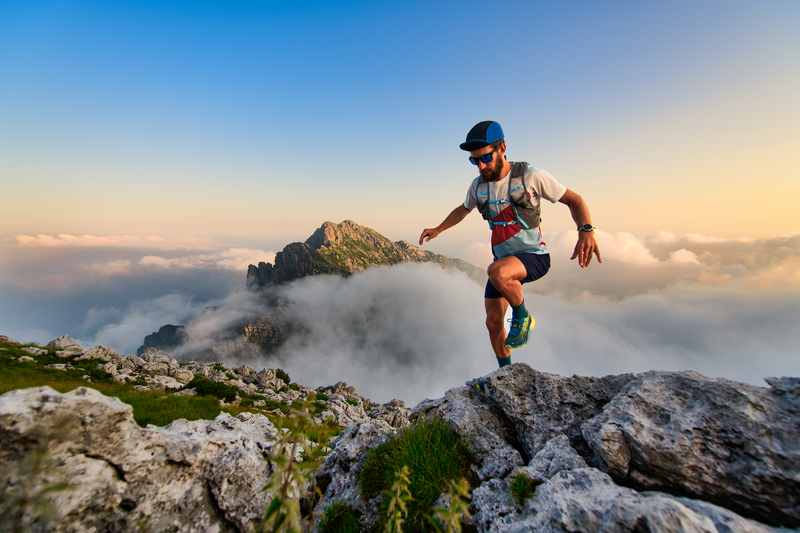
95% of researchers rate our articles as excellent or good
Learn more about the work of our research integrity team to safeguard the quality of each article we publish.
Find out more
ORIGINAL RESEARCH article
Front. Soft Matter , 30 November 2023
Sec. Colloids and Emulsions
Volume 3 - 2023 | https://doi.org/10.3389/frsfm.2023.1328195
This article is part of the Research Topic 1st Galapagos Soft Matter Conference - Research Topic View all 11 articles
The combination of surfactants and fatty alcohols leads to the formation of lamellar gel network (LGNs) which are widely used in cosmetic and pharmaceutical industries. Alkyl polyglucosides are known to stabilize oil-in-water emulsions and here, we report their use in combination with fatty alcohol for the stabilization of all-aqueous water-in-water (W/W) emulsions based on dextran-in-poly(ethylene glycol). Two different APGs were used: decyl glucoside and lauryl glucoside. We systematically studied the influence of the concentrations of APGs, and the molar ratio between the APGs and decanol as model fatty alcohol on the size and stability of the aqueous emulsion droplets with time. The self-assembled structure formed by decanol/APGs was characterized by using Small Angle X-ray Scattering and confocal microscopy, and shown to be lamellar in the bulk phase and probably also at the surface of dextran-rich droplets. We also demonstrated that the stabilization of W/W emulsions can be extended to other alkyl polyglucoside and to other fatty alcohols. In addition, we reported the production of a foam using such W/W emulsions as the continuous phase. Our results show that it is possible to stabilized W/W emulsions using LGNs based on different APGs and fatty alcohols, which will undoubtedly expand the use of W/W emulsions for various fields.
Emulsions, which are commonly used in a wide range of applications, are made up of two thermodynamically incompatible solutions or solvents (Leal-Calderon et al., 2007). The most conventional ones are oil-in-water or water-in-oil emulsions. However, a more unusual emulsion system is based on an aqueous solution that forms droplets into another aqueous solution, the so-called ‘water-in-water emulsions’ (W/W emulsions) (Esquena, 2016; 2023; Dickinson, 2019). The formation of W/W emulsions occurs when a moderately concentrated solution of incompatible polymers exhibits thermodynamic phase separation (Dickinson, 2019). In contrast to oil-in-water emulsions, W/W emulsions are much more difficult to stabilize since the surface tension is very low (around 0.1–100 μN. m−1) and the interface thickness is large (at least, few tens of nanometers) (Antonov et al., 2004; Esquena, 2016). For these physical reasons, W/W emulsions cannot be stabilized by the common surfactants usually used for the stabilization of the oil-in-water or water-in-oil emulsions. Thus, when formed upon mixing, droplets coalesce with time more or less quickly, depending on the system investigated and then, macroscopic phase separation occurs. The lack of stability of W/W emulsions is the main drawback that limits their use for practical applications (Esquena, 2016). Several applications of W/W emulsions have been reported in various fields: food, biomedical, drug delivery, etc. (Esquena, 2016; Esquena, 2023). For example, they can be used to encapsulate active components and labile molecules, and then be used as delivery systems. They can also be used as microreactors for the synthesis of high value-added products (Esquena, 2016; Esquena, 2023). The field of water-in-water emulsions is experiencing growth, driven by the discovery of new methods to improve their stability. Therefore, finding new methods to effectively stabilize W/W emulsions is a major challenge for scientists to expand their use.
Despite the difficulties in stabilizing them, W/W emulsions have been shown to be efficiently stabilized by using a wide variety of chemicals and particles as reviewed by several authors (Nicolai and Murray, 2017; Dickinson, 2019; Chao and Shum, 2020). For example, W/W emulsions can be stabilized by polymer particles (Poortinga, 2008; Douliez et al., 2018; Douliez et al., 2019), microgels (Merland et al., 2022; You et al., 2023), cellulose nanocrystals (Ben Ayed et al., 2018; Xie et al., 2023), protein particles (Nguyen et al., 2013), etc. Other stabilization methods based on the use of amphiphiles are also described in the literature such as: block copolymers, self-assembly of fatty acids and phospholipids, oligonucleotides, liposomes, etc., (Dewey et al., 2014; Chao and Shum, 2020). These chemicals or particles are positioned at the interface of the droplets, which prevents their coalescence and, subsequently, macroscopic phase separation. The mechanisms by which this occurs remain unclear in most cases and is probably due to an equal affinity of the particles or chemicals for the droplet-forming polymer and the continuous aqueous phase enriched in the other polymer.
Recently, stable W/W emulsions have been designed by using sodium oleate (surfactant) and decanol (fatty alcohol) with a simple addition process of the components to the two aqueous phase system (Coudon et al., 2022). The mixture of surfactant and fatty alcohols are widely used forming Lamellar gel Network (LGNs) that are of strong interest in the field of cosmetic and pharmaceutical industries (Eccleston, 1997). Lamellar gel networks (LGNs), which are also called “α-gel” in the literature, are used to give creamy texture to the products (Eccleston, 1997; Iwata, 2017). LGNs are based on the mixture of long-chain fatty alcohols with surfactants in aqueous solution (Iwata, 2017; Colafemmina et al., 2020; de Oliveira et al., 2020). By using appropriate concentrations and molar ratio of these two components, the formation of LGNs occurs. It can be described as a multi-phase colloidal structure mainly composed of lamellar phase and bulk water. When oil droplets are present in the formulation, they are entrapped and stabilized by the LGNs both present in the bulk aqueous phase and surrounding them (Junginger, 1984; Eccleston, 1997; Nakarapanich et al., 2001). The study of Coudon et al. was the first one showing the use of LGNs to stabilize water-in-water (W/W) emulsions (Coudon et al., 2022).
In the current context of growing concern for the environment, natural-based surfactants became popular as an alternative to the synthetic ones to produce LGNs and are widely used for cosmetic and pharmaceutical applications (Savic et al., 2005; Terescenco et al., 2018a; Terescenco et al., 2018b). Alkyl polyglucoside (APG) is one of the well-known families of natural surfactants, derived from natural glucose and a fatty alcohol. It is considered as non-toxic, mild and environmentally friendly emulsifier (Dari et al., 2023). Our aim here was to study the stabilization of W/W emulsions by using LGNs based on various APGs. Interestingly, these surfactants (APGs) are non-ionic surfactant whereas most of the chemical components and particles that have been used in the literature for stabilizing W/W emulsions are either charged or at least, zwitterionic (Esquena, 2023). This could be an advantage when the systems require changes in pH or ionic strength that may alter the stability of the emulsion, as is the case when W/W emulsions are used for bacterial culture (Xie et al., 2023; Zhang et al., 2024).
Here, we used two different APGs: decyl glucoside (DG) and lauryl glucoside (LG). DG and LG differ in their alkyl chain length composition. DG comprises a mixture of alkyl chains ranging from 8 to 16 carbon atoms, while LG consists of a mixture ranging from 12 to 16 carbon atoms (Keck et al., 2014; Wu et al., 2017). In addition, they differ in their degree of glycosidation: DG has a value of 1.5 and LG, 1.4. These APGs were combined with decanol as model fatty alcohol. The model W/W emulsions system was based on dextran-in-poly(ethylene glycol) (PEG) droplets. We systematically investigated the influence of the concentrations of APG, and the molar ratio between this surfactant and the fatty alcohol on the size and stability with time of the aqueous emulsion droplets. Then, we gained insight into the structure by combining confocal microscopy and Small Angle X-ray Scattering (SAXS). We also demonstrated the versatility of these surfactants mixtures by extending the approach to another APG (coco-glucoside, CG) and other fatty alcohols (dodecanol and myristyl alcohol). Furthermore, we show that a foamulsion could be produced from such stabilized W/W emulsion. Our results show that it is possible to use the combination of fatty alcohols and APGs for the formulation of different type of W/W emulsions, which will surely broaden the use of W/W emulsions for various fields such as cosmetics and pharmaceuticals.
The following chemicals were purchased from Sigma-Aldrich (Saint Quentin Fallavier, France) and used as received: dextran 450–650 kg/mol, poly(ethylene glycol) 20 kg/mol (PEG), Nile red, fluorescein isothiocyanate-dextran 500 kg/mol (FITC-dextran), 1-decanol, 1-dodecanol, ethanol, and sodium dodecyl sulfate (SDS) with 98.5% purity. Lauryl glucoside (plantacare 1200 up) (LG) with 51.3 wt% of active matter, decyl glucoside (plantacare 2000 up) (DG) with 53.0 wt% of active matter, coco glucoside (plantacare 818 up) with 51.4 wt% of active matter and myristyl alcohol (lanette 14) were provided by BASF (Ludwigshafen, Germany) and used as received. Milli-Q water was used for all experiments.
To prepare the all-aqueous emulsion, both the polymers PEG and Dextran were weighed in a flask and Milli-Q water was added so that the PEG concentration was 7 wt% and the dextran concentration was 3.25 wt%. Then, the mixture was stirred magnetically at 60°C until polymers were completely dissolved. It formed a slightly turbid dispersion formed by polydisperse droplets enriched in Dextran in the continuous PEG phase, which coalesced with time until macroscopic phase separation occurs as expected for such aqueous emulsion (not shown).
A solution of APG (DG, LG or CG) was prepared by weighing into a flask the APG and adding Milli-Q water so that the final concentration of the APG active matter was 5 wt%, followed by stirring magnetically at 60°C until the APG was completely dissolved. Nile red was dissolved in ethanol to obtain a final concentration of 5 mg mL−1, then an aliquot of this solution was added to each of the APGs solutions to obtain a final concentration of 0.1 mg mL−1. A solution of FITC-dextran was prepared by weighing the dye and adding Milli-Q water so that the concentration was 20 mg mL−1. All these stock solutions were stored at around 4°C in a fridge.
Different protocols were used to prepare W/W emulsions depending on the fatty alcohols used and its melting point. To produce PEG-in-dextran W/W emulsion, 5 mL of the PEG-dextran mixture was taken and placed in a 15 mL tube (Falcon) while stirring the mixture. 50 μL of the FITC-dextran stock solution were added to the tube and all was mixed by vortexing; followed by the addition of a given volume of the stock solution of APG (DG, LG or CG) doped with nile red. Then the emulsion was vortexed for another 30 s. The same protocol was used to prepare the inverse W/W emulsion (PEG-in-dextran emulsions) but starting with the preparation of PEG at 2 wt% and Dextran at 14 wt%.
To prepare the dextran-in-PEG emulsions in the presence of APG and dodecanol, 5 mL of the PEG-dextran mixture was taken and placed in a 15 mL tube (Falcon) while stirring the mixture at 40°C. Then, 50 μL of the FITC-dextran stock solution was added to the tube and all were mixed by vortexing. A given volume of 1-dodecanol at 40°C was added to the previous mixture and mixed thoroughly by vortexing, followed by the addition of a given volume of the stock solution of LG or DG doped with Nile red, then the emulsion was vortexed for another 30 s.
To prepare dextran-in-PEG emulsions in the presence of APG and myristyl alcohol, the myristyl alcohol was first heated to 90°C, until it was completely melted, and then the required amount was weighed in a beaker. 5 mL of the PEG-dextran mixture was taken and placed in the same baker while stirring the mixture, 50 μL of the FITC-dextran stock solution was added, and all were mixed together under magnetic stirring at 60°C until the myristyl alcohol was completely dissolved. The resulting mixture was placed in a 15 mL tube (Falcon) and a given volume of LG or DG doped with Nile red was added while vortexing. Then, the emulsion was vortexed for another 30 s.
A dextran-in-PEG emulsion (10 wt% of dextran and 10 wt% of PEG) stabilized with LG and decanol was prepared as previously described. A given volume of the emulsion was transferred to a beaker and a given volume of surfactant was added. The mixture was slowly mixed with magnetic stirring for 30 s. To prepare the foamulsion, the double-syringe foaming technique was used (Gaillard et al., 2017). Two 10-mL syringes were connected with a Luer-lock connector. One syringe was filled with 3.5 mL of the mixture and 6.5 mL of air. The second syringe was maintained with the piston in the fully closed position. The foam was then produced by pushing the plungers of the connected syringes 30 times by hand. The stability of the resulting foams was followed by measuring both the evolution of the foam and the volume of drained liquid over time with the naked eye.
The bulk stability of the W/W emulsions with different molar ratio (R) of decanol:APG was followed with the naked eye (phase separation), and also by confocal fluorescence microscopy observations. Experiments were performed immediately after the production of W/W emulsions (t = 0) and after 24 h (t = 24 h) of conservation at room temperature. Pictures of the tubes containing the samples were taken with an iPhone mini 13 (Apple). Microscopy images were acquired using a fluorescence confocal microscope (LSM 700, Zeiss) with ×63 oil immersion objective. Dyes were excited by using the following excitation (λex): FITC-dextran, λex = 488 nm; Nile Red, λex = 555 nm. The microscopy images were processed using ImageJ software, following the method introduced by Gaillard et al. (2015) in order to obtain the area of 50 droplets for each sample. Long-term evolution of the W/W was also done through microscopy observations of the samples using an epifluorescence microscope (Zeiss Axioskop 2 Plus, Oberkochen, Germany) with ×40 objective.
SAXS experiments were performed on a XEUSS 2.0 device (XENOCS, Grenoble, France) operating under vacuum with a GeniX3D microsource (λ = 1.54 Å) at 0.6 mA and 50 kV and a 2D Pilatus 3R 200 K detector. The sample was loaded in thin quartz capillaries (optical path 1.5 mm, WJM-Glas/Müller GmbH, Germany) and the signal collected for 3 h. The detector was placed perpendicularly to the direct beam at distance of 2.3 m, calibrated in both cases with a Silver Behenate standard. Peak fit® software was used for diffractogram deconvolution by using combination of Gaussian and Lorentzian functions.
The CMC values of the surfactants were obtained by using the automatized surface tension plate reader Kibron Delta-8 (Kibron, Finland). A volume of 50 μL of aqueous dispersion was placed on the 96-hole platform. Measurements were performed in duplicate at 28°C ± 1°C after a waiting time of 10 min to ensure equilibrium at the air-water interface. A calibration was performed by using ultrapure water at 28°C ± 1°C.
The samples were centrifuged in a centrifuge (Sigma 6K15, Thermo Fisher Scientific, Strasbourg, France) at 1500 × g for 10 min. Microscopy images of the samples were taken before and after centrifugation using an epifluorescence microscope (Zeiss Axioskop 2 Plus, Oberkochen, Germany) with ×40 objective.
The radius of 50 droplets was calculated for each sample and the average and standard deviation were determined. The results were compared by one-way analysis of variance and Tukey’s test to analyze statistical differences (p < 0.05). The analysis was performed using SAS V8.0 software (SAS Institute, Gary, NC, United States).
Dextran-in-PEG emulsions were prepared at 3.25 wt% (W/W) dextran (450–650 kg/mol) and at 7 wt% (W/W) PEG (20 kg/mol), since at this composition the polymers were fully phase-separated as determined previously in the literature (Diamond and Hsu, 1989). In these experimental conditions, dextran enriched droplets formed in the continuous enriched PEG phase. Droplets of ca. 10–50 µm formed upon shaking when observed immediately after preparation but coalesced with time yielding macroscopic phase separation as already described in the literature (Coudon et al., 2022).
Then, we added different quantities of decyl glucoside (DG) and decanol varying from 1 to 12 mM as described in the Materials and Methods Section. The concentration of the APGs varied from 2 to 20 times the CMC (Supplementary Figures S1, S2). The molar ratio (R) corresponds to the moles number between decanol and DG, and it varied from 1:0 to 0:1. The stability of the W/W emulsions were determined after 24 h at room temperature with the naked eye to eventually determine the presence of a phase separation or a supernatant (Figure 1). When phase separation occurred, we defined this W/W emulsion as unstable. When a small supernatant was observed, we classified this emulsion as medium stability, and when no macroscopic change was observed, we defined these emulsions as stable (Figure 1).
FIGURE 1. Partial phase diagram determined by visual observations by varying the decyl glucoside (DG) concentration as a function of the molar ratio between decanol and decyl glucoside showing the stable domain of W/W emulsion (dashed black line). Red crosses correspond to samples where macroscopic phase separation occurred after 24 h. Orange circles correspond to samples where a slight macroscopic phase separation occurred after 24 h. Green circles correspond to stable samples after 24 h. On the right side, pictures of samples illustrate the three regimes. The samples are from left to right: unstable W/W emulsion for DG = 9 mM and R = 0:1, medium stability W/W emulsion for DG = 6 mM and R = 3:1, and high stability W/W emulsion for DG = 9 mM and R = 2:1.
The addition of the pure components, either DG or decanol, whatever the concentrations led to complete phase separation of the aqueous emulsion within 3 h. Then, the pure components did not have a significant effect on the behavior of the polymer’s mixtures, and they could not stabilize dextran-rich droplets. However, the addition of the two components (DG and decanol) led to stabilization of emulsions for a relatively large domain of concentrations and molar ratios. A minimal concentration of DG (6 mM) and a minimal fraction of decanol (R = 0.5:1) were required to produce emulsions with medium stability. At the same DG concentration (6 mM), and with higher decanol fractions (R = 1:1 and R = 2:1) emulsions with high stability were observed. However, when the decanol fraction was further increased (R ≥ 3:1), a supernatant was observed after 24 h leading to an emulsion with medium stability. When the concentration of DG was increased to 9 mM, an emulsion with medium stability was only observed for low R (R = 0.5:1). Then, emulsions with no supernatant were observed after 24 h for all the other R studied (1:1 > R ≥ 9:1). At a DG concentration of 12 mM, emulsions with high stability were obtained both at low decanol fraction and at high decanol fractions (from R = 0.5:1 to R = 9:1).
We then decided to test the substitution of the DG by another APG with a longer alkyl chain: lauryl glucoside (LG). Dextran-in-PEG emulsions were then prepared with different concentrations of LG from 1 to 12 mM and with a molar ratio (R) between decanol and LG, R varying from 1:0 to 0:1. The stability of the W/W emulsions were determined in the same way as for the emulsions stabilized with DG described previously (Figure 2 and Supplementary Table S1B). The addition of pure LG whatever the concentration led to complete phase separation within 3 h, as previously described for pure DG or pure decanol. The addition of decanol in combination with LG was necessary to stabilize the emulsions. A minimal concentration of LG (6 mM) and a minimal fraction of decanol (R ≥ 0.5:1) were required to produce emulsions with medium stability. This result was similar to the one obtained for DG. When the molar ratio was increased (R ≥ 1:1), emulsions with high stability were obtained for all the concentrations (6, 9 and 12 mM). The increase of the concentration of LG to 12 mM led to emulsions with high stability even at a minimal fraction of decanol (R = 0.5:1). Stable emulsions with LG were produced for a wider range of R in comparison to DG (Figures 1, 2).
FIGURE 2. Partial phase diagram determined by visual observations by varying the lauryl glucoside (LG) concentration as a function of the molar ratio between decanol and Lauryl glucoside showing the stable domain of W/W emulsion (dashed black line). Red crosses correspond to samples where macroscopic phase separation occurred after 24 h. Orange circles correspond to samples where a slight macroscopic phase separation occurred after 24 h. Green circles correspond to stable samples after 24 h.
Then, we also determined the droplets size evolution between the initial size (t = 0, that stands for observations done immediately after mixing all components) and after 24 h with statistical analysis by confocal fluorescence microscopy of the samples doped with FITC-dextran and Nile red (Figure 3, Supplementary Figure S3 and Supplementary Tables S1A, B). First, we compared the initial droplets size between DG and LG for all the R and concentrations to determine the main parameters leading to the smallest droplets size. For DG with R = 0.5:1, by varying the concentration from 6 to 12 mM, we observed a decrease of the average radius from 7.3 ± 3.6 to 2.1 ± 1 μm. The same trend was observed for all the concentrations and all the R showing that the initial droplet sizes decreased by increasing the DG concentration (Figure 3). For LG with R = 0.5:1, by varying the concentration from 6 to 12 mM, we observed a decrease of the average radius from 5.9 ± 3.1 to 3 ± 1.1 μm, respectively for 6 and 9 mM. However, there was no statistical differences from 9 to 12 mM, with an average radius of 3 ± 1.1 and of 2 ± 0.5 μm for example, for R = 0.5:1. The concentration of the APG was a key parameter for controlling the initial droplet size. We hypothesize that by increasing APGs concentration leading also to a concomitant increase of decanol in the formulation, an increase of viscosity of the bulk phase could occur leading to a decrease of the droplets size. However, it is important to keep in mind that other parameters could also explain this phenomenon: quantity and structure of the self-assembly formed, interaction between APGs and polymers, etc.
FIGURE 3. Effect of both DG concentration and decanol:DG ratio (R) on W/W emulsions droplets size. Confocal microscopy images of the W/W emulsions taken at t = 0 for two different DG concentrations: 6 mM (top) and 12 mM (bottom), and three different R: R = 3:1(left), R = 6:1 (middle) and R = 9:1 (right). Droplets are labeled with FITC-dextran dye. The scale bar represents 15 μm.
Between the two APGs, we observed also statistical differences between the initial droplet sizes, with bigger droplets for DG than for LG when the concentration was set at 6 and 9 mM. However, the initial droplet size was the same at 12 mM for both APGs. The difference between DG and LG could be explained by the fact that APGs have different CMC values (Supplementary Figure S1), with LG having a lower CMC than DG. Here, all the samples were produced above the CMC. However, at the same concentration, LG with a longer alkyl chains and lower CMC than DG produce more easily rod-like micelles (Platz et al., 1995). Thus, it is easier to transit from rod-like micelles to lamellar phases by adding a small amount of fatty alcohol than from spherical micelles. Moreover, the presence of an excess of lauryl glucoside under rod-like micelles leading to a more viscous bulk continuous phase than for DG under spherical micelles could also explain the difference of stability at the lowest concentration (6 mM) (Stradner et al., 2000; Chu et al., 2013). We believe that the presence of rod-like micelles helps with the formation of the lamellar phases that stabilize the droplets. Then, we studied the effect of the molar ratio (R) between decanol and the APGs (DG or LG) on the initial droplets size (Supplementary Tables S1A, B). For DG at the three concentrations, we observed that for R = 0.5:1 the initial droplet size was always higher than for all the others R. The same trend was observed for LG. For R = 0.5:1, there was an excess of APGs in comparison to the decanol which could lead to the formation of a low quantity of lamellar structures in a mixture with APGs micelles and mixed micelles containing decanol (Möller et al., 1998). The quantity of lamellar structures could be not sufficient to stabilize small droplets during the production of the W/W emulsion. Then, we observed for both LG and DG that for R with high amount of decanol in comparison to the APG (R = 6:1 and R = 9:1), the droplet size was statistically lower than for R = 0.5:1 with an excess of APGs, but always higher than for intermediate R: R = 3:1, R = 2:1 and R = 1:1 (Figure 3 and Supplementary Figure S3). The best R to have high stability with small droplet size were R = 3:1, R = 2:1 and R = 1:1. These 3R lead to the smallest droplet size. Therefore, R is also a key parameter to take into account to reach small droplet size. A large excess of APG (R = 0.5:1) or decanol (R ≥ 6:1) is not appropriate for small droplet size. We compared also the droplet size evolution after 24 h for all the stable samples detected by visual observations (Figures 1, 2). No statistical difference was observed showing that in all cases the droplet size was stable after 24 h (Figure 3, Supplementary Figure S3 and Supplementary Tables S1A, B). Again, the composition (concentration and molar ratio) had been shown to influence the diameter of droplets in a similar system (Coudon et al., 2022).
Our results confirm that stabilization of the interface between hydrophilic polymers is not possible using amphiphilic molecules alone (Esquena, 2016). Instead, changing the self-assembly of these molecules by adding fatty alcohol proves to be an effective method to produce stable all-aqueous droplets (Coudon et al., 2022; Esquena, 2023). In addition, our results highlight that to obtain stable emulsions based on mixture of fatty alcohol and APGs, whatever the type of oil-in-water or water-in-water emulsion, a minimum molar ratio of 1:1 fatty alcohol:surfactant is required (Terescenco et al., 2018b). As described in the literature for LGNs formation based on mixture of fatty alcohols and surfactants, a minimum amount of fatty alcohol is required, and it is reported to be close to a weight ratio 1:1 as found in this study (Eccleston, 1997). Moreover, comparing our results to those obtained for LGNs based on sodium oleate and decanol, we observe that whatever the nature of the surfactants (anionic or non-ionic surfactants) with fatty alcohol, stable W/W emulsions are only possible for a molar ratio higher than 1:1 (Coudon et al., 2022).
The long-term stability of the emulsions was studied by microscopy observations during 42 days. Fluorescence microscopy observations of the emulsions stabilized with DG at a concentration of 12 mM and with R = 3:1 showed the presence of uniform droplets that did not change significantly in size after 7 days compared to t = 0 (average radius = 1.4 ± 0.4 μm) (Supplementary Figure S4A). However, after 7 days a macroscopic phase separation was observed with the naked eye. Microscopy observations of the emulsions stabilized with LG at a concentration of 12 mM and with R = 3:1 showed the presence of uniform droplets that did not change significantly in size after 42 days compared to t = 0 (average radius = 1.6 ± 0.6 μm) (Supplementary Figure S4B). In addition, we tested the robustness of the emulsions with centrifugation. After centrifugation at 1500 × g for 10 min, we observed neither phase separation nor supernatant showing that the self-assembled structure formed by decanol and APGs form a robust layer protecting the droplets against mechanical stress. We also checked the effect of the centrifugation directly on the droplets by measuring the droplets size before and after the centrifugation (Figure 4). The results show that both emulsions could be centrifuged without significant change in the droplet radius (average radius before centrifugation: 2.1 ± 0.7 and 1.7 ± 0.5 μm, respectively for DG and LG; average radius after centrifugation = 2.0 ± 0.6 μm for DG, and 1.8 ± 0.4 μm for LG) confirming that the self-assembled structures formed by APGs and decanol both in bulk and at the interface led to strong protection of the emulsion against destabilization.
FIGURE 4. Effect of centrifugation on the stability of the W/W emulsions. Epifluorescence microscopy images of W/W emulsions stabilized with DG (top) or LG (bottom) at a concentration of 12 mM with a molar ratio of R = 3:1, taken before centrifugation (left) and after centrifugation (right). Droplets are labeled with FITC-dextran dye. The scale bar represents 60 μm.
After establishing the phase diagrams of the W/W emulsions stabilized by the mixture of decanol and APGs, we tried to characterize the structure formed by the decanol:APGs in bulk and at the interface leading to the long-term stabilization of the droplets for specific ratios. By using confocal fluorescence microscopy with Nile red staining, the fatty alcohol, it was possible to highlight the presence of surfactant assemblies in the bulk phase surrounding the droplets (Figure 5A). We observed the presence of lamellar structures in the bulk phase surrounding the droplets forming the so-called LGN.
FIGURE 5. (A) Confocal microscopy image of dextran-in-PEG emulsion stabilized with LG at a concentration of 9 mM and with a molar ratio of decanol:LG at 1:1, showing the presence of lamellar phases (some of them identified with white arrows) in the continuous phase of the emulsion. Nile red was used to label the surfactant and fatty alcohol. The scale bar represents 15 μm. (B) 1D SAXS diffractogram of a water-in-water emulsion stabilized by a mixture of LG and decanol with a LG concentration of 12 mM and a molar ratio of decanol:LG at 3:1. Only the third order Bragg peak (3Q0) is identified on the scattering pattern, labeled with a black arrow. Schematic showing the stabilization of the W/W emulsion by lamellar phase surrounding the dextran-rich droplets.
However, with this microscopy technique it is impossible to study the interfacial structure and the eventual formation of LNGs around the droplets, since only a red layer is observed by confocal microscopy surrounding the droplets (Figure 5A). That is why we used SAXS to get information at the interface scale. The stable emulsion formulated with LG at 12 mM and with R = 3:1 was chosen for SAXS analysis due to the long-term stability and high concentration to optimize the SAXS signal. On the SAXS scattering pattern, only one main peak could be distinguished at Q = 0.035 Å−1 (Figure 5B). By comparing with the literature and the SAXS results obtained by Coudon et al. (2022) on similar water-in-water emulsion system stabilized by a mixture of fatty alcohol and sodium oleate, we hypothesize that the peak at 0.035 Å−1 is the third order peak of a lamellar phase surrounding the dextran-rich droplets. The lamellar phase would have a lamellar interspacing (d) of around 52 nm (d = 2π/Q0). Indeed, in the study from Coudon et al., they determined a lamellar spacing of around 78 nm, corresponding to a third order peak around 0.024 Å−1 close to the peak determined here (Coudon et al., 2022). In our system, the lamellar spacing was lower than in the case of the lamellar phase made of sodium oleate and decanol system, which can be explained by the fact that we are working with non-ionic surfactant and lamellar phases are stabilized by steric repulsion and not electrostatic repulsion such as in the case of sodium oleate/decanol system. It is known that steric repulsion produced in lamellar gel network based on fatty alcohol leads to lower interlamellar spacing than LGNs produced by anionic surfactants (Iwata, 2017). Therefore, the long-term stabilization and the resistance to centrifugation of the W/W emulsions could come from the LGNs formed by APGs and decanol both in bulk and at the interface leading to strong protection of the emulsion against destabilization. However, to confirm the interfacial structures, further investigations need to perform. For example, the use of freeze-Fracture transmission electron microscopy could help to better characterize the self-assembled structures formed at the interface as already demonstrated by Coudon et al. (2022). It is important also to notice that we do not know the quantity of each chemical components inside the self-assembled structures, and the concentration could be different from the initial weight ratios used to produce the W/W emulsion. Most probably lamellar phases are in coexistence with APGs micelles.
Here, we varied the experimental conditions, either the concentration of polymers or the nature of LGNs but investigated only few different concentrations of each to demonstrate the versatility of the systems. First, we studied the effect of LGNs on the stabilization of the inverse W/W emulsion, that is, when polymer concentrations are adjusted so that PEG-rich droplets formed in a continuous dextran-rich phase (Supplementary Figure S5). Stable W/W emulsion were produced from both DG and LG at 12 mM with R = 3:1, since no change in the droplets size was observed after 24 h. This result shows a general mechanism based on APG and fatty alcohol for stabilizing PEG and dextran interfaces regardless the nature of the droplets, which is in accordance with the observations of Coudon et al. on the stabilization W/W emulsion with decanol and sodium oleate (Coudon et al., 2022).
In a second step, we extended the production of W/W emulsions to another well-known and widely used APG: coco-glucoside (CG), composed of a mixture of alkyl groups with carbon chain lengths ranging from 8 to 16 atoms and with a higher proportion of longer alkyl chains in comparison to DG and LG (Aguirre et al., 2014). Self-assembled structures (lamellar phases and/or micelles) produced with CG 12 mM at different decanol: CG molar ratios, from R = 1:1 to R = 6:1, effectively stabilized dextran-in-PEG emulsions, since microscopy images showed that no significant differences in the droplet size were observed after 24 h compared to the initial size (Supplementary Figure S6).
Finally, we extended our approach of stabilizing W/W emulsions produced from DG and LG to other fatty alcohols with longer alkyl chain length (dodecanol and myristyl alcohol) (Figure 6 and Supplementary Figure S7). Microscopy images showed that dextran-in-PEG emulsions with high stability can be produced with DG at a concentration of 12 mM in combination with dodecanol (dodecanol:DG molar ratio R = 6:1), since no significant differences in the droplet size were shown after 24 h compared to the initial size (average radius 4 ± 1.2 µm). The same observations were performed for emulsions stabilized with LG at a concentration of 12 mM (dodecanol:LG molar ratio R = 6:1) but the droplet size was slightly smaller (average radius 2.2 ± 0.7 μm at t = 0). The stabilization of dextran-in-PEG emulsion with APG and myristyl alcohol was also possible. Emulsions stabilized with DG at a concentration of 12 mM and myristyl alcohol (R = 6:1), showed high stability without significant changes in droplet size after 24 h compared to the initial size (average radius = 3.2 ± 0.7 µm). The same emulsion stabilized with LG at a concentration of 12 mM (R = 6:1) led to a decrease in droplet size (average radius 1.6 ± 0.3 μm at t = 0), with no significant change in size after 24 h. These results show that it is possible to formulate W/W emulsions using APGs and different fatty alcohols. Thus, W/W emulsion stabilization is possible by using APGs and fatty alcohol when suitable concentration and ratios are used to obtain self-assembled structures such as lamellar phases.
FIGURE 6. W/W emulsions stabilized with DG in combination with two different fatty alcohols: dodecanol and myristil alcohol. Epifluorescence microscopy images of W/W emulsions stabilized with DG at 12 mM and Dodecanol: DG ratio equal to 6:1 (left) and DG at 12 mM and myristyl alcohol: DG ratio equal to 6:1 (right), taken at t = 0 (top) and at t = 24 h (bottom). Droplets are labeled with FITC-dextran dye. The scale bars represent 60 μm.
For certain applications, bubbles are added into emulsions to change their mechanical properties and/or sensorial performances (Salonen, 2020). It is the case, for example, for ice creams, whipped creams and pharmaceutical or cosmetic applications (Parsa et al., 2019; Murray, 2020; Luengo et al., 2021). These three phase systems are called foamulsions or “foamed emulsions,” where the gas bubbles are surrounded by a continuous phase, traditionally composed of oil and water (Salonen, 2020; Zheng et al., 2022). The continuous phase is generally present in the form of oil-in-water emulsions, although it can also be in the form of water-in-oil emulsions (Salonen, 2020). However, to the best of our knowledge, a foamulsion where the continuous phase is composed of W/W emulsions has never been reported. We took the advantage of the high stability of the W/W emulsions developed here to study the possibility of producing a foamulsion by using dextran-in-PEG emulsion as the continuous phase of the foam. First, we prepared a dextran-in-PEG emulsion (10 wt% of dextran and 10 wt% of PEG) stabilized with LG at a concentration of 12 mM and with a molar ratio of decanol: LG equal to 3:1. Then, the double-syringe technique was used to produce the foams (Gaillard et al., 2017). First, we measured the foamability, that is to say the quantity of foam produced at the end of the foaming process. Then, the stability of the foams was followed by measuring both the evolution of the foam volume and the volume of drained liquid over time with the naked eye (Supplementary Figure S8).
Production of foam from the W/W emulsion stabilized by lamellar structures formed by APGs and fatty alcohols without the addition of additional surfactants was not possible (Supplementary Figure S8A). Then, we tested the addition of surfactant inside the W/W emulsion and we studied the resulting foaming properties. With the addition of DG at a concentration of 18 mM, no foam was produced (Supplementary Figure S8B). However, in the presence of SDS at a concentration of 18 mM, a high quantity of foam which was relatively stable with time was obtained (Figure 7A). By fluorescence microscopy, the presence of droplets surrounding the air bubbles were clearly distinguished similar to the ones observed for foamulsion based on oil-in-water emulsion (Figure 7B) (Salonen, 2020). The initial foam volume was around 10.5 mL showing the good foamability of the foamulsion. Then, the foam volume decreased slightly in the first 4 h to reach a foam volume around 7 mL associated with drainage (liquid volume inside the foam around 1 mL after 4 h). After 24 h, no more foam was present only few bubbles remained (Figure 7A and Supplementary Figure S9). We performed control experiments by producing foams with only dextran and SDS or only PEG and SDS at the same concentration (18 mM). The foamulsion stabilized by mixture of fatty alcohols and APGs with 18 mM of SDS was observed to be much more stable than the foams produced with only dextran or only PEG since these controlled foams disappeared after 2–3 h (Supplementary Figures S10A, B). In the same way, no more foam was present after 3 h when foam was produced directly from the pure W/W emulsion not stabilized by fatty alcohols and APGs and with only SDS (Supplementary Figure S10C).
FIGURE 7. (A) Stability of the foamulsion. Pictures of the foam produced with the W/W emulsion (LG 12 mM, decanol: LG ratio 3:1) with the addition of SDS at 18 mM and its evolution over time. (B) Epifluorescence microscopy image of the foamulsion at t = 0. Droplets are labeled with FITC-dextran dye. The scale bar represents 30 μm.
In this study, we demonstrated that W/W emulsions can be efficiently stabilized by mixtures of APGs and fatty alcohol. The stability of the W/W emulsions is linked to the APGs concentration and the molar ratio between the APG and the fatty alcohol. We demonstrated the robustness of this system by using various APGs: decyl glucoside, lauryl glucoside and coco-glucoside, and also by using fatty alcohols with different alkyl chain lengths (decanol, dodecanol and myristyl alcohol). An effect of the APGs alkyl chain length between DG and LG was observed, since the use of LG made it possible to produce emulsions with the same droplet size as with DG but at a lower concentration. In addition, emulsions prepared with LG were more stable in the long-term than emulsions prepared with DG. The molar ratio between the fatty alcohol and the APG is also a key parameter governing the stability. We hypothesized that the mixture of fatty alcohols and APGs led to the formation of LGNs stabilizing the W/W emulsions due to their presence both in the bulk phase and surrounding the droplets. The structure of the LGNs seems to depend on the molar ratio and APG concentration in the same way already described in aqueous solution (Terescenco et al., 2018a; Terescenco et al., 2018b).
Altogether, this confirms that stabilization of such aqueous droplets occurs when the chemicals (and eventually their assemblies) used for this task have an equivalent affinity for both the polymer within droplets and the polymer in the continuous phase. We believe that adjusting the concentrations and molar ratio control this affinity and affords chemicals to come and stay at the droplet interface, further preventing droplet coalescence. Further investigations using freeze-Fracture transmission electron microscopy and small angle neutron scattering are planned to better characterize the self-assembled structures formed both in the bulk and at the interface, and to confirm the formation of LGNs.
With preliminary results, we showed for the first time that the production of a foam based on W/W emulsion is possible; and that its stability is better than the foam produced with the polymers alone or with polymers in mixture without being stabilized by self-assembled structures formed by APGs and fatty alcohols. The inclusion of a third phase to emulsions could be a promising strategy to expand the use of W/W emulsions in various fields (Luengo et al., 2021; Zhili, 2022). Our results offer the possibility to formulate W/W emulsions based on chemical components widely used at industrial scale and at low cost associated with an easy and simple formulation process. Our approach could be extended to more complicated systems such as multiple water-in-water emulsion systems, and they could be used for encapsulation, drug delivery, as micro reactors, etc. (Solans et al., 2003; Singh et al., 2018; Michaux et al., 2021).
The original contributions presented in the study are included in the article/Supplementary Material, further inquiries can be directed to the corresponding author.
CD: Conceptualization, Formal Analysis, Investigation, Methodology, Validation, Writing–original draft, Writing–review and editing. YS: Investigation, Methodology, Writing–review and editing. J-PD: Conceptualization, Methodology, Writing–review and editing. J-FT: Investigation, Writing–review and editing. TB: Formal Analysis, Writing–review and editing. PSC: Conceptualization, Investigation, Resources, Supervision, Writing–review and editing. A-LF: Conceptualization, Funding acquisition, Investigation, Methodology, Project administration, Supervision, Writing–original draft, Writing–review and editing.
The authors declare financial support was received for the research, authorship, and/or publication of this article. This research was funded by the Region Hauts de France and the INRAe TRANSFORM department. This study was also supported by the French government through the Program “Investissements d’avenir” (I-SITE ULNE/ANR-16-IDEX-0004 ULNE) managed by the National Research Agency.
We acknowledge financial support from INRAe, TRANSFORM department, and region Hauts de France for the PhD grant of CD. CD would like to thank “l’École internationale de recherche d’Agreenium (EIR-A)” and the graduate program “Science for a Changing Planet” for the allocation of her travel grant to University of Edingburgh. Chevreul Institute (FR 2638), Ministère de l’Enseignement Supérieur, de la Recherche et de l’Innovation, Région Hauts de France and FEDER are acknowledged for SAXS facilities.
The authors declare that the research was conducted in the absence of any commercial or financial relationships that could be construed as a potential conflict of interest.
The authors declared that they were an editorial board member of Frontiers, at the time of submission. This had no impact on the peer review process and the final decision.
All claims expressed in this article are solely those of the authors and do not necessarily represent those of their affiliated organizations, or those of the publisher, the editors and the reviewers. Any product that may be evaluated in this article, or claim that may be made by its manufacturer, is not guaranteed or endorsed by the publisher.
The Supplementary Material for this article can be found online at: https://www.frontiersin.org/articles/10.3389/frsfm.2023.1328195/full#supplementary-material
Aguirre, T. A. S., Rosa, M., Guterres, S. S., Pohlmann, A. R., Coulter, I., and Brayden, D. J. (2014). Investigation of coco-glucoside as a novel intestinal permeation enhancer in rat models. Eur. J. Pharm. Biopharm. 88, 856–865. doi:10.1016/j.ejpb.2014.10.013
Antonov, Y. A., Van Puyvelde, P., and Moldenaers, P. (2004). Interfacial tension of aqueous biopolymer mixtures close to the critical point. Int. J. Biol. Macromol. 34, 29–35. doi:10.1016/j.ijbiomac.2004.01.001
Ben Ayed, E., Cochereau, R., Dechancé, C., Capron, I., Nicolai, T., and Benyahia, L. (2018). Water-in-water emulsion gels stabilized by cellulose nanocrystals. Langmuir 34, 6887–6893. doi:10.1021/acs.langmuir.8b01239
Chao, Y., and Shum, H. C. (2020). Emerging aqueous two-phase systems: from fundamentals of interfaces to biomedical applications. Chem. Soc. Rev. 49, 114–142. doi:10.1039/C9CS00466A
Chu, Z., Dreiss, C. A., and Feng, Y. (2013). Smart wormlike micelles. Chem. Soc. Rev. 42, 7174–7203. doi:10.1039/C3CS35490C
Colafemmina, G., Palazzo, G., Mateos, H., Amin, S., Fameau, A.-L., Olsson, U., et al. (2020). The cooling process effect on the bilayer phase state of the CTAC/cetearyl alcohol/water surfactant gel. Colloids Surfaces A Physicochem. Eng. Aspects 597, 124821. doi:10.1016/j.colsurfa.2020.124821
Coudon, N., Navailles, L., Nallet, F., Ly, I., Bentaleb, A., Chapel, J.-P., et al. (2022). Stabilization of all-aqueous droplets by interfacial self-assembly of fatty acids bilayers. J. Colloid Interface Sci. 617, 257–266. doi:10.1016/j.jcis.2022.02.138
Dari, C., Dallagi, H., Faille, C., Dubois, T., Lemy, C., Deleplace, M., et al. (2023). Decontamination of spores on model stainless-steel surface by using foams based on alkyl polyglucosides. Molecules 28, 936. doi:10.3390/molecules28030936
de Oliveira, T. E., Leonforte, F., Nicolas-Morgantini, L., Fameau, A.-L., Querleux, B., Thalmann, F., et al. (2020). Fluid bilayer phase in aqueous mixtures of fatty alcohol and cationic surfactant. Phys. Rev. Res. 2, 013075. doi:10.1103/PhysRevResearch.2.013075
Dewey, D. C., Strulson, C. A., Cacace, D. N., Bevilacqua, P. C., and Keating, C. D. (2014). Bioreactor droplets from liposome-stabilized all-aqueous emulsions. Nat. Commun. 5, 4670. doi:10.1038/ncomms5670
Diamond, A. D., and Hsu, J. T. (1989). Phase diagrams for dextran-PEG aqueous two-phase systems at 22°C. Biotechnol. Tech. 3, 119–124. doi:10.1007/BF01875564
Dickinson, E. (2019). Particle-based stabilization of water-in-water emulsions containing mixed biopolymers. Trends Food Sci. Technol. 83, 31–40. doi:10.1016/j.tifs.2018.11.004
Douliez, J.-P., Martin, N., Beneyton, T., Eloi, J.-C., Chapel, J.-P., Navailles, L., et al. (2018). Preparation of swellable hydrogel-containing colloidosomes from aqueous two-phase pickering emulsion droplets. Angew. Chem. Int. Ed. Engl. 57, 7780–7784. doi:10.1002/anie.201802929
Douliez, J.-P., Perro, A., and Béven, L. (2019). Stabilization of all-in-water emulsions to form capsules as artificial cells. Chembiochem 20, 2546–2552. doi:10.1002/cbic.201900196
Eccleston, G. M. (1997). Functions of mixed emulsifiers and emulsifying waxes in dermatological lotions and creams. Colloids Surfaces A Physicochem. Eng. Aspects 123 (124), 169–182. doi:10.1016/S0927-7757(96)03846-0
Esquena, J. (2016). Water-in-water (W/W) emulsions. Curr. Opin. Colloid & Interface Sci. 25, 109–119. doi:10.1016/j.cocis.2016.09.010
Esquena, J. (2023). Recent advances on water-in-water emulsions in segregative systems of two water-soluble polymers. Curr. Opin. Food Sci. 51, 101010. doi:10.1016/j.cofs.2023.101010
Gaillard, T., Honorez, C., Jumeau, M., Elias, F., and Drenckhan, W. (2015). A simple technique for the automation of bubble size measurements. Colloids Surfaces A Physicochem. Eng. Aspects 473, 68–74. doi:10.1016/j.colsurfa.2015.01.089
Gaillard, T., Roché, M., Honorez, C., Jumeau, M., Balan, A., Jedrzejczyk, C., et al. (2017). Controlled foam generation using cyclic diphasic flows through a constriction. Int. J. Multiph. Flow 96, 173–187. doi:10.1016/j.ijmultiphaseflow.2017.02.009
Iwata, T. (2017). “Lamellar gel network,” in Cosmetic science and technology (Netherlands: Elsevier), 415–447. doi:10.1016/B978-0-12-802005-0.00025-2
Junginger, H. E. (1984). Colloidal structures of O/W creams. Pharm. Weekbl. Sci. Ed. 6, 141–149. doi:10.1007/BF01954041
Keck, C. M., Kovačević, A., Müller, R. H., Savić, S., Vuleta, G., and Milić, J. (2014). Formulation of solid lipid nanoparticles (SLN): the value of different alkyl polyglucoside surfactants. Int. J. Pharm. 474, 33–41. doi:10.1016/j.ijpharm.2014.08.008
Leal-Calderon, F., Bibette, J., and Schmitt, V. (2007). Emulsion science: basic principles. New York, NY: Springer New York. doi:10.1007/978-0-387-39683-5
Luengo, G. S., Fameau, A.-L., Léonforte, F., and Greaves, A. J. (2021). Surface science of cosmetic substrates, cleansing actives and formulations. Adv. Colloid Interface Sci. 290, 102383. doi:10.1016/j.cis.2021.102383
Merland, T., Waldmann, L., Guignard, O., Tatry, M.-C., Wirotius, A.-L., Lapeyre, V., et al. (2022). Thermo-induced inversion of water-in-water emulsion stability by bis-hydrophilic microgels. J. Colloid Interface Sci. 608, 1191–1201. doi:10.1016/j.jcis.2021.10.074
Michaux, M., Salinas, N., Miras, J., Vílchez, S., González-Azón, C., and Esquena, J. (2021). Encapsulation of BSA/alginate water–in–water emulsions by polyelectrolyte complexation. Food Hydrocoll. 113, 106406. doi:10.1016/j.foodhyd.2020.106406
Möller, A., Lang, P., Findenegg, G. H., and Keiderling, U. (1998). Location of butanol in mixed micelles with alkyl glucosides studied by SANS. J. Phys. Chem. B 102, 8958–8964. doi:10.1021/jp981819q
Murray, B. S. (2020). Recent developments in food foams. Curr. Opin. Colloid & Interface Sci. 50, 101394. doi:10.1016/j.cocis.2020.101394
Nakarapanich, J., Barameesangpet, T., Suksamranchit, S., Sirivat, A., and Jamieson, A. M. (2001). Rheological properties and structures of cationic surfactants and fatty alcohol emulsions: effect of surfactant chain length and concentration. Colloid Polym. Sci. 279, 671–677. doi:10.1007/s003960000470
Nguyen, B. T., Nicolai, T., and Benyahia, L. (2013). Stabilization of water-in-water emulsions by addition of protein particles. Langmuir 29, 10658–10664. doi:10.1021/la402131e
Nicolai, T., and Murray, B. (2017). Particle stabilized water in water emulsions. Food Hydrocoll. 68, 157–163. doi:10.1016/j.foodhyd.2016.08.036
Parsa, M., Trybala, A., Malik, D. J., and Starov, V. (2019). Foam in pharmaceutical and medical applications. Curr. Opin. Colloid Interface Sci. 44, 153–167. doi:10.1016/j.cocis.2019.10.007
Platz, G., Poelike, J., Thunig, C., Hofmann, R., Nickel, D., and von Rybinski, W. (1995). Phase behavior, lyotropic phases, and flow properties of alkyl glycosides in aqueous solution. Langmuir 11, 4250–4255. doi:10.1021/la00011a015
Poortinga, A. T. (2008). Microcapsules from self-assembled colloidal particles using aqueous phase-separated polymer solutions. Langmuir 24, 1644–1647. doi:10.1021/la703441e
Salonen, A. (2020). Mixing bubbles and drops to make foamed emulsions. Curr. Opin. Colloid & Interface Sci. 50, 101381. doi:10.1016/j.cocis.2020.08.006
Savic, S., Vuleta, G., Daniels, R., and Muller-Goymann, C. C. (2005). Colloidal microstructure of binary systems and model creams stabilized with an alkylpolyglucoside non-ionic emulsifier. Colloid Polym. Sci. 283, 439–451. doi:10.1007/s00396-004-1174-4
Singh, P., Medronho, B., Miguel, M. G., and Esquena, J. (2018). On the encapsulation and viability of probiotic bacteria in edible carboxymethyl cellulose-gelatin water-in-water emulsions. Food Hydrocoll. 75, 41–50. doi:10.1016/j.foodhyd.2017.09.014
Solans, C., Esquena, J., and Azemar, N. (2003). Highly concentrated (gel) emulsions, versatile reaction media. Curr. Opin. Colloid & Interface Sci. 8, 156–163. doi:10.1016/S1359-0294(03)00021-9
Stradner, A., Glatter, O., and Schurtenberger, P. (2000). A hexanol-induced sphere-to-flexible cylinder transition in aqueous alkyl polyglucoside solutions. Langmuir 16, 5354–5364. doi:10.1021/la991679r
Terescenco, D., Picard, C., Clemenceau, F., Grisel, M., and Savary, G. (2018a). Influence of the emollient structure on the properties of cosmetic emulsion containing lamellar liquid crystals. Colloids Surfaces A Physicochem. Eng. Aspects 536, 10–19. doi:10.1016/j.colsurfa.2017.08.017
Terescenco, D., Savary, G., Clemenceau, F., Merat, E., Duchemin, B., Grisel, M., et al. (2018b). The alkyl polyglucoside/fatty alcohol ratio effect on the formation of liquid crystal phases in binary systems. J. Mol. Liq. 253, 45–52. doi:10.1016/j.molliq.2017.12.149
Wu, P.-S., Lin, C.-H., Kuo, Y.-C., and Lin, C.-C. (2017). Formulation and characterization of hydroquinone nanostructured lipid carriers by homogenization emulsification method. J. Nanomater. 2017, 1–7. doi:10.1155/2017/3282693
Xie, Y., Ruan, M., Zhang, J., Kibtia, M., Li, Y., Li, B., et al. (2023). Water-in-water Pickering emulsion stabilized by cellulose nanocrystals as space-confined encapsulating systems: from establishment to stability. Food Hydrocoll. 141, 108719. doi:10.1016/j.foodhyd.2023.108719
You, K.-M., Murray, B. S., and Sarkar, A. (2023). Tribology and rheology of water-in-water emulsions stabilized by whey protein microgels. Food Hydrocoll. 134, 108009. doi:10.1016/j.foodhyd.2022.108009
Zhang, J., Xie, Y., Liu, C., Cao, H., Li, Y., Li, B., et al. (2024). Water-in-water Pickering emulsion: a fascinating microculture apparatus for embedding and cultivation of Lactobacillus helveticus. Food Hydrocoll. 147, 109398. doi:10.1016/j.foodhyd.2023.109398
Zheng, R., Hu, X., Su, C., Jiang, J., Cui, Z., and Binks, B. P. (2022). Edible oil-water foamulsions stabilized by vesicle network of sucrose ester. J. Mol. Liq. 371, 121066. doi:10.1016/j.molliq.2022.121066
Keywords: emulsion, lamellar phase, fatty alcohol, alkyl polyglucoside, foam
Citation: Dari C, Si Y, Douliez J-P, Tahon J-F, Benezech T, Clegg PS and Fameau A-L (2023) Mixture of fatty alcohols and alkyl polyglucosides stabilizing water-in-water emulsions. Front. Soft Matter 3:1328195. doi: 10.3389/frsfm.2023.1328195
Received: 26 October 2023; Accepted: 17 November 2023;
Published: 30 November 2023.
Edited by:
Ali Miserez, Nanyang Technological University, SingaporeReviewed by:
Erica Pensini, University of Guelph, CanadaCopyright © 2023 Dari, Si, Douliez, Tahon, Benezech, Clegg and Fameau. This is an open-access article distributed under the terms of the Creative Commons Attribution License (CC BY). The use, distribution or reproduction in other forums is permitted, provided the original author(s) and the copyright owner(s) are credited and that the original publication in this journal is cited, in accordance with accepted academic practice. No use, distribution or reproduction is permitted which does not comply with these terms.
*Correspondence: Anne-Laure Fameau, QW5uZS1sYXVyZS5mYW1lYXVAaW5yYWUuZnI=
Disclaimer: All claims expressed in this article are solely those of the authors and do not necessarily represent those of their affiliated organizations, or those of the publisher, the editors and the reviewers. Any product that may be evaluated in this article or claim that may be made by its manufacturer is not guaranteed or endorsed by the publisher.
Research integrity at Frontiers
Learn more about the work of our research integrity team to safeguard the quality of each article we publish.