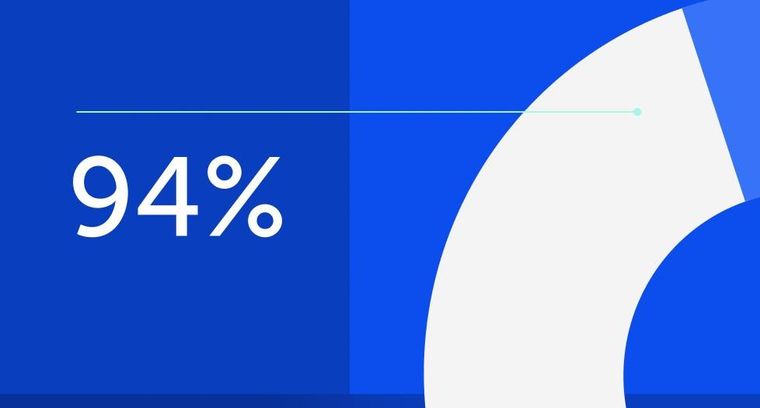
94% of researchers rate our articles as excellent or good
Learn more about the work of our research integrity team to safeguard the quality of each article we publish.
Find out more
MINI REVIEW article
Front. Soft Matter, 14 September 2023
Sec. Colloids and Emulsions
Volume 3 - 2023 | https://doi.org/10.3389/frsfm.2023.1260211
This article is part of the Research TopicCelebrating 1 Year of Frontiers in Soft MatterView all 5 articles
In the context of a more sustainable economy, bio-surfactants become increasingly important, due to their independence of petrol-based chemistry, their usually mild synthesis conditions, and in certain cases their pharmacological activity. We have recently discussed self-assembly studies in binary systems of bio-surfactants of microbial origin, or saponins extracted from plants (Hellweg et al., Frontiers in Soft Matter, 2023, 2). In the present review, we focus on the formation of microemulsions based on these molecules. We review the formation and structure of microemulsion systems formed by oil, water, and biosurfactants, with a particular focus on Quillaja saponins and rhamnolipids.
Since microemulsions are thermodynamically stable, they are spontaneously formed upon mixing water, oil, surfactant and in some cases a co-surfactant (Hoar and Schulman, 1943; Strey et al., 1994). Microemulsions are clear, isotropic, and homogeneous liquids exhibiting a nanostructure of polar (water) and nonpolar (oil) domains separated by a surfactant layer. The formation of such nanostructures is governed by the combination of constraints of available surface to be covered by surfactant, the volume of each phase, and the interfacial curvature. Resulting nanostructures range from oil-in-water droplets to inverse water-in-oil droplets. The continuous path between both passes through bicontinuous microemulsions and is called the Shinoda cut in the phase diagram (Shinoda and Kunieda, 1973; Olsson et al., 1986). Applications of microemulsions are in particular related to the solubilization of hydrophobic molecules (detergency, drug delivery, … ) (Schwuger et al., 1995; Solans and Kunieda, 1997).
At present most microemulsions are made by using synthetic surfactants based on petrolchemistry. Biosurfactants, i.e., surfactants from natural resources, represent an attractive alternative, in particular in the context of sustainability. These molecules possess a high degree of chemical diversity, and many different classes exist according the presence of chemical groups, like phospholipids, glycolipids (in particular rhamnolipids discussed here), or lipopeptides (Bezerra et al., 2018). These molecules are usually produced under mild synthesis conditions in bioreactors for microbial biosurfactants (yeast or bacteria) or by extraction for plant biosurfactants (plant saponins). Other advantages of biosurfactants are their (often) lower toxicity and increased biocompatibility, and sometimes their pharmacological activity, which may be antiviral (Bailly and Vergoten, 2020; Bailly and Vergoten, 2020; Bailly and Vergoten, 2020) or antimicrobial, leading to growing interest in their large-scale production (Kitamoto et al., 2002; Kitamoto et al. 2002; Kitamoto et al. 2002).
Surface activity is the main characteristic of surfactant molecules, which leads to a decrease of the air-water interfacial tension. For example, the high surface activity of microbial surfactin has been known since the 1960s (Fracchia et al., 2012; Lewinska et al., 2022). On the other hand, the self-assembly properties of biosurfactants lead to the formation of supramolecular aggregates in selective solvents. The self-assembly of such molecules has been reviewed by us (Hellweg et al., 2023), and here we wish to extend this review to the formation of microemulsions with biosurfactants. The size and shape of the structures formed on the nanoscale depend mainly on the surfactants molecular architecture: In particular, the spontaneous curvature of the hydrophilic-hydrophobic interface is the result of opposing forces acting on each moiety: the attractive hydrophobic effect for the tails, and the head group repulsion for the hydrophilic head groups. Ideally, molecules adopt an amphiphilic film curvature corresponding to these interactions, but other constraints like the available surfaces or volumes of each phase may then not be met, and energetic compromises need to be adopted. There is a cost for deviations from the spontaneous curvature, called the bending energy, and the most successful model has been proposed by Helfrich exactly 50 years ago (Helfrich, 1973). Available shapes of the oil or water domains in microemulsions are thus subjected to these free energy contributions.
In this short review we address the formation of microemulsions of saponins and microbial surfactants, in particular rhamnolipids. To tune the curvature of biosurfactant-containing amphiphilic films, synthetic surfactants or hydrophobic alcohols were often added. We also briefly discuss emulsions stabilized by biosurfactants, as they could be a useful application of the more readily degradable biosurfactants, given the limited shelf life of most industrial products. Saponin-based microemulsions are exemplarily discussed in Section 2 for Quillaja saponins, while we have focused on rhamnolipids in the third section. In Section 4, some microemulsions stabilized by other biosurfactants are discussed.
Saponins are biosurfactants which can be extracted from plants, like, e.g., horse chestnuts (used to produce aescin), licorice (glycyrrhizin), soapbark tree Quillaja Saponaria, common foxglove (digitonin), oleander (oleandrin), or Saponaria Oficinalis (gypsogenin). These molecules participate in the defense against invasion by fungi and bacteria. Due to their molecular amphiphilicity based on hydrophobic parts having a triterpenic or steroidic structure with attached sugar moieties, they possess foaming and emulsifying properties. This is for example, the case of Quillaja bark saponins which have been used for washing processes, and their general properties and specifically their interfacial behaviour have been described, see for example, (Nord and Kenne, 1999; Wojciechowski, 2013; Wojciechowski et al., 2014; Góral and Wojciechowski, 2020). These saponins have a large structural variety, which impacts their surface activity (Kezwon and Wojciechowski, 2014). In water, they form micelles above a certain concentration, the critical micelle concentration (CMC) (Dargel et al., 2019; Geisler et al., 2019). Nowadays, they are targeted as molecules with potential cosmetic or pharmacological activity (Herzog et al., 2020; Wojciechowski et al., 2021). Regarding the separation and purification of surface-active saponins from multicomponent plant extracts, Obasi et al., (2017) reported an approach reminiscent of the three-phase extraction procedure proposed for the purification of nonionic alkyl polyglycol ethers, in which water- and oil-soluble impurities were extracted into the water and oil excess phases of a Winsor III system (Schubert et al., 1990). Similarly, saponins from the defatted root extract of Securidaca longipedunculata were first dispersed in water to self-assemble into micelles. They report that the addition of ethyl ether initially swells these micelles, and upon further addition causes a phase inversion to a Winsor II type system. The purified saponin was then extracted from the upper ethyl ether-rich phase via three additional purification cycles, while polar impurities were separated in the lower aqueous phase.
Applications of Quillaja saponin-based microemulsions have been proposed in the literature. These mixtures, however, do not always form thermodynamically stable microemulsions. Often, a strong energy input like high pressure homogenization is necessary to form emulsions with relatively unstable droplets in the micro to millimeter range. And sometimes more stable nanoemulsions are formed, where stability before coalescence lasts longer due to the small nanometric droplet size. In spite of the lack of thermodynamic stability, we discuss three articles here which pave the way to “all natural” microemulsions for food and drug incorporation. Schober et al. studied the efficiency of Quillaja saponins to emulsify limonene and alkanes by determining the droplet diameters of mostly bimodal emulsions with a particle sizer (Schober et al., 2017). These studies showed that Quillaja saponins have a significantly higher emulsifying power than various conventional Tween surfactants under the same conditions. The headgroup area of 1.37 nm2 was found to be slightly larger than that determined by Wojciechowski (Wojciechowski, 2013) and suggests a lay-on configuration of Quillaja saponins in the internal interface of oil-in-water emulsions, where the hydrophobic triterpenoid rings of a saponin molecule reside in the interface and the hydrophilic glucoside tails protrude into the water. Another example is the incorporation of vitamin E, which refers to a fat-soluble group of molecules which have anti-oxydant properties. The transport in a drug carrier is also thought to increase the bioavailability on the intestinal sites. In the form of the more stable vitamin E acetate, which can then be broken down to vitamin E during digestion, the uptake of this lipophilic molecule into nano-emulsion droplets (nonequilibrium emulsion) containing food-grade oils (triglyceride) and surfactants, among which “(marketing) label friendly” saponins, has been studied by Yang and McClements (Yang and McClements, 2013). The formation of nano-emulsion droplets has been shown to be less efficient with saponins in comparison to different Tweens, in the sense that they remain rather macroscopic, with a strong impact on both stability and optical appearance (Mayer et al., 2013). In contrast to this, below the formation of a vitamin E-containing microemulsion stable over at least a month obtained with microbial biosurfactant will be discussed.
Apart from the different production mode, microbial biosurfactants have similar properties as saponins. Their hydrophobic moiety contains one or several branched fatty acid chains, and depending on the hydrophilic head group, they are categorized as phospholipids, lipopeptides, or glycolipids. The latter include threhalolipids, sophorolipids, mannosylerythritol lipids (Kim et al., 2002), or rhamnolipids which are produced by the bacterium Pseudomonas aeruginosa (Rodrigues, 2015; Jahan et al., 2020). Rhamnolipids have a strong surface activity, as they lower the surface tension of the water/air interface by more than a factor of two (Rodrigues, 2015), form micelles above a critical micelle concentration (CMC) and also favor the formation of bacterial biofilms. The potential of rhamnolipids as biodegradable and low-toxicity alternatives to synthetic surfactants for stabilizing microemulsions has been investigated since the beginning of the new millennium. Especially in the deprotonated form, rhamnolipids are quite hydrophilic, resulting in amphiphilic films that are strongly curved around the oil (o/w structures, Winsor I type emulsions). An efficient approach to form microemulsions with hydrophilic surfactants or even drive the system through the phase inversion is to add hydrophobic co-surfactants (Penders and Strey, 1995; Sottmann et al., 2002). In 2005, Ye et al. were among the first to systematically study the influence of alcohol chain length on the phase behavior of a mixture of water/NaOH, n-heptane, rhamnolipid and n-alcohols as co-surfactants (Xie et al., 2005). They used a 50/50 mixture by weight of mono-rhamnolipid and di-rhamnolipids and added sodium hydroxide to adjust a pH of 9 ensuring that the rhamnolipid was completely deprotonated and thus an anionic surfactant. The isothermally recorded Gibbs triangles (25°C) in which the rhamnolipid/n-alcohol mixture is considered as a pseudo-component show that the phase diagrams become more complex as the chain length of the n-alcohol is increased. From n-pentanol, liquid crystalline phases such as lamellar and hexagonal phases appeared, due to the increasing bending rigidity of the mixed amphiphilic film. In a follow-up paper (Xie et al., 2007), the influence of the water concentration on the microstructure was studied in a mixture of 25% n-heptane and 75% of a one-to-one mixture of n-butanol and rhamnolipid. Electrical conductivity showed that the structure reverses from a water-in-oil to an oil-in-water microemulsion upon increasing water concentration. Freeze-fracture electron microscopy (FFEM) images might show percolating w/o-droplets at 30 wt% water, while o/w-droplets might be imaged at 90% water. DLS studies performed with a zetasizer gave hydrodynamic radii of 6.2 and 19.3 nm, respectively.
Sabatini et al. studied the phase behavior and oil/water-interfacial tension of an alcohol-free symmetric microemulsion made of water/NaCl (brine), oil and the same 50/50 mixture of mono- and di-rhamnolipids at 23°C (Nguyen et al., 2008). For toluene as oil, they showed very nicely that at neutral pH the system undergoes a phase inversion from Winsor I to Winsor II when the NaCl concentration is increased and even passes a Winsor III region–where the microemulsion phase coexists with excess water and oil phases–at intermediate salt concentrations. Here, i.e., at the optimum salt concentration, the oil/water interfacial tension passes through a pronounced minimum of 10–5 Nm-1, as is the case for classical microemulsions (Salager et al., 1979; Sottmann and Strey, 1996). Fish-shaped phase boundaries, as known, e.g., from microemulsion systems with non-ionic surfactants (Kahlweit and Strey, 1985), are found when the surfactant concentration is plotted logarithmically against the salt concentration (Figure 1). With the aim of solubilizing hydrophobic oils such as limonene and diesel, they used mixtures of rhamnolipid and sodium bis(2-ethylhexyl) sulfosuccinate (AOT) in a follow-up work and investigated the phase behavior and oil/water interfacial tension of symmetric microemulsions (Nguyen and Sabatini, 2008). When using an equimolar mixture of the two surfactants, the oil/water interfacial tension was likewise found to pass through a pronounced minimum in the Winsor III region with increasing NaCl concentration. The addition of oleyl alcohol (referred to as a lipophilic linker) to the surfactant mixture resulted in a further decrease in the minimum. As a measure of the tendency to form o/w- or w/o-microemulsions, the characteristic curvature Cc (Acosta et al., 2008) of the rhamnolipid mixture used was determined to be Cc = −1.41. When compared to the Cc of AOT (Cc(AOT) = -0.92), this means that the rhamnolipid blend is slightly more hydrophilic than AOT. A year later, in order to formulate microemulsions without the synthetic surfactants, Sabatini et al. studied a combination of the more hydrophobic biosurfactant sophorolipid (in its nonionic lactone form) and soybean lecithin to compensate for the hydrophilicity of the rhamnolipid (Nguyen et al., 2010). The characteristic phase behavior of microemulsions, reflected in fish-shaped phase boundaries, was obtained when the rhamnolipid to soy lecithin + sophorolipid ratio is used to tune the curvature of the mixed amphiphilic film. In microemulsions with the hydrophobic isopropyl myristate (IPM), the optimal microemulsion (Winsor III and IV), was achieved by a small amount of rhamnolipid, whereas the optimal solubilization of the more hydrophilic limonene required more than twice the amount of rhamnolipid. In 2011, Nguyen and Sabatini provided an overview of microemulsions formulated with rhamnolipids and/or sophorolipids and discussed the use of such microemulsions in various applications (Nguyen and Sabatini, 2011).
FIGURE 1. The phase boundaries feature the “fish shape” well-known for classical microemulsion systems (rotated by 90° due to the choice of axes), with the phase inversion from Winsor I to Winsor II induced by an increase in the NaCl concentration. Figure adapted from ref. (Nguyen et al., 2008) with permission from Elsevier.
Guo et al. investigated the effect of styrene added to an aqueous rhamnolipid solution consisting of an equimolar ratio of mono- and di-rhamnolipid on aggregate morphology using SANS adjusting two contrasts (deuterated and hydrogenated styrene) (Guo et al., 2011). Analysis of the data recorded for the aqueous rhamnolipid solution showed that the cylindrical micelles formed at low concentration changed into a mixture of the latter with vesicles as the concentration increased. They rationalized this transition by the fact that the H+-ions released from the carboxyl group of the rhamnolipid lead to a decreasing pH at which they had observed the formation of unilamellar vesicles in a previous SANS study (Dahrazma et al., 2008). Similar transitions have been observed by SANS and electron microscopy in synthetic surfactant systems (Oberdisse et al., 1998).
Biosurfactant based microemulsions are found in a variety of different applications ranging from Diesel fuel via the use as reaction medium to food products. One example for making Diesel fuel from natural oils like, e.g., castor oil is discussed by Zhu et al. (Zhu et al., 2014). In this work rhamnolipid based microemulsions are used to obtain systems with lower viscosity compared to the original oil leading to less coking in the engine. Moreover, bio-based oil made by liquefaction of biomass is a promising alternative for petrol derived diesel fuel. However, these are usually very complex mixtures containing water and other rather polar compounds. The fuel properties can be improved by microemulsion-formation achieved by simple addition of surfactant. Leng has studied this microemulsification process using a rhamnolipid as micro-emulsifier (Leng, 2018). Unfortunately, the exact composition of the used rhamnolipid is not given. The same group has studied the influence of making biodiesel based microemulsions with small amounts of water and rhamnolipid on the so-called ignition delay (Leng et al., 2022). Biodiesel/rhamnolipid/water microemulsion are found to have a positive effect on the ignition delay and moreover are found to reduce NOx emissions. Hence, such rhamnolipid based biodiesel microemulsions are a more sustainable alternative for conventional Diesel fuel. Besides for Diesel fuel, rhamnolipids can also be used to treat crude oil (Hajimohammadi & Johari-ahar 2017). However, the shown images in this publication indicated that only a crude oil emulsion was made and not a microemulsion, implying that the mixture is not at thermal equilibrium and would sooner or later demix.
Rhamnolipids exhibit structural similarities to AOT and are therefore rather well suited for the stabilization of oil-continuous microemulsions (see above). Moya-Ramirez and co-workers have produced rhamnolipid-based oil continuous microemulsions (Moya-Ramirez et al., 2017). These microemulsions are used as reaction media for the enzyme catalyzed conversion of used frying oil. The water-in-oil microemulsion system under study contained lipase as catalyst. Compared to the AOT based systems the degree of hydrolysis was 35% higher, which the authors explain by a better compatibility of the lipase and the rhamnolipid in comparison to the synthetic surfactant. Another example for the use of an oil-continuous rhamnolipid-based microemulsion as reaction medium has been given by Palanisamy and Raichur (Palanisamy and Raichur, 2009). These authors made NiO nanoparticles in a n-heptane/rhamnolipid/water microemulsion. However, as in most studies using oil-continuous microemulsions, no real templating effect of the microemulsion could be proven. The resulting nanoparticles are rather polydisperse and large (micron range). Unfortunately, the authors have not studied the microemulsion droplet sizes. An application of microemulsions in food industry has been described in 2017 by Amiri-Rigi and Abbasi (Amiri-Rigi and Abbasi, 2017). In this work 4 synthetic and 4 bio-surfactants were used to make microemulsions for the extraction of lycopene from tomato processing residuals. The used biosurfactants are lecithin, rhamnolipid, saponin, and sucrose monopalmitate. Lycopene is an interesting compound with strong anti-oxidant activity which can, e.g., be used in skin care products. It is fat soluble and therefore, microemulsions are an interesting extraction medium for it. In the work by Amiri-Rigi and Abbasi only droplet sizes measured by using a zetasizer are presented. No deeper characterization of the structures has been done and therefore the structures obtained by using rhamnolipid and saponin might be vesicles and not microemulsion droplets. However, the extraction process is found to be rather efficient and the lycopene is stabilized by all used surfactants. In this case, e.g., small angle scattering techniques might yield more information about the resulting structures.
Besides saponins and glycolipid biosurfactants such as rhamnolipids and sophorolipids, also mannosylerythritolipids (MELs), which are abundantly produced by yeast strains of the genus Pseudozyma from renewable resources, were successfully used to stabilize microemulsions. Worakitkanchanakul et al. found that the diacetylated MEL-A on its own is capable of forming water-in-n-decane microemulsions (Worakitkanchanakul et al., 2008). Using DLS, they showed that the diameter of the microemulsion droplets increases from 20 to 60 nm when the ratio of water to surfactant is increased (Figure 2left), as anticipated. This increase in droplet diameter has also been imaged using freeze-fracture electron microscopy (FFEM) (Figure 2, right). Note, however, that the diameters obtained are large for microemulsions, especially given the rather small w0 ratios (Foster et al., 2008). Note that in the DLS analysis the sample viscosity is used instead of n-decane and that FFEM is prone to artifacts. Much more suitable methods to assess the size and shape of the formed structures would be SAXS and SANS. In a follow-up paper, the same group studied the phase behavior of ternary microemulsions consisting of water, n-decane and MEL-A and the monacetylated MEL-B, respectively (Worakitkanchanakul et al., 2009). The Gibbs phase triangles recorded by polarized optical microscopy and SAXS at 25°C show that at high biosurfactant concentrations the phase diagram of the MEL-A system is dominated by a water-in-n-decane microemulsion, while a lamellar phase is formed in the MEL-B system. These results agree with the phase diagrams of the respective aqueous binary systems summarized by Kitamoto et al. (Kitamoto et al., 2009). While the spontaneous curvature of MEL-A was found to be slightly negative, leading to bilayers forming sponge (L3) and inverted bicontinuous cubic (V2) phases, the spontaneous curvature of MEL-B is almost zero, resulting in the formation of vesicles and/or the lamellar (Lα) phase.
FIGURE 2. DLS diameter and FFEM pictures of w/o-microemulsion droplets formed in the system water/n-decane/MEL-A with increasing molar ratio w0 of water-to-MEL-A [from w0 = 1.5 (A), 8.3 (B) and 14.3 (C)] at constant molar n-decane-to-MEL-A ratio of 4.8 at T = 25°C. Figure reprinted from ref (Worakitkanchanakul et al., 2008) with permission from the Japan Oil Chemists’ Society.
Coming back to the incorporation of vitamin E for patients having intake problems, a possible solution is the use of vitamin E microemulsions. In recent work by Kouchi and co-workers such a microemulsion is made by using a crude biosurfactant from Yarrowia lipolytica (Kouchi et al., 2022). The authors present data with droplet sizes below 100 nm, and stability of at least a month. The “crude” biosurfactant mix of microbial origin appears to be better suited than saponins for this purpose, but it is unclear if this is the result of chemical variety, or the precise molecular structure.
We have reviewed recent progress in the formation of biosurfactant-water-oil microemulsions. One conclusion of our previous mini-review was that the study of biosurfactants from a physical-chemical point of view is still in a rather early stage (Hellweg et al., 2023). This is even more true in the case of biosurfactant-based microemulsions. There is considerable interest in applications, but a lack of systematic and quantitative studies on their properties and especially on the microstructures formed by the amphiphilic biosurfactant film. We therefore recommend a strong focus on structural studies, especially using scattering techniques. The latter are often missing in the literature, although they provide robust results even in complex mixtures. In-house DLS and SAXS are suitable and easily accessible scattering techniques. However, the contrast in electron density, and thus the SAXS intensity, is often limited because typical microemulsion components, as well as the biosurfactants, are composed of H, C, N, and O. Most promising for elucidating complex aggregate morphologies stabilized by mixtures of co-surfactants and biosurfactants is SANS and, in particular, contrast variation SANS using deuteration as demonstrated, for example, for microemulsions stabilized by alcohol/surfactant or amphiphilic diblock copolymer/surfactant mixtures (Endo et al., 2001; Bumajdad et al., 2003). Further complementary diffusion NMR and electrical conductivity measurements that probe the continuity of a structure and the study of the dynamic behavior by neutron spin echo will help to elucidate the properties of these promising new microemulsion systems (Hellweg et al., 2001; Reimer et al., 2003). As far as systems are concerned, the “royal path” would be to work with highly purified biosurfactants in order to connect nanostructures to molecular features–probably slowing down progress in an exaggerated manner. We would thus recommend to work with (chemically) polydisperse systems first, and invest energy in purifications only if promising enough. In conclusion, we anticipate biosurfactants to be of growing importance in progressive replacement and improvement of synthetic surfactants by sustainable and biodegradable molecules in many applications, from pharmacology via fuels to detergency.
TH: Writing–original draft, Writing–review and editing. JO: Conceptualization, Writing–original draft, Writing–review and editing. TS: Conceptualization, Writing–original draft, Writing–review and editing.
The author(s) declare that no financial support was received for the research, authorship, and/or publication of this article.
The authors declare that the research was conducted in the absence of any commercial or financial relationships that could be construed as a potential conflict of interest.
The author(s) declared that they were an editorial board member of Frontiers, at the time of submission. This had no impact on the peer review process and the final decision
All claims expressed in this article are solely those of the authors and do not necessarily represent those of their affiliated organizations, or those of the publisher, the editors and the reviewers. Any product that may be evaluated in this article, or claim that may be made by its manufacturer, is not guaranteed or endorsed by the publisher.
Acosta, E. J., Yuan, J. S., and Bhakta, A. S. (2008). The characteristic curvature of ionic surfactants. J. Surfactants Deterg. 11 (2), 145–158. doi:10.1007/s11743-008-1065-7
Amiri-Rigi, A., and Abbasi, S. (2017). Stability assessment of lycopene microemulsion prepared using tomato industrial waste against various processing conditions. J. Sci. Food Agric. 97 (14), 4922–4928. doi:10.1002/jsfa.8368
Bailly, C., and Vergoten, G. (2020). Glycyrrhizin: an alternative drug for the treatment of COVID-19 infection and the associated respiratory syndrome? Pharmacol. & Ther. 214, 107618. doi:10.1016/j.pharmthera.2020.107618
Bezerra, K. G. O., Rufino, R. D., Luna, J. M., and Sarubbo, L. A. (2018). Saponins and microbial biosurfactants: potential raw materials for the formulation of cosmetics. Biotechnol. Prog. 34 (6), 1482–1493. doi:10.1002/btpr.2682
Bumajdad, A., Eastoe, J., and Heenan, R. K. (2003). Properties of mixed alcohol-zwitterionic surfactant films in quaternary water-in-oil microemulsions. Langmuir 19 (18), 7219–7225. doi:10.1021/la034496k
Dahrazma, B., Mulligan, C. N., and Nieh, M.-P. (2008). Effects of additives on the structure of rhamnolipid (biosurfactant): A small-angle neutron scattering (SANS) study. J. Colloid Interface Sci. 319 (2), 590–593. doi:10.1016/j.jcis.2007.11.045
Dargel, C., Geisler, R., Hannappel, Y., Kemker, I., Sewald, N., and Hellweg, T. (2019). Self-assembly of the bio-surfactant aescin in solution: A small-angle x-ray scattering and fluorescence study. Colloids Interfaces 3 (2), 47. doi:10.3390/colloids3020047
Endo, H., Mihailescu, M., Monkenbusch, M., Allgaier, J., Gompper, G., Richter, D., et al. (2001). Effect of amphiphilic block copolymers on the structure and phase behavior of oil-water-surfactant mixtures. J. Chem. Phys. 115 (1), 580–600. doi:10.1063/1.1377881
Foster, T., Sottmann, T., Schweins, R., and Strey, R. (2008). Small-angle neutron scattering from giant water-in-oil microemulsion droplets. i. ternary system. J. Chem. Phys. 128 (5), 054502. doi:10.1063/1.2779322
Fracchia, L., Cavallo, M., Giovanna, M., and Banat, M. (2012). “Biosurfactants and bioemulsifiers biomedical and related applications present status and future potentials,” in Biomedical science, engineering and technology (InTech). doi:10.5772/23821
Geisler, R., Dargel, C., and Hellweg, T. (2019). The biosurfactant β-aescin: A review on the physico-chemical properties and its interaction with lipid model membranes and Langmuir monolayers. Molecules 25 (1), 117. doi:10.3390/molecules25010117
Góral, I., and Wojciechowski, K. (2020). Surface activity and foaming properties of saponin-rich plants extracts. Adv. Colloid Interface Sci. 279, 102145. doi:10.1016/j.cis.2020.102145
Guo, Y., Mulligan, C. N., and Nieh, M.-P. (2011). An unusual morphological transformation of rhamnolipid aggregates induced by concentration and addition of styrene: A small angle neutron scattering (SANS) study. Colloids Surfaces A Physicochem. Eng. Aspects 373 (1-3), 42–50. doi:10.1016/j.colsurfa.2010.10.007
Hajimohammadi, R., and Johari-ahar, S. (2017). Determination of optimal parameters of rhamnolipid biosurfactant production: an agent for emulsification of heavy crude oil. Petroleum Sci. Technol. 36 (3), 186–192. doi:10.1080/10916466.2017.1406501
Helfrich, W. (1973). Elastic properties of lipid bilayers: theory and possible experiments. Z. Naturforsch. 28c, 693–703. doi:10.1515/znc-1973-11-1209
Hellweg, T., Gradzielski, M., Farago, B., and Langevin, D. (2001). Shape fluctuations of microemulsion droplets: A neutron spin–echo study. Colloids Surfaces A 183-185, 159–169. doi:10.1016/s0927-7757(01)00567-2
Hellweg, T., Sottmann, T., and Oberdisse, J. (2023). Recent advances in biosurfactant-based association colloidsself-assembly in water. Front. Soft Matter 2. doi:10.3389/frsfm.2022.1081877
Herzog, M., Tiso, T., Blank, L. M., and Winter, R. (2020). Interaction of rhamnolipids with model biomembranes of varying complexity. Biochimica Biophysica Acta (BBA) - Biomembr. 1862 (11), 183431. doi:10.1016/j.bbamem.2020.183431
Hoar, T. P., and Schulman, J. H. (1943). Transparent water-in-oil dispersions: the oleopathic hydro-micelle. Nature 152, 102–103. doi:10.1038/152102a0
Jahan, R., Bodratti, A. M., Tsianou, M., and Alexandridis, P. (2020). Biosurfactants, natural alternatives to synthetic surfactants: physicochemical properties and applications. Adv. Colloid Interface Sci. 275, 102061. doi:10.1016/j.cis.2019.102061
Kahlweit, M., and Strey, R. (1985). Phasenverhalten ternärer systeme des typs h2o-öl-nichtionisches amphiphil (mikroemulsionen). Angew. Chem. 97, 655–669. doi:10.1002/ange.19850970806
Kezwon, A., and Wojciechowski, K. (2014). Interaction of quillaja bark saponins with food-relevant proteins. Adv. Colloid Interface Sci. 209, 185–195. doi:10.1016/j.cis.2014.04.005
Kim, H.-S., Jeon, J.-W., Kim, S.-B., Oh, H.-M., Kwon, T.-J., and Yoon, B.-D. (2002). Surface and physico-chemical properties of a glycolipid biosurfactant, mannosylerythritol lipid, from candida Antarctica. Biotechnol. Lett. 24 (19), 1637–1641. doi:10.1023/a:1020309816545
Kitamoto, D., Isoda, H., and Nakahara, T. (2002). Functions and potential applications of glycolipid biosurfactants from energy-saving materials to gene delivery carriers. J. Biosci. Bioeng. 94 (3), 187–201. doi:10.1016/s1389-1723(02)80149-9
Kitamoto, D., Morita, T., Fukuoka, T., aki Konishi, M., and Imura, T. (2009). Self-assembling properties of glycolipid biosurfactants and their potential applications. Curr. Opin. Colloid & Interface Sci. 14 (5), 315–328. doi:10.1016/j.cocis.2009.05.009
Kouchi, M. M., Amani, H., Naseri, A., and Kariminezhad, H. (2022). Development of an effective and safe system for bioavailability of vitamin e supplements in the stomach. J. Surfactants Deterg. 25 (5), 635–642. doi:10.1002/jsde.12595
Leng, L. (2018). Biosurfactant rhamnolipid assisted microemulsification of bio-oil components in diesel. Energy Sources, Part A Recovery, Util. Environ. Eff. 41 (7), 829–843. doi:10.1080/15567036.2018.1520362
Leng, L., Wei, L., Li, W., Xu, X., Leng, S., Li, J., et al. (2022). Determination and comparison of the activation energies of biodiesel microemulsion and biodiesel blends. Energy Sources, Part A Recovery, Util. Environ. Eff. 44 (1), 116–125. doi:10.1080/15567036.2019.1648594
Lewinska, A., Domza-Kedzia, M., Wójtowicz, K., and Bazylinska, U. (2022). Surfactin-stabilized poly(D,L-lactide) nanoparticles for potential skin application. Colloids Surfaces A Physicochem. Eng. Aspects 648, 129216. doi:10.1016/j.colsurfa.2022.129216
Mayer, S., Weiss, J., and McClements, D. J. (2013). Vitamin e-enriched nanoemulsions formed by emulsion phase inversion: factors influencing droplet size and stability. J. Colloid Interface Sci. 402, 122–130. doi:10.1016/j.jcis.2013.04.016
Moya-Ramirez, I., Garcia-Roman, M., and Fernandez-Arteaga, A. (2017). Rhamnolipids: highly compatible surfactants for the enzymatic hydrolysis of waste frying oils in microemulsion systems. ACS Sustain. Chem. & Eng. 5 (8), 6768–6775. doi:10.1021/acssuschemeng.7b01008
Nguyen, T., and Sabatini, D. (2011). Characterization and emulsification properties of rhamnolipid and sophorolipid biosurfactants and their applications. Int. J. Mol. Sci. 12 (2), 1232–1244. doi:10.3390/ijms12021232
Nguyen, T. T., Edelen, A., Neighbors, B., and Sabatini, D. A. (2010). Biocompatible lecithin-based microemulsions with rhamnolipid and sophorolipid biosurfactants: formulation and potential applications. J. Colloid Interface Sci. 348 (2), 498–504. doi:10.1016/j.jcis.2010.04.053
Nguyen, T. T., and Sabatini, D. A. (2008). Formulating alcohol-free microemulsions using rhamnolipid biosurfactant and rhamnolipid mixtures. J. Surfactants Deterg. 12 (2), 109–115. doi:10.1007/s11743-008-1098-y
Nguyen, T. T., Youssef, N. H., McInerney, M. J., and Sabatini, D. A. (2008). Rhamnolipid biosurfactant mixtures for environmental remediation. Water Res. 42 (6-7), 1735–1743. doi:10.1016/j.watres.2007.10.038
Nord, L. I., and Kenne, L. (1999). Separation and structural analysis of saponins in a bark extract from quillaja saponaria molina. Carbohydr. Res. 320 (1-2), 70–81. doi:10.1016/s0008-6215(99)00134-2
Obasi, T. C., Moldovan, R., Toiu, A., Braicu, C., Bodoki, E., Berindan-Neagoe, I., et al. (2017). Molecular-trapping in emulsion’s monolayer: A new strategy for production and purification of bioactive saponins. Sci. Rep. 7 (1), 14511. doi:10.1038/s41598-017-15067-4
Oberdisse, J., Regev, O., and Porte, G. (1998). Experimental study of the micelle-to-vesicle transition. J. Phys. Chem. B 102 (7), 1102–1108. doi:10.1021/jp972390q
Olsson, U., Shinoda, K., and Lindman, B. (1986). Change of the structure of microemulsions with the hydrophile lipophile balance of nonionic surfactant as revealed by nmr self-diffusion studies. J. Phys. Chem. 90 (17), 4083–4088. doi:10.1021/j100408a050
Palanisamy, P., and Raichur, A. M. (2009). Synthesis of spherical NiO nanoparticles through a novel biosurfactant mediated emulsion technique. Mater. Sci. Eng. 29 (1), 199–204. doi:10.1016/j.msec.2008.06.008
Penders, M., and Strey, R. (1995). Phase behavior of the quaternary system h2io/n-octane/c8e5/n-octanol: role of the alcohol in microemulsions. J. Phys. Chem. 99, 10313–10318. keine Kopie im Archiv. doi:10.1021/j100025a037
Reimer, J., Södermann, O., Sottmann, T., Kluge, K., and Strey, R. (2003). Microstructure of alkyl glucoside microemulsions: control of curvature by interfacial composition. Langmuir 19, 10692–10702. doi:10.1021/la034847v
Rodrigues, L. R. (2015). Microbial surfactants: fundamentals and applicability in the formulation of nano-sized drug delivery vectors. J. Colloid Interface Sci. 449, 304–316. doi:10.1016/j.jcis.2015.01.022
Salager, J., Morgan, J., Schechter, R., Wade, W., and Vasquez, E. (1979). Optimum formulation of surfactant/water/oil systems for minimum interfacial tension or phase behavior. Soc. Petroleum Eng. J. 19 (02), 107–115. doi:10.2118/7054-pa
Schober, A., Zhang, J., Subramaniam, A., and Normand, V. (2017). Emulsification efficacy of quillaja saponins at very low concentration: model development and role of alcohols. Colloids Surfaces B Biointerfaces 159, 829–837. doi:10.1016/j.colsurfb.2017.08.041
Schubert, K. V., Strey, R., and Kahlweit, M. (1990). A new purification technique for alkyl polyglycol ethers and miscibility gaps for water-CiEj. J. Colloid Interface Sci. 141 (1), 21–29. Das Phasenverhalten von einige binaeren Systemen wird beschrieben. Die Abhaengigkeit von der Tensidreinheit wird dargetstellt. doi:10.1016/0021-9797(91)90298-m
Schwuger, M.-J., Stickdorn, K., and Schomäcker, R. (1995). Microemulsions in technical processes. Chem. Rev. 95, 849–864. doi:10.1021/cr00036a003
Shinoda, K., and Kunieda, H. (1973). Conditions to produce so-called microemulsions: factors to increase the mutual solubility of oil and water by solubilizer. J. Colloid Interface Sci. 42 (2), 381–387. doi:10.1016/0021-9797(73)90303-2
Solans, C., and Kunieda, H. (1997). Industrial applications of microemulsions. New York: Marcel Dekker.
Sottmann, T., Kluge, K., Strey, R., Reimer, J., and Söderman, O. (2002). General patterns of the phase behavior of mixtures of h2o, alkyl glucosides, and cosurfactants. Langmuir 18, 3058–3067. doi:10.1021/la011665x
Sottmann, T., and Strey, R. (1996). Shape similarities of ultra-low interfacial tension curves in ternary microemulsion systems of the water-alkane-ciej type. Ber. Bunsenges. Phys. Chem. 100 (3), 237–241. doi:10.1002/bbpc.19961000309
Strey, R. (1994). Microemulsion microstructure and interfacial curvature. Colloid and Polym. Sci. 272, 1005–1019. doi:10.1007/bf00658900
Xie, Y., Li, Y., and Ye, R. (2005). Effect of alcohols on the phase behavior of microemulsions formed by a biosurfactantrhamnolipid. J. Dispersion Sci. Technol. 26 (4), 455–461. doi:10.1081/dis-200054576
Wojciechowski, K., Jurek, I., Góral, I., Campana, M., Geue, T., and Gutberlet, T. (2021). Surface-active extracts from plants rich in saponins - effect on lipid mono- and bilayers. Surfaces Interfaces 27, 101486. doi:10.1016/j.surfin.2021.101486
Wojciechowski, K., Orczyk, M., Marcinkowski, K., Kobiela, T., Trapp, M., Gutberlet, T., et al. (2014). Effect of hydration of sugar groups on adsorption of quillaja bark saponin at air/water and Si/water interfaces. Colloids Surfaces B Biointerfaces 117, 60–67. doi:10.1016/j.colsurfb.2014.02.010
Wojciechowski, K. (2013). Surface activity of saponin from Quillaja bark at the air/water and oil/water interfaces. Colloids Surfaces B Biointerfaces 108, 95–102. doi:10.1016/j.colsurfb.2013.02.008
Worakitkanchanakul, W., Imura, T., Fukuoka, T., Morita, T., Sakai, H., Abe, M., et al. (2009). Phase behavior of ternary mannosylerythritol lipid/water/oil systems. Colloids Surfaces B Biointerfaces 68 (2), 207–212. doi:10.1016/j.colsurfb.2008.10.009
Worakitkanchanakul, W., Imura, T., Morita, T., Fukuoka, T., Sakai, H., Abe, M., et al. (2008). Formation of w/o microemulsion based on natural glycolipid biosurfactant, mannosylerythritol lipid-a. J. Oleo Sci. 57 (1), 55–59. doi:10.5650/jos.57.55
Xie, Y., Ye, R., and Liu, H. (2007). Microstructure studies on biosurfactant-rhamnolipid/n-butanol/water/n-heptane microemulsion system. Colloids Surfaces A Physicochem. Eng. Aspects 292 (2-3), 189–195. doi:10.1016/j.colsurfa.2006.06.021
Yang, Y., and McClements, D. J. (2013). Encapsulation of vitamin e in edible emulsions fabricated using a natural surfactant. Food Hydrocoll. 30 (2), 712–720. doi:10.1016/j.foodhyd.2012.09.003
Keywords: biosurfactant, rhamnolipid, saponin, microemulsion, droplets, microstructure, scattering techniques
Citation: Hellweg T, Oberdisse J and Sottmann T (2023) Mini review: recent advances in biosurfactant-based association colloids–formation of microemulsions. Front. Soft Matter 3:1260211. doi: 10.3389/frsfm.2023.1260211
Received: 17 July 2023; Accepted: 01 September 2023;
Published: 14 September 2023.
Edited by:
Francisco Monroy, Complutense University of Madrid, SpainReviewed by:
Sylvain Prévost, Institut Laue-Langevin, FranceCopyright © 2023 Hellweg, Oberdisse and Sottmann. This is an open-access article distributed under the terms of the Creative Commons Attribution License (CC BY). The use, distribution or reproduction in other forums is permitted, provided the original author(s) and the copyright owner(s) are credited and that the original publication in this journal is cited, in accordance with accepted academic practice. No use, distribution or reproduction is permitted which does not comply with these terms.
*Correspondence: Thomas Hellweg, thomas.hellweg@uni-bielefeld.de; Thomas Sottmann, thomas.sottmann@ipc.uni-stuttgart.de
Disclaimer: All claims expressed in this article are solely those of the authors and do not necessarily represent those of their affiliated organizations, or those of the publisher, the editors and the reviewers. Any product that may be evaluated in this article or claim that may be made by its manufacturer is not guaranteed or endorsed by the publisher.
Research integrity at Frontiers
Learn more about the work of our research integrity team to safeguard the quality of each article we publish.