at inward sampling mouth to against blocking (20). (B) Sketch drawing of static closed chamber sampling gas from soil column.
Measurement of the CH4 Flux, Soil and Surface Water
The GHG sample was collected using a column-shaped static chamber (height: 20 cm, inner diameter: 10 cm) with another frequency (2, 3, 6, 15, 30 and 55 days) as described above. Before gas sampling, the column was capped with the chamber, and the seam crossing was glued with tape to prevent air leakage. Approximately 50 mL air was repeatedly exhausted using a syringe to completely mix the collected air. This was the zero time sample, and the other two samples were sampled in the same manner every 30 min. The CH4 concentration was determined using high performance gas chromatography (GC; Agilent 7890, Agilent technologies, Santa Clara, CA, USA) equipped with a flame ionization detector (FID) at a temperature of 250 k. The CH4 fluxes were calculated from the CH4 accumulation between time zero and after incubation for 30 min. The CH4 gas standards were obtained from Beijing ZG Special Gases Science & Technology Co., Ltd.
After the leaching test, 0-10 cm mixed soil was sampled from each column. Soil water-extractable DOC and MBC were analyzed using a total organic carbon (TOC) analyzer (Shimadzu Inc., TOC-VCHP, Japan). The soil ammonium (), nitrate ( ) nitrogen, total nitrogen (TN) and microbial biomass nitrogen (MBN) were determined using an AA3 continuous flow analyzer (SEAl Inc., AA3, German). The DOC, -N, and N concentrations in the surface water were also detected using a TOC analyzer and an AA3 continuous flow analyzer, respectively.
Soil DNA Extraction and PCR Amplification
The soil DNA was extracted using a Power Soil DNA Isolation Kit (MoBio Laboratories, Carlsbad, CA) following the manufacturer’s instructions. The purity and quality of the genomic DNA were examined on 0.8% agarose gels.
The V3-V4 hypervariable region of the bacterial 16S rRNA genes was amplified using the primers [338F (ACTCCTACGGGAGGCAGCAG) and 806R (GGACTACHVGGGTWTCTAAT)] (22). The V3-V4 variable region of the archaeal 16s rRNA gene was amplified utilizing the primers [344F (ACGGGGYGCAGCAGGCGCGA) and 806R (GGACTACVSGGGTATCTAAT)]. For each soil sample, a 10-digit barcode sequence was added to the 5’ end of the forward and reverse primers (provided by Allwegene Company, Beijing). The PCR was conducted on a Mastercycler Gradient (Eppendorf, Germany) using 25 μL reaction volumes, containing 12.5 μL 2× Taq PCR Master Mix, 3 μL BSA (2 ng/μL), 2 Primers (5 µM), 2 μL template DNA, and 5.5 μL ddH2O. The cycling parameters were as follows: 95°C for 5 min, followed by 32 cycles of 95°C for 45 sec, 55°C for 50 sec and 72°C for 45 sec with a final extension at 72°C for 10 min. Three PCR products per sample were pooled to mitigate any reaction-level PCR biases. The PCR products were purified using a QIA Quick Gel Extraction Kit (QIAGEN, Germany), quantified using Real Time PCR, and sequenced at the Allwegene Company, Beijing.
High-Throughput Sequencing and Bioinformatic Analyses
Deep sequencing was performed on a MiSeq platform at the Allwegene Company (Beijing). After the run, image analysis, base calling and error estimation were performed using Illumina Analysis Pipeline Version 2.6. The raw data were first screened, and sequences were removed from consideration if they were shorter than 200 bp, had a low-quality score (≤ 20), contained ambiguous bases or did not exactly match the primer sequences and barcode tags. Qualified reads were separated using the sample-specific barcode sequences and trimmed using Illumina Analysis Pipeline Version 2.6. The dataset was analyzed using QIIME. The sequences were clustered into operational taxonomic units (OTUs) at a similarity level of 97% (23), to generate rarefaction curves and calculate the richness and diversity indices. The Ribosomal Database Project (RDP) Classifier tool was used to classify all the sequences into different taxonomic groups (24, 25).
Statistical Analysis
The raw data were processed in Microsoft Excel (Microsoft Excel 2010, O’Reilly, Microsoft USA). The data were tested for the homogeneity of the group variances using the Pearson test. An ANOVA and a Duncan’s test (both set to P < 0.05) were used to evaluate the difference among the treatments using SPSS 20.0 (SPSS Inc., Chicago, USA). All the figures in this study were drawn using Origin 9.0 (Origin 2015, USA). Statistical tests were evaluated at the 95% confidence interval. Therefore, differences were considered significant at p < 0.05.
Results
Basic Characteristics of the Paddy Soil and Biochar
Different pyrolysis temperature resulted in a greater variation in the properties of biochar (Table 1). The pH of the biochar increased with increasing pyrolysis temperatures (10.13, 10.47, and 10.86, respectively), which significantly exceeded the pH of the highest soil pH (7.38). However, the nutrient contents of the biochar decreased at BC700. The EC and TC contents and the C:N ratio of the BC500 were 422 μs cm-1, 62.63% and 65.05%, respectively, which were higher than those of the BC300 (315 μs cm-1, 59.46% and 55.41%, respectively) and BC700 (400 μs cm-1, 60.84% and 56.43%, respectively). The total nitrogen (TN) of the biochar derived from different temperatures did not differ significantly. However, the CEC of the BC300 (74.67 cmol kg-1) was significantly higher than those in the BC500 (54.37 cmol kg-1) and BC700 (35.66 cmol kg-1) (p<0.05).
Variations in the CH4 Emissions, Surface Water and Soil Properties
Dynamic changes of the CH4 emissions among the different treatments (CK: 0-10 cm soil without biochar and fertilizer; F: fertilizer; FBC300: with fertilizer and 300°C biochar; FBC500: with fertilizer and 500°C biochar, and FBC700: with fertilizer and 700°C biochar) are shown in Figure 2.
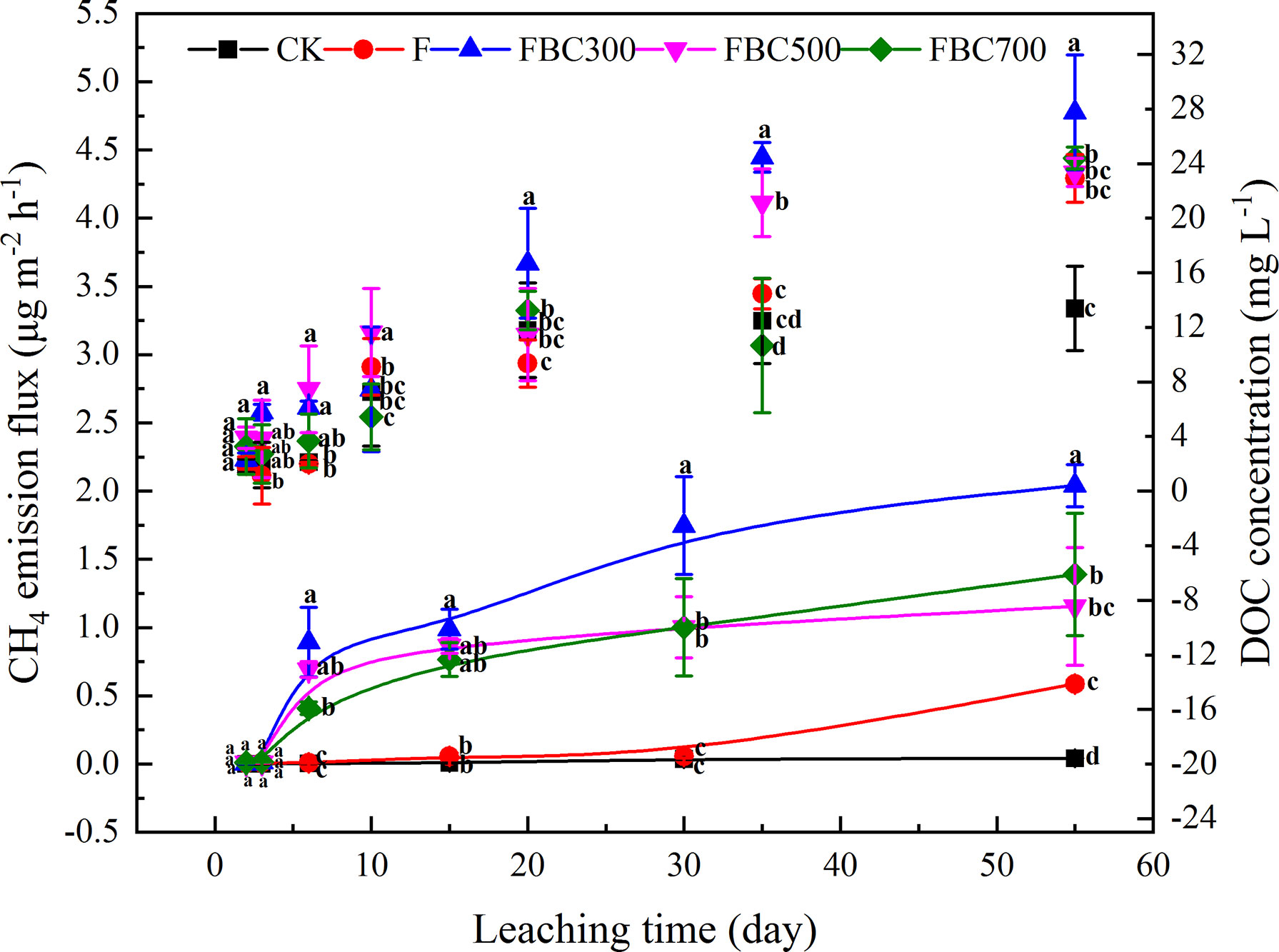
Figure 2 Temporal changes of methane (CH4) emission flux and surface water dissolved organic carbon (DOC) during 55 days column leaching of paddy soil incorporated with or without biochar amendments and/or fertilizer (i.e. CK: Control treatment without biochar and fertilizer; F: Only fertilizer added; FBC300: 300°C biochar combined with fertilizer; FBC500: 500°C biochar combined with fertilizer; FBC700: 700°C biochar combined with fertilizer). Scatter diagram represented DOC dynamic changes. Curve chart represented CH4 emission flux. Letters represent the different statistical classes among the treatments at p<0.05.
In this study, the CH4 emissions were higher in the biochar amendment than those of the control, especially for the biochar derived at low temperatures (Figure 2). Overall, the production of the CH4 increased until the end of the experiment. CH4 emission fluxes differed significantly after day 6 and increased up to 2.04, 1.55, and 1.39 μg m-2 h-1 at the end of leaching for FBC300, FBC500, and FBC700, respectively. Compared to the BC amendments, the CH4 production in the control treatment remained stable, but the F treatment presented a peak of CH4 emission in the end. In addition, the surface water DOC changes were also determined to evaluate the characteristics of the CH4 emissions. The surface water DOC concentration increased in an almost linear manner during the whole leaching stage, and FBC300 displayed a higher DOC content than the control and other treatments. The variations of the surface water, -N and N concentrations were also determined during the process of leaching. As shown in Figure 3, the biochar amendments effectively inhibited the leaching loss of -N, while increasing the leaching loss of N, especially at 10 days of leaching.
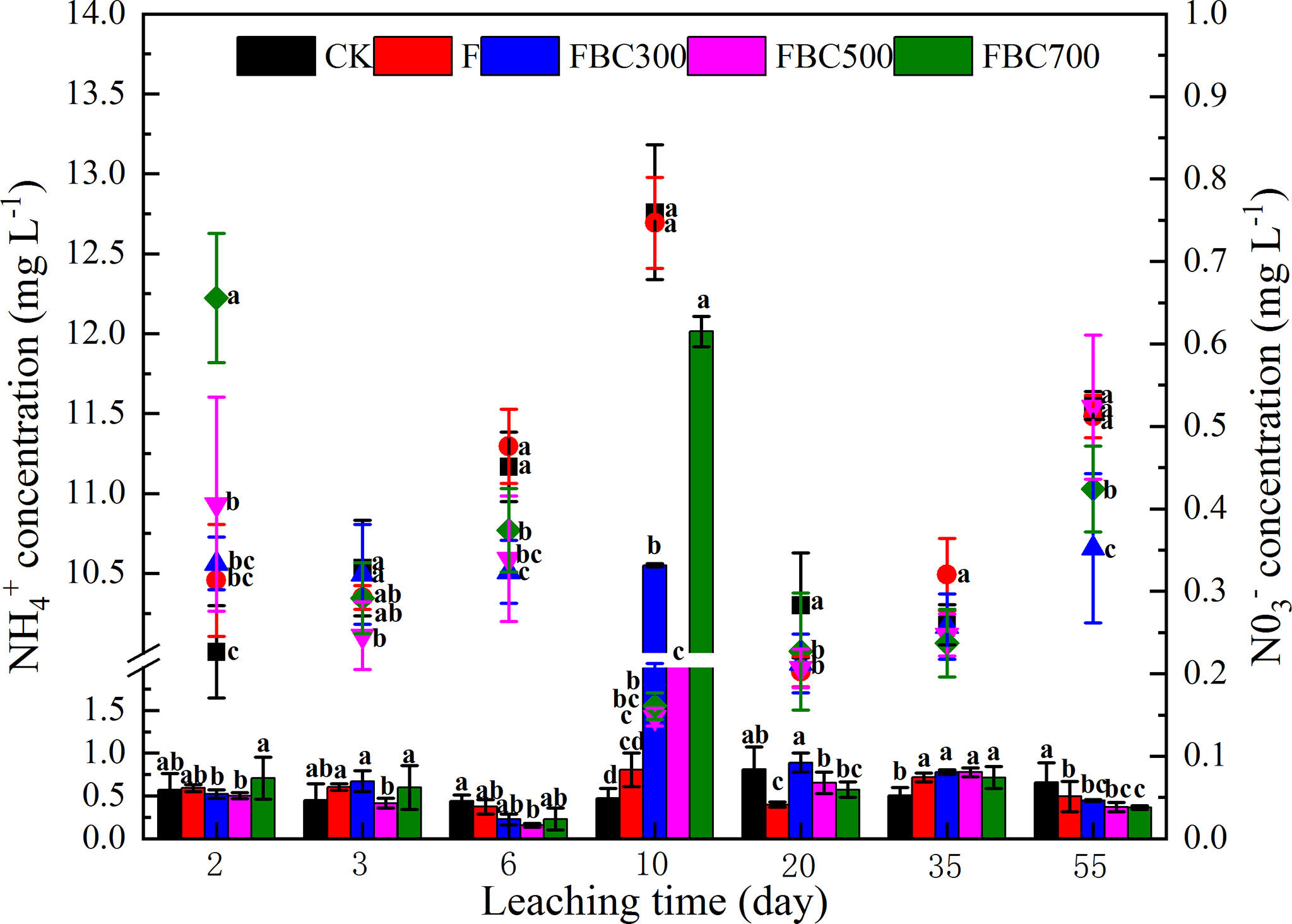
Figure 3 Dynamic changes of surface water ammonium nitrogen () and nitrate nitrogen () during 55 days column leaching of paddy soil incorporated with or without biochar amendments and/or fertilizer (i.e. CK: Control treatment without biochar and fertilizer; F: Only fertilizer added; FBC300: 300°C biochar combined with fertilizer; FBC500: 500°C biochar combined with fertilizer; FBC700: 700°C biochar combined with fertilizer). Point and bar represent mean values. Standard deviation and error bars are also presented. Scatter diagram represented N dynamic changes. Column chart represented -N dynamic changes. Letters represent the different statistical classes among the treatments at p<0.05.
Compared to the CK and F, the biochar amendments significantly increased the soil DOC contents, especially using biochar produced at a lower temperature (p<0.05). In contrast, the soil MBC contents showed an opposite tendency with increasing temperature (FBC300<FBC500<FBC700), and the MBC concentration of FBC700 was significantly higher than that of the other treatments (p<0.05). In addition, the biochar amendments increased the soil pH, TN, and DON contents, while decreasing the ratio of DOC/DON due to high DOC concentrations (Table 3).

Table 3 Soil pH, total N, dissolved inorganic N, dissolved organic N, microbial biomass N, dissolved organic C, microbial biomass C, DOC/DON ratio of topsoil (0-10cm) collected after 55 days leaching .
Changes of the Soil Archaeal and Bacterial Community Composition
To understand the variations of the microbial community and structure in the soil incorporated with BC at the end of the leaching test, a high-throughput sequencing technique was employed to identify the changes of the soil archaea and bacteria. The Shannon index analysis is shown in Figure 4, which demonstrated that the biochar amendments increased the soil archaeal and bacterial community diversities.
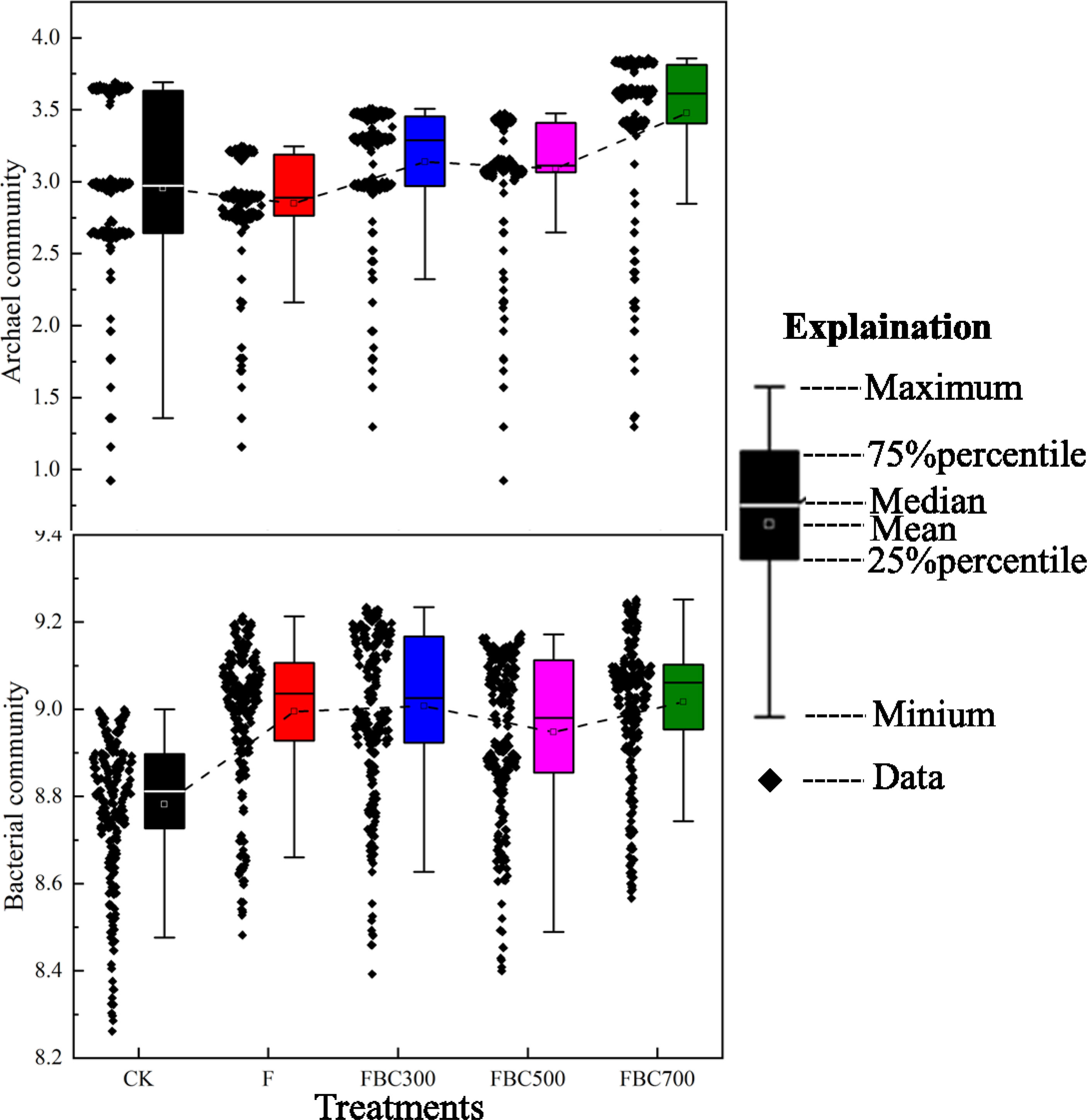
Figure 4 Variations of soil archaeal (A) and bacterial (B) community diversities by Shannon index analysis among treatments incorporated with or without biochar amendments and/or fertilizer (i.e. CK: Control treatment without biochar and fertilizer; F: Only fertilizer added; FBC300: 300°C biochar combined with fertilizer; FBC500: 500°C biochar combined with fertilizer; FBC700: 700°C biochar combined with fertilizer).
The major compositions of the archaeal community in the paddy soil (collected after 55 day) were SCG (Soil Crenarchaeotic Group), Methanomicrobia, Methanobacteria, and GC3 (Group C3) at the class level with a dominance of Methanomicrobia. Compared to the CK (46%) and F (44%) treatments, the distribution of Methanomicrobia in the FBC300, FBC500, and FBC700 amendments increased to 56%, 49% and 51%, respectively. The distribution of the SCG deceased to 29%, 34% and 33%, respectively, in the biochar addition treatments. Methanobacteria were closely associated with the production of CH4, accounting for 6%, 9% and 7% in soil with biochar (300°C, 500°C, and 700°C, respectively), suggesting that biochar could influence the CH4 production via regulating the activity and abundance of the soil microorganisms.
The dominant composition of the bacterial community was alpha proteobacteria, Anaerolineae and Deltaproteobacteria at the class level. In general, biochar amendments do not induce a distinct difference in the soil bacterial community. Compared with the CK (18%), alpha proteobacteria in the F, FBC300 and FBC700 treatments increased to 19%, 19% and 20%, respectively, while it decreased by 1% in the FBC500 treatment. The application of biochar increased the abundance of Anaerolineae compared to the CK treatment. Similarly, delta proteobacteria, an important type of methanotrophic proteobacteria, displayed lower growth rates of 2% and 1% in FBC300 and FBC700, respectively, but decreased by 1% in FBC300 in contrast to the CK (12%) and F (12%).
Relationship of the Bacterial and Archaeal Communities to Environmental Factors
The redundancy analysis (RDA) of the archaeal (A) and bacterial (B) community composition at the class level was also used to explain the ordination of the environmental factors (Figure 6). In the ordination plot, the soil -N, N, MBC, MBN, pH and DOC showed the highest correlation coefficient (longest arrow) with the soil archaeal community composition (Figure 6A). However, the soil properties soil -N and TN were the primary driving factors that regulated the soil bacterial community composition (Figure 6B). For the soil archaeal community, the first two axes explained 3.4% and 76.2% of the total variation, while the first two axes accounted for 9.0% and 39.6% of the total variation in the soil bacterial community composition.
Discussion
Biochar Characteristics and its Effects on Soil Properties
Biochar preparation conditions, including temperature, pyrolysis time, and oxygen, determine its physicochemical properties and potential application function. Generally, a pyrolysis reaction at high temperature and low or oxygen-poor conditions produces biochar that contains a high carbon content comprised of aromatic and stable structures that is resistant to degradation by microorganisms (26). In this study, the basic properties of the soil and biochar were observed. The results of the BC pH changes were consistent with previous studies (27, 28). In addition, carbonates are regarded to be the major alkaline contributor of biochar generated from crop straws at a high temperature (29). The reason for the increase in the pH can also be explained by the release of volatile matter comprised of acid functional groups with the increasing temperature (30). The EC in BC500 was significantly higher than that in BC300 and BC700, primarily resulting from the abundant original nutrient contents (31). As reported by Zhao et al., the CEC was expected to decrease due to the loss of the acidic functional groups by the action of the pyrolysis temperature (32). With the increase in the pyrolysis temperature, the pore volume and specific surface area of the biochar generally increased, primarily resulting from the generation of micropore carbon (33).
Moreover, Biochar amendments could be expected to reserve soil nutrients and prevent N, P loss, and therefore, to supply more nutrient elements for rice growth (18, 34). The higher soil DOC and MBC content after biochar combined with fertilization compared with the CK and F treatments proved the role of biochar in contributing to a C pool in paddy soil (12). Kuzyakov revealed that only 6% of the original biochar was mineralized to CO2 after 8.5 years incubation; therefore, increasing the C stock (35). Thus, wheat straw-derived biochar was accepted as an underlying nutrient source in the rice cultivation systems.
Biochar Amendments Increase CH4 Emissions Flux Attributed to the Changes of Soil Bacterial and Archaeal Community Composition
Most studies have reported that biochar amendments decreased the CH4 emissions, which result from the increase in the soil pH (9, 36), the enhancement of the soil particles on CH4 (37), or the stimulation of methanotrophic activity (38, 39). However, the increase of CH4 emissions under biochar amendments has also been reported, and the production of increased CH4 emissions has been attributed to the reduction of the redox potential (40, 41), or the foundation of a favorable environment for CH4 emission-related microbial activity under biochar incorporation (42, 43). Similarly, in this study, biochar combined with fertilization significantly increased the CH4 emissions from the paddy soils compared with those without the biochar treatments. How to efficiently and effectively utilize biochar to conserve soil productivity and fertility is a problem to be urgently solved. In this study, the highest CH4 emission was observed for wheat straw biochar produced at low temperatures (FBC300 Figure 1), which outclasses that of the CK and F treatments. These results are consistent with the previous report of a 37% increase with biochar incorporation (41). In addition, Zhang et al. (18) reported a similar result that biochar amendments increased CH4 emissions but decreased N2O emissions from paddy soils (18). However, in contrast, Liu et al. (19) reported that approximately 91.2% of the decrease in the CH4 emissions occurred under the biochar amendments (19). As is well known, the factors influencing paddy soil CH4 emissions are attributed to the physical and chemical properties of the treated soil and biochar and are even determined by the biochar production procedure, including biomass type and pyrolysis temperature or time (44).
The changes of the soil bacterial and archaeal community composition were analyzed at the class level (Figure 5). Previous studies showed that the soil microbial activity and microbial biomass could be enhanced under biochar amendments (45, 46). In this study, three temperatures of biochar amendments significantly increased the soil DOC contents, which can provide more C sources for methanogenic growth (Table 2). Methanomicrobia and Methanobacteria were the primary compositions of the methanogenic archaeal community, altering the basal metabolic activities in the paddy soils (47). Our results indicated that the dominant methanogenic archaea related at the class level were stimulated by biochar amendments, leading to the logical conclusion (Figure 5A). In the ordination plot, the soil -N, N, MBC, MBN, pH and DOC showed the highest correlation coefficient (longest arrow) with the soil archaeal community composition (Figure 6A). However, of the soil properties tested, soil -N and TN were the primary driving factors in regulating the soil bacterial community composition (Figure 6B). In addition, the dominant methanotrophic proteobacteria were also examined in this study. Consequence, the abundances of alpha proteobacteria, Anaerolineae and Deltaproteobacteria decreased slightly under the biochar amendments, which lead to the increase in the ratio of methanogens to methanotrophs and increase paddy soil CH4 emissions (1, 3). It is hypothesized that CH4 is retained by the porous structure of biochar (45) but is not a major regulatory mechanism for CH4 emissions.
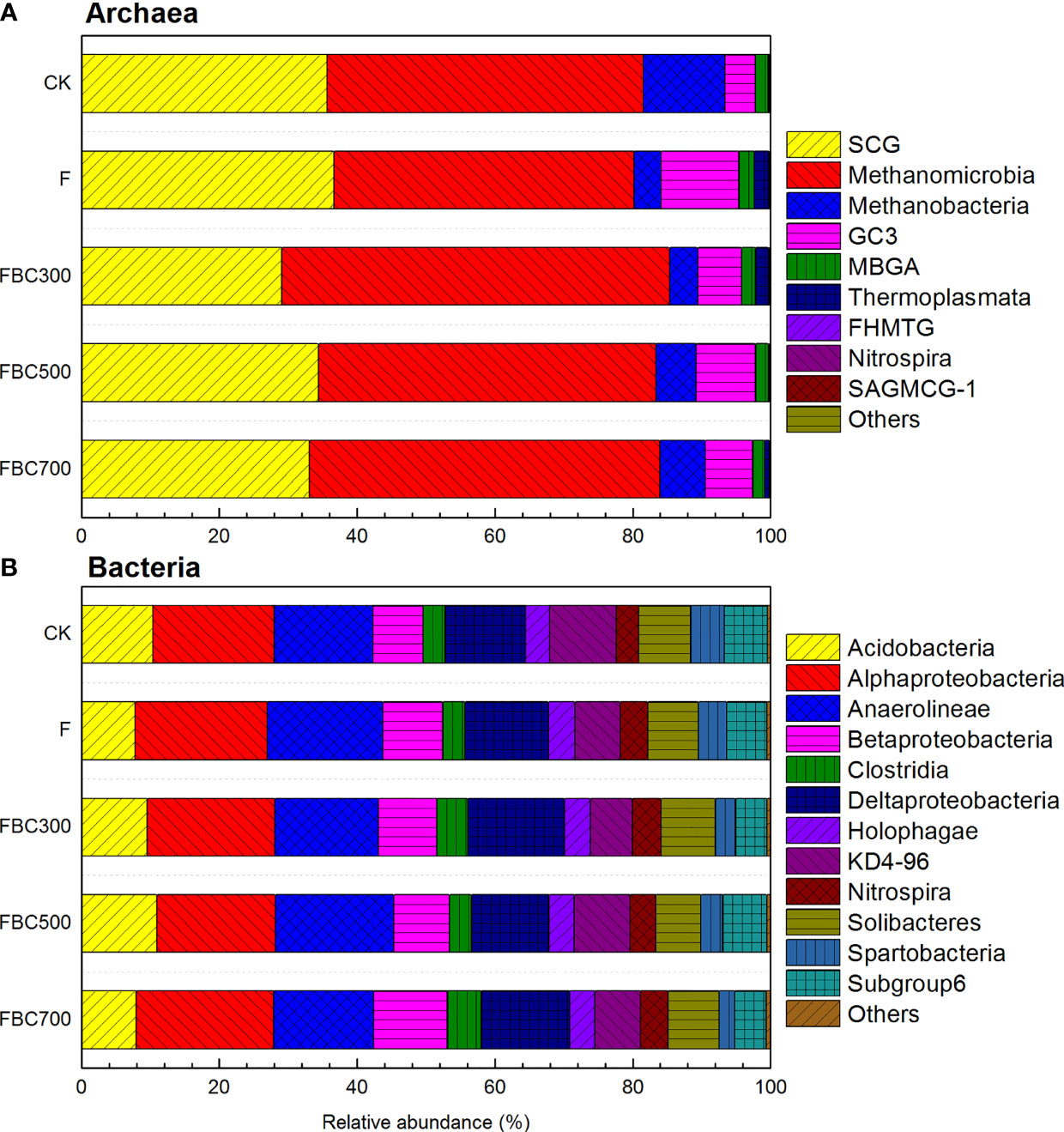
Figure 5 Distributions of archaeal (A) and bacterial (B) community composition at class level in soil samples collected after 55 days column leaching of paddy topsoil (0-10cm) without fertilizer and biochar (CK), with fertilizer only (F), with fertilizer and biochar produced by wheat straw at 300°C,500°C, and 700°C (FBC300, FBC500 and FBC700). SCG represents Soil Crenarchaeotic Group; GC3 represents Group C3; MBGA represents Marine Benthic Group A; FHMTG represents FHMall terrestrial group; SAGMA-1 represents South African Gold Mine Group-1. Others of archaeal and bacterial communities include the average of low abundance but excluding the unidentified sequences.
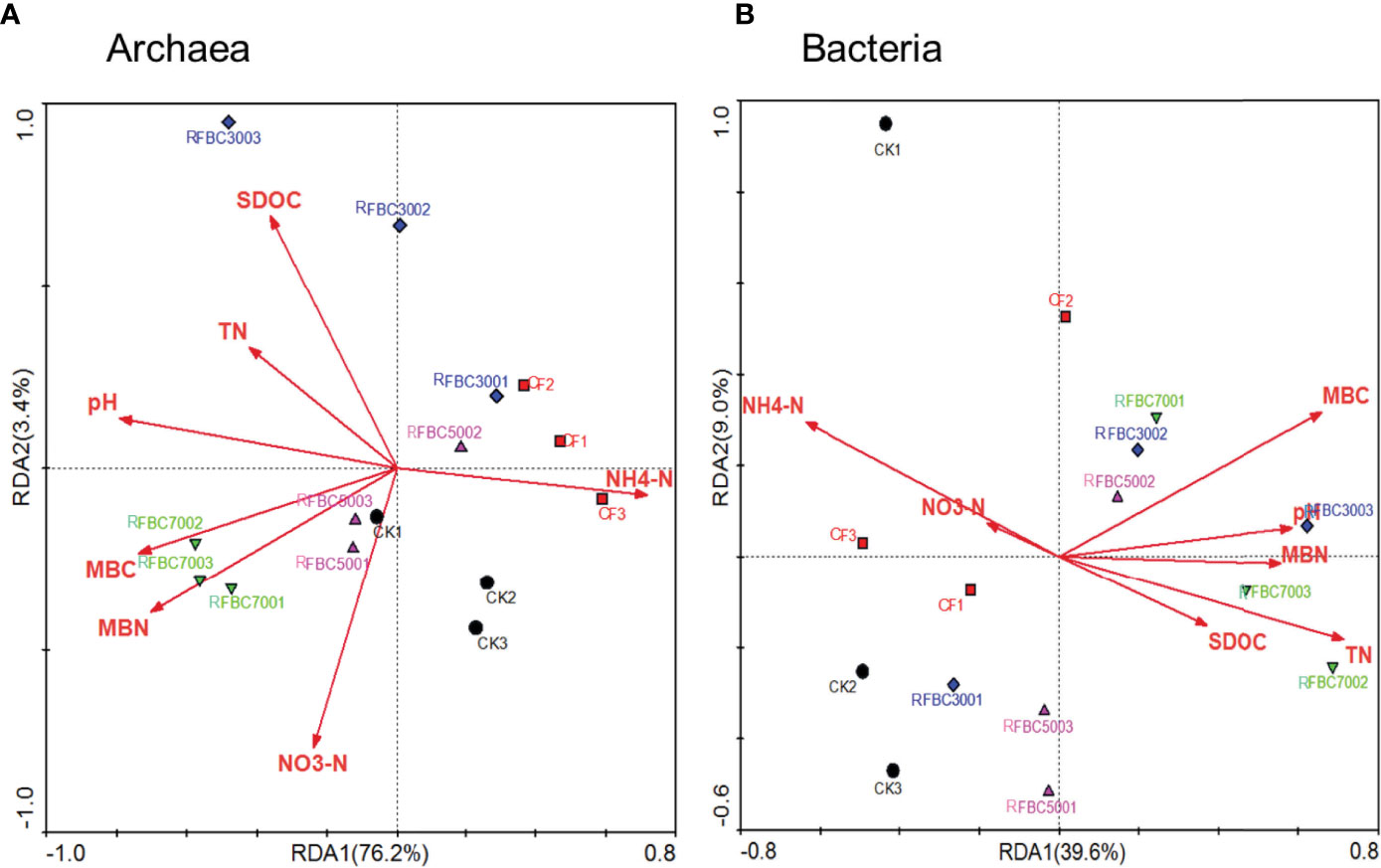
Figure 6 Redundancy analysis (RDA) of archaeal (A) and bacterial (B) community composition at class level in soil samples collected after 55 days column leaching of paddy soil incorporated with or without biochar amendments and/or fertilizer (i.e. CK: Control treatment without biochar and fertilizer; F: Only fertilizer added; FBC300: 300°C biochar combined with fertilizer; FBC500: 500°C biochar combined with fertilizer; FBC700: 700°C biochar combined with fertilizer).
From the perspective of microbiology, CH4 emissions from paddy soil largely depend on the methanogenic archaeal and methanotrophic proteobacterial communities. Therefore, the application of methanotroph consortium can be considered to regulate the methane emission, meanwhile, increase the grain yield (48).
Conclusion
In this study, biochar produced at different temperature expresses various agricultural properties, such as the pH, CEC, EC and C, N contents, and therefore, biochar results in disparate functions when incorporated into the soil. Through column leaching simulation and from microbiological information, we found that the biochar amendments not only improve the availability of soil nutrients but also stimulate soil microbial activities. As a result, it is possible that the methanogenic archaea were stimulated more than the methanotrophic bacteria under the biochar amendments, resulting in higher CH4 emissions from paddy soil. Thus, the use of biochar amendments combined with fertilizer should be prudently considered. However, since our study was a laboratory simulation test, and limited replicates were set, a field trial and/or long-term fertilization experiment is merited in the future to offer more explicit answers of biochar application in the paddy fields, including CH4 emissions, to determine whether it influences soil properties and rice yields.
Data Availability Statement
The datasets presented in this study can be found in online at https://www.ncbi.nlm.nih.gov/ with the accession number PRJNA852553, and in the Supplementary Material. Any further enquires should be directed to the corresponding author.
Author Contributions
JG: Draft of the manuscript. LL: Analysis of the data. ZS: Development of the corresponding method. JL: Amendment of the manuscript. All authors contributed to the article and approved the submitted version.
Funding
This research was funded by the Shaanxi Science and Technology Coordination Innovation Project (2016KTZDNY03-01). This study received funding from China Tobacco Henan Industrial Limited Company (2020410001340006). The funder was not involved in the design of experiments, the writing of paper and the decision to submit it for publication.
Conflict of Interest
The authors declare that the research was conducted in the absence of any commercial or financial relationships that could be construed as a potential conflict of interest.
Publisher’s Note
All claims expressed in this article are solely those of the authors and do not necessarily represent those of their affiliated organizations, or those of the publisher, the editors and the reviewers. Any product that may be evaluated in this article, or claim that may be made by its manufacturer, is not guaranteed or endorsed by the publisher.
Acknowledgments
We acknowledge the co-authors’ contributions for this work, the reviewers and the journal Editors for their helpful professional suggestions that greatly improved the quality of this manuscript.
Supplementary Material
The Supplementary Material for this article can be found online at: https://www.frontiersin.org/articles/10.3389/fsoil.2022.801227/full#supplementary-material
References
1. Feng Z, Zhu L. Impact of Biochar on Soil N2O Emissions Under Different Biochar-Carbon/Fertilizer-Nitrogen Ratios at a Constant Moisture Condition on a Silt Loam Soil. Sci Tot. Environ (2017) 584–5:776–82. doi: 10.1016/j.scitotenv.2017.01.115
2. Neubauer SC, Megonigal JP. Moving Beyond Global Warming Potentials to Quantify the Climatic Role of Ecosystems. Ecosystems (2015) 18:1000–13. doi: 10.1007/s10021-015-9879-4
3. Kong D, Li S, Jin Y, Wu S, Chen J, Hu T, et al. Linking Methane Emissions to Methanogenic and Methanotrophic Communities Under Different Fertilization Strategies in Rice Paddies - Sciencedirect. Geoderma (2018) 347:233–43. doi: 10.1016/j.geoderma.2019.04.008
4. IPCC. In Climate Change 2001 (2001). Available at: http://www.grida.no/publications/other/ipcc_tar/?src¼/climate/ipcc_tar/wg1/.
5. Su J, Hu C, Yan X, Jin Y, Chen Z, Guan Q. Expression of Barley Susiba2 Transcription Factor Yields High-Starch Low-Methane Rice. Nature (2015) 523(7562):602–6. doi: 10.1038/nature14673
6. Watanabe T, Kimura M, Asakawa S. Dynamics of Methanogenic Archaeal Communities Based on rRNA Analysis and Their Relation to Methanogenic Activity in Japanese Paddy Field Soils. Soil Biol Biochem (2007) 39:2877e2887. doi: 10.1016/j.soilbio.2007.05.030
7. Bosse U, Frenzel P. Activity and Distribution of Methane-Oxidizing Bacteria in Flooded Rice Soil Microcosms and in Rice Plants (Oryza Sativa). Appl Environ Microbiol (1997) 63(4):1199–207. doi: 10.1128/aem.63.4.1199-1207.1997
8. Feng Y, Xu Y, Yu Y, Xie Z, Lin X. Mechanisms of Biochar Decreasing Methane Emission From Chinese Paddy Soils. Soil Biol Biochem (2012) 46(1):80–8. doi: 10.1016/j.soilbio.2011.11.016
9. Khan S, Chao C, Waqas M, Arp HP, Zhu YG. Sewage Sludge Biochar Influence Upon Rice (Oryza Sativa L) Yield, Metal Bioaccumulation and Greenhouse Gas Emissions From Acidic Paddy Soil. Environ Sci Technol (2013) 47(15):8624–32. doi: 10.1021/es400554x
10. Butnan S, Deenik JL, Toomsan B, Antal MJ, Vityakon P. Biochar Characteristics and Application Rates Affecting Corn Growth and Properties of Soils Contrasting in Texture and Mineralogy. Geoderma (2015) 237–238:105–16. doi: 10.1016/j.geoderma.2014.08.010
11. Van Zwieten L, Kimber S, Morris S, Chan KY, Downie A, Rust J, et al. Effects of Biochar From Slow Pyrolysis of Papermill Waste on Agronomic Performance and Soil Fertility. Plant Soil. (2010) 327:235e246. doi: 10.1007/s11104-009-0050-x
12. Demisie W, Liu Z, Zhang M. Effect of Biochar on Carbon Fractions and Enzyme Activity of Red Soil. Catena (2014) 121(5):214–21. doi: 10.1016/j.catena.2014.05.020
13. Liang B, Lehmann J, Sohi SP, Thies JE, O’Neill B, Trujillo L. Black Carbon Affects the Cycling of non-Black Carbon in Soil. Org Geochem. (2010) 41(2):206–13. doi: 10.1016/j.orggeochem.2009.09.007
14. Gul S, Whalen JK, Thomas BW, Sachdeva V, Deng H. Physico-Chemical Properties and Microbial Responses in Biochar-Amended Soils: Mechanisms and Future Directions. Agr. Ecosyst Environ (2015) 206:46–59. doi: 10.1016/j.agee.2015.03.015
15. Quilliam RS, Glanville HC, Wade SC, Jones DL. Life in the ‘Charosphere’ – Does Biochar in Agricultural Soil Provide a Significant Habitat Formicroorganisms? Soil Biol Biochem (2013) 65:287–93. doi: 10.1016/j.soilbio.2013.06.004
16. Case SD, Mcnamara NP, Reay DS, Whitaker J. The Effect of Biochar Addition on N2O and CO2 Emissions From a Sandy Loam Soilethe Role of Soil Aeration. Soil Biol Biochem (2012) 51:125e134. doi: 10.1016/j.soilbio.2012.03.017
17. Cheng Y, Cai ZC, Chang SX, Wang J, Zhang JB. Wheat Straw and its Biochar Have Contrasting Effects on Inorganic N Retention and N2O Production in a Cultivated Black Chernozem. Biol Fertil. Soil. (2012) 48:941–6.
18. Zhang A, Cui L, Pan G, Li L, Hussain Q, Zhang X. Effect of Biochar Amendment on Yield and Methane and Nitrous Oxide Emissions From a Rice Paddy From Tai Lake Plain, China. Agr Ecosyst Environ (2010) 139(4):469–75. doi: 10.1016/j.agee.2010.09.003
19. Liu Y, Yang M, Wu Y, Wang H, Chen Y, Wu W. Reducing CH4 and CO2 Emissions From Waterlogged Paddy Soil With Biochar. J Soil. Sediment. (2011) 11(6):930–9. doi: 10.1007/s11368-011-0376-x
20. Gao J, Liu L, Shi Z, Lv J. Characteristics of FluorescentDissolved Organic Matter in Paddy SoilAmended With Crop Residues After Column (0–40 cm) Leaching. Front Environ Sci (2022) 10:766795. doi: 3389/fenvs.2022.766795
21. Paz-Ferreiro J, Fu S, Méndez A, Gascó G. Interactive Effects of Biochar and the Earthworm Pontoscolex Corethrurus, on Plant Productivity and Soil Enzyme Activities. J Soil. Sediment. (2014) 14(3):483–94. doi: 10.1007/s11368-013-0806-z
22. Munyaka PM, Khafipour A, Wang H, Eissa N, Khafipour E, Ghia JE. Mo1774 Prenatal Antibiotic Treatment Increases Offspring’s Susceptibility to Experimental Colitis: A Role of the Gut Microbiota. PloS One (2015) 10(11):e0142536. doi: 10.1016/S0016-5085(15)32404-5
23. Edgar RC. Uparse: Highly Accurate OTU Sequences From Microbial Amplicon Reads. Nat Methods (2013) 10(10):996–8. doi: 10.1038/nmeth.2604
24. Cole JR, Wang Q, Cardenas E, Fish J, Chai B, Farris RJ, et al. The Ribosomal Database Project: Improved Alignments and New Tools for rRNA Analysis. Nucleic Acids Res (2009) 37(Database issue):D141–5. doi: 10.1093/nar/gkn879
25. Jami E, Israel A, Kotser A, Mizrahi I. Exploring the Bovine Rumen Bacterial Community From Birth to Adulthood. Isme J (2013) 7(6):1069. doi: 10.1038/ismej.2013.2
26. Luo L, Xu C, Chen Z, Zhang S. Properties of Biomass-Derived Biochars: Combined Effects of Operating Conditions and Biomass Types. Bioresour. Technol (2015) 192:83–9. doi: 10.1016/j.biortech.2015.05.054
27. Subedi R, Taupe N, Pelissetti S, Petruzzelli L, Bertora C, Leahy JJ. Greenhouse Gas Emissions and Soil Properties Following Amendment With Manure-Derived Biochars: Influence of Pyrolysis Temperature and Feedstock Type. J Environ Manage (2016) 166:73–83. doi: 10.1016/j.jenvman.2015.10.007
28. Wang N, Chang ZZ, Xue XM, Yu JG, Shi XX, Ma LQ. Biochar Decreases Nitrogen Oxide and Enhances Methane Emissions via Altering Microbial Community Composition of Anaerobic Paddy Soil. Sci Tot. Environ (2017) 581-582:689–96. doi: 10.1016/j.scitotenv.2016.12.181
29. Yuan JH, Xu RK, Zhang H. The Forms of Alkalis in the Biochar Produced From Crop Residues at Different Temperatures. Bioresour. Technol (2011) 102(3):3488–97. doi: 10.1016/j.biortech.2010.11.018
30. Enders A, Hanley K, Whitman T, Joseph S, Lehmann J. Characterization of Biochars to Evaluate Recalcitrance and Agronomic Performance. Bioresour. Technol (2012) 114(3):644. doi: 10.1016/j.biortech.2012.03.022
31. Joseph SD, Campsarbestain M, Lin Y, Munroe P, Chia CH, Hook J. An Investigation Into the Reactions of Biochar in Soil. Soil Res (2010) 48(7):5. doi: 10.1071/SR10009
32. Zhao L, Cao X, Mašek O, Zimmerman A. Heterogeneity of Biochar Properties as a Function of Feedstock Sources and Production Temperatures. J Haz. Mater (2013) 256-257(1):1–9. doi: 10.1016/j.jhazmat.2013.04.015
33. Vamvuka D, Karouki E, Sfakiotakis S. Gasification of Waste Biomass Chars by Carbon Dioxide via Thermogravimetry. Part I: Effect of Mineral Matter. Fuel (2011) 90(3):1120–7. doi: 10.1016/j.fuel.2010.12.001
34. Qin X, Li Y, Hong W, Chong L, Li J, Wan Y. Long-Term Effect of Biochar Application on Yield-Scaled Greenhouse Gas Emissions in a Rice Paddy Cropping System: A Four-Year Case Study in South China. Sci Tot. Environ (2016) 569-570:1390–401. doi: 10.1016/j.scitotenv.2016.06.222
35. Kuzyakov Y, Bogomolova I, Glaser B. Biochar Stability in Soil: Decomposition During Eight Years and Transformation as Assessed by Compound-Specific 14 C Analysis. Soil Biol Biochem (2014) 70(6):229–36. doi: 10.1016/j.soilbio.2013.12.021
36. Shen J, Tang H, Liu J, Wang C, Li Y, Ge T. Contrasting Effects of Straw and Straw-Derived Biochar Amendments on Greenhouse Gas Emissions Within Double Rice Cropping Systems. Agric Ecosyst Environ (2014) 188:264–74. doi: 10.1016/j.agee.2014.03.002
37. Yu L, Tang J, Zhang R, Wu Q, Gong M. Effects of Biochar Application on Soil Methane Emission at Different Soil Moisture Levels. Biol Fertil. Soil. (2013) 49:119–28. doi: 10.1007/s00374-012-0703-4
38. Dong D, Yang M, Wang C, Wang H, Li Y, Luo J. Responses of Methane Emissions and Rice Yield to Applications of Biochar and Straw in a Paddy Field. J Soil. Sediment. (2013) 13:1450–60. doi: 10.1007/s11368-013-0732-0
39. Sonoki T, Furukawa T, Jindo K, Suto K, Aoyama M, Sánchez-Monedero M.á. Influence of Biochar Addition on Methane Metabolism During Thermophilic Phase of Composting. J Bas. Microbiol (2013) 53:617–21. doi: 10.1002/jobm.201200096
40. Watanabe A, Yoshida M, Kimura M. Contribution of Rice Straw Carbon to CH4 Emission From Rice Paddies Using 13C-Enriched Rice Straw. J Geophys Res Atmos. (1998) 103:8237–42. doi: 10.1029/97JD03460
41. Wang J, Pan X, Liu Y, Zhang X, Xiong Z. Effects of Biochar Amendment in Two Soils on Greenhouse Gas Emissions and Crop Production. Plant Soil (2012) 360(1-2):287–98. doi: 10.1007/s11104-012-1250-3
42. Kögel-Knabner I, Amelung W, Cao Z, Fiedler S, Frenzel P, Jahn R. Biogeochemistry of Paddy Soils. Geoderma (2010) 157:1–14.01-515. doi: 10.1016/j.geoderma.2010.03.009
43. Lehmann J, Rillig MC, Thies J, Masiello CA, Hockaday WC, Crowley D. Biochar Effects on Soil Biota–a Review. Soil Biol Biochem (2011) 43:1812–36. doi: 10.1016/j.soilbio.2011.04.022
45. Steinbeiss S, Gleixner G, Antonietti M. Effect of Biochar Amendment on Soil Carbon Balance and Soil Microbial Activity. Soil Biol Biochem (2009) 41(6):1301–10. doi: 10.1016/j.soilbio.2009.03.016
46. Azeem M, Hayat R, Hussain Q, Ahmed M, Imran M, Crowley DE. Effect of Biochar Amendment on Soil Microbial Biomass, Abundance and Enzyme Activity in the Mash Bean Field. J Biod. Environ Sci (2016) 8(6):2222–3045.
47. Watanabe T, Kimura M, Asakawa S. Community Structure of Methanogenic Archaea in Paddy Field Soil Under Double Cropping (Rice–Wheat). Soil Biol Biochem (2006) 38(6):1264–74. doi: 10.1016/j.soilbio.2005.09.020
Keywords: biochar, CH4 emission, paddy soil, dissolved organic carbon, soil
Citation: Gao J, Liu L, Shi Z and Lv J (2022) Biochar Amendments Facilitate Methane Production by Regulating the Abundances of Methanogens and Methanotrophs in Flooded Paddy Soil. Front. Soil Sci. 2:801227. doi: 10.3389/fsoil.2022.801227
Received: 25 October 2021; Accepted: 17 May 2022;
Published: 01 July 2022.
Edited by:
Helen Catherine Glanville, Keele University, United KingdomReviewed by:
Ali El-Naggar, Ain Shams University, EgyptBharati Kollah, Indian Institute of Soil Science (ICAR), India
Copyright © 2022 Gao, Liu, Shi and Lv. This is an open-access article distributed under the terms of the Creative Commons Attribution License (CC BY). The use, distribution or reproduction in other forums is permitted, provided the original author(s) and the copyright owner(s) are credited and that the original publication in this journal is cited, in accordance with accepted academic practice. No use, distribution or reproduction is permitted which does not comply with these terms.
*Correspondence: Jialong Lv, ljlll@nwsuaf.edu.cn