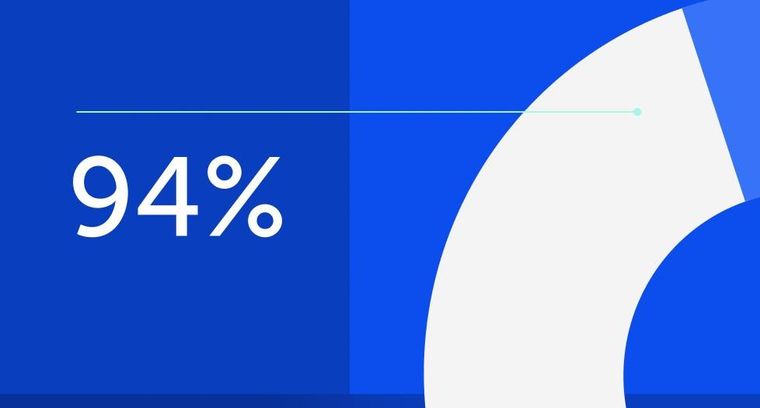
94% of researchers rate our articles as excellent or good
Learn more about the work of our research integrity team to safeguard the quality of each article we publish.
Find out more
ORIGINAL RESEARCH article
Front. Soft Matter, 27 March 2024
Sec. Biological Soft Matter
Volume 4 - 2024 | https://doi.org/10.3389/frsfm.2024.1341129
This article is part of the Research Topic1st Galapagos Soft Matter Conference - Research TopicView all 11 articles
In insects vulnerable to dehydration, the mechanistic reaction of blood after wounding is rapid. It allows insects to minimize blood loss by sealing the wound and forming primary clots that provide scaffolding for the formation of new tissue. Using nano-rheological magnetic rotational spectroscopy with nickel nanorods and extensional rheology, we studied the properties of blood dripping from the wound of caterpillars of the Carolina sphinx moth (Manduca sexta) with a high concentration of blood cells. We discovered that wound sealing followed a two-step scenario. First, in a few seconds, the Newtonian low-viscosity blood turns into a non-Newtonian viscoelastic fluid that minimizes blood loss by retracting the dripping blood back into the wound. Next, blood cells aggregate, starting from the interfaces and propagating inward. We studied these processes using optical phase-contrast and polarized microscopy, X-ray imaging, and modeling. Comparative analyses of the cell-rich and cell-poor blood of different insects revealed common features of blood behavior. These discoveries can help design fast-working thickeners for vertebrate blood, including human blood.
Insects have developed special strategies to deal with wounding and potential infection (Salt, 1970; Wigglesworth and Gupta, 1979; Gillespie et al., 1997; Theopold et al., 2002; Strand, 2008; Dushay, 2009; Jiang et al., 2010; Hillyer and Strand, 2014; Eleftherianos et al., 2021). Wounding triggers humoral and cellular reactions associated with insect blood (hemolymph) (Salt, 1970; Gregoire and Rockstein, 1974; Wigglesworth and Gupta, 1979; Bohn and Brehelin, 1986; Gillespie et al., 1997; Theopold et al., 2002; Strand, 2008; Dushay, 2009; Jiang et al., 2010; Hillyer and Strand, 2014; Eleftherianos et al., 2021). These reactions span spatial scales, from the nanometer to microscopic level, and time scales, from microseconds to hours. At the wound site, a primary clot nucleates within minutes after wounding (Salt, 1970; Wigglesworth and Gupta, 1979), while the formation of a scab and new epidermal tissue happen on a time scale more than two orders of magnitude greater (Salt, 1970; Wigglesworth and Gupta, 1979; Parle et al., 2016). The physical features of primary clot formation in the first minutes of hemolymph leakage from the wound are not sufficiently detailed, and a quantitative analysis of the kinetics of clot nucleation in cell-rich hemolymph was only recently reported (Aprelev et al., 2019).
The concentration of cells (hemocytes) in the blood of different insect species varies significantly; hence, insects developed various strategies for wound sealing. In the hemocyte-poor hemolymph, as in butterflies and moths, the humoral reactions in the plasma cause the self-assembly of lipoproteins (Bohn and Brehelin, 1986; Zdybicka-Barabas and Cytrynska, 2013; Ling et al., 2018; Miserez et al., 2023). Lipoproteins, with their surfactant-like action, recognize the foreign interface and immediately engage hemocytes or other large proteins, such as hemocytin (molecular weights of hemocytin in the moth Bombyx mori and the fly Drosophila melanogaster are ∼260 and ∼300 kDa, respectively) (Hu et al., 2024), to aggregate into filamentary threads consisting of more complex biopolymer subunits (Mulnix et al., 1995) and then into gel-like wound covers (Gregoire and Rockstein, 1974; Bohn and Brehelin, 1986). The gel tightening of the hemocytes at the wound surface renders the complex mesh impermeable to microorganisms and hinders water evaporation.
Lipoproteins are also found in the primary clots of the hemocyte-rich hemolymph (Brehelin, 1979; Boman and Hultmark, 1987; Chino et al., 1987; Mulnix et al., 1995; Theopold et al., 2004; Dushay, 2009; Jiang et al., 2010; Maravilla et al., 2020), such as exchangeable apolipophorin-III in the cockroach Periplaneta americana (Coodin and Caveney, 1992; Dushay, 2009). In contrast to the hemocyte-poor blood of butterflies and moths (Lepidoptera), the hemolymph of their larvae (i.e., caterpillars) is hemocyte-rich. The comparative biomolecular studies of clotting in lepidopteran larvae and adults and in other insects suggest that the hemocytin and lipoproteins common in adults and larvae could be among the first to respond and trigger complex immune reactions, resulting in the formation of filamentary structures and primary clots (Boman and Hultmark, 1987; Mulnix et al., 1995; Theopold et al., 2004; Rahman et al., 2006; Jiang et al., 2010; Zdybicka-Barabas and Cytrynska, 2013; Staczek et al., 2018; Maravilla et al., 2020; Hu et al., 2024).
The limiting step for primary clot formation in the larvae of the Carolina sphinx moth (Manduca sexta) is the aggregation of hemolymph cells (hemocytes), assisted by pseudopodia, the thread-like extensions of hemocytes (Gregoire, 1955). In the larvae of M. sexta, the cells aggregate in approximately 3–5 min. Within a few minutes, the cells consolidate, and the aggregate stiffens, as manifested by a weak elastic reaction (Aprelev et al., 2019) approximately 4–5 min from the moment of aggregation. The primary clot remains soft relative to the repaired wound (Parle et al., 2016) not only in Lepidoptera, such as M. sexta, but also in other insects. In Drosophila, for example, primary clots are initially soft and can be pulled from the hemolymph using a metal needle (Bidla et al., 2005). The biomechanics of primary clot nucleation, consolidation, and stiffening remain poorly understood.
In view of these knowledge gaps, we investigated the material properties of clotting hemolymph to reveal the physical determinants that stop the bleeding. The hemocyte-rich blood of the fifth-instar (i.e., mature) larvae of M. sexta was chosen as our model system.
We first studied the time needed for the insect to react to wounding and to stop the loss of hemocyte-rich hemolymph. For the caterpillars of M. sexta, the time to change the rheological properties of the hemolymph is surprisingly short, i.e., only a few seconds. This fast reaction and the small volume of the available droplets present a challenge to the investigation of the material properties of the hemolymph. Therefore, we developed new approaches to study the rheological and surface properties of the droplets. The comparative analysis of the short-time clotting phenomena associated with the preliminary sealing of the insect wound revealed a general strategy for wound healing: first, the insect turns the Newtonian, almost inviscid hemolymph into a viscoelastic fluid. The acquired elasticity prevents the hemolymph from escaping from the wound. The fluid film or a drop covering the wound surface then forms a crust prepared from a gel-like biopolymer mesh (in hemocyte-poor hemolymph) or a composite hemocyte-polymer mesh (in hemocyte-rich hemolymph).
To evaluate the relevant time scale of the formation of a primary clot, we used a setup (Figure 1A) in which the wound was oriented downward and the hemolymph dripped on its own. Caterpillars of 9–10 g and 1–2 days before pre-pupation were used. Each caterpillar was constrained in a tube, and the hemolymph was extracted via a 1–2-mm incision in the third proleg.
FIGURE 1. (A) Cylindrical plastic sleeve (1) supported by an X-shaped holder (4). The plastic sleeve has a window exposing a portion of the side of the mid-abdomen of a larva of Manduca sexta with a wounded proleg. The dripping hemolymph formed long filaments (2) with droplets. The hemolymph is collected in a Petri dish (3). (B) The sleeve (1) with the caterpillar is flipped upside down, and a metal ball (5) is brought in contact with the wound to form a hemolymph meniscus. The glass rod (6) with the ball (5) is controlled by a manipulator that allows the ball to be lifted to a prescribed height and to form a liquid bridge. (C) Hemolymph filament can be obtained from a pool of hemolymph, using either the same ball (5) as in (B) or by applying a rod (6).
This setup allowed us to probe the dripping hemolymph several seconds after making the incision. Filming the features of the drop and liquid bridge formation had a success rate of approximately 5%, because shortly after the incision, the caterpillar moved vigorously, causing difficulties in focusing the camera. Nonetheless, our naked-eye observations and filming confirmed that in the first several seconds after the incision, the detachment of the falling drop from the wound followed the scenario common for simple fluids, such as water (Eggers, 1997; Montanero and Ponce-Torres, 2020); the drop formed a liquid bridge connecting it with the wound (Figure 2A), and then, the bridge necked (Figures 2B, C) and broke, producing satellites (Figures 2D, E). This behavior suggested that in the first seconds, a mostly water-like, low-viscosity hemolymph dripped from the wound.
FIGURE 2. (A–E) Hemolymph dripping from the wound of a caterpillar several seconds after the wound was made. No filaments were observed, indicating that the hemolymph at this time scale was not highly viscous or elastic.
During the next 5–10 s (i.e., ∼10–15 s after incision), drop detachment showed a different scenario (Figure 3). The necking stage, shown in Figures 2B, C, proceeded similarly (Figures 3A, B), but after that, instead of breaking up the bridge, as shown in Figure 2D, the hemolymph formed filaments of uniform radii (Figure 3C). Before the breakup, the straight filament formed a bulge in the middle (Figure 3D), and the bulge grew to form a second drop (Figures 3E, F). The droplets moved toward the menisci pulled by the connecting filaments. The filaments that formed 10–15 s after the incision broke up in ∼20–40 ms (Figure 3G).
FIGURE 3. (A–G) Hemolymph dripping from the wound of a caterpillar within 10–15 s after wounding. A straight filament in (D) indicates that the hemolymph either increased its viscosity or became viscoelastic.
During the next 50–60 s (i.e., ∼75 s after incision), drop detachment followed the scenario shown in Figure 3 (Supplementary Video S1). However, the lifetime of these late filaments increased significantly by approximately one order of magnitude to ∼240 µs.
These results indicate that the properties of the hemolymph change quickly; the hemolymph starts dripping as an almost inviscid water-like fluid and then quickly changes its rheological properties. Typically, bleeding stopped after 60–90 s.
The scenario of wound sealing in the larvae of M. sexta was validated with that of the cockroach P. americana (Figure 4). Although the hemolymph composition of cockroaches could be somewhat different, both the insects have hemocyte-rich hemolymph (Chapman, 2013), and, as shown below, the plasma viscosity for both species is similar. After the detachment of an antennal flagellomere (i.e., an individual unit of the last antennal segment), a drop of hemolymph appeared at the cut end. The drop fell, and the remaining hemolymph moved back into the antennal lumen. No other drops appeared, and after approximately 10 min, the wound had sealed with a rigid crust.
FIGURE 4. Hemolymph dripping from the severed antenna of the cockroach Periplaneta americana. The head (not pictured) is to the left. (A) After cutting off the end of the antenna, the hemolymph continued flowing to this end. Over approximately 6 s, a drop formed. After t = 5.6 s, the drop fell, and no other drops appeared. (B) After ∼10 min, the wound was completely sealed by a rigid crust: a tungsten needle was not able to penetrate the crust. When the needle was applied (t = 0.6 s) to a cantilevered antenna, the antenna bent, tilting its end as shown in the figure. When the needle was removed (t = 5.5 s and t = 6.4 s), the antenna returned to its original state, and the sealed end appeared undeformed.
The almost constant radius of the cylindrical filaments indicated that the hemolymph had either thickened by increasing its shear viscosity or had become elastic (McKinley and Sridhar, 2002; Montanero and Ponce-Torres, 2020). In other words, one could think of the filaments as being similar to those either produced by thick honey-like fluids or thin dog saliva-like fluids. To obtain honey-like filaments, the shear viscosity of the hemolymph must increase significantly. We, therefore, measured the shear viscosity by magnetic rotational spectroscopy (MRS) with nickel (Ni) nanorods.
Ferromagnetic nickel nanorods coated with poly(vinylpyrrolidone) were dispersed in a hemolymph drop and were continuously rotated by applying a rotating magnetic field and changing their rotating frequency
FIGURE 5. (A) Nickel nanorods in a hemolymph drop from the larvae of M. sexta. The different nanorods were imaged using a ×10 Olympus BX51 objective. The nanorods were observed through the hemolymph–air interface, and the images were taken at (A)
When a nanorod rotates, it experiences resistance from the surrounding hemolymph. The MRS theory uses the Jeffery (Jeffery, 1922) model of rotation of an ellipsoid in a Newtonian fluid of viscosity
The nanorods were tracked using a camera (Figures 5A–C), and the videos were post-processed to fit the nanorod angle versus time, using this model. Two distinguishable scenarios of nanorod rotation are shown in Figures 5D, E.
FIGURE 6. (A) Angular dependence of
At low-driving frequencies,
This analysis of the shear viscosity suggests that the hemolymph dripping from the wound does not behave as a thick honey-like fluid. Furthermore, the hemocytes had no time to aggregate. Therefore, the appearance of cylindrical filaments when the hemolymph drips from the wound cannot be explained by the significant increase in its shear viscosity.
Thus, we hypothesized that as the first step in primary clot formation, the hemolymph has to be turned into a viscoelastic fluid.
To evaluate the rheological properties of hemolymph filaments, we used the setup shown in Figure 1B. The wound can be probed for only a few minutes after an incision. That is, the time for experimental preparation was long, and when we applied the probe, no dripping occurred. Thus, the wound was partially sealed by the primary clot. Upon placing the probe on the wound and lifting the probe (Figure 1B), we formed the first filament connecting the ball with the wound (Figure 7A). This filament was quite stable; despite the caterpillar’s movements, the meniscus that formed at the filament foot slid across the drop surface and did not break (Figures 7B, C). When the wounded proleg was moved up or down, the filament stretched or contracted, respectively (Figures 7E, H). The first filament took 1–20 s to become thin and break up (Figures 7I, J). We call these liquid bridges “long-lived filaments” (LLFs), indicating that their lifetime exceeded 1 s. These long-lived filaments sometimes developed single or multiple droplets, which remained on the filament until its breakup or moved to the ends. The droplet that moved to the wound was re-absorbed by the wound, and the droplet that moved to the ball was re-absorbed by the film on the ball.
FIGURE 7. (A–J) Series of frames illustrating the features of the formation and breakup of a long-lived filament (LLF). The filament formed between a stainless-steel probe (p) at the top and a wound of the M. sexta caterpillar (c) at the bottom. The camera was focused on the filament, and the wound was not visible. The caterpillar constantly moved, causing the filament to move.
When the probe was applied for the second time, the filament was less stable and broke in less than 1 s (Figure 8). We called these filaments “short-lived filaments” (SLFs). They behaved like those dripping from the wound (Figure 3).
FIGURE 8. (A–J) Series of frames illustrating the features of the formation and breakup of a short-lived filament (SLF); (p) is a stainless-steel probe, and (c) is the wound of the M. sexta caterpillar. It takes approximately 45 ms for the SLF to break up and disappear.
After the second trial, when the probe touched the wound and was then raised, the liquid bridges broke in less than 10 ms, following the breakup stages shown in Figure 2. These observations suggested that the hemolymph behaved as a simple low-viscosity Newtonian liquid, like water, that does not form cylindrical filaments (Eggers, 1997; Montanero and Ponce-Torres, 2020) (see an example in Supplementary Video S2).
Using short-lived filaments as representatives of those dripping from the wound and assuming that they would reflect the properties of the dripping filaments, we characterized their rheological properties. The rheological properties of the hemolymph were evaluated by tracking the radius of the short-lived filaments with time. By analyzing the kinetics of liquid-bridge thinning, we discovered that the bridge radius followed the exponential law
with relaxation time
FIGURE 9. (A) Relaxation time
To evaluate the cause of the distinct kinetics of the SLF and LLF thinning, we turned to the structural analysis of their composition. When we probed the wound with the ball in the first 3 min, long-lived filaments were formed 13 times, short-lived filaments were formed 18 times, and 22 trials produced no filaments. For wounds older than 8 min, we observed a long-lived filament only once, while short-lived filaments were observed 11 times, and 52 trials produced no filaments. The long-lived filaments did not necessarily follow the Oldroyd-B model or have a broad dispersion of the relaxation times ranging from milliseconds to seconds. This scattering of the material parameters led us to hypothesize that because of the caterpillar’s movements, the ball might have probed the hemolymph from different depths of ball immersion. We suspected that the ball forming the long-lived filaments might have probed the primary clot together with the layer of hemocytes covering the wound surface. Sometimes, the flowing hemocytes can be observed inside the LLF (Supplementary Video S4).
By collecting long-lived filaments on a glass slide and studying them using phase-contrast microscopy, we discovered filamentous cores made up of polymeric filaments with embedded hemocytes (Figures 9B–D). These filaments were long and bundled, even without hemocytes. When a long-lived filament was broken at one end, some droplets sometimes remained on a dried filament and formed a crusted surface, while the interior of the drop remained full of liquid hemolymph (Figure 9E).
These observations confirm that LLFs contain primary clots, and hence, their thinning kinetics is controlled by the deformation and flow of the highly inhomogeneous composite structure of primary clots. The more mature the primary clot is, the slimier it is, and the slower the filament breaks up.
To further investigate the phenomenon of crust formation, we examined the behavior of hemolymph drops using X-ray micro-CT and SEM imaging. X-ray imaging is attractive because it distinguishes between the structure of the multiphase and multicomponent materials (Andrews et al., 2011; Maire and Withers, 2014). Before submitting the hemolymph drop for X-ray imaging, we rapidly froze the drop on a metal substrate and slowly sublimated the water (as explained in Materials and methods). Then, a freeze-dried drop was imaged using an X-ray microscope (Bruker SkyScan 1176). The images were reconstructed (Figures 10A, B), and we schematically show how the cross-sections parallel and perpendicular to the substrate were taken (Figure 10C). These images show the density distribution of primary aggregates or clots through the drop thickness. The material closer to the air/hemolymph and hemolymph/substrate interfaces had a greater density.
FIGURE 10. Micro-CT scans of a freeze-dried hemolymph drop. Yellow represents a high-density material, and purple represents a low-density material. (A) Series of drop cross-sections was taken perpendicular to the substrate, as specified in (C). The cross-sectional area increased from the drop edge to the center. (B) Series of drop cross-sections was taken parallel to the substrate, as specified in (C). The cross-sectional area decreased from the substrate to the droplet top. The interfacial layer of the hemolymph appears to have a high-density material. (C) Diagram depicting the sample preparation procedure and the locations of the four parallel and perpendicular sections. (D) SEM micrographs of hemolymph residues attached to the substrate. Small dark spots form either chain-like or solitary aggregates. (E) Solitary aggregates appeared in the framed area in (D). (F) Filamentary residues sticking out from the aggregate are also common for larger aggregates.
To evaluate the microstructure of this interfacial layer, the frozen drop was broken and removed from the substrate. The remaining material that adhered to the substrate was examined by scanning electron microscopy. Figures 10D–F show residues on the substrate from the droplet sitting on it for longer than 20 min before freezing. Chain-like aggregates are observed attached to the substrate. These aggregates are much larger than the individual hemocytes, suggesting that the hemocytes were already assembled into chain-like primary clots. The possible building blocks of these chain-like aggregates are shown in Figure 10E, where solitary aggregates of approximately 30
All these findings favor the hypothesis that in larval Lepidoptera, the primary clots first nucleate at the interfaces and form a crust-like shell preventing water evaporation. The shell propagates inward to occupy the entire drop.
Hemocytes interact with one another closer to the surface. It has long been hypothesized that some hemocytes extrude thread-like projections (i.e., pseudopodia) in response to foreign surfaces; then, other hemocytes adhere to the formed network (Salt, 1970; Gregoire and Rockstein, 1974). This hypothesis implicitly assumes that the hemocyte density is high enough to allow the connection of two neighboring hemocytes by pseudopodia (Wigglesworth, 1937; Salt, 1970).
We investigated this hypothesis by observing the hemocyte behavior in the droplets released from the wound. Within approximately 3 min from the moment of drop formation, the hemocytes from the larvae of M. sexta began adhering to each other. When a magnetic nanorod was introduced to the drop, the pseudopodia anchored the nanorods to the hemocyte aggregates (Figure 5F); the nanorods never broke the hemocytes. In contrast to the free nanorods, the nanorods attached to hemocyte aggregates did not make full revolutions (Aprelev et al., 2019). Instead, they beat in response to the applied rotating field around an equilibrium direction, revealing hidden interconnections between hemocytes (Supplementary Video S3). During these oscillations, the surrounding cells moved in unison with the nanorod. Thus, the hemocytes with the attached nanorods mechanically communicated with the neighboring hemocytes that were not visibly connected to one another. A hidden biopolymer network provides a far-field reaction to a local perturbation, engaging hemocytes in the movement. This local perturbation of an oscillating nanorod propagates through an area with a radius of ∼5–8 nanorod lengths from the probing nanorod (Figure 11). The analysis of nanorod oscillations in these aggregates suggested that the aggregates with a hidden network that connects them behaved as a viscoelastic material with temporary cross-links (Aprelev et al., 2019), as described by the Green–Tobolsky theory of gels (Green and Tobolsky, 1946).
FIGURE 11. (A) Image of a nanorod surrounded by a network of cells. The encircled hemocytes are engaged in the movement of the nanorod. As the nanorod attempts to rotate, the hemocytes inside a circled aggregate move together. The hemocytes outside the circle do not respond to the motion of the nanoprobe, indicating that the hemocytes form an aggregate shielding a foreign object from the surrounding hemocytes. (B) Phase-contrast image of a growing pseudopodium (arrows). After ∼180 s, the two hemocytes become connected through a pseudopodial bridge [adapted from Aprelev et al., 2019].
The high density of hemocytes can only be expected in the neighborhood of the wounded tissue; otherwise, the hemocyte density in a healthy caterpillar is insufficient for hemocyte bridging. We illustrated this statement by showing that after ∼3 min of being close to one another in a large population of hemocytes, only two of them were bridged by a pseudopodium; the other hemocytes remained mobile (Figure 11B).
An illustrative scenario of hemocytes gathering in a primary clot during the first 10 min of hemolymph coagulation is presented in Figure 12 (Aprelev et al., 2019). A pseudopodium extends from the originating mother hemocyte, stretches further, and bends on its way, targeting another hemocyte, to which it later adheres. The pseudopodium functions as a hydraulic spring; on its way to finding the target, it stretches the constituent material. After contact, the pseudopodium tends to spread along the hemocyte surface. The tension generated in the hemocyte during stretching tends to relax, squeezing the constituent material back to the mother hemocyte and dragging the targeted hemocyte with it. As the pseudopodium contracts toward the mother hemocyte, it may encounter another hemocyte, attach to it, and bring it to the mother hemocyte. The process repeats, and the aggregates grow larger and change their shape, leading to the formation of a primary clot (Salt, 1970; Gregoire and Rockstein, 1974; Aprelev et al., 2019).
FIGURE 12. Gallery of time-lapse frames, depicting a four-hemocyte aggregate in a primary clot of the hemolymph of a larva of M. sexta. The pseudopodia are formed by hemocytes first deforming the interface in finger-like extensions. Each extension could deform and propagate over long distances searching for a target. The hemocytes are artificially colored in red, yellow, purple, and pink, and the aggregate-involved pseudopodia are in blue. (A) Initially, the red and yellow hemocytes touch weakly, and the pink hemocyte is free near the extending pseudopodia. (B,C) With time, the interface between the red and yellow hemocytes increases, while the pseudopodium attaches to the pink hemocyte and drags it to the right. (D) The purple hemocyte, which is attached to both red and pink hemocytes, comes into focus, while the pseudopodium continues to drag the pink hemocyte to the right. (E) The red/purple and the pink/purple interfaces grow, and the pink hemocyte, which is half-engulfed in pseudopodia, first touches the red hemocyte. (F) The pink hemocyte disconnects from the pseudopodia and attaches to the red and yellow hemocytes. (G) The red and pink hemocytes disconnect, and the four-hemocyte aggregate forms a hole in the center. (H) The purple and yellow hemocytes form an interface, and the four hemocytes remain in this configuration until the end of the observation [images were extracted from the video in the study by Aprelev et al., 2019].
The mechanism of hemocyte adhesion by pseudopodia can be understood from the analysis of the strength of adhesive bonds between hemocytes in the aggregate. The interfacial tensions and the associated interfacial energies of films separating the adjacent hemocytes offer quantitative metrics for the analysis of aggregation phenomena (Figure 13). These interfacial tensions control the opening angle θ, introduced as the angle of the wedge that the two hemocyte interfaces form at contact (Figure 13D). We distinguish the wedges filled with different media of interest. At least three types of wedges could be formed by the two hemocyte interfaces in the primary aggregate when hemocytes contacted each other (Figures 13A–C). When the wedge is filled with the hemocyte interior, and two interfaces in the wedge separate three different hemocytes, the wedge angle is given as θ = α. When the wedge is filled with plasma, and two interfaces separate hemocytes from the plasma, the wedge angle is given as θ = β. When the wedge is filled with pseudopodia, and two interfaces separate hemocytes from the pseudopodia, the wedge angle is given as θ = γ.
FIGURE 13. Opening angle of the wedge formed by two hemocyte interfaces in different environments. (A) When three hemocytes reside in a primary clot and their interfaces form a wedge of angle α filled with the hemocyte interior; (B) when two hemocyte interfaces form a wedge of angle β filled with plasma; and (C) when two hemocyte interfaces form a wedge of angle γ filled with pseudopodia. (D) Free-body diagram for the interfacial tensions acting at the vertex. The associated tensions, the opening angle θ, and the complementary angle ϕ are shown in different colors. (E) Bar graph of relative interfacial energies calculated using Eq. 3, where asterisks correspond as follows: *p <0.05 and **p <0.0001. The number of measurements for each scenario is nα = 12, nβ = 14, and nγ = 7.
We introduce the interfacial tension
Balancing the x-components of forces, we obtain
The measured angle α = 120° ± 5° is close to α = 120°, as predicted by Plateau’s law (Plateau, 1873) for the bubble and droplet aggregates with the same interfacial tension
Using the measured angles α = 120° ± 5°, β = 70° ± 11°, and γ = 113° ± 6°, the relative interfacial energies of hemocytes in a primary clot (
This analysis emphasizes the importance of interfacial forces in gathering and holding hemocytes together during the development of primary clots.
In recent years, the fruitful analogy between the cell aggregates of developing tissues and liquids with a viscoelastic rheology has offered new perspectives in biological soft matter (Steinberg, 2007; Janmey et al., 2009; Gonzalez-Rodriguez et al., 2012; Foty and Steinberg, 2013; Beaune et al., 2014; Beris et al., 2021; Patteson et al., 2022). Although the mainstream research in this direction is focused on vertebrate and human blood, little is known about the material properties of insect blood. We, therefore, investigated the hemolymph clotting phenomena using materials science approaches. The main question that we asked was, “What are the material determinants of fast wound sealing in insects?” By evaluating the rheological properties of the hemolymph over short periods of time, we investigated the mechanisms leading to the arrest of bleeding and to the nucleation and formation of primary clots in insects with hemocyte-rich hemolymph.
Our experiments were triggered by observations of the hemolymph extracted directly from a wound. When a metal pin is dipped in a hemolymph drop with primary clots and then immediately pulled back, one observes a liquid filament bridging the droplet surface using the pin. The hemolymph filament does not break as quickly as a water bridge formed with the same pin drawn from a water droplet. This feature of hemolymph stringing (Gregoire, 1955; Chino et al., 1987; Scherfer et al., 2004; Aprelev et al., 2019) is the first indication of clotting. It can be used for screening different clot inhibitors or promoters, as demonstrated in the hemolymph of fruit fly larvae (D. melanogaster) (Bidla et al., 2005; Lesch and Theopold, 2008). Bidla et al. (2005) and Lesch and Theopold (2008) studied the effect of the protein phenoloxidase (PO) on clot tenacity by measuring the maximum pull-out length of the filament in the hemolymph droplets with and without PO. This protein is thought to facilitate the cross-linking and hardening of the primary clot. One would expect that the greater the PO concentration, the harder the clot, and the shorter the pull-out length of the filament before it breaks. This prediction is correct (Bidla et al., 2005; Lesch and Theopold, 2008). Although these observations are useful, no quantitative data on the material properties of the hemolymph have been provided. The most challenging biological question regarding the mechanism of fast wound sealing remain enigmatic.
We found that the larvae of M. sexta, which have hemocyte-rich hemolymph, stopped bleeding after 60–90 s. At this time scale, the hemolymph viscosity is low (Table 1), and high-speed videography is needed to film the filament breakup (Figures 2, 3, 8). The appearance of a long cylindrical filament in the dripping hemolymph points to its possible viscoelasticity (Macosko, 1994; Larson, 1999). Many low-shear viscosity biofluids demonstrate viscoelasticity (Bazilevsky et al., 2011; Haward et al., 2011). The hemolymph could behave like saliva dripping from a dog’s mouth, with water-level low-shear viscosity, which would still be capable of producing long filaments. Making an aqueous fluid elastic allows an animal to stabilize the flow of blood through the circulatory system or prevent the breakup of liquid bodies, as in the case of dripping hemolymph.
The kinetics of the breakup of a cylindrical filament allows one to evaluate the rheological properties of fluids and distinguish between the specifics of the viscoelastic non-Newtonian behavior of the material (Bazilevsky et al., 1990; Entov and Hinch, 1997; McKinley and Sridhar, 2002). We, therefore, analyzed the kinetics of the filament breakup and observed that before the arrest of bleeding, the hemolymph became viscoelastic with relaxation time
Knowing the relaxation time and shear viscosity (Table 1),
Typically, hemolymph plasma contains 0.01–0.1
Even after the arrest of bleeding, wound closure must be fast to prevent water and solute loss, maintain hydrostatic pressure, and form a barrier against infection. At this step, the viscoelasticity of the low-viscosity hemolymph does not help much, and the insect develops a new strategy by forming a clot that spreads to form a stiff wound seal. The hemocyte-rich hemolymph contains adhesive and non-adhesive hemocytes (Salt, 1970; Lavine and Strand, 2002; Ribeiro and Brehélin, 2006). One scenario of clot nucleation assumes that adhesive cells aggregate at the wound surface or any other surface (Gregoire, 1953; Lai-Fook, 1966; Wigglesworth and Gupta, 1979; Brodland, 2002; Steinberg, 2007; Gonzalez-Rodriguez et al., 2012; Mattix et al., 2014) and are involved in wound healing, phagocytosis (Gregoire, 1955; Salt, 1970; Arnold and Rockstein, 1974; Rowley and Ratcliffe, 1976; Pech and Strand, 1996; Gillespie et al., 1997; Pech and Strand, 2000; Lavine and Strand, 2002; Ribeiro and Brehélin, 2006), and the regrowth of epidermal cells (Wigglesworth, 1937; Godt and Tepass, 1998; Hayashi and Carthew, 2004; Carthew, 2005; Bulgakova et al., 2012). In this scenario, primary clots are formed at the wound surface or any other foreign surface. We showed that in the larvae of M. sexta, primary clots are formed at the interfaces (Figure 10), which propagate from the damaged surface toward the hemolymph interior. This scenario has also been observed for other insects (Gregoire, 1955; Salt, 1970; Wigglesworth and Gupta, 1979; Theopold et al., 2004).
Hemocytes spread over and embed in a fibrous network of primary clots, as shown by the analysis of the core of long-lived filaments (Figure 9). The importance of interfacial forces in gathering and holding hemocytes together during the development of primary clots is shown in Figures 11, 12 and by the theoretical analysis in Section 2.5.
The Ni-nanorod experiments and phase-contrast imaging of these primary clots showed that fibrous connectors are essential for holding hemocytes inside the aggregate and to form connections between the aggregates. We showed that approximately 90% of the adhesion energy between hemocytes comes from the surrounding fibrous bed. To permanently lock the hemocytes in the aggregate, only ∼10% of it needs to be added to the adhesion energy. These additional bonds are formed later in clot development during the consolidation of the aggregates (Aprelev et al., 2019).
In summary, we observed that insects with hemocyte-rich hemolymph develop a two-step strategy to form clots. In the first step, the Newtonian plasma of the hemolymph turns into a non-Newtonian viscoelastic fluid, remaining at very-low viscosity. This transformation allows the retraction of the dripping hemolymph to the wound. In the second step, primary clots nucleate and form a crust at the air–hemolymph and hemolymph–substrate interfaces. The clots propagate from the interface toward the droplet center.
Given the adaptive value of viscous-to-viscoelastic fluid conversion, we expect this physical phenomenon to be widespread in insects and other organisms with hemocyte-rich hemolymph. In addition to insects, for example, hemocyte-rich fluids are present in some mollusks (e.g., snails), where they promote wound healing (Machalowski and Jesionowski, 2021). As the hemocyte density changes with age and the infection level in some insects (Stoepler et al., 2013), we also expect the physical behavior of the hemolymph to change. Intriguing experimental subjects include insects that partition hemocyte-rich and hemocyte-poor hemolymph in the same body. The larvae of at least some mosquito species, for instance, are hemocyte-rich at specific body sites, such as the openings into the heart, where they rapidly mobilize to fight infection (League and Hillyer, 2016).
The period from the initiation of the immune cascade reaction to the first microscopically visual detection of a rheological response of the hemolymph remains the unquantified bridge between molecular biology and materials science. Since the last century (Gregoire, 1953), biologists have known that vertebrate red blood cells can initiate an immune cascade reaction in the hemolymph of insects. The material manifestation of viscous-to-viscoelastic conversion of insect hemolymph in a few seconds is attractive for biomedical applications. We hope that our findings will trigger the interest of biochemists and molecular biologists to design fast-working thickeners for vertebrate blood, including human blood.
The larvae of M. sexta were obtained from Carolina Biological Supply (https://www.carolina.com) or were reared in-house on food from Great Lakes Hornworm (https://www.greatlakeshornworm.com/), with some feeding ad libitum on hornworm food obtained from Carolina Biological Supply. The deposited eggs were obtained from adults that emerged in a net enclosure (ca. 27°C and relative humidity ca. 65%). Rearing containers were wide-mouthed 1-L glass jars with strips (ca. 3 × 15 cm) of plastic gutter guard (Frost King Model VX620) as a climbing substrate and food support. Larvae were maintained at controlled room temperature (approximately 25°C) and 24 h of artificial light. To provide gas exchange, the jar lids were replaced with aluminum window screens. Food (ca. 10 mL) was added in the first three instars as needed. In later instars, larvae were removed from jars and placed in clean jars with more food added as needed. The number of larvae per jar was reduced as they grew, with 10 or fewer per jar in the last instars. Larvae entering pre-pupation (i.e., those with a more yellowish thorax, no longer eating, and in a wandering phase) were not used as a source of hemolymph.
The larvae of M. sexta, which were 1–2 days before pre-pupation and weighed more than 8.5 g, were used. The caterpillars were washed free of contaminants with DI water and dried using a paper towel. To minimize the movement of larvae, they were placed in specially designed containers (Figure 1) that gripped the larva along the length of the body while exposing the second and third prolegs. Hemolymph was extracted via a 1–2-mm incision made using a razor blade in the third proleg. The hemolymph exiting the wound was collected on a glass slide or probed directly on the body. All experiments were conducted at 20°C–22°C.
To investigate the LLF structure, a liquid bridge was created between a hemolymph drop and the metal ball (Figure 1C). A glass slide was placed next to the ball perpendicular to the drop surface so that the slide touched the ball’s side. Pulling the ball parallel to the glass slide above the slide edge stretched the liquid bridge and formed a filament. By moving the ball with the filament toward the glass surface, we deposited the filament on the slide. The deposited filament was covered with a cover slip to prevent evaporation during phase-contrast imaging.
To study cell aggregation, drops of blood were placed under an inverted transmitted-light phase-enhanced microscope (Nikon Eclipse Ti; ×40 Oil Objective, S Fluor, NA = 1.30, DIC H/N2; Photometrics CoolSNAP HQ2 camera, 272-ms exposure, time interval = 1 s), and the cell behavior was recorded. Cellular aggregation during the first 10 min of coagulation was observed under high magnification.
A drop of hemolymph was rapidly frozen on a metal block in liquid nitrogen, freeze-dried under vacuum, detached from the metal block, mounted on a low-density Styrofoam block, and imaged using X-ray micro-CT (Bruker SkyScan 1176). The image was digitally reconstructed, and the cross-sections were studied. The methodology can be summarized as follows: an X-ray source in tandem with a detector was rotated around the sample, and projection images of the sample were taken. The images were digitally reconstructed into a three-dimensional structure. The cross-sections of the three-dimensional structure could then be visualized. The resolution of the instrument was 9 μm per pixel, which was inadequate to observe the internal structure of the freeze-dried clots but was sufficient to determine the internal distribution of the material density.
Nickel nanorods of diameter
The SLF and LLF breakup was filmed using an IDT Technologies MotionPro X3 camera at 200–900 fps with a resolution of 512–640 pixels and a Grasshopper Point Gray camera at 100–140 fps with a resolution of 1,920–1,200 pixels. The time dependence of the filament radius was obtained with a specially developed LabVIEW Vision Development Module that allowed us to extract and analyze the profile of the liquid bridge for each frame of the videos (Sun et al., 2022; Sun et al., 2023). The module used the IMAQ Extract Contour algorithm to provide the filament profile. Then, the radius of the cylindrical neck of the filament was fit by Eq. 1, and the goodness of fit was evaluated as discussed by Sun et al. (2022) and Sun et al. (2023). The procedure was repeated for each filament, and the average relaxation time for the series was reported.
The LLF hemolymph puddles from 10 caterpillars were tested using the setup shown in Figure 1C. The method required approximately 1.0–1.5 min to collect the hemolymph from the bleeding caterpillar and to prepare the experimental setup. Liquid bridges were formed from the same
The original contributions presented in the study are included in the article/Supplementary Material; further inquiries can be directed to the corresponding author.
Ethical approval was not required for the study involving animals in accordance with the local legislation and institutional requirements because research on insect blood does not require approval.
PaA: data curation, formal analysis, investigation, methodology, software, validation, visualization, and writing–review and editing. AB: data curation, formal analysis, investigation, software, validation, visualization, and writing–review and editing. TB: visualization and writing–review and editing. CB: investigation, resources, and writing–review and editing. PeA: formal analysis, funding acquisition, investigation, supervision, and writing–review and editing. KK: conceptualization, formal analysis, funding acquisition, investigation, methodology, project administration, resources, writing–original draft, and writing–review and editing.
The author(s) declare that financial support was received for the research, authorship, and/or publication of this article. This work was partially supported by the NSF grant IOS 2014664, by the Clemson University Creative Inquiry project to KK, and by the SC BioCRAFT facilities supported by the National Institute of General Medical Sciences (NIGMS) of the National Institutes of Health under award number P30GM131959 through the voucher program to KK. The work of PeA was also partially supported by the NIFA/USDA, under project number SC-1700527, with technical contribution no. 7237 of the Clemson University Experiment Station. The work of PaA was partly supported by NASA through the SC Space Grant Consortium Graduate Assistantship, NASA grant: NNX15AL49H.
The authors thank Guzeliya Korneva and Bryan Wiggers for helping with the optical imaging and characterization of hemolymph properties and Ahva Zadeh for helping them collect the MRS data on the hemolymph of cockroaches.
The authors declare that the research was conducted in the absence of any commercial or financial relationships that could be construed as a potential conflict of interest.
All claims expressed in this article are solely those of the authors and do not necessarily represent those of their affiliated organizations, or those of the publisher, the editors, and the reviewers. Any product that may be evaluated in this article, or claim that may be made by its manufacturer, is not guaranteed or endorsed by the publisher.
The Supplementary Material for this article can be found online at: https://www.frontiersin.org/articles/10.3389/frsfm.2024.1341129/full#supplementary-material
Andrews, J. C., Meirer, F., Liu, Y. J., Mester, Z., and Pianetta, P. (2011). Transmission X-ray microscopy for full-field nano imaging of biomaterials. Microsc. Res. Tech. 74 (7), 671–681. PubMed PMID: WOS:000292570900011. doi:10.1002/jemt.20907
Aprelev, P., Bruce, T. F., Beard, C. E., Adler, P. H., and Kornev, K. G. (2019). Nucleation and formation of a primary clot in insect blood. Sci. Rep. 9, 3451. doi:10.1038/s41598-019-40129-0
Aprelev, P., McKinney, B., Walls, C., and Kornev, K. G. (2017). Magnetic stage with environmental control for optical microscopy and high-speed nano- and microrheology. Phys. Fluids 29 (7), 072001. PubMed PMID: WOS:000406765200003. doi:10.1063/1.4989548
Arakawa, T., Kato, Y., Hattori, M., and Yamakawa, M. (1996). Lipophorin: a carrier for lipids in insects participates in superoxide production in the haemolymph plasma. Insect Biochem. Mol. Biol. 26 (4), 403–409. PubMed PMID: WOS:A1996UQ42100011. doi:10.1016/0965-1748(95)00110-7
Arnold, J. W. (1974). “The hemocytes of insects,” in The physiology of insecta 5 Editor M Rockstein. 2nd ed. (New York: Academic Press), 201–254.
Bazilevskii, A. V., Entov, V. M., and Rozhkov, A. N. (2001). Breakup of an Oldroyd liquid bridge as a method for testing the rheological properties of polymer solutions. Polym. Sci. Ser. A 43 (7), 716–726. PubMed PMID: WOS:000170282300008.
Bazilevskii, A. V., Meyer, J. D., and Rozhkov, A. N. (2005). Dynamics and breakup of pulse microjets of polymeric liquids. Fluid Dyn. 40 (3), 376–392. PubMed PMID: WOS:000207870400005. doi:10.1007/s10697-005-0078-4
Bazilevsky, A. V., Entov, V. M., and Rozhkov, A. N. (1990). Liquid filament microrheometer and some of its applications (Edinburgh, UK: The Golden Jubilee Meeting of the British Society of Rheology and Third European Rheology Conference).
Bazilevsky, A. V., Entov, V. M., and Rozhkov, A. N. (2011). Breakup of a liquid bridge as a method of rheological testing of biological fluids. Fluid Dyn. 46 (4), 613–622. PubMed PMID: WOS:000294174000011. doi:10.1134/s0015462811040119
Beaune, G., Stirbat, T. V., Khalifat, N., Cochet-Escartin, O., Garcia, S., Gurchenkov, V. V., et al. (2014). How cells flow in the spreading of cellular aggregates. Proc. Natl. Acad. Sci. U. S. A. 111 (22), 8055–8060. PubMed PMID: WOS:000336687900051. doi:10.1073/pnas.1323788111
Beris, A. N., Horner, J. S., Jariwala, S., Armstrong, M. J., and Wagner, N. J. (2021). Recent advances in blood rheology: a review. Soft Matter 17 (47), 10591–10613. PubMed PMID: WOS:000719451600001. doi:10.1039/d1sm01212f
Bidla, G., Lindgren, M., Theopold, U., and Dushay, M. S. (2005). Hemolymph coagulation and phenoloxidase in larvae. Dev. Comp. Immunol. 29, 669–679. PubMed PMID: 15854679. doi:10.1016/j.dci.2004.11.007
Bohn, H. (1986). “Hemolymph clotting in insects,” in Immunity in invertebrates, cells, molecules and defense reactions. Editor M. Brehelin (Heidelberg: Springer), 188–207.
Boman, H. G., and Hultmark, D. (1987). Cell-free immunity in insects. Annu. Rev. Microbiol. 41, 103–126. PubMed PMID: WOS:A1987K194900005. doi:10.1146/annurev.mi.41.100187.000535
Brasovs, A., Palaoro, A. V., Aprelev, P., Beard, C. E., Adler, P. H., and Kornev, K. G. (2023). Haemolymph viscosity in hawkmoths and its implications for hovering flight. Proc. R. Soc. B 290, 20222185. doi:10.1098/rspb.2022.2185
Brehelin, M. (1979). Hemolymph coagulation in Locusta-migratoria - evidence for a functional equivalent of fibrinogen. Comp. Biochem. Physiol. B-Biochem Mol. Biol. 62 (4), 329–334. PubMed PMID: WOS:A1979GS50300007. doi:10.1016/0305-0491(79)90098-1
Brodland, G. W. (2002). The differential interfacial tension hypothesis (DITH): a comprehensive theory for the self-rearrangement of embryonic cells and tissues. J. Biomech. Eng-Trans ASME. 124 (2), 188–197. PubMed PMID: WOS:000175343800006. doi:10.1115/1.1449491
Bulgakova, N. A., Klapholz, B., and Brown, N. H. (2012). Cell adhesion in Drosophila: versatility of cadherin and integrin complexes during development. Curr. Opin. Cell Biol. 24 (5), 702–712. PubMed PMID: WOS:000310943300019. doi:10.1016/j.ceb.2012.07.006
Carthew, R. W. (2005). Adhesion proteins and the control of cell shape. Curr. Opin. Genet. Dev. 15 (4), 358–363. PubMed PMID: WOS:000231205400002. doi:10.1016/j.gde.2005.06.002
Chapman, R. F. (2013). The insects: structure and function. Cambridge, UK: Cambridge University Press.
Chino, H., Hirayama, Y., Kiyomoto, Y., Downer, R. G. H., and Takahashi, K. (1987). Spontaneous aggregation of locust lipophorin during hemolymph collection. Insect Biochem. 17 (1), 89–97. PubMed PMID: WOS:A1987E892900013. doi:10.1016/0020-1790(87)90148-x
Coodin, S., and Caveney, S. (1992). Lipophorin inhibits the adhesion of cockroach (Periplaneta americana) hemocytes in vitro. J. Insect Physiology 38 (11), 853–862. PubMed PMID: WOS:A1992JZ89400005. doi:10.1016/0022-1910(92)90096-v
Dushay, M. S. (2009). Insect hemolymph clotting. Cell. Mol. Life Sci. 66, 2643–2650. PubMed PMID: 19418022. doi:10.1007/s00018-009-0036-0
Eggers, J. (1997). Nonlinear dynamics and breakup of free-surface flows. Rev. Mod. Phys. 69 (3), 865–930. PubMed PMID: WOS:A1997XL66500009. doi:10.1103/RevModPhys.69.865
Eleftherianos, I., Heryanto, C., Bassal, T., Zhang, W., Tettamanti, G., and Mohamed, A. (2021). Haemocyte-mediated immunity in insects: cells, processes and associated components in the fight against pathogens and parasites. Immunology 164 (3), 401–432. PubMed PMID: WOS:000679933500001. doi:10.1111/imm.13390
Entov, V. M., and Hinch, E. J. (1997). Effect of a spectrum of relaxation times on the capillary thinning of a filament of elastic liquid. J. Newt. Fluid Mech. 72 (1), 31–53. PubMed PMID: WOS:A1997XP64400002. doi:10.1016/s0377-0257(97)00022-0
Erickson, H. P. (2009). Size and shape of protein molecules at the nanometer level determined by sedimentation, gel filtration, and electron microscopy. Biol. Proced. Online 11, 32–51. PubMed PMID: 19495910. doi:10.1007/s12575-009-9008-x
Foty, R. A., and Steinberg, M. S. (2013). Differential adhesion in model systems. Wires Dev. Biol. 2 (5), 631–645. PubMed PMID: WOS:000323306600004. doi:10.1002/wdev.104
Geng, C. (1990). Studies of hemolymph coagulation in Manduca sexta. Ph.D. dissertation. West Lafayette, IN: Purdue University Proquest Dissertation Publishers.
Geng, C. X., and Dunn, P. E. (1988). Hemostasis in larvae of Manduca sexta: formation of a fibrous coagulum by hemolymph proteins. Biochem. Biophys. Res. Commun. 155 (2), 1060–1065. PubMed PMID: WOS:A1988Q050400077. doi:10.1016/s0006-291x(88)80604-1
Gillespie, J. P., Kanost, M. R., and Trenczek, T. (1997). Biological mediators of insect immunity. Annu. Rev. Entomology 42, 611–643. PubMed PMID: WOS:A1997WD49500025. doi:10.1146/annurev.ento.42.1.611
Godt, D., and Tepass, U. (1998). Drosophila oocyte localization is mediated by differential cadherin-based adhesion. Nature 395 (6700), 387–391. PubMed PMID: WOS:000076083800055. doi:10.1038/26493
Gonzalez-Rodriguez, D., Guevorkian, K., Douezan, S., and Brochard-Wyart, F. (2012). Soft matter models of developing tissues and tumors. Science 338 (6109), 910–917. PubMed PMID: WOS:000311083600035. doi:10.1126/science.1226418
Green, S. M., and Tobolsky, A. V. (1946). A new approach to the theory of relaxing polymeric media. J. Chem. Phys. 14, 80–92. doi:10.1063/1.1724109
Gregoire, C. (1974). “Hemolymph coagulation,” in The physiology of insecta. 5. Editor M. Rockstein (New York: Academic Press), 309–360.
Gregoire, C. H. (1953). Blood coagulation in Arthropods. HI. Reactions of insect hemolymph to coagulation inhibitors of vertebrate blood. Biol. Bull. 104 (3), 372–393. PubMed PMID: BCI:BCI19532700027216. doi:10.2307/1538491
Gregoire, C. H. (1955). Blood coagulation in arthropods. V. Studies on hemolymph coagulation in 420 species of insects. Arch. Biol. 66 (1), 103–148. PubMed PMID: BCI:BCI19563000004464.
Gu, Y., and Kornev, K. G. (2016). Ferromagnetic nanorods in applications to control of the in-plane anisotropy of composite films and for in situ characterization of the film rheology. Adv. Funct. Mater. 26 (22), 3796–3808. PubMed PMID: WOS:000379128700004. doi:10.1002/adfm.201504205
Haward, S. J., Sharma, V., and Odell, J. A. (2011). Extensional opto-rheometry with biofluids and ultra-dilute polymer solutions. Soft Matter 7 (21), 9908–9921. PubMed PMID: WOS:000296026700017. doi:10.1039/c1sm05493g
Hayashi, T., and Carthew, R. W. (2004). Surface mechanics mediate pattern formation in the developing retina. Nature 431 (7009), 647–652. PubMed PMID: WOS:000224299300030. doi:10.1038/nature02952
He, Y., Cao, X. L., Zhang, S. G., Rogers, J., Hartson, S., and Jiang, H. B. (2016). Changes in the plasma proteome of Manduca sexta larvae in relation to the transcriptome variations after an immune challenge: evidence for high molecular weight immune complex formation. Mol. Cell. Proteomics 15 (4), 1176–1187. PubMed PMID: WOS:000373992600001. doi:10.1074/mcp.M115.054296
Hillyer, J. F., and Strand, M. R. (2014). Mosquito hemocyte-mediated immune responses. Curr. Opin. Insect Sci. 3, 14–21. PubMed PMID: WOS:000209578700004. doi:10.1016/j.cois.2014.07.002
Hoath, S. D., Vadillo, D. C., Harlen, O. G., McIlroy, C., Morrison, N. F., Hsiao, W. K., et al. (2014). Inkjet printing of weakly elastic polymer solutions. J. Newt. Fluid Mech. 205, 1–10. PubMed PMID: WOS:000333857000001. doi:10.1016/j.jnnfm.2014.01.002
Hu, H. W., Hu, Q. B., Weng, Q. F., and Wang, J. J. (2024). Hemocytin, the special aggregation factor connecting insect hemolymph immunity, a potential target of insecticidal immunosuppresant. Pesticide Biochem. Physiology 198, 105704. PubMed PMID: WOS:001135149200001. doi:10.1016/j.pestbp.2023.105704
Janmey, P. A., Winer, J. P., and Weisel, J. W. (2009). Fibrin gels and their clinical and bioengineering applications. J. R. Soc. Interface 6 (30), 1–10. PubMed PMID: WOS:000262756800001. doi:10.1098/rsif.2008.0327
Jeffery, G. B. (1922). The motion of ellipsoidal particles in a viscous fluid. Proc. R. Soc. Lond. Ser. a-Containing Pap. a Math. Phys. Character 102 (715), 161–179. PubMed PMID: WOS:000202801300004. doi:10.1098/rspa.1922.0078
Jiang, H., Vilcinskas, A., and Kanost, M. R. (2010). “Immunity in Lepidopteran insects,” in Invertebrate immunity. Advances in experimental medicine and biology. 708. Editor K. Soderhall (Berlin: Springer-Verlag Berlin), 181–204.
Kenny, M. C., Giarra, M. N., Granata, E., and Socha, J. J. (2018). How temperature influences the viscosity of hornworm hemolymph. J. Exp. Biol. 221 (21), jeb186338. PubMed PMID: WOS:000449824800016. doi:10.1242/jeb.186338
Kojic, N., Bico, J., Clasen, C., and McKinley, G. H. (2006). Ex vivo rheology of spider silk. J. Exp. Biol. 209 (21), 4355–4362. PubMed PMID: WOS:000242132500027. doi:10.1242/jeb.02516
Kornev, K. G., Gu, Y., Aprelev, P., and Tokarev, A. (2016). “Magnetic rotational spectroscopy for probing rheology of nanoliter droplets and thin films,” in Springer book series on characterization tools for nanoscience and nanotechnology. 6. Editor CSSR Kumar (New York: Springer), 51–83.
Kornev, K. G., Neimark, A. V., and Rozhkov, A. N. (1999). Physical mechanisms of foam flow in porous media. Rheol. Ser. 8, 1151–1182. doi:10.1016/s0169-3107(99)80016-1
Lai-Fook, J. (1966). The repair of wounds in the integument of insects. J. insect physiology 12, 195–226. doi:10.1016/0022-1910(66)90136-3
Larson, R. G. (1999). The structure and rheology of complex fluids. New York: Oxford University Press, 663.
Lavine, M. D., and Strand, M. R. (2002). Insect hemocytes and their role in immunity. Insect Biochem. Mol. Biol. 32, 1295–1309. PubMed PMID: 12225920. doi:10.1016/s0965-1748(02)00092-9
League, G. P., and Hillyer, J. F. (2016). Functional integration of the circulatory, immune, and respiratory systems in mosquito larvae: pathogen killing in the hemocyte-rich tracheal tufts. Bmc Biol. 14, 78. PubMed PMID: WOS:000383839100001. doi:10.1186/s12915-016-0305-y
Lesch, C., and Theopold, U. (2008). “Methods to study hemolymph clotting in insects,” in Insect Immunology. Editor N. E. Beckage (Academic Press), 1–12.
Ling, S. J., Kaplan, D. L., and Buehler, M. J. (2018). Nanofibrils in nature and materials engineering. Nat. Rev. Mater 3 (4), 18016. PubMed PMID: WOS:000430173500002. doi:10.1038/natrevmats.2018.16
Machalowski, T., and Jesionowski, T. (2021). Hemolymph of molluscan origin: from biochemistry to modern biomaterials science. Appl. Phys. a-Materials Sci. Process. 127 (1), 3. PubMed PMID: WOS:000597637300001. doi:10.1007/s00339-020-04166-1
Maire, E., and Withers, P. J. (2014). Quantitative X-ray tomography. Int. Mater. Rev. 59 (1), 1–43. PubMed PMID: WOS:000330498000001. doi:10.1179/1743280413y.0000000023
Maravilla, E., Le, D. P., Tran, J. J., Chiu, M. H., Prenner, E. J., and Weers, P. M. M. (2020). Apolipophorin III interaction with phosphatidylglycerol and lipopolysaccharide: a potential mechanism for antimicrobial activity. Chem. Phys. Lipids 229, 104909. PubMed PMID: WOS:000531098600005. doi:10.1016/j.chemphyslip.2020.104909
Mattix, B., Olsen, T. R., Gu, Y., Casco, M., Herbst, A., Simionescu, D. T., et al. (2014). Biological magnetic cellular spheroids as building blocks for tissue engineering. Acta Biomater. 10 (2), 623–629. PubMed PMID: WOS:000330921700006. doi:10.1016/j.actbio.2013.10.021
McKinley, G. H., and Sridhar, T. (2002). Filament-stretching rheometry of complex fluids. Annu. Rev. Fluid Mech. 34, 375–415. PubMed PMID: WOS:000174038400017. doi:10.1146/annurev.fluid.34.083001.125207
Miserez, A., Yu, J., and Mohammadi, P. (2023). Protein-Based biological materials: molecular design and artificial production. Chem. Rev. 123, 2049–2111. PubMed PMID: WOS:000925857700001. doi:10.1021/acs.chemrev.2c00621
Montanero, J. M., and Ponce-Torres, A. (2020). Review on the dynamics of isothermal liquid bridges. Appl. Mech. Rev. 72 (1). PubMed PMID: WOS:000525416400003. doi:10.1115/1.4044467
Mulnix, A. B., and Dunn, P. E. (1995). “Molecular biology of immune response,” in Molecular model systems in the Lepidoptera. Editors M. R. Goldsmith, and A. S. Wilkins (Cambridge, UK: Cambridge University Press), 369–396.
Parle, E., Dirks, J. H., and Taylor, D. (2016). Bridging the gap: wound healing in insects restores mechanical strength by targeted cuticle deposition. J. R. Soc. Interface 13 (117), 20150984. PubMed PMID: WOS:000378311800003. doi:10.1098/rsif.2015.0984
Patteson, A. E., Asp, M. E., and Janmey, P. A. (2022). Materials science and mechanosensitivity of living matter. Appl. Phys. Rev. 9 (1), 011320. PubMed PMID: WOS:000783171000001. doi:10.1063/5.0071648
Pech, L. L., and Strand, M. R. (1996). Granular cells are required for encapsulation of foreign targets by insect haemocytes. J. Cell Sci. 109, 2053–2060. PubMed PMID: WOS:A1996VC21500009. doi:10.1242/jcs.109.8.2053
Pech, L. L., and Strand, M. R. (2000). Plasmatocytes from the moth Pseudoplusia includens induce apoptosis of granular cells. J. Insect Physiology 46 (12), 1565–1573. PubMed PMID: WOS:000090079400007. doi:10.1016/s0022-1910(00)00083-4
Plateau, J. (1873). Statique Experimentale et The´orique des Liquides Soumis aux Seules Forces Mole´culaires. Paris: Gauthier-Villars.
Rahman, M. M., Ma, G., Roberts, H. L. S., and Schmidt, O. (2006). Cell-free immune reactions in insects. J. Insect Physiology 52 (7), 754–762. PubMed PMID: WOS:000239081500012. doi:10.1016/j.jinsphys.2006.04.003
Ribeiro, C., and Brehélin, M. (2006). Insect haemocytes: what type of cell is that? J. Insect Physiology 52, 417–429. PubMed PMID: 16527302. doi:10.1016/j.jinsphys.2006.01.005
Rodd, L. E., Scott, T. P., Cooper-White, J. J., and McKinley, G. H. (2005). Capillary break-up rheometry of low-viscosity elastic fluids. Appl. Rheol. 15 (1), 12–27. PubMed PMID: WOS:000233116600004. doi:10.1515/arh-2005-0001
Rowley, A. F., and Ratcliffe, N. A. (1976). The granular cells of Galleria mellonella during clotting and phagocytic reactions in vitro. Tissue Cell 8, 437–446. doi:10.1016/0040-8166(76)90004-5
Salt, G. W. (1970). The cellular defence reactions of insects. Cambridge: Cambridge University Press.
Scherfer, C., Karlsson, C., Loseva, O., Bidla, G., Goto, A., Havemann, J., et al. (2004). Isolation and characterization of hemolymph clotting factors in Drosophila melanogaster by a pullout method. Curr. Biol. 14 (7), 625–629. PubMed PMID: WOS:000220809900030. doi:10.1016/j.cub.2004.03.030
Staczek, S., Zdybicka-Barabas, A., Mak, P., Sowa-Jasilek, A., Kedracka-Krok, S., Jankowska, U., et al. (2018). Studies on localization and protein ligands of Galleria mellonella apolipophorin III during immune response against different pathogens. J. Insect Physiology 105, 18–27. PubMed PMID: WOS:000427218000003. doi:10.1016/j.jinsphys.2017.12.009
Steinberg, M. S. (2007). Differential adhesion in morphogenesis: a modern view. Curr. Opin. Genet. Dev. 17 (4), 281–286. PubMed PMID: WOS:000249366400004. doi:10.1016/j.gde.2007.05.002
Stoepler, T. M., Castillo, J. C., Lill, J. T., and Eleftherianos, I. (2013). Hemocyte density increases with developmental stage in an immune-challenged forest caterpillar. Plos One 8 (8), e70978. PubMed PMID: WOS:000324401500055. doi:10.1371/journal.pone.0070978
Strand, M. R. (2008). The insect cellular immune response. Insect Sci. 15 (1), 1–14. PubMed PMID: WOS:000252587700001. doi:10.1111/j.1744-7917.2008.00183.x
Sun, Y., Ma, J., Peng, F., and Kornev, K. G. (2022). Making droplets from highly viscous liquids by pushing a wire through a tube. Phys. Fluids 34 (3), 032119. doi:10.1063/5.0082003
Sun, Y. M., Bazilevsky, A. V., and Kornev, K. G. (2023). Classification of axisymmetric shapes of droplets on fibers. Could non-wettable fibers support axisymmetric droplets? Phys. Fluids 35 (7), 072004. PubMed PMID: WOS:001025235200004. doi:10.1063/5.0151950
Theopold, U., Li, D., Fabbri, M., Scherfer, C., and Schmidt, O. (2002). The coagulation of insect hemolymph. Cell. Mol. Life Sci. 59, 363–372. PubMed PMID: 11915949. doi:10.1007/s00018-002-8428-4
Theopold, U., Schmidt, O., Soderhall, K., and Dushay, M. S. (2004). Coagulation in arthropods: defence, wound closure and healing. Trends Immunol. 25 (6), 289–294. PubMed PMID: WOS:000222302100004. doi:10.1016/j.it.2004.03.004
Wigglesworth, V. B. (1937). Wound healing in an insect (Rhodnius prolixus, Hemiptera). J. Exp. Biol. 14 (3), 364–381. PubMed PMID: WOS:000200577900010. doi:10.1242/jeb.14.3.364
Wigglesworth, V. B. (1979). “Hemocytes and growth in insects,” in Insect hemocytes: development, forms, functions, and techniques. Editor A. P. Gupta (Cambridge, UK: Cambridge University Press), 303–318.
Yan, X. J., Carr, W. W., and Dong, H. M. (2011). Drop-on-demand drop formation of polyethylene oxide solutions. Phys. Fluids 23 (10), 107101. PubMed PMID: WOS:000296528000029. doi:10.1063/1.3643269
Zdybicka-Barabas, A., and Cytrynska, M. (2013). Apolipophorins and insects immune response. Isj-Invertebrate Surviv. J. 10 (1), 58–68. PubMed PMID: WOS:000328155800001.
Keywords: cell aggregation, hemolymph, hemocytes, clotting kinetics, Lepidoptera, rheology
Citation: Aprelev P, Brasovs A, Bruce TF, Beard CE, Adler PH and Kornev KG (2024) To seal a wound, caterpillars transform blood from a viscous to a viscoelastic fluid in a few seconds. Front. Soft Matter 4:1341129. doi: 10.3389/frsfm.2024.1341129
Received: 19 November 2023; Accepted: 13 February 2024;
Published: 27 March 2024.
Edited by:
Frank Alexis, Universidad San Francisco de Quito, EcuadorReviewed by:
Luca Lanotte, INRA Centre Bretagne-Normandie, FranceCopyright © 2024 Aprelev, Brasovs, Bruce, Beard, Adler and Kornev. This is an open-access article distributed under the terms of the Creative Commons Attribution License (CC BY). The use, distribution or reproduction in other forums is permitted, provided the original author(s) and the copyright owner(s) are credited and that the original publication in this journal is cited, in accordance with accepted academic practice. No use, distribution or reproduction is permitted which does not comply with these terms.
*Correspondence: Konstantin G. Kornev, a2tvcm5ldkBjbGVtc29uLmVkdQ==
†These authors have contributed equally to this work
Disclaimer: All claims expressed in this article are solely those of the authors and do not necessarily represent those of their affiliated organizations, or those of the publisher, the editors and the reviewers. Any product that may be evaluated in this article or claim that may be made by its manufacturer is not guaranteed or endorsed by the publisher.
Research integrity at Frontiers
Learn more about the work of our research integrity team to safeguard the quality of each article we publish.