- 1Department of Physics and Atmospheric Science, Dalhousie University, Halifax, NS, Canada
- 2School of Biomedical Engineering, Dalhousie University, Halifax, NS, Canada
Collagen is one of the main constituents of mammalian extracellular matrix and is used extensively as a coating for tissue culture dishes and medical implants to promote cell growth and proliferation. By modulating the topography of the collagen coating at the nanometer to micrometer length scales, it is possible to achieve spatial control over cell growth and morphology. In this work, we are exploring the self-assembly of a thin collagen film on a glass substrate as a way to create new nanoscale surface features. By controlling the collagen concentration and adding an oscillatory flow, we are able to enrich the collagen film surface with a localized pattern of ripples oriented perpendicular to the flow direction. We propose that these ripples are the result of dewetting of the collagen film that leads to the formation of adjacent holes. We observe that individual holes form with an anisotropic rim due to the microstructure of the deposited collagen fibril network. This intrinsic anisotropy and the oscillatory flow yield new holes being formed in the film next to existing rims. As holes keep growing deeper, the rims extend along the flow direction, and the holes appear rectangular in shape, which gives the linear array of holes the apparent morphology of a ripple. Overall, we are able to create localized ripples at the surface of collagen films that would be difficult to produce via standard nanofabrication techniques.
Introduction
Fibrillar collagen type I is a key constituent of the extracellular matrix of mammalian connective tissues (Revell et al., 2021). Collagen fibrils are essential for the structural integrity of these tissues and act as a hub for a host of molecular interactions, including binding sites for key cell receptors such as integrins (San Antonio et al., 2020). As a result, collagen type I, along with other extracellular matrix proteins, is often used as a coating to promote cell adhesion and growth on non-biological substrates such as glass and plastic dishes for tissue culture (Elliott et al., 2003; Elliott et al., 2007) and metal (Müller et al., 2006) or polymer (Liu et al., 2011) implants. However, a protein coating is generally not enough to control cell behavior on a substrate, especially when trying to specifically differentiate stem cells (Fu et al., 2011). This is because cells are sensitive to the topography of the substrate in the nanometers to micrometers range (Leclech and Barakat, 2021). In order to combine biological and topographical cues, researchers typically use nano- and micro-fabrication techniques to structure the substrate, followed by functionalization with proteins and polysaccharides. One example is an array of parallel trenches that can promote cell alignment through contact guidance. Such pattern can easily be produced on a flat substrate by photolithography at the microscale (Andersson et al., 2003) and ion or electron beam etching at the nanoscale (Yang and Keller, 2021). However, these fabrication techniques are more challenging on curved surfaces (Ruchhoeft et al., 1999) or complex geometries.
Another strategy to create protein coatings with well-defined nanoscale or microscale features is to take advantage of self-assembly and surface instabilities such as dewetting that lead to hole formation. Dewetting of a thin liquid film is a multistep process that leads to pattern formation (Mukherjee and Sharma, 2015). This process has been extensively studied in polymer thin films due to slow dynamics and the availability of atomic force microscopy as a high-resolution surface imaging technique. It starts with hole nucleation with a uniform rim and a small depression in the film just outside the rim that can serve as the nucleation site for a new hole (Seemann et al., 2001; Kargupta and Sharma, 2002). In some cases, nucleation at the rim leads to the formation of chains of holes and ultimately, the surface becomes patterned with close packed holes, creating a continuous pattern of ridges from the rims (Mukherjee and Sharma, 2015). For this process to develop, the constituent of the film must be able to diffuse.
In the case of Collagen I, self-assembly in solution starts by nucleation of thin linear aggregates, microfibrils, comprising 5 axially staggered collagen triple helices in cross-sections about 4 nm in diameter (Darvish, 2022). This nucleation phase is diffusion limited and is observed as a lag phase that can last for tens of minutes, depending on collagen concentration, in optical density measurements (Darvish, 2022). Microfibrils proceed to fuse end to end and laterally to create collagen fibrils with diameters of up to a few hundred nanometers. Lateral aggregation is observed as a steep increase in optical density measurements just after the lag phase (Darvish, 2022). Adding a crystalline and charged surface like mica can direct microfibril growth and lateral assembly along crystallographic directions, leading to textured films, especially at collagen concentrations below 0.05 mg/mL (Leow and Hwang, 2011). For amorphous surfaces such as glass, collagen assembly leads to the formation of a thin layer of tightly packed microfibrils or thin fibrils on which is further deposited a network of mature collagen fibrils (Elliott et al., 2003). Using flow, it is possible to align microfibrils and thin fibrils as they deposit (Saeidi et al., 2011) and delay, in principle, the deposition of the collagen fibril network from solution, allowing, the collagen film to nucleate holes. Alternatively, decreasing the concentration of collagen should have a similar effect.
In this study, we expose glass substrates to collagen I solution at the start of assembly in the absence or presence of an oscillatory flow. As long as the thickness of the collagen film is lower than 100 nm after drying, we observe the formation of holes and a strong tendency for holes to nucleate next to existing holes, as observed in polymer thin films. Holes are observed without flow for short incubation times and low concentrations. Turning on the flow appears to have the same effect as reducing the collagen concentration without flow. As a result of this hole formation process, we are able to produce thin films of collagen with a unique nanostructure that may improve the biocompatibility of surfaces used in tissue culture and implanted medical devices.
Materials and methods
Collagen thin films preparation
All collagen films analyzed in this study were prepared using pepsin solubilized (atelocollagen) bovine collagen type I in 0.01 M HCl at a concentration of 3 mg/mL (PureCol, Advanced Biomatrix). Collagen self-assembly was initiated using the warm-start method at 30°C (Kadler et al., 1987). For each thin film, we used 10 mL of atelocollagen in phosphate buffered saline (PBS) at a final concentration of 0.03, 0.15 or 0.3 mg/mL. The substrate was a glass coverslip (24 mm by 60 mm) placed at the bottom of a plastic box (30 mm by 75 mm by 20 mm). The box was filled with the entire 10 mL of warm solution and placed immediately in an incubator at 30°C for 1, 3 or 24 h. During incubation, the box was either not shaken or placed on a linear shaker table oscillating at 1, 5, 10 or 30 Hz. After incubation, glass coverslips were washed with nanopure water and dried under a nitrogen stream.
Atomic force microscopy
The surface of each collagen thin film was imaged using an atomic force microscope (AFM) (Agilent 5,500) operating in amplitude controlled resonance mode (AC). Silicon cantilevers with either 150 or 300 kHz, nominal natural frequency (Budget Sensors), were used for all samples. Free oscillation amplitude was set to 2 V and scanning frequency was set to 1 Hz. All images were acquired at a resolution of 512 by 512 pixels and a typical scan size of 20 μm. The glass substrates were always imaged in such a way that the flow direction was always horizontal. For each thin film, we imaged at least 3 different locations. All images were processed using Gwyddion (http://www.gwyddion.net). Specifically, images were levelled using a second order polynomial fit applied line by line. To estimate the thickness of the film we used the minimum height measured after levelling. For display purposes images were oversampled 4 times using 4th-order Schaum interpolation as implemented in Gwyddion.
Results
Observation of arrays of holes in thin collagen films
For this study, we produced 27 collagen thin films and randomly collected 128 images each over an area of 20 × 20 μm2. Using the maximum depth of each image after levelling, we were able to obtain thickness estimates for each film (Table 1). Images could be separated into 3 categories, regions with ultrathin collagen films with an average thickness of 17 ± 5 nm (n = 34) that show no evidence of holes with a non-uniform rim, regions with holes with a non-uniform rim that have a thickness between 10 and 96 nm (average of 50 ± 24 nm, n = 80), and 15 images all obtained after 24 h incubation that were covered with a dense network of collagen fibrils (Table 1). In most cases, the surface did not appear uniform in thickness, and the holes tended to form next to each other, creating a pattern of holes and rims that resembles ripples in a buckled thin film (Stafford et al., 2004) (Figure 1). Note that we do not observe a sharp thickness threshold for hole formation as we observed many regions without holes where the film was apparently thicker than 10 nm.
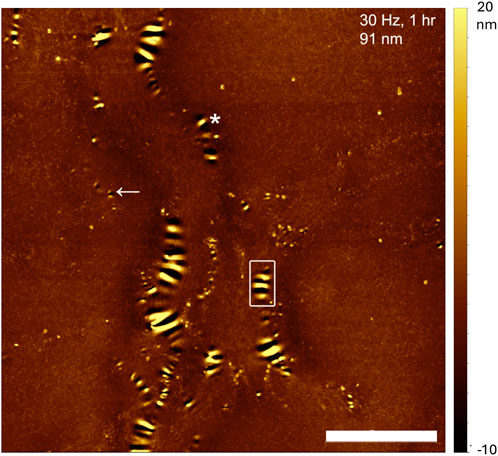
Figure 1. AFM image of a collagen thin film on glass obtained after 1 h incubation of 0.3 mg/mL collagen in PBS at 30°C and a shaking frequency of 30 Hz. The film thickness is 91 nm and the shaking direction was horizontal. Many holes, elliptical in appearance, of various lengths are clearly visible and clustered next to each other, creating ripples. Scale bar is 5 μm.
Across all thin films, the hole-rim patterns shared the following characteristics. The long axis of the rims was generally parallel to the length of the rectangular glass plates which was also the direction of shaking (Figure 1). Holes appeared to form first either on their own, surrounded by an incomplete rim (Figure 1, arrow) or as a pair of holes sharing an incomplete rim (Figure 1, asterisk). Hole-rim patterns appeared to be tallest or deepest at their center and profiles taken perpendicular to the rim’s long axis had a characteristic wave-packet appearance (Figure 2). Peak to peak distances along the trace are all slightly different from 400 to 500 nm (Figure 2) indicating that this is not a truly periodic phenomenon.
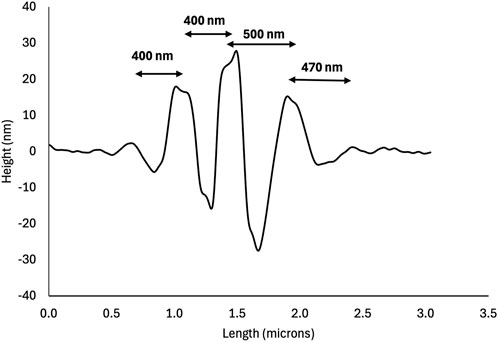
Figure 2. AFM height profile of the hole-rim pattern boxed in Figure 1. Note the wave-packet shape of the profile. The central rim is the highest and is surrounded by two deep holes indicating that these two holes nucleated as a pair sharing the same rim as in Figure 1 (see asterisk).
Effect of collagen concentration and incubation time on film thickness and hole formation
In the absence of shaking, the deposited film thickness and the presence of holes were a function of both incubation time and collagen concentration. Collagen thin films obtained by deposition of 0.3 mg/mL Collagen I in PBS at 30°C without shaking revealed a progressive accumulation of fibrils at the surface of the film from a few short fibrils after 1 h incubation (Figure 3A), to longer fibrils after 3 h (Figure 3B) and finally an extensive fibril network after 24 h (Figure 3C). As the incubation time increases, the thickness of the film appears to increase from 14 to 273 nm (Figure 3; Table 1). Small arrays of holes were visible after 1 or 3 h incubation but not at 24 h, where the surface was covered by a network of fibrils.
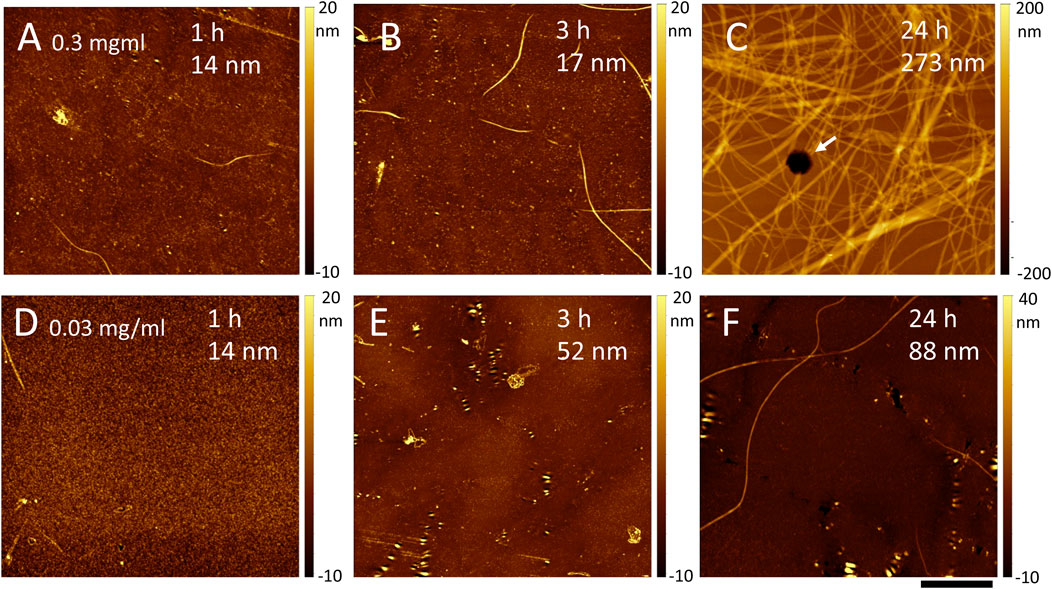
Figure 3. AFM images of a collagen thin film on glass obtained after incubation of 0.3 (A–C) or 0.03 (D–F) mg/ml collagen in PBS at 30°C and no shaking. The incubation time and estimated film thickness is indicated on each image. Scale bar is 5 μm for all images. In C, a hexagonal hole is visible as a black spot in the middle of the image (arrow).
Decreasing Collagen I concentration to 0.03 mg/mL led to very little deposition after 1 h incubation (Figure 3D). After 3 h, arrays of non-round holes were clearly visible (Figure 3E) with a film thickness around 50 nm (Table 1). Finally, at 24 h, the film had almost doubled in thickness to about 86 nm on average (Table 1) and both holes and long fibrils were visible (Figure 3F).
It is worth pointing out that there was regional variability in thickness, presence or absence of holes and/or fibrils for all samples that were not shaken (Table 1). This is particularly clear for samples that were incubated at a Collagen I concentration of 0.15 mg/mL. We expected a result similar to the 0.3 mg/mL sample after 24 h of incubation and observed a mostly bare substrate (Table 1) with images similar to Figure 3D. We speculate that the collagen fibrils formed a thin gel, that was removed by washing and drying under the nitrogen stream, rather than a layer of fibrils as in Figure 3C.
Shaking enhances hole formation
Shaking a solution of 0.3 mg/mL Collagen I in PBS at 30°C during incubation yielded thin films with non-round holes in all the conditions investigated (Table 1). Increasing the shaking frequency appeared to increase your chance to randomly observe hole formation. Without shaking or at 1 Hz frequency, the chance of observing holes in an image was 46% and 45%, respectively (Table 1). This probability increased to 88% at 5 Hz and 100% at 30 Hz (Table 1). At 10 Hz, the probability dropped to 69% because after 24 h of incubation, a portion of the film was covered with a dense fibril network reminiscent of Figure 3C. In addition to suppressing the deposition of fibrils on the surface, shaking keeps the thickness of the film within a broad range that appears suitable for hole formation (see above and Table 1). After 1 h or 3 h of incubation, there is no effect of shaking frequency on film thickness with averages of 47 ± 20 nm (n = 67) and of 53 ± 16 nm (n = 19), respectively. After 24 h of incubation, the behavior is complex (Supplementary Figure S1), with low frequencies (1 and 5 Hz) leading to a thinning of the films compared to shorter incubation times, whereas at high frequencies (10 and 30 Hz) the average film thickness is 58 ± 24 nm (n = 9) and similar to the values at shorter incubation times. Note that we avoided acquiring images at the border of the glass plates and suspect that at low frequencies, collagen accumulated at both ends of the rectangular box. This is consistent with the observation of a wavy surface pattern parallel to the flow direction in most images of thin films obtained under oscillatory flow (Supplementary Figure S2), which is reminiscent of developing beach sand ripples.
Discussion
Diffusion anisotropy and non-round hole formation
Hole formation is a common occurrence in thin polymer films coated on a substrate as the initial step of dewetting (Mukherjee and Sharma, 2015). Due to the isotropic nature of polymeric fluids, circular holes are generally observed unless confinement is used to generate anisotropic holes (Suh and Lee, 2001). In this study, we present a rare observation of non-round holes forming in an unconfined collagen thin film (Figure 1). For thin polymer films deposited on clean substrates, holes form randomly even so clusters of holes do occur [see Figure 2B in (Mukherjee and Sharma, 2015)]. In the case of collagen thin films, we mostly observe linear arrays of holes rather than individual ones (Figure 1). Hole formation occurs for collagen thin film thicknesses below 100 nm (Table 1), which is comparable to what is observed for polymer films where holes are easily formed in 5–25 nm films (Mukherjee and Sharma, 2015) and nanoscale flows occur over hours in stepped films, 100 nm tall (Chai et al., 2014).
In our case. however, the films have probably a hierarchical nature due to the coupling between sedimentation that favors the deposition of low molecular weight species first and self-assembly that causes the molecular weight of diffusing species to increase in time. This hierarchy is visible at high magnification where the deepest part of the collagen film topography appears as a meshwork of either microfibrils or thin fibrils (Supplementary Figure S3, arrows) on top of which thicker fibrils are deposited. It is unclear whether this thin meshwork as a preferential symmetry however we did observe after 24 h of deposition without flow hexagonal holes that go through the film (Figure 3C, arrow). In crystalline, atomic thin films, anisotropic hole growth can be observed with geometric shapes that mirror the symmetry of the underlying atomic lattice (Amram et al., 2012; Ye and Thompson, 2011). The key difference is that the holes in these atomically crystalline films are anisotropic in shape, but their rims are uniform all around the holes. In our case, all the holes we have observed except for the few hexagonal holes in the thickest films have an elongated shape and non-uniform rims (Figure 1).
This points to an anisotropy in diffusion coefficients within the collagen film rather than an effect of the collagen network structure. The molecular origin of this diffusion anisotropy is likely the high aspect ratio of the collagen molecules and microfibrils, along with their high bending rigidity. Triple helical collagen molecules are about 300 nm long and have a persistence length of 60 nm in water that increases to 120 nm with the addition of 100 mM KCl (Rezaei et al., 2018). Microfibrils are composed of 5 collagen molecules in cross-section, can grow to several micrometers in length, and are expected to be even more rigid than individual molecules; even so, the staggered arrangement of molecules leads to a modulation of the molecular density and elastic properties every 67 nm (Gisbert et al., 2021). Considering the height profile in Figure 2, it is easier for collagen molecules or microfibrils to diffuse along a fraction of their length to create a 200 nm wide hole than to do so in the perpendicular direction.
Comparison with the “bee structure” of bitumen surface
Even so, our observations are consistent with hole formation. It is possible that an entirely different mechanism is at play. The arrays of holes presented in Figure 1 look like ripples and resemble the “bee structure” observed frequently on bitumen films (Yu et al., 2015), including the same wave-packet height profile presented in Figure 2. Bitumen contains multiple chemical phases, including wax. As a film of bitumen is cooled, it is proposed that small patches of stiff, thin, crystalline paraffin wax film start to wrinkle on top of an amorphous paraffin wax phase on which they were originally floating. These wrinkles have a well-defined wavelength and the overall oval shape of a bee’s body giving rise to the name of “bee structure” (Hung and Fini, 2015). It is possible that the shape similarities are just a coincidence for multiple reasons. For collagen films, the ripples do not have one wavelength (Figure 1) or a well-defined oval shape. On a more fundamental level, wrinkling requires both a compliant substrate underneath the stiff, thin film and a compressive load (Jiang et al., 2007). It is unclear how those two conditions are met in a thin collagen film obtained by self-assembly onto a glass substrate that is at least one order of magnitude stiffer than a dry collagen film. One possibility we cannot entirely dismiss is that the ripples occur during the drying process and not as the film is formed in solution. Future studies of this system should endeavor to observe the process in situ, either using atomic force microscopy or an optical technique.
Conclusion
We are reporting the formation of ripple patterns at the surface of self-assembled collagen thin films that we attribute to a not fully understood hole formation process. Our observations show that macromolecular films obtained by a combination of sedimentation and self-assembly yield rich morphologies that can be partially controlled through oscillatory flow. This is opening the road for further studies and practical applications of collagen and other self-assembling fibrillar systems, such as amyloids in the development of nanostructured films for applications in tissue engineering and smart devices (Han et al., 2023).
Data availability statement
Publicly available datasets were analyzed in this study. This data can be found here: https://github.com/Kreplak/Collagen-ripples-data-set.
Author contributions
ME: Supervision, Writing–review and editing, Data curation, Formal Analysis, Investigation, Validation, Visualization. KB: Formal Analysis, Investigation, Validation, Visualization, Writing–review and editing, Methodology. EA: Formal Analysis, Investigation, Methodology, Validation, Visualization, Writing–review and editing. RL: Formal Analysis, Investigation, Methodology, Validation, Visualization, Writing–review and editing. EO: Formal Analysis, Investigation, Methodology, Validation, Visualization, Writing–review and editing. LK: Methodology, Writing–review and editing, Conceptualization, Funding acquisition, Project administration, Resources, Supervision, Writing–original draft.
Funding
The author(s) declare that financial support was received for the research, authorship, and/or publication of this article. LK is the recipient of a Discovery grant award from the Natural Sciences and Engineering Research Council of Canada, RGPIN-2018-03781.
Conflict of interest
The authors declare that the research was conducted in the absence of any commercial or financial relationships that could be construed as a potential conflict of interest.
Publisher’s note
All claims expressed in this article are solely those of the authors and do not necessarily represent those of their affiliated organizations, or those of the publisher, the editors and the reviewers. Any product that may be evaluated in this article, or claim that may be made by its manufacturer, is not guaranteed or endorsed by the publisher.
Supplementary material
The Supplementary Material for this article can be found online at: https://www.frontiersin.org/articles/10.3389/frsfm.2024.1448615/full#supplementary-material
References
Amram, D., Klinger, L., and Rabkin, E. (2012). Anisotropic hole growth during solid-state dewetting of single-crystal Au–Fe thin films. Acta Mater. 60, 3047–3056. doi:10.1016/j.actamat.2012.02.009
Andersson, A.-S., Olsson, P., Lidberg, U., and Sutherland, D. (2003). The effects of continuous and discontinuous groove edges on cell shape and alignment. Exp. Cell Res. 288, 177–188. doi:10.1016/s0014-4827(03)00159-9
Chai, Y., Salez, T., McGraw, J. D., Benzaquen, M., Dalnoki-Veress, K., Raphaël, E., et al. (2014). A direct quantitative measure of surface mobility in a glassy polymer. Science 343, 994–999. doi:10.1126/science.1244845
Darvish, D. M. (2022). Collagen fibril formation in vitro: from origin to opportunities. Mater. Today Bio 15, 100322. doi:10.1016/j.mtbio.2022.100322
Elliott, J. T., Tona, A., Woodward, J. T., Jones, P. L., and Plant, A. L. (2003). Thin films of collagen affect smooth muscle cell morphology. Langmuir 19, 1506–1514. doi:10.1021/la026216r
Elliott, J. T., Woodward, J. T., Umarji, A., Mei, Y., and Tona, A. (2007). The effect of surface chemistry on the formation of thin films of native fibrillar collagen. Biomaterials 28, 576–585. doi:10.1016/j.biomaterials.2006.09.023
Fu, R.-H., Wang, Y.-C., Liu, S.-P., Huang, C.-M., Kang, Y.-H., Tsai, C.-H., et al. (2011). Differentiation of stem cells: strategies for modifying surface biomaterials. Cell Transpl. 20, 37–47. doi:10.3727/096368910x532756
Gisbert, V. G., Benaglia, S., Uhlig, M. R., Proksch, R., and Garcia, R. (2021). High-speed nanomechanical mapping of the early stages of collagen growth by bimodal force microscopy. ACS Nano 15, 1850–1857. doi:10.1021/acsnano.0c10159
Han, Y., Cao, Y., Zhou, J., Yao, Y., Wu, X., Bolisetty, S., et al. (2023). Interfacial electrostatic self-assembly of amyloid fibrils into multifunctional protein films. Adv. Sci. 10, 2206867. doi:10.1002/advs.202206867
Hung, A. M., and Fini, E. H. (2015). AFM study of asphalt binder “bee” structures: origin, mechanical fracture, topological evolution, and experimental artifacts. RSC Adv. 5, 96972–96982. doi:10.1039/c5ra13982a
Jiang, H., Khang, D.-Y., Song, J., Sun, Y., Huang, Y., and Rogers, J. A. (2007). Finite deformation mechanics in buckled thin films on compliant supports. Proc. Natl. Acad. Sci. 104, 15607–15612. doi:10.1073/pnas.0702927104
Kadler, K. E., Hojima, Y., and Prockop, D. J. (1987). Assembly of collagen fibrils de novo by cleavage of the type I pC-collagen with procollagen C-proteinase. Assay of critical concentration demonstrates that collagen self-assembly is a classical example of an entropy-driven process. J. Biol. Chem. 262, 15696–15701. doi:10.1016/s0021-9258(18)47783-6
Kargupta, K., and Sharma, A. (2002). Creation of ordered patterns by dewetting of thin films on homogeneous and heterogeneous substrates. J. Colloid Interface Sci. 245, 99–115. doi:10.1006/jcis.2001.7860
Leow, W. W., and Hwang, W. (2011). Epitaxially guided assembly of collagen layers on mica surfaces. Langmuir 27, 10907–10913. doi:10.1021/la2018055
Liu, X., Yue, Z., Higgins, M. J., and Wallace, G. G. (2011). Conducting polymers with immobilised fibrillar collagen for enhanced neural interfacing. Biomaterials 32, 7309–7317. doi:10.1016/j.biomaterials.2011.06.047
Mukherjee, R., and Sharma, A. (2015). Instability, self-organization and pattern formation in thin soft films. Soft Matter 11, 8717–8740. doi:10.1039/c5sm01724f
Müller, R., Abke, J., Schnell, E., Scharnweber, D., Kujat, R., Englert, C., et al. (2006). Influence of surface pretreatment of titanium- and cobalt-based biomaterials on covalent immobilization of fibrillar collagen. Biomaterials 27, 4059–4068. doi:10.1016/j.biomaterials.2006.03.019
Revell, C. K., Jensen, O. E., Shearer, T., Lu, Y., Holmes, D. F., and Kadler, K. E. (2021). Collagen fibril assembly: new approaches to unanswered questions. Matrix Biol. Plus 12, 100079. doi:10.1016/j.mbplus.2021.100079
Rezaei, N., Lyons, A., and Forde, N. R. (2018). Environmentally controlled curvature of single collagen proteins. Biophys. J. 115, 1457–1469. doi:10.1016/j.bpj.2018.09.003
Ruchhoeft, P., Colburn, M., Choi, B., Nounu, H., Johnson, S., Bailey, T., et al. (1999). Patterning curved surfaces: template generation by ion beam proximity lithography and relief transfer by step and flash imprint lithography. J. Vac. Sci. Technol. B Microelectron. Nanom. Struct. Process. Meas. Phenom. 17, 2965–2969. doi:10.1116/1.590935
Saeidi, N., Sander, E. A., Zareian, R., and Ruberti, J. W. (2011). Production of highly aligned collagen lamellae by combining shear force and thin film confinement. Acta Biomater. 7, 2437–2447. doi:10.1016/j.actbio.2011.02.038
San Antonio, J. D., Jacenko, O., Fertala, A., and Orgel, J. P. (2020). Collagen structure-function mapping informs applications for regenerative medicine. Bioengineering 8, 3. doi:10.3390/bioengineering8010003
Seemann, R., Herminghaus, S., and Jacobs, K. (2001). Shape of a liquid front upon dewetting. Phys. Rev. Lett. 87, 196101. doi:10.1103/physrevlett.87.196101
Stafford, C. M., Harrison, C., Beers, K. L., Karim, A., Amis, E. J., VanLandingham, M. R., et al. (2004). A buckling-based metrology for measuring the elastic moduli of polymeric thin films. Nat. Mater. 3, 545–550. doi:10.1038/nmat1175
Suh, K. Y., and Lee, H. H. (2001). Anistropic hole formation in thin polymer films confined by walls. J. Chem. Phys. 115, 8204–8208. doi:10.1063/1.1409540
Yang, Y., and Keller, A. (2021). Ion beam nanopatterning of biomaterial surfaces. Appl. Sci. 11, 6575. doi:10.3390/app11146575
Ye, J., and Thompson, C. V. (2011). Anisotropic edge retraction and hole growth during solid-state dewetting of single crystal nickel thin films. Acta Mater. 59, 582–589. doi:10.1016/j.actamat.2010.09.062
Keywords: thin film, self-assembly, ripples, nanoscale, topography
Citation: Erkan M, Blakney K, Andrews E, Leslie R, Ozsan E and Kreplak L (2024) Formation of linear arrays of holes in self-assembled collagen films. Front. Soft Matter 4:1448615. doi: 10.3389/frsfm.2024.1448615
Received: 13 June 2024; Accepted: 15 August 2024;
Published: 28 August 2024.
Edited by:
Thomas A. Vilgis, Max Planck Institute for Polymer Research, GermanyReviewed by:
Yoshihisa Fujii, Mie University, JapanBruno Zappone, National Research Council (CNR), Italy
Copyright © 2024 Erkan, Blakney, Andrews, Leslie, Ozsan and Kreplak. This is an open-access article distributed under the terms of the Creative Commons Attribution License (CC BY). The use, distribution or reproduction in other forums is permitted, provided the original author(s) and the copyright owner(s) are credited and that the original publication in this journal is cited, in accordance with accepted academic practice. No use, distribution or reproduction is permitted which does not comply with these terms.
*Correspondence: Laurent Kreplak, kreplak@dal.ca