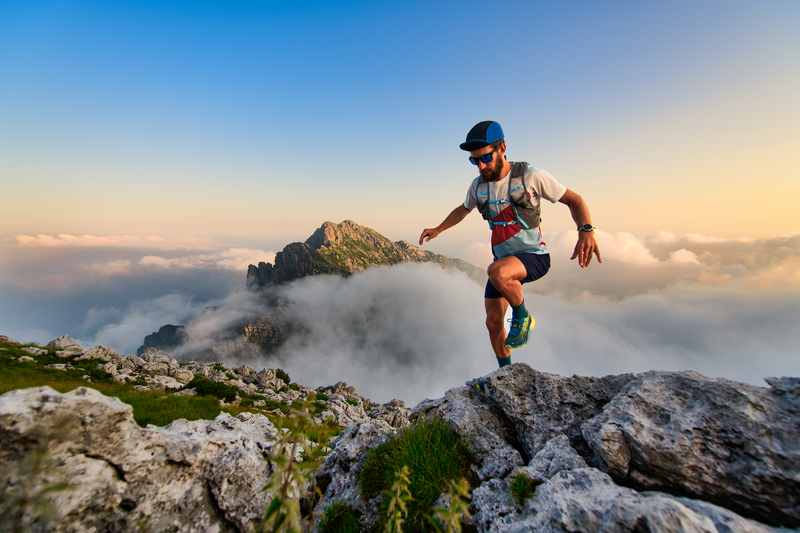
95% of researchers rate our articles as excellent or good
Learn more about the work of our research integrity team to safeguard the quality of each article we publish.
Find out more
ORIGINAL RESEARCH article
Front. Soft Matter , 08 September 2023
Sec. Colloids and Emulsions
Volume 3 - 2023 | https://doi.org/10.3389/frsfm.2023.1240878
This article is part of the Research Topic Celebrating 1 Year of Frontiers in Soft Matter View all 5 articles
Understanding the mechanical behavior of biological membranes is of paramount importance in cell biophysics and in developing new biomaterials for medicine. In this study, we delve into the mechanical impact of β-escin, commonly referred to as escin, a naturally occurring biosurfactant derived from the seeds of the horse chestnut tree. To examine the modulable interaction between escin and dimyristoylphosphatidylcholine (DMPC), which is an archetypical fluid phospholipid and an essential constituent of the cellular fluid membrane, we have used artificial models based on the liquid crystal structure, such as bilayer vesicles and Langmuir monolayers. We have focused on the energetic and kinetic aspects of escin insertion when transversally adsorbed or longitudinally integrated within these model membranes. By employing surface microscopies of epifluorescence and Brewster angle reflectivity, we have elucidated the structural phase behavior of hybrid escin–phospholipid membranes, which exhibit dual mechanical properties characterized by high rigidity and reduced fluidity. Notably, at low temperatures, we observe a soft, glassy rheological behavior reminiscent of liquid crystalline ordered phases, which turns into a fluid-like viscoelasticity resembling more disordered phases at physiological temperatures. The hybrid membranes behave in one way or another as both are driven by an adsorption potential well imposed by escin cohesivity. These intriguing findings are discussed from a physicochemical perspective, highlighting their potential for future pharmacological designs and biomedical applications that exploit the dual mechanical impact of escin on biological membranes.
The fluid mosaic paradigm is a structural concept that describes biological membranes as flexible objects with lateral molecular mobility (Singer and Nicolson, 1972). The heterogeneous mosaic-like composition allows embedded membrane proteins to move laterally within lipid domains that are dispersed in a continuous liquid phase (Simons and Ikonen, 1997). Although flexible membranes resist shear deformations as solids, they flow as incompressible liquids under applied shear stress. Biological membranes require structural rigidity and lateral fluidity, combining the solid-like and liquid-like characteristics, to maintain functional homeostasis (Sprong et al., 2001; Van Meer et al., 2008; Philips et al., 2009). Two previous pivotal studies have hypothesized that this mechanical duality arises from the viscoelastic nature of the proteolipid membrane, which is mainly influenced by the heterogeneous composition of the constituent lipid monolayers (Evans and Hochmuth, 1976; Espinosa et al., 2011). The dual viscoelasticity of biological membranes, characterized by high dynamic viscosity for regulated fluidity and a finite shear modulus for structural resistance (López-Montero et al., 2008), is supported by the liquid crystalline arrangement of membrane lipids (López-Montero et al., 2010; Catapano et al., 2011; Castro et al., 2014; Hermans and Vermant, 2014). Experimental studies with real cells have further substantiated the viscoelastic duality hypothesis of biological membranes as regulated by lipid traffic flows (Catapano et al., 2017; Deseri and Steigmann, 2017; Alonso and Goñi, 2018; Le Roux et al., 2019; Xu et al., 2020). For instance, metabolic conversions of fluid sphingomyelins into solid ceramide led to lateral tension Marangoni gradients (Catapano et al., 2017; Alonso and Goñi, 2018), resulting in cell shape transformations with a functional significance (López et al., 2012). Furthermore, lipid membranes have the functional capacity to propagate mechanical signals, playing a crucial role in cellular mechanobiology (Le Roux et al., 2019; Mussel and Schneider, 2019). Moreover, membrane viscoelastic stresses have been implicated in cancer development (Tse et al., 2012; Xu et al., 2020) and phenotypic cell hereditariness (Deseri and Steigmann, 2017; Vorselen et al., 2018). Despite the important advances in understanding the functional dynamics of biomembranes through experimental models (López-Montero et al., 2008; Arriaga et al., 2009; Arriaga et al., 2010a; López-Montero et al., 2010; Catapano et al., 2011; Espinosa et al., 2011; Schmid, 2013; Castro et al., 2014; Hermans and Vermant, 2014; Hormel et al., 2014; Langevin and Monroy, 2014; Wu et al., 2015; Catapano et al., 2017; Al-Rekabia and Contera, 2018) and the relevant theoretical approaches related to their liquid crystalline viscoelasticity (Helfrich, 1973; Lipowsky, 1991; Seifert, 1997; Arriaga et al., 2008; Rahimi and Arroyo, 2012; Mell et al., 2015; Santiago et al., 2019; Liu and Kim, 2020; Santiago and Monroy, 2020), our current understanding of the mechanical paradigm of biological membranes remains incomplete, particularly regarding the molecular impacts of order-imparting components on membrane stresses. Here, we present a rheological investigation of the order-imparting molecule β-escin, a saponin biosurfactant that mimics the effects of solid-like mechanical stresses in model phospholipid membranes. We have analyzed the surface rheology of these membrane models by recording the solid saponin molecule (β-escin) while simultaneously enhancing surface stiffness but dropping viscous friction (Sreij et al., 2017; Kharbedia et al., 2021). Dual mechanical effects have been discriminated in two differential structural scenarios: longitudinal escin integration mimicking intrinsic lateral membranogenesis or transverse escin insertion after bulk diffusion recapitulating extrinsic membrane traffic. Such mechanical duality as a high stiffness followed by high fluidity, and the emergent properties tunable therefrom, confer β-escin in particular and saponins in general with two scopes of applicability, biotechnological as novel biosurfactants with singular surface rheology imparting thermodynamic stability without loss of mechanical performance (Böttcher and Drusch, 2017) and biomedical as natural therapeutic agents targeting cell membrane rigidness being a key regulator of inflammation (Sirtori, 2001; Lorent et al., 2014).
Natural saponins are a family of triterpenoid saccharides present in many common plants, such as potatoes, beans, soy, and ginseng (Hostettmann and Marston, 1995). They can also be obtained from phytochemical extracts of several medicinal plants, including Quillaja saponaria, Yucca schidigera, Saponaria officinalis, and Aesculus hippocastanum (Sparg et al., 2004; Cheok et al., 2014). Because of their self-assembly and surface-active behavior at aqueous interfaces, they endow emulsifier and foaming activities in aqueous media (Böttcher and Drusch, 2017; Geisler et al., 2020a). Their biochemical classification as biosurfactants makes them useful for the technological development of functional foods, cosmetics, phytosanitary products, and natural drugs (Güçlü-Ustündağ and Mazza, 2007; Böttcher and Drusch, 2017). Particularly, β-escin(aka escin), the major saponin component extracted from the chestnut seed of A. hippocastanum, is currently being exploited as a convenient biosurfactant in a variety of technological applications (Hostettmann and Marston, 1995; Sparg et al., 2004; Güçlü-Ustündağ and Mazza, 2007; Cheok et al., 2014; Böttcher and Drusch, 2017; Geisler et al., 2020a). This triterpenoid derivative of glucopyranosic acid has the chemical formula shown in Figure 1A, which reveals the amphiphilic molecular structure discernible as an oligosaccharide flexible subsystem—the glyconic saccharide constituting the polar head—which is linked to a triterpene core ring planar subsystem—the aglycone constituting the hydrophobic moiety (see Figure 1A; inset). The glyconic part contains glucose and glucuronic acid, both hydrophilic, whereas several polar oxygen groups are attached to the aglyconic part. They appear one-sided in the triterpene, thus conferring lateral (longitudinal) polarity additional to the conventional (transverse) surfactant-like bipolarity (De Groot et al., 2018). This quadrupolar amphipilic structure confers high solubility followed by enhanced hydrophilicity responsible for a relatively high critical micellar concentration (
FIGURE 1. (A) Chemical formula of β-escin, or simply escin (MW 1,131.26 g/mol). Notice the quadrupolar structure of the molecule with a polar head constituted by a trisaccharide sugar (blue trapezoid) and a laterally amphiphilic moiety of pentacyclic terpenoid, an aglycone basis prone to membrane insertion under lateral stacking (yellow arrow). DMPC is the structural phospholipid chosen to recapitulate a fluid and flexible membrane. (B) Structural scenarios for saponin membrane incorporation under lateral stacking. The intrinsic integration corresponds to ab initio incorporation in the endogenous composition (i.e., before membrane formation, also referred to as longitudinal escin integration). The extrinsic insertion scenario corresponds to ad finem incorporation under exogenous adsorption (i.e., after membrane formation, also referred to as transverse escin insertion). Adsorption mechanism: 1) escin first interacts hydrophilically with the outer membrane through the polar phospholipid heads; 2) once adsorbed, it reorganizes hydrophobically with the terpenoid ring embedded by lateral stacking in the organic core of the lipid membrane; see zoomed inset for final equilibrium insertion. Both scenarios are comparatively studied.
In structural terms referred to the adsorption compaction status at aqueous membrane interfaces, the amphiphilic quadrupolar nature of escin outfits with its potential ability to further interact longitudinally with other amphipathic membrane partners (Sreij et al., 2017; Glickman et al., 2020; Kharbedia et al., 2021). These extraordinary (in-plane) intrinsic interactions are superposed to ordinary (out-of-plane) extrinsic adsorption, thus causing additional mosaic membrane ordering by lateral membrane stacking mediated by the amphiphilic terpenoid moiety interacting with other membrane phospholipids, sphingolipids and cholesterol (Sreij et al., 2017; Sreij et al., 2019; Geisler et al., 2020a; Geisler et al., 2020b). The formation of surface-structured films in some saponin extracts, other than escin, has been revealed to be concomitant with compact hydrophobic domains attributed to additional hydrogen bonding between sugar residues (e.g., in triterpenoid saponins of oleanane or dammarane aglycone type) (Böttcher and Drusch, 2017; Geisler et al., 2020a). Furthermore, some structural viscoelastic relationships have been derived between the botanical origin of the different saponins and their interfacial behavior, as determined by the formation of hydrophobic surface domains laterally compacted under hydrogen bond stacking (Golemanov et al., 2013; Böttcher et al., 2017). In particular, the most stable films were identified for saponins of oleanane type as mesoscopic shear rigidity is mediated by static solid domains rapidly formed at equilibrium (Golemanov et al., 2014).
Saponins with different botanic origins exhibit a variety of biological activities, such as natural antibiotics and biocides (Miyakoshi et al., 2000; Sparg et al., 2004). Moreover, some cytotoxic effects and anti-tumorigenic potentials have been shown on cancer cells treated with certain saponins, including β-escin (Lorent et al., 2014; Koczurkiewicz et al., 2015; Elekofehinti et al., 2021). However, their propensity to induce systemic hemolysis limits toxicological safety in cancer therapies (Sirtori, 2001; Gauthier et al., 2009). Saponins are included among a class of natural medicines called phlebotonics, which are used to treat thrombophlebitis and other venous insufficiencies, mainly varicose veins of the legs and hemorrhoids (Sirtori, 2001). They can be safely administrated by ingestion or topical application, and depending on the dose, they produce different phlebotonic effects, such as imparting rigidity and fluidity into the cellular membranes of the venous endothelial walls (Sirtori, 2001; Lorent et al., 2014; Gwozdzinski et al., 2023). Particularly referring to β-escin extracted from chestnut seeds (A. hippocastanum), anti-inflammatory, anti-edematous, and venotonic effects have been attributed to this natural compound (Sirtori, 2001; Gallelli, 2019). Many clinical studies have been conducted to date (Underland et al., 2012), specifically confirming escin as a safe and effective phlebotonic to treat chronic venous insufficiency (CVI) (Pittler and Ernst, 2012; Dudek-Makuch and Studzińska-Sroka, 2015). In pathophysiological terms, the escin-based regulation of cell membrane rigidness seems to be the key factor for reducing varicose inflammation (Gwozdzinski et al., 2023). Although the etiology of inflammatory venous diseases is very complex, it involves genetic susceptibility, cellular responses, and environmental factors that cause vein wall inflammation, such as hypoxia, abnormal flow stresses, and venous blood stasis elicited by altered levels of nitric oxide and prostaglandins in circulation (Smith et al., 2006; Cogolludo et al., 2019). The mechanical status of membrane stiffness and fluidity in the venous endothelium, its glycocalyx, and the circulating blood cells is expected to be critical for determining the pathophysiological changes that occur in the venous bloodstream, for the expression of membrane-receptor cytokines and other cell adhesion molecules necessary for normal venous circulation. Despite the evident etiological involvement of altered membrane mechanics (Gallelli, 2019; Gwozdzinski et al., 2023), the possible pathogenic role of altered membrane fluidity remains unidentified. Therefore, studying the rheological impacts of escin on model biomimetic membranes is of utmost importance for assessing phlebotonic potentials, not only for CVI treatments, but also in other venous inflammatory diseases, considering cell membrane rigidness, fluidity, and adhesivity as therapeutic targets potentially modulable by the surface action of this saponin.
In this study, we have explored the mechanical influence of surface-structuring escin in model viscoelastic membranes reconstituted under compositional mosaicity, particularly in Langmuir monolayers and bilayer vesicles composed of the zwitterionic phase-segregating phospholipid dimyristoylphosphatidylcholine (DMPC). By exploiting the DMPC mesogenic transition between liquid crystal bilayer phases (from the ordered gel LO-phase near room temperature to the disordered Lα-phase at
The purified molecule β-D-glucopyranosyl-(1→2)-[β-D-glucopyranosyl-(1→4)]-(22α-(acetyloxy)-16α,24,28-trihydroxy-21β-{[(2Z)-2-methylbut-2-enoyl]oxy}olean-12-en-3β-yl(β-D-glucopyranosidu-ronic acid), shortly named β-escin, was purchased from Sigma [purity grade as a pharmaceutical primary standard) and used without further purification (M.W. 1,131.26 g/mol). Escin was dissolved in ultrapure Milli-Q water (0.1 mM final submicellar concentration;
To measure penetration pressures in adsorption monolayers at the air/water (A/W) interface, we used a computer-controlled Langmuir trough (KSV/NIMA, small, 77.5 cm2, Biolin Scientific Holding Ab, Stockholm, Sweden). By using a paper Wilhelmy-type sensor and two mobile barriers fabricated in Teflon®, the Langmuir trough is equipped with a pressure-area measuring system,
Brewster angle microscopy (BAM) imaging revealed the internal structure of the monolayer at the micrometric level. BAM measurements were performed in a Langmuir trough installed on an I-Elli2000 ellipsometry station equipped with a Nd:YAG diode laser (
in terms of the surface film thickness (
Hence, the bare A/W interface appeared homogeneously black under the BAM (if
We measured the isothermal compression modulus of the surface film as
We measured two-dimensional shear modulus (
GUVs were prepared using the electroformation method according to the optimized protocol described by Mathivet et al. (1996). To prepare the GUVs, the lipid powder was first dissolved (at 1 mg/ml) in a chloroform–methanol mixture (2:1 v/v), then a drop of 20–30 μL was deposited on the ITO slide, and finally, the solvent was removed by evaporation in a stream of dry nitrogen. To prepare the GUVs of DMPC in the fluid state, we assembled ITO chambers first filled with an aqueous sucrose solution (200 mM) and then placed them inside an oven at ca. 38°C, well above the melting temperature of the phospholipid (Tm = 23.6°C). Afterward, the sealed electroformation chambers were connected to an electric field for 3 h (8 Hz, 1.8 V). For subsequent GUV visualization under an optical microscope, the GUV sample previously electroformed in sucrose was further diluted in a glucose solution at a slightly higher concentration (208 mM). Such density contrast properly favored GUV sedimentation and visualization near the microscopy slide. For intrinsic integration assays (ab initio method), escin dissolved in methanol was admixed with the phospholipid solution in chloroform (30% w/w escin with respect to DMPC). For extrinsic insertion assays (ad finem method), a solution of escin monomers was added after the DMPC vesicles were electroformed in the suspending medium (40 µM final submicellar concentration) (De Groot et al., 2018). For fluorescence microscopy, a small amount of RhPE was added (0.1% w/w with respect to DMPC). Confocal microscopy observations were performed at room temperature.
From previous studies, escin is known to lead to the formation of solid domains when incorporated into fluid membranes (ab initio method) (Sreij et al., 2017; Geisler et al., 2020b; Glickman et al., 2020). It structurally behaves as integrated into the membrane composition through membrane biogenesis (see Figure 1; intrinsic longitudinal integration). To evaluate such longitudinal insertion in GUVs composed of a fluid DMPC membrane (at high temperature
FIGURE 2. Confocal microscopy images obtained on the escin-integration/insertion scenarios as designed in Figure 1B for electroformed giant unilamellar vesicle (GUV) membranes. The microscopy observations were performed at room temperature (ca. 22°C). Under the considered experimental conditions, the fluorescent phospholipid dye RhPE was selective for the fluid-disordered phospholipid Lα-phase and excluded from the LO-phase (see Section 2). Scale bars: 2 microns. (A) Intrinsic escin integration (ab initio) or longitudinal phase-separation scenario in which escin is included within DMPC before GUV electroformation. (Left panel) A pure DMPC vesicle in the homogeneous liquid-disordered phase (Lα). (Right panels) A hybrid, phase-separated (Lα–LO) vesicle as electroformed from the DMPC–escin mixture (30% w/w.). (Inset) Zenithal view of a Lα–LO separated vesicle showing circular LO-domains in Lα-continuous. (Bottom) Two “matrioska” vesicles appear with the same heterogeneous Lα–LO phase segregated configuration. (B) Extrinsic escin insertion (ad finem), or transverse phase-separation scenario in which escin is membrane inserted under adsorption from the suspending medium after DMPC GUVs are electroformed. (Left panels) Initial transformation stage, thereby a pure DMPC vesicle in the fluid Lα-phase becomes deformed under the action of a solid top layer locally adsorbed after 1 h incubation [in 40 μM escin, below CMC]. (Inset) Polygonal edge profile of a solidified GUV under rigidization by an escin top layer. (Bottom) Matrioska vesicles with the outer membrane rigidized in contact with escin, thus appearing solid-like (flat edges). The inner membranes remain fluid-like as becoming flexibly adapted to the inner space (rounded edges). (Right panel) Final equilibrium of the DMPC–escin vesicles as being Lα–LO phase-separated (after 24 h of escin-incubation). Once adsorbed and further reorganized, the escin-rich LO-domains appear as flat edges excluding the fluorescent dye. The DMPC-rich Lα-phase adapts the rigid flat domains as a continuous flexible junction (see inset). Schematics: The bottom panels systematize the observed behavior in each insertion scenario.
When escin was externally incorporated into previously formed DMPC vesicles, as inserted from the outer medium, we defined it as a transverse insertion (ad finem method). Figure 2B shows the rationale designed for this purpose (extrinsic insertion). We first prepared pure DMPC GUVs at the Lα-phase (escin absent in the lipid formula). Subsequently, we added escin monomers dissolved sub-micellar in the vesicle outside (at 40 μM final concentration). The hybrid DMPC–escin GUVs were stable in escin suspension (as no escin micelles were present to dissolve the lipids from the GUV bilayers at
The aforementioned empirical observations reveal how the transverse escin integration in DMPC membranes follows a two-stage adsorption–reorganization mechanism, as observed in other fluid membranes (Serrien et al., 1992; Muñoz et al., 2000; Langevin and Monroy, 2014). Firstly, escin adsorption induces extrinsic membrane stiffening in the flexible (Lα) DMPC membrane; primary relaxation. Once adsorbed, escin has enough time to reorganize and then equilibrate within the DMPC bilayer. Hence, the hybrid membrane undergoes phase segregation from 1Φ (Lα-phase) to 2Φ (phase demixing); secondary relaxation. The rigid escin-rich domains appear solid-like in the flattened vesicle edges, becoming mechanically equilibrated through flexible joints constituted by rounded DMPC-rich vertices constituted by the continuous Lα-phase (see schematics in Figure 2B; bottom). The rigid domains are presumably escin-rich because the fluorescent dye is excluded out from the laterally ordered hydrophobic arrangement constituted as lateral stacks between the steroidal moieties of the escin molecule (Sreij et al., 2017; Penfold et al., 2018). In agreement with previous hypotheses about structural condensation (Sreij et al., 2017; Geisler et al., 2019; Glickman et al., 2020), our results suggest reaching the equilibrium status of escin inside the phospholipid bilayer through the direct interaction between the acyl chain hydrophobic core of the membrane and the aglycone counterpart of the rigidizing molecule, similar to the structural compaction mediated by cholesterol between phospholipids and sphingolipids in real biomembranes (Van Meer et al., 2008; Espinosa et al., 2011).
To confirm the crystalline nature of the membrane escin-rich domains at low temperatures (
FIGURE 3. Crystal nucleation and growth process under escin insertion in Langmuir monolayers at low temperature (
As a further insight going beyond the aforementioned qualitative analysis on low-temperature crystallization, we studied the transversally organized incorporation of escin by taking advantage of the dye-free visualization enabled under the BAM after DMPC monolayer formation in the homogenous LO-state at low temperature (
The aforementioned observations on escin crystal formation at low temperatures suggested a two-step mechanism mediated by initial nucleation and further growth. Figure 4 shows low-temperature biphasic kinetics driven by bulk-to-surface diffusive adsorption somewhat limited by a terminal reorganization process (Dukhin et al., 1994). Although similar adsorption kinetics occurs at higher temperatures, the surface system remains monophasic into a disordered fluid phase. In a previous study, we evidenced a preference for escin for heterogeneous demixing in DMPC monolayers at the lower temperatures studied (Sreij et al., 2017). In the current work, we further explored this phase-separation behavior in more detail, detecting the inductive role of ordered phospholipids in solid escin insertion into quasi-2D crystalline monolayers. The adsorption kinetics at the A–W interface was studied as a function of the packing state of the lipids on the supporting DMPC membrane. Specifically, we tracked surface pressure (
FIGURE 4. (A) Adsorption kinetics of escin on a DMPC monolayer at different initial states (
Figure 4A shows the kinetic plots recorded in temperature regimes: low-T at which the phospholipids chains become tightly packed as mesogenically arranged in an ordered state (LO-phase at
According to the adsorption theory by Graham and Phillips (1979), substantiated by Pethica’s problem for monolayer penetration (Pethica, 1955), and Buttler’s theory for the thermodynamics of the surface of solutions (Buttler, 1932), the observable kinetics follows an adsorption–reorganization process, thereby a pressure penetration jump occurs with respect to the initial status
where
This bimodal BPGP kinetics considers two consecutive steps (Buttler, 1932; Pethica, 1955; Graham and Phillips, 1979; Dukhin et al., 1994). First, the initial adhesive adsorption is characterized by the diffusive time
under cohesive energy
corresponding to escin and DMPC interacting with each other under condensing partial areas, that is,
Therefore, the constitutive BPGP parameters depend on the initial pressure (
From the adsorption kinetics observed in the experiments (see Figure 4), two different regimes can thus be inferred as limiting dynamic behaviors:
A) Diffusion controlled at short insertion times (i.e.,
B) Insertion controlled under internal reorganization (i.e.,
For adequate parametrization of the adsorption process, the experimental results in Figure 4 demonstrate the two sequential regimes predicted by the BPGP theory (also inferred from the qualitative analyses of the microscopic membrane textures in Figures 2, 3): 1) initial extrinsic incorporation of escin toward the bulk vicinity of the adsorbent monolayer and 2) subsequent intrinsic penetration of escin into the phospholipid monolayer. We further analyze the corresponding energetics and relaxation times as determined from the initial monolayer pressure in differential DMPC compaction statuses determined by temperature.
The equilibrium pressures in Figure 4B demonstrate the energy trade-off predicted between the final escin insertion and the initial DMPC state [see Eq. (2)]. For constant escin concentration, we detect in both phases the linear relationship
TABLE 1. Energetic surface compaction parameters for the DMPC–escin system (as determined from the experimental data in Figure 3B). The structural parameters for the corresponding cross-sectional areas are taken from literature data (as in Section 3.3) (for DMPC: Van Meer et al., 2008; Philips et al., 2009; for escin: Sreij et al., 2017; Geisler et al., 2020a).
At structural compatibility with the high- and low-temperature states of DMPC (liquid-disordered LD/liquid-ordered LO), we detected differences determined by the molecular malleability of the reconfigurable escin (see schematics in Figure 3). The observed compaction changes are larger in the fluid-like LD-phase than in the solid-like LO-phase (Geisler et al., 2020a). Specifically, we found
According to the adsorption BPGP kinetics, the values measured for
The kinetic data in Figure 4D show the escin-specific relaxation times as experimentally derived from the BPGP kinetics in Eq. 1:
TABLE 2. Experimental kinetic parameters for the DMPC–escin system in the high- and low-temperature states (as determined from the experimental data in Figure 3D). The estimated interaction energies are determined as per molecule.
The characteristic relaxations were faster with increasing temperature (
Comparing the reorganization times calculated with regard to the BPGP theory, we found the longest ones for the lowest temperature corresponding to the most escin-inserted states (escin-rich LO-phase; i.e.,
The solid-like behavior of the bare escin molecule has been well-established in previous studies on Langmuir monolayers and bilayer vesicles of the pure component (Sreij et al., 2017; Sreij et al., 2019; Geisler et al., 2020a). However, little is known about its mechanical impact as imparting rigidity in phospholipid membranes, particularly in the biologically relevant packing status (i.e., the hypothetical liquid-ordered phase compatible with the bilayer packing) (Marsh, 1996). In a recent study on membrane mechanics using NSE with LUVs, we reported the impact of the escin–DMPC molecular interaction on the bending stiffness of highly curved bilayer membranes (Sreij et al., 2018). From the analysis of the NSE relaxation times performed at the molecular scale, we inferred an escin-dependent membrane stiffening as imparted on the fluid phase well above the melting transition of DMPC
Figure 5 shows the two rheological scenarios explored at low temperatures (solid-like: blue symbols and upper panels) and high physiological-like temperatures (fluid-like: red symbols and lower panels). For the highly rigid escin monolayers, the stress-strain plots exhibit nonlinear softening above a yield point detected at very small strains (about
FIGURE 5. Surface shear rheology of escin–DMPC monolayers (at the biological reference status
In addition to the surface shearing experiments in the linear regime, we performed mechanical experiments probing the compression isotherms and the linear compression modulus of the DMPC monolayers spread over an escin-containing subphase (Catapano et al., 2011). Figure 6 shows measurements performed in the physiologically relevant states of escin–DMPC interaction at high temperatures compatible with the liquid-disordered molecular status of the phospholipid that always appeared monophasic as per entropic dominance. These high-temperature biomimetic systems were compared with the respective enthalpic statuses of high compaction compatible with escin adhesivity that appeared either monophasic for pure escin or biphasic after the mixing interaction with the liquid-ordered phase of DMPC (at low temperatures). The compression isotherms plotted in Figure 6A reveal a high-temperature dataset with an expanded-like monophasic behavior (left panel), contrasting the highly ordered condensed-like character of the biphasic statuses recorded at low temperature (right panel). On the one hand, high-temperature conditions impose the surface pressure to monotonically increase without strong structural change from the initial state up to collapse (
FIGURE 6. Experimental isotherms for hydrostatic compression of escin–DMPC monolayers. (A) Hydrostatic
Figure 6B shows the calculated values of the hydrostatic compression modulus
Nevertheless, we must remember that the hybrid DMPC–escin system can eventually remain in a heterogeneous biphasic state with solid escin-rich domains rafting the continuous DMPC-rich phase (see Figure 3). They can eventually lead to additional rigidities with dominant shear rigidity for the highest escin insertions. Hence, an intermediate mechanical impact is observed in these 2Φ-statuses, especially at low temperatures (see Figure 5; central panels). Hence, we visualize the global rigidness as a mesoscopic equivalent
The rheological data showed a nonlinear softening behavior, clearly evidenced for the pure escin monolayers as a plastic plateau above yield stress (see Figure 5A). They exhibited an evident dissipative origin: first, a decrease in the apparent shear modulus as the consequence of the stress softening above the yield point (the elastic stress
For the binary monolayers of liquid-like escin–DMPC, since transversely penetrated by solid-like escin, the biphasic monolayer was observed to produce an intermediate rheological scenario (see Figure 3). At low temperatures, we detected a dominance of the solid escin domains in the linear part of the stress–strain plot (in both temperature regimes, we detected
In rheological terms, a typical soft solid is dynamically characterized by a weak dependence of the viscoelastic moduli on the deformation frequency. Particularly, in the Hookean (linear) regime, the rigidity modulus and the slightly lower loss modulus are expected to scale with a similar power law as
FIGURE 7. Frequency dependence of the surface shear rheology of escin–DMPC monolayers in the linear regime of shear deformation for the biological regulation status at
On the one hand, we detected the solid-like escin monolayers behaving SGR-like with a shear modulus displaying a weak 5power-law dependency (
This study addressed the physicochemical role of β-escin in the functional regulation of mosaic membrane mechanics recreated in model systems. In biomedical terms, this natural saponin acts as a phlebotonic medicine used, for instance, to treat chronic CVI. We showed the amphiphilic character of escin, conferring a dual mechanical impact on model membranes. Membrane escin is prone to either self-aggregate extrinsically into rigid solid phases that cause local stiffening but fluidization under mesoscopic disorder or intrinsic compaction with other membrane components that also produce rigidification but a global decrease in fluidity. The experiments performed here with model systems demonstrate membrane escin incorporation either intrinsically along the longitudinal in-plane integration mode or extrinsically across the transverse out-of-plane penetration. These insertion pathways differently impact the effective rheology of the escin-modified membrane. If escin is internally incorporated into the lipid membrane (intrinsic longitudinal integration), the system immediately raises the mechanical equilibrium as a soft, glassy solid more rigid than fluid. However, when escin is externally incorporated from the adjacent subphase (extrinsic transverse insertion), a bimodal adsorption mechanism emerges, governed by bulk diffusion–membrane reorganization, leading to more fluid than rigid behavior. In particular, when adsorbed from the bulk on a phospholipid membrane surface, a thin saponin layer adsorbs the surface from its outer side to become later integrated as a hybrid escin–lipid membrane. First, the transversely incorporated molecules extrinsically promote phase segregation, leading to structural disorganization (during the initial steps of adhesive membrane adsorption). Later, intrinsic molecular condensation leads to local structuration followed by equilibrium (during terminal cohesive relaxation). These dual dynamic behaviors emerge from the multiple scales involved in the adsorption–insertion process, as described by the BPGP theory (Buttler, 1932; Pethica, 1955; Graham and Phillips, 1979; Fainerman and Vollhardt, 1999) from the bottom scale of the diffusing escin monomers dissolved in the bulk subphase, through the intermediate bulk–surface infrastructure (where they adhere and reorganize within the polar heads of the membrane components) up to the superior membrane superstructure (where cohesive escin and adhesive membrane interact, constituting a heterogeneous quasi-2D system able to undergo mesoscale phase transitions) (refer to Dukhin et al., (1994), for a comprehensive monography on adsorption dynamics at fluid interfaces. Although such a class of condensing interactions, here mediated by solid-like escin, is also frequent in other adhesive systems, such as single sugars (Koster et al., 1994) and small nanoparticles (Salassi et al., 2021; Kariuki et al., 2022), the amphiphilic quadrupole in escin imposes a very particular surface bidirectional cohesivity that makes membrane interactions dual in raising enhanced stability, which can extrinsically adhere more bulk material and intrinsically compact the already inserted material.
As a relevant contribution to membrane mechanics, our complementary measurements of compression modulus (
Our experimental results support the hypothesis of the compacting incorporation of escin, depending not only on the solid or fluid DMPC-phase state, but also on the vectorized direction particularly chosen for escin insertion (longitudinal along membrane traffic or transverse across bulk diffusion). We demonstrated that the pre-existing molecular ordering acted as a mechanical scaffold with a dual mechanical function, a key regulator of membrane rheology. Escin elicited either structural stiffening or dynamic softening, depending on the molecular orientation adopted during the incorporation process (longitudinal or transverse, respectively). When the escin molecules became an integral part of the membrane assembly (which would represent a longitudinal insertion similar to natural bilayer assembly
For physicochemical analysis of the escin–membrane interaction, the amphiphilic molecule escin was presented at a constant submicellar concentration such that membrane insertion occurred from the monomer status (at
Mechanical duality is not rare in complex models of membrane heterogeneity, endowing the compositional mosaicity typical of biological membranes (Finegold, 1992). Likewise, a plausible functional paradigm for mosaic membranes could emerge from the dual membrane energetics observed here for solid-like escin exchanging with the fluid-like lipid membrane. This cohesive energetics was revealed as heterogeneous as locally governed by the condensation status of the exchanging molecules, either extrinsic or intrinsic. Local membrane condensation (cf. mosaicity) determined the adsorption–reorganization kinetics, leading to cohesive diffusivities.
The mechanical duality that emerged from the saponin–phospholipid assembly could play an important role in the therapeutic efficacy of pharmacological procedures involving aglycone moieties interacting with membrane phospholipids. In particular, the β-escin could promote dual cell rigidness, softening, or both at interference with membrane sterols and other structural mediators of membrane rigidness and fluidity (Güçlü-Ustündağ and Mazza, 2007; Sreij et al., 2019; Geisler et al., 2020b; Glickman et al., 2020). The medicinal extract from chestnut seeds (A. hippocastanum), standardized for the content of β-escin, is currently used as an efficient and toxicologically safe oral treatment for CVI (Underland et al., 2012). For years, escin has been proven to be highly bioavailable, non-toxic, safe, and well-tolerated under oral ingestion (Liehn et al., 1972; Loew et al., 2000). No new safety concerns were identified in the updated Cochrane review, published in 2012 (Underland et al., 2012). The usual therapeutic dose is 100 mg of escin per day (Underland et al., 2012), although higher doses, up to 100 times, are admitted as dietary supplements (Pittler and Ernst, 2012). In an adult human, the admitted dosages are on the safety interval
By varying the dose of the membrane-active saponin, its therapeutic efficacy could be subsequently modulated under mechanical changes induced by its vectorized incorporation into the cell membrane (either transverse by extrinsic diffusivity or longitudinal by intrinsic lipid traffic). Therefore, the present results represent a relevant physicochemical insight, opening new avenues for membrane-targeted pharmacological treatments of venous inflammation based on saponins. Mechanically behaving as solids and liquids, these pharmacologically active molecules (phlebotonics), particularly β-escin, could dually render into structural rigidness and fluidity with a therapeutic value in the cellular membranes of the pathogenic venous vasculature.
The original contributions presented in the study are included in the article/supplementary material. Further inquiries can be directed to the corresponding authors.
LM, DH-A, MM-R, NC, CD, and RG conducted research, provided experimental data, and contributed to data. JS, TH, and FM supervised the research and drafted the manuscript. TH and FM supported the search for funding, planned and supervised the research, contributed to data analysis, and wrote the manuscript. All authors contributed to the article and approved the submitted version.
LM was contracted by the María Zambrano Program from Ministerio de Universidades de España for the attraction of international talent under Next-Generation European Union funding (Grant CT19/22). This work was supported by the Spanish Ministry of Science and Innovation (MICINN–Agencia Española de Investigación AEI) under Grants PID 2019-108391RB-100 and TED2021-132296B-C52 (to FM) and Comunidad de Madrid under Grants S2018/NMT-4389 and Y2018/BIO-5207 (to FM). The authors also acknowledge the financial support of the German Research Foundation DFG Grant HE 2995/7-1 (to TH) and the Open Access Publication Fund of Bielefeld University for the publication costs. This study was also funded by the REACT-EU program PR38-21-28 ANTICIPA-CM, a grant by Comunidad de Madrid and the European Union under the FEDER program in response to the COVID-19 pandemic.
The authors acknowledge Prof. Juan J. Giner-Casares for making available Langmuir troughs and the BAM facility in his laboratory at Universidad de Cordoba and also for fruitful discussions on the monolayer domain morphologies. LM gratefully thanks him for hospitality.
The authors declare that the research was conducted in the absence of any commercial or financial relationships that could be construed as a potential conflict of interest.
All claims expressed in this article are solely those of the authors and do not necessarily represent those of their affiliated organizations or those of the publisher, the editors, and the reviewers. Any product that may be evaluated in this article, or claim that may be made by its manufacturer, is not guaranteed or endorsed by the publisher.
Al-Rekabia, Z., and Contera, S. (2018). Multifrequency AFM reveals lipid membrane mechanical properties and the effect of cholesterol in modulating viscoelasticity. Proc. Nat. Acad. Sci. 115, 2658–2663. doi:10.1073/pnas.1719065115
Alonso, A., and Goñi, F. M. (2018). The physical properties of ceramides in membranes. Annu. Rev. Biophys. 47, 633–654. doi:10.1146/annurev-biophys-070317-033309
Arriaga, L. R., López-Montero, I., Ignés-Mullol, J., and Monroy, F. (2010b). Domain-growth kinetic origin of nonhorizontal phase coexistence plateaux in Langmuir monolayers: compression rigidity of a raft-like lipid distribution. J. Phys. Chem. B 114, 4509–4520. doi:10.1021/jp9118953
Arriaga, L. R., López-Montero, I., Orts-Gil, G., Farago, B., Hellweg, T., and Monroy, F. (2009). Fluctuation dynamics of spherical vesicles: frustration of regular bulk dissipation into subdiffusive relaxation. Phys. Rev. E. 80, 031908. doi:10.1103/PhysRevE.80.031908
Arriaga, L. R., López-Montero, I., Rodríguez-García, R., and Monroy, F. (2008). Nonlinear dilational mechanics of Langmuir lipid monolayers: A lateral diffusion mechanism. Phys. Rev. E 77, 061918. doi:10.1103/PhysRevE.77.061918
Arriaga, L. R., Rodriguez-Garcia, R., López-Montero, I., Farago, B., Hellweg, T., and Monroy, F. (2010a). Dissipative curvature fluctuations in bilayer vesicles: coexistence of pure-bending and hybrid curvature-compression modes. Eur. Phys. J. E 31, 105–113. doi:10.1140/epje/i2010-10551-1
Baumgart, T., Hunt, G., Farkas, E. R., Webb, W. W., and Feigenson, G. W. (2007). Fluorescence probe partitioning between Lo/Ld phases in lipid membranes. Biochim. Biophys. Acta 68, 2182–2194. doi:10.1016/j.bbamem.2007.05.012
Böttcher, S., Keppler, J. K., and Drusch, S. (2017). Mixtures of Quillaja saponin and beta-lactoglobulin at the oil/water-interface: adsorption, interfacial rheology and emulsion properties. Colloids Surf. A 518, 46–56. doi:10.1016/j.colsurfa.2016.12.041
Böttcher, S., and Drusch, S. (2017). Saponins — self-assembly and behavior at aqueous interfaces. Adv. Colloid Interf. Sci. 243, 105–113. doi:10.1016/j.cis.2017.02.008
Brunauer, S., Emmett, P. H., and Teller, E. (1938). Adsorption of gases in multimolecular layers. J. Am. Chem. Soc. 60, 309–319. doi:10.1021/ja01269a023
Buttler, J. A. V. (1932). The thermodynamics of the surfaces of the solutions. Proc. R. Soc. A 138, 348. doi:10.1098/rspa.1932.0040
Castro, B. M., Prieto, M., and Silva, L. C. (2014). Ceramide: A simple sphingolipid with unique biophysical properties. Prog. Lipid Res. 54, 53–67. doi:10.1016/j.plipres.2014.01.004
Catapano, E. R., Arriaga, L. R., Espinosa, G., Monroy, F., Langevin, D., and López-Montero, I. (2011). Solid character of membrane ceramides: A surface rheology study of their mixtures with sphingomyelin. Biophys. J. 101, 2721–2730. doi:10.1016/j.bpj.2011.10.049
Catapano, E. R., Natale, P., Monroy, F., and López-Montero, I. (2017). The enzymatic sphingomyelin to ceramide conversion increases the shear membrane viscosity at the air-water interface. Adv. Colloid. Interf. Sci. 247, 555–560. doi:10.1016/j.cis.2017.07.014
Cheok, C. Y., Salman, H. A. K., and Sulaiman, R. (2014). Extraction and quantification of saponins: A review. Food Res. Int. 59, 16–40. doi:10.1016/j.foodres.2014.01.057
Cogolludo, A., Villamor, E., Perez-Vizcaino, F., and Moreno, L. (2019). Ceramide and regulation of vascular tone. Int. J. Mol. Sci. 20, 411. doi:10.3390/ijms20020411
Dargel, C., Geisler, R., Hannappel, Y., Kemker, I., Sewald, N., and Hellweg, T. (2019). Self-assembly of the biosurfactant aescin in solution: A small-angle X-ray scattering and fluorescence study. Colloids Interf. 3, 47. doi:10.3390/colloids3020047
De Groot, C., Müsken, M., and Müller-Goymann, C. C. (2018). Novel colloidal microstructures of β-escin and the liposomal components cholesterol and DPPC. Planta Medica 84, 1219–1227. doi:10.1055/a-0624-2706
Deseri, L. (2017). “Elasticity and hereditariness,” in The role of mechanics in the study of lipid bilayers. CISM international centre for mechanical sciences. Editor E. J. Steigmann (Cham: Springer). doi:10.1007/978-3-319-56348-0_2
Díez-Pascual, A. M., Monroy, F., Ortega, F., Rubio, R. G., Miller, R., and Noskov, B. A. (2007). Adsorption of water-soluble polymers with surfactant character. Dilational viscoelasticity. Dilational Viscoelasticity Langmuir 23, 3802–3808. doi:10.1021/la062936c
Dudek-Makuch, M., and Studzińska-Sroka, E. (2015). Horse chestnut – efficacy and safety in chronic venous insufficiency: an overview. Rev. Bras. Farmacogn. 25, 533–541. doi:10.1016/j.bjp.2015.05.009
Dukhin, S. S., Kretzschmar, G., and Miller, R. (1994). Dynamics of adsorption at liquid interfaces: Theory, experiment, application. Amsterdam, Netherlands: Elsevier.
Elekofehinti, O. O., Iwaloye, O., Olawale, F., and Ariyo, E. O. (2021). Saponins in cancer treatment: current progress and future prospects. Pathophysiology 28, 250–272. doi:10.3390/pathophysiology28020017
Espinosa, G., López-Montero, I., Monroy, F., and Langevin, D. (2011). Shear rheology of lipid monolayers and insights on membrane fluidity. Proc. Nat. Acad. Sci. 108, 6008–6013. doi:10.1073/pnas.1018572108
Evans, E. A., and Hochmuth, R. M. (1976). Membrane viscoelasticity. Biophysical J. 16, 1–11. doi:10.1016/S0006-3495(76)85658-5
Fainerman, V. B., and Vollhardt, D. (1999). Equations of state for Langmuir monolayers with two-dimensional phase transitions. J. Phys. Chem. B 103, 145–150. doi:10.1021/jp983109q
Gallelli, L. (2019). A review of its anti-edematous, anti-inflammatory, and venotonic properties. Drug Des. devel. Ther. 13, 3425–3437. doi:10.2147/DDDT.S207720
Gauthier, C., Legault, J., Girard-Lalancette, K., Mshvildadze, V., and Pichette, A. (2009). Haemolytic activity, cytotoxicity and membrane cell permeabilization of semi-synthetic and natural lupane- and oleanane-type saponins. Bioorg. Med. Chem. 17, 2002–2008. doi:10.1016/j.bmc.2009.01.022
Geisler, R., Dargel, C., and Hellweg, T. (2020a). The biosurfactant β-aescin: A review on the physico-chemical properties and its interaction with lipid model membranes and Langmuir monolayers. Molecules 25, 117. doi:10.3390/molecules25010117
Geisler, R., Pedersen, M. C., Hannappel, Y., Schweins, R., Prévost, S., Dattani, R., et al. (2019). Aescin-induced conversion of gel-phase lipid membranes into bicelle-like lipid nanoparticles. Langmuir 35, 16244–16255. doi:10.1021/acs.langmuir.9b02077
Geisler, R., Prévost, S., Dattani, R., and Hellweg, T. (2020b). Effect of cholesterol and ibuprofen on DMPC-β-aescin bicelles: A temperature-dependent wide-angle X-ray scattering study. Crystals 10, 401. doi:10.3390/cryst10050401
Glickman, D., García Rey, N., Richert, M., Meister, K., and Braunschweig, B. (2020). pH effects on the molecular structure and charging state of β-Escin biosurfactants at the air-water interface. J. Colloid Interface Sci. 607, 1754–1761. doi:10.1016/j.jcis.2021.09.086
Golemanov, K., Tcholakova, S., Denkov, N., Pelan, E., and Stoyanov, S. D. (2013). Remarkably high surface viscoelasticity of adsorption layers of triterpenoid saponins. Soft Matter 9, 5738–5752. doi:10.1039/C3SM27950B
Golemanov, K., Tcholakova, S., Denkov, N., Pelan, E., and Stoyanov, S. D. (2014). The role of the hydrophobic phase in the unique rheological properties of saponin adsorption layers. Soft Matter 10, 7034–7044. doi:10.1039/C4SM00406J
Graham, D. E., and Phillips, C. (1979). Proteins at liquid interfaces. J. Colloid Interface Sci. 70, 403–414. doi:10.1016/0021-9797(79)90048-1
Güçlü-Ustündağ, O., and Mazza, G. (2007). Saponins: properties, applications and processing. Crit. Rev. Food Sci. Nutr. 47, 231–258. doi:10.1080/10408390600698197
Gwozdzinski, L., Bernasinska-Slomczewska, J., Hikisz, P., Wiktorowska-Owczarek, A., Kowalczyk, E., and Pieniazek, A. (2023). The effect of diosmin, escin, and bromelain on human endothelial cells derived from the umbilical vein and the varicose vein: A preliminary study. Biomedicines 11, 1702. doi:10.3390/biomedicines11061702
Helfrich, W. (1973). Elastic properties of lipid bilayers — theory and possible experiments. Z. Naturforsch C 28, 693–703. doi:10.1515/znc-1973-11-1209
Hermans, E., and Vermant, J. (2014). Interfacial shear rheology of DPPC under physiologically relevant conditions. Soft Matter 10, 175–186. doi:10.1039/C3SM52091A
Hill, R. M., and Dissado, L. A. (1985). Debye and non-Debye relaxation. J. Phys. C. Solid State Phys. 18, 3829–3836. doi:10.1088/0022-3719/18/19/021
Hormel, T. T., Kurihara, S. Q., Brennan, M. K., Wozniak, M. C., and Parthasarathy, R. (2014). Measuring lipid membrane viscosity using rotational and translational probe diffusion. Phys. Rev. Lett. 112, 188101. doi:10.1103/PhysRevLett.112.188101
Jeon, J., and Voth, G. A. (2005). The dynamic stress responses to area change in planar lipid bilayer membranes. Biophys. J. 88, 1104–1119. doi:10.1529/biophysj.104.052183
Kariuki, R., Penman, R., Bryant, S. J., Orrell-Trigg, R., Meftahi, N., Crawford, R. J., et al. (2022). Behavior of citrate-capped ultrasmall gold nanoparticles on a supported lipid bilayer interface at atomic resolution. ACS Nano 16, 17179–17196. doi:10.1021/acsnano.2c07751
Kharbedia, M., Caselli, N., López-Menéndez, H., Enciso, E., Santiago, J. A., Herráez-Aguilar, D., et al. (2021). Moulding hydrodynamic 2D-crystals upon parametric Faraday waves in shear-functionalized water surfaces. Nat. Commun. 12, 1130. doi:10.1038/s41467-021-21403-0
Koczurkiewicz, P., Czyż, J., Podolak, I., Wójcik, K., Galanty, A., Janeczko, Z., et al. (2015). Multidirectional effects of triterpene saponins on cancer cells - mini-review of in vitro studies. Acta Biochim. Pol. 62, 383–393. doi:10.18388/abp.2015_1089
Koster, K. L., Webb, M. S., Bryant, G., and Lynch, D. V. (1994). Interactions between soluble sugars and POPC (1-palmitoyl-2-oleoylphosphatidylcholine) during dehydration: vitrification of sugars alters the phase behavior of the phospholipid. Acta – Biomembr. 1193, 143–150. doi:10.1016/0005-2736(94)90343-3
Langevin, D., and Monroy, F. (2014). Marangoni stresses and surface compression rheology of surfactant solutions. Achievements and problems. Adv. Colloid. Interf. Sci. 206, 141–149. doi:10.1016/j.cis.2014.01.006
Le Roux, A. L., Quiroga, X., Walani, N., Arroyo, M., and Roca-Cusachs, P. (2019). The plasma membrane as a mechanochemical transducer. Phil. Trans. R. Soc. B 374, 20180221. doi:10.1098/rstb.2018.0221
Lheveder, C., Meunier, J., and Henon, S. (2000). “Brewster angle microscopy,” in Physical Chemistry of biological interfaces. Editors A. Baszkin, and W. Norde (New York City: Marcel Dekker Inc.).
Liehn, H. D., Franco, P. A., Hampel, H., and Hofrichter, G. (1972). A toxicological study of extractum Hippocastani semen (EHS). Panminerva Med. 14, 84–91. PMID: 4681124.
Liu, Z., and Kim, C. (2020). Deformation analysis of lipid membranes subjected to general forms of intra-membrane viscous flow and interactions with an elliptical-cross-section substrate. Sci. Rep. 10, 478. doi:10.1038/s41598-019-57179-z
Loew, D., Schrödter, A., Schwankl, W., and März, R. W. (2000). Measurement of the bioavailability of aescin-containing extracts. Methods Find. Exp. Clin. Pharmacol. 22, 537–542. doi:10.1358/mf.2000.22.7.802264
López, D. J., Egido-Gabas, M., López-Montero, I., Busto, J. V., Casas, J., Garnier, M., et al. (2012). Accumulated bending energy elicits neutral sphingomyelinase activity in human red blood cells. Biophys. J. 102, 2077–2085. doi:10.1016/j.bpj.2012.03.020
López-Montero, I., Arriaga, L. R., Monroy, F., Rivas, G., Tarazona, P., and Vélez, M. (2008). High fluidity and soft elasticity of the inner membrane of Escherichia coli revealed by the surface rheology of model Langmuir monolayers. Langmuir 24, 4065–4076. doi:10.1021/la703350s
López-Montero, I., Catapano, E. R., Espinosa, G., Arriaga, L. R., Langevin, D., and Monroy, F. (2013). Shear and compression rheology of Langmuir monolayers of natural ceramides: solid character and plasticity. Langmuir 29, 6634–6644. doi:10.1021/la400448x
López-Montero, I., Monroy, F., Vélez, M., and Devaux, P. F. (2010). Ceramide: from lateral segregation to mechanical stress. Biochim. Biophys. Acta – Biomembr. 1798, 1348–1356. doi:10.1016/j.bbamem.2009.12.007
Lorent, J. H., Quetin-Leclercq, J., and Mingeot-Leclercq, M. P. (2014). The amphiphilic nature of saponins and their effects on artificial and biological membranes and potential consequences for red blood and cancer cells. Org. Biomol. Chem. 12, 8803–8822. doi:10.1039/c4ob01652a
Marsh, D. (1996). Lateral pressure in membranes. Biochim. Biophys. Acta - Biomembr. 1286, 183–223. doi:10.1016/S0304-4157(96)00009-3
Mathivet, L., Cribier, S., and Devaux, P. F. (1996). Shape change and physical properties of giant phospholipid vesicles prepared in the presence of an AC electric field. Biophys. J. 70, 1112–1121. doi:10.1016/S0006-3495(96)79693-5
Mell, M., Moleiro, L. H., Hertle, Y., López-Montero, I., Cao, F. J., Fouquet, P., et al. (2015). Fluctuation dynamics of bilayer vesicles with intermonolayer sliding: experiment and theory. Chem. Phys. Lipids 185, 61–77. doi:10.1016/j.chemphyslip.2014.11.005
Miyakoshi, M., Tamura, Y., Masuda, H., Mizutani, K., Tanaka, O., Ikeda, T., et al. (2000). Antiyeast steroidal saponins from Yucca schidigera (Mohave Yucca), a new anti-food-deteriorating agent. J. Nat. Prod. 63, 332–338. doi:10.1021/np9904354
Monroy, F., Giermanska-Kahn, J., and Langevin, D. (1998). Dilational viscoelasticity of surfactant monolayers. Colloids Surfaces A 143, 251–260. doi:10.1016/S0927-7757(98)00373-2
Muñoz, M. G., Monroy, F., Ortega, F., Rubio, R. G., and Langevin, D. (2000). Monolayers of symmetric triblock copolymers at the air−water interface. 2. Adsorption kinetics. Langmuir 16, 1094–1101. doi:10.1021/la9901433
Mussel, M., and Schneider, M. F. (2019). It sounds like an action potential: unification of electrical, chemical and mechanical aspects of acoustic pulses in lipids. J. R. Soc. Interface 16, 20180743. doi:10.1098/rsif.2018.0743
Penfold, J., Thomas, R. K., Tucker, I., Petkov, J. T., Stoyanov, S. D., Denkov, N., et al. (2018). Saponin adsorption at the air-water interface-neutron reflectivity and surface tension study. Langmuir 34, 9540–9547. doi:10.1021/acs.langmuir.8b02158
Pethica, B. A. (1955). The thermodynamics of monolayer penetration at constant area. Part 1. Trans. Faraday Soc. 51, 1402. doi:10.1039/TF9555101402
Philips, R., Ursell, T., Wiggins, P., and Sens, P. (2009). Emerging roles for lipids in shaping membrane-protein function. Nature 459, 379–385. doi:10.1038/nature08147
Pittler, M. H., and Ernst, E. (2012). Horse chestnut seed extract for chronic venous insufficiency. Cochrane Database Syst. Rev. 11, Cd003230. doi:10.1002/14651858.CD003230.pub4
Rahimi, M., and Arroyo, M. (2012). Shape dynamics, lipid hydrodynamics, and the complex viscoelasticity of bilayer membranes. Phys. Rev. E 86, 011932. doi:10.1103/PhysRevE.86.011932
Salassi, S., Caselli, L., Cardellini, J., Lavagna, E., Montis, C., Berti, D., et al. (2021). A Martini coarse grained model of citrate-capped gold nanoparticles interacting with lipid bilayers. J. Chem. Theory Comput. 17, 6597–6609. doi:10.1021/acs.jctc.1c00627
Santiago, J. A., Chacón-Acosta, G., and Monroy, F. (2019). Membrane stress and torque induced by frank's nematic textures: A geometric perspective using surface-based constraints. Phys. Rev. E 100, 012704. doi:10.1103/PhysRevE.100.012704
Santiago, J. A., and Monroy, F. (2020). Mechanics of nematic membranes: euler–lagrange equations, noether charges, stress, torque and boundary conditions of the surface frank's nematic field. J. Phys. A. Math. Theor. 53, 165201. doi:10.1088/1751-8121/ab7cff
Schmid, F. (2013). Fluctuations in lipid bilayers. Biophys. Rev. Lett. 8, 1–20. doi:10.1142/S1793048012300113
Seifert, U. (1997). Configurations of fluid membranes and vesicles. Adv. Phys. 46, 13–137. doi:10.1080/00018739700101488
Serrien, G., Geeraerts, G., Ghosh, L., and Joos, P. (1992). Dynamic surface properties of adsorbed protein solutions: BSA, casein and buttermilk. Colloids Surf. A 68, 219–233. doi:10.1016/0166-6622(92)80208-J
Simons, K., and Ikonen, E. (1997). Functional rafts in cell membranes. Nature 387, 569–572. doi:10.1038/42408
Singer, S. J., and Nicolson, G. L. (1972). The fluid mosaic model of the structure of cell membranes. Science 175, 720–731. doi:10.1126/science.175.4023.720
Singh, M. K., Shweta, H., Khan, M. F., and Sen, S. (2016). New insight into probe-location dependent polarity and hydration at lipid/water interfaces: comparison between gel- and fluid-phases of lipid bilayers. Phys. Chem. Chem. Phys. 18, 24185–24197. doi:10.1039/C6CP01201A
Sirtori, C. R. (2001). Aescin: pharmacology, pharmacokinetics and therapeutic profile. Pharmacol. Res. 44, 183–193. doi:10.1006/phrs.2001.0847
Smith, A. R., Visioli, F., Frei, B., and Hagen, T. M. (2006). Age-related changes in endothelial nitric oxide synthase phosphorylation and nitric oxide dependent vasodilation: evidence for a novel mechanism involving sphingomyelinase and ceramide-activated phosphatase 2a. Aging Cell 5, 391–400. doi:10.1111/j.1474-9726.2006.00232.x
Sollich, P., Lequeux, F., Hébraud, P., and Cates, M. E. (1997). Rheology of soft glassy materials. Phys. Rev. Lett. 78, 2020–2023. doi:10.1103/PhysRevLett.78.2020
Sparg, S. G., Light, M. E., and van Staden, J. (2004). Biological activities and distribution of plant saponins. J. Ethnopharmacol. 94, 219–243. doi:10.1016/j.jep.2004.05.016
Sprong, H., Van der Sluijs, P., and Van Meer, G. (2001). How proteins move lipids and lipids move proteins. Nat. Rev. Mol. Cell. Biol. 2, 504–513. doi:10.1038/35080071
Sreij, R., Dargel, C., Geisler, P., Hertle, Y., Radulescu, A., Pasini, S., et al. (2018). DMPC vesicle structure and dynamics in the presence of low amounts of the saponin aescin. Phys. Chem. Chem. Phys. 20, 9070–9083. doi:10.1039/C7CP08027A
Sreij, R., Dargel, C., Moleiro, L. H., Monroy, F., and Hellweg, T. (2017). Aescin incorporation and nanodomain formation in DMPC model membranes. Langmuir 33, 12351–12361. doi:10.1021/acs.langmuir.7b02933
Sreij, R., Dargel, C., Schweins, R., Prévost, S., Dattani, R., and Hellweg, T. (2019). Aescin-cholesterol complexes in DMPC model membranes: A dsc and temperature-dependent scattering study. Sci. Rep. 9, 5542. doi:10.1038/s41598-019-41865-z
Srivastava, S., Leiske, D., Basu, J. K., and Fuller, G. G. (2011). Interfacial shear rheology of highly confined glassy polymers. Soft Matter 7, 1994–2000. doi:10.1039/C0SM00839G
Tse, J. M., Cheng, G., Tyrrell, J. A., Wilcox-Adelman, S. A., Boucher, Y., Jain, R. K., et al. (2012). Mechanical compression drives cancer cells toward invasive phenotype. Proc. Nat. Acad. Sci. 109, 911–916. doi:10.1073/pnas.1118910109
Underland, V., Sæterdal, I., and Strømme Nilsen, E. (2012). Cochrane summary of findings: horse chestnut seed extract for chronic venous insufficiency. Glob. Adv. Health Med. 1, 122–123. doi:10.7453/gahmj.2012.1.1.018
Van Meer, G., Voelker, D. R., and Feigenson, G. W. (2008). Membrane lipids: where they are and how they behave. Nat. Rev. Mol. Cell. Biol. 9, 112–124. doi:10.1038/nrm2330
Vorselen, D., Van Dommelen, S. M., Sorkin, R., Roos, W. H., Schiller, J., Döpp, S. T., et al. (2018). The fluid membrane determines mechanics of erythrocyte extracellular vesicles and is softened in hereditary spherocytosis. Nat. Commun. 9, 4960. doi:10.1038/s41467-018-07445-x
WH de Jeu (1980). Physical properties of liquid crystalline materials. 1. Philadelphia, Pennsylvania, United States: Gordon and Breach Science.
Wu, S. H., Sankhagowit, S., Biswas, R., Wu, S., Povinelli, M. L., and Malmstadt, N. (2015). Viscoelastic deformation of lipid bilayer vesicles. Soft Matter 11, 7385–7391. doi:10.1039/c5sm01565k
Keywords: β-escin, membrane phospholipids, model lipid membranes, Langmuir monolayers, bilayer vesicles, adsorption kinetics, fluorescence microscopy, Brewster angle microscopy
Citation: Moleiro LH, Martín-Romero MT, Herráez-Aguilar D, Santiago JA, Caselli N, Dargel C, Geisler R, Hellweg T and Monroy F (2023) Dual mechanical impact of β-escin on model lipid membranes. Front. Soft Matter 3:1240878. doi: 10.3389/frsfm.2023.1240878
Received: 15 June 2023; Accepted: 22 August 2023;
Published: 08 September 2023.
Edited by:
Jordi Ignés-Mullol, University of Barcelona, SpainReviewed by:
Natalia Wilke, National University of Cordoba (CIQUIBIC), ArgentinaCopyright © 2023 Moleiro, Martín-Romero, Herráez-Aguilar, Santiago, Caselli, Dargel, Geisler, Hellweg and Monroy. This is an open-access article distributed under the terms of the Creative Commons Attribution License (CC BY). The use, distribution or reproduction in other forums is permitted, provided the original author(s) and the copyright owner(s) are credited and that the original publication in this journal is cited, in accordance with accepted academic practice. No use, distribution or reproduction is permitted which does not comply with these terms.
*Correspondence: Thomas Hellweg, dGhvbWFzLmhlbGx3ZWdAdW5pLWJpZWxlZmVsZC5kZQ==; Francisco Monroy, bW9ucm95QHVjbS5lcw==
Disclaimer: All claims expressed in this article are solely those of the authors and do not necessarily represent those of their affiliated organizations, or those of the publisher, the editors and the reviewers. Any product that may be evaluated in this article or claim that may be made by its manufacturer is not guaranteed or endorsed by the publisher.
Research integrity at Frontiers
Learn more about the work of our research integrity team to safeguard the quality of each article we publish.