- UNAM-National Nanotechnology Research Center and Institute of Materials Science & Nanotechnology, Bilkent University, Ankara, Turkey
DNA is the ultimate molecule that encodes life through genes. Genes positioned along the meter-long DNA molecule have to be expressed at the right time and in the right amount via strict regulatory processes. Regulation of a gene starts with the binding of a DNA-binding protein known as a transcription factor (TF) to a target regulatory element along the double-stranded DNA molecule. Often, TFs attach to DNA in a sequence-specific manner and can target DNA motifs of various lengths, yet some TFs can also interact with DNA nonspecifically. The resulting DNA-TF complexes can control gene expression directly via controlling the recruitment of RNA polymerase on the target DNA sequence. Recent single-molecule experiments have added a new dimension to such control mode; the lifetime of a DNA-TF complex (i.e., the residence time of the protein on its DNA site) can function as a regulatory component. This breakthrough inevitably suggests that any physiochemical constituent that can alter the residence time of a DNA-binding protein can also be involved in gene regulation. In this perspective, we argue that a TF protein’s cellular concentration can contribute to the cell-scale transcription activity by modulating the DNA-residence time of the protein. Cells can achieve this either by enabling a concentration-dependent dissociation mechanism or by promoting the formation of multiprotein-DNA complexes. While our discussion here will consider examples from prokaryotic cells, we will also briefly argue that similar mechanisms could also be functional in eukaryotic cells.
Introduction
Transcription Factors (TFs) dynamically regulate gene expression through temporal interactions with regulatory DNA elements. The nascent understanding of transcriptional regulation at a single target site level has gathered speed in parallel to the advancements in single-molecule studies and involves DNA-residence times of TFs as a regulatory component (Lickwar et al., 2012; Clauß et al., 2017). The DNA-residence time of a TF, or in general of a DNA-binding protein, is defined as the elapsed time between the initial binding of the protein to its DNA target sequence and its complete microscopic unbinding from the same DNA sequence.
The DNA-binding process of a TF in the available cellular volume is mainly diffusion-controlled (Halford and Marko, 2004). A TF can bind to its target site directly from the phase solution (i.e., cytoplasm or nucleoplasm). Alternatively, a TF can migrate from previously bound nonspecific neighboring sites to the target binding sites via 1 d sliding. A TF can also jump or be transferred between juxtaposed DNA segments. Yet, this segmental jump (or intersegmental transfer) only occurs in a subset of proteins that have multiple binding domains since it requires the TF to be concurrently bound to multiple DNA segments. Regardless of how the protein arrives at its target site, the probability of DNA binding often depends linearly on the protein concentration (Halford and Marko, 2004).
Contrarily, the mechanism of unbinding (or dissociation) of a TF from its binding site has been considered a concentration-independent process until recently. The classical text view assumes that the TF dissociation from DNA to occur solely due to thermal fluctuations that help the protein overcome the binding-energy barrier (Berg, 1993). For the same reason, only concentration dependence on the dissociation constant (i.e., Kd) is assumed to come from the on-rate (i.e., association rate constant). However, recent single-molecule experiments and molecular dynamics studies have marked the discovery of a concentration-dependent mechanism that affects unbinding rates of certain DNA-binding proteins, thus, their DNA-residence times (Graham et al., 2011; Joshi et al., 2012; Gibb et al., 2014; Sing et al., 2014; Kamar et al., 2017) Accordingly, a tightly DNA-bound TF can microscopically dissociate (e.g., by partially losing steric contacts with the DNA via unbinding of one of its DNA-binding domains) and expose the binding site temporally. During this exposure, a solution-phase protein (or a DNA segment) can bind to this DNA-TF complex. If the resulting ternary complex is less stable (Tsai et al., 2016), it can accelerate the dissociation of the originally DNA-bound TF. This competition-driven dissociation is referred to as “Facilitated Dissociation” (FD) (Kamar et al., 2017; Erbaş and Marko, 2019). FD becomes more effective as the solution-phase concentration of competitors is increased since a higher concentration suggests a greater binding probability for the competing molecular species to the exposed site (Figure 1). This concentration dependency of FD could lead to a wide range of residence times for different concentration-variation patterns as some proteins have only a few hundred copies per cell (i.e., with a concentration of <100 nM) while others might vary their cellular levels more than 1000-fold depending on the growth phase or external stimuli (Azam et al., 1999; Dillon and Dorman, 2010). Therefore, TFs, whose abundance fluctuates in a dynamic manner, can utilize FD to change their residence times and thus possibly their regulatory roles dynamically.
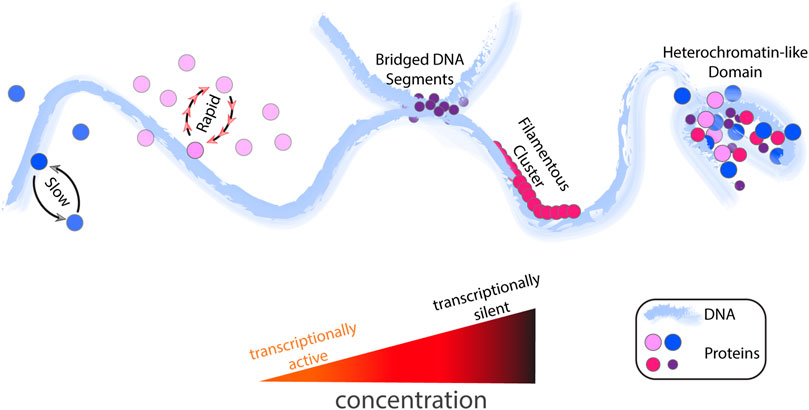
FIGURE 1. A schematic representation of DNA-protein interactions and cluster formations in response to an increase in protein concentration. From left to right, protein concentration increases. In response, unbinding becomes more rapid. At the more elevated TF concentrations, the protein-DNA complexes (or clusters) are formed. The combination of various DNA-binding proteins can lead to the formation of transcriptionally silent extended protein occupancy domains (EPODs) (i.e., heterochromatin-like domains). Note that, for simplicity, different concentration stages are shown on the same DNA polymer, but each event might also occur in distinct stages of the cell cycle.
FD can lead to a broader spectrum of residence times under large concentration fluctuations (e.g., ∼10 μM), and bacterial nucleoid-associated proteins (NAPs), another type of DNA-binding proteins, can provide such micromolar level concentration variations (Azam et al., 1999). While NAPs are involved in the 3 d organization of nucleoid architecture, they can also regulate transcription due to their dual-purpose properties both as TFs and structural proteins (Dillon and Dorman, 2010; Dorman, 2013). For example, the Factor for Inversion Simulation, Fis, is a dual-function NAP that regulates over 300 genes in E. coli (Bradley et al., 2007), and its cellular levels fluctuate drastically from vanishing levels to tens of thousands of copies per cell (Ball et al., 1992). Indeed, Fis is one of the NAPs that repeatedly exhibit obvious FD patterns in vitro by dropping its residence time from hours to minutes following concentration increases on the order of ∼100 nM (Graham et al., 2011; Hadizadeh et al., 2016; Kamar et al., 2017). Thus, in principle, each concentration level could correspond to a unique residence time; moreover, each residence time can regulate a different set of genes on different target sites, as previously demonstrated for yeast Abf1 TF (de Jonge et al., 2020), or lead to different transcript levels (Callegari et al., 2019).
How can the DNA-residence time of a TF affect the transcription of a gene at the molecular scale? Despite the lack of direct evidence from bacterial DNA-binding proteins, the studies on eukaryotic TFs highlighted several key molecular mechanisms. For instance, repression of a gene can be achieved by long residence times of a repressor TF such that RNA polymerase is sterically hindered from transcribing the DNA sequence (Lickwar et al., 2012). The extended DNA-residence times can also be functional to generate a continuous gene-expression effect (Gurdona et al., 2020). Also, a repressor TF can stall a transcribing RNA polymerase (Clauß et al., 2017). Moreover, a TF quickly exchanging with identical solution-phase TFs can lead to a shorter residence time on average and provide a repression mechanism by preventing the stable binding of RNA polymerase or protein cofactors (Trojanowski et al., 2022). Indeed, this suggests that an efficient activator TF should stay on the target sequence for an optimal amount of time such that RNA polymerase (or other protein cofactors) can be recruited to the binding site to initiate the transcription (Popp et al., 2021).
It is intuitive to think that a single TF can achieve all or some of the residence-time-controlled regulatory modes that we mention above via FD by modulating its cellular concentration levels. For instance, bacterial Fis binds to multiple fairly similar sites in a sequence-specific manner (Shao et al., 2008) and regulates a plethora of genes (Bradley et al., 2007). Thus, activation of a gene by Fis could be altered by manipulating Fis residence times on various promoters via FD. In addition, since mRNA expression could be highly dependent on the residence time of an activator TF (Lickwar et al., 2012), there might also be a nonlinear relation where increasing protein levels could result in further competition and do not enhance transcription activity. For instance, increasing TF concentration may decrease the DNA-residence time of the TF so radically that the gene expression levels might drop due to rapid exchange between DNA-bound and unbound TFs despite the TF’s activatory role (Figure 1). Such a scenario would also allow a switch mechanism for negative feedback loops. For instance, Fis can regulate its own transcription negatively. Hence, when Fis levels reach a critical level, Fis-residence time could be too short to enable Fis to repress its own transcription.
While TFs are often depicted as sequence-specific DNA-binding proteins, they can also bind to nonspecific regions on DNA. Nonspecific interactions between TFs and DNA depend on the TF’s chemical composition, and they are, in principle, weaker compared to the strength of specific interactions (Agback et al., 1998). Some DNA-binding proteins interact with DNA solely in a sequence-independent manner, while some interact only sequence-dependently or both ways (Dillon and Dorman, 2010). Indeed, the ability of such TFs to bind DNA results in protein-dense multiprotein DNA complexes, and the formation of such complexes is correlated with TF concentration. For instance, Fis can coat DNA by binding to DNA nonspecifically and form DNA inter-segmental bridges at micromolar concentrations (Schneider et al., 2001; Skoko et al., 2006). Such protein-dense domains (i.e., extended protein occupancy domains) (Figure 1) in bacteria seem to mainly contain transcriptionally inactive genes, which were suggested to have a role similar to heterochromatin domains in eukaryotic cells (Amemiya et al., 2022). As Fis concentration changes drastically throughout the cell cycle, this repressive activity might be altered via FD by making these domains less or more stable by allowing exchange between DNA-bound and unbound proteins.
Not only Fis but other highly abundant nonspecifically binding NAP, such as HU and H-NS, are also involved in gene regulation indirectly by forming such dense domains (Lucchini et al., 2006; Hammel et al., 2016; Remesh et al., 2020) even though they have no direct regulatory functions over genes (Stojkova et al., 2019). A recent simulation study with a coarse-grained bacterium model consisting of a high-molecular-weight DNA polymer and multivalent TFs demonstrated the distinct unbinding strategy preferences of specific and nonspecific DNA-binding proteins (Koşar et al., 2022). Those simulations suggested that the dissociation mechanism that a TF employs depends on its nonspecificity. For nonspecific proteins, 1d sliding is by far the most dominant way of moving along the genome, while specific proteins usually utilize jumps between DNA segments. Notably, 3d escape is rare in all cases (Koşar et al., 2022), which is consistent with the mostly DNA-bound nature of such NAPs in vivo (Stracy et al., 2021).
In short, a DNA-bound TF can alter gene regulation either by blocking a gene sterically or recruiting other transcriptional components to the target site. It is very likely that both mechanisms are correlated with the concentration levels of corresponding TFs. Further, the distinct nature of TF binding to DNA, whether specific or nonspecific, can influence the FD response of the protein to concentration variations. In molecular dynamics simulations, specific DNA-binding proteins display typical FD behavior (i.e., the higher the concentration is, the shorter the DNA-residence time is), nonspecific proteins, on the other hand, show more stable DNA-binding and more resistance against binding competition and thus exhibit longer average residence times as the protein levels are increased (Koşar et al., 2022). This could be due to filamentous structures (Figure 1) formed by nonspecific proteins (e.g., HU or H-NS) that could limit the 1d sliding of DNA-bound proteins to neighboring DNA sites.
Besides FD, another way that TF concentration could impact gene expression is through alterations in the 3d chromosome organization if the corresponding TF has structural roles. Indeed, in eukaryotes, histone proteins can shape chromosomes (Bannister and Kouzarides, 2011) and lead to the formation of histone-dense regions, which are often attributed to transcriptionally inactive regions (Elgin and Grewal, 2003). NAPs can play similar roles in the chromosomal organization (Dillon and Dorman, 2010; Lioy et al., 2018; Yildirim et al., 2018). Experimental evidence suggests that the concentration increase of a NAP is correlated with the formation of dense multiprotein-DNA complexes that can compact the DNA (van Noort et al., 2004; Lin et al., 2012; Macvanin et al., 2012; Winardhi et al., 2015; Hancock et al., 2016; Verma et al., 2019). Several molecular dynamics studies confirmed that micromolar-range increases in NAP levels enhance nucleoid compaction by inducing various protein-DNA structures from bridges to bundles and filaments (Figure 1) as expected (Brackley et al., 2013; Koşar et al., 2022). Consistently, a very recent experimental study has demonstrated the regulatory effects of such multiprotein-DNA complexes in living E. coli (Amemiya et al., 2022). Notably, the different protein-DNA complexes and resulting structures could have different regularity strengths, as demonstrated for H-NS TFs, for which bridged-filamentous structures are more efficient in transcription repression than isolated filaments (Singh et al., 2014; Kotlajich et al., 2015). Thus, it is also plausible that specific and nonspecific proteins give rise to non-uniform compaction throughout the nucleoid, where some chromosomal domains are more protein occupied than others. This heterogeneous protein distribution could simultaneously result in transcriptionally silent or active regions (due to different forms of DNA-protein structures) even for a single TF type and can dictate various transcriptional roles for the same TF.
Another way of controlling gene regulation via alterations in protein levels could be due to liquid-liquid phase separation (LLPS). The LLPS enables rapid formation or dissolving of biomolecular condensates, which can carry out specialized tasks. These structures of liquid droplets have recently drawn significant attention due to their diverse and crucial cellular functions (Brangwynne et al., 2015). As very well-known from polymer physics, the formation of transcriptional condensates depends on concentrations of constituting components such as TF, RNA polymerase, and coactivators (Li et al., 2012; Strom et al., 2017). In bacteria, similar TF and RNA polymerase rich structures have been reported in growing cells (Azam et al., 2000; Wang et al., 2011), which are in accord with gene-rich, presumably protein-driven chromosome structures (Lioy et al., 2018). Interestingly, a very recent study investigating the correlation between such phase-separated condensates and transcription activation does not observe a clear difference in transcript levels between TF-rich and poor liquid droplets (Trojanowski et al., 2022). However, such condensates could be more effective in gene repression, or they can possibly accelerate the mRNA production by making other transcription components immediately available in a small membraneless structure.
Conclusion
In the classical picture of bacterial transcription activation, as the concentration of a TF increases, more TF can bind to promoter sites, and in turn, the cell can increase corresponding mRNA levels. Recent single-molecule experiments on protein-DNA interaction kinetics discovered that a protein’s binding stability on DNA (i.e., its DNA-residence time) changes with increasing protein levels. Thus, higher protein levels may not necessarily correspond to higher transcript levels. This notion is in conjecture with recent experiments, in which DNA-residence times have emerged as an essential regulator component of the transcription (Lickwar et al., 2012; Clauß et al., 2017), making TF unbinding kinetics as vital as binding kinetics. In this perspective article, by considering bacterial NAPs as our case system, we argue that large concentration fluctuations (e.g., ∼10 μM) can result in a broad range of regulatory responses by altering DNA-residence times of highly abundant TFs. We also argue that, in the case of dual-purpose bacterial TFs that have both transcriptional and structural roles, TF levels can modulate the exposure of a gene to transcription-related proteins by changing the chromosomal structure in a concentration-dependent manner and thus function as a regulatory component. Consistently, during the preparation of this article, an experimental study demonstrated the possibility of such regulation in E. coli (Amemiya et al., 2022). For repressive roles of a TF, higher protein levels can coat gene-rich regions via binding to DNA nonspecifically. Such protein-dense DNA-protein complexes could be more resistant to an FD-like mechanism; however, proteins at the boundaries of such complexes can still be replaced by solution proteins, enabling an efficient disassembly of such complexes when needed.
As a final note, despite our discussion was limited to prokaryotic cells, FD can play regulatory roles also in eukaryotic cells, while the role of 3d genome architecture on transcription has been already under consideration for a long time (Cremer and Cremer, 2001; Marshall, 2003; Lanctôt et al., 2007). Given that FD is more functional under relatively large concentration fluctuations, structural chromatin proteins such as histone components and heterochromatin protein 1 (HP1) seemingly can satisfy the conditions necessary for FD functionality (de Koning et al., 2009; Chari et al., 2019). Indeed, both proteins exhibit a turnover mechanism with their solution counterparts (Kilic et al., 2015; Gruszka et al., 2020). Yet, more experimental and computational work is needed to shed light on the role of TF concentration in the transcription kinetics in cell nuclei.
Author Contributions
ZK prepared the initial draft, AE provided the revised and corrected manuscript and wrote the conclusion. ZK finalized the manuscript.
Conflict of Interest
The authors declare that the research was conducted in the absence of any commercial or financial relationships that could be construed as a potential conflict of interest.
Publisher’s Note
All claims expressed in this article are solely those of the authors and do not necessarily represent those of their affiliated organizations, or those of the publisher, the editors and the reviewers. Any product that may be evaluated in this article, or claim that may be made by its manufacturer, is not guaranteed or endorsed by the publisher.
References
Agback, P., Baumann, H., Knapp, S., Ladenstein, R., and Härd, T. (1998). Architecture of Nonspecific Protein-DNA Interactions in the Sso7d-DNA Complex. Nat. Struct. Mol. Biol. 5 (7 5), 579–584. doi:10.1038/836
Ali Azam, T., Iwata, A., Nishimura, A., Ueda, S., and Ishihama, A. (1999). Growth Phase-dependent Variation in Protein Composition of the Escherichia coli Nucleoid. J. Bacteriol. 181, 6361–6370. doi:10.1128/jb.181.20.6361-6370.1999
Amemiya, H. M., Goss, T. J., Nye, T. M., Hurto, R. L., Simmons, L. A., and Freddolino, P. L. (2022). Distinct Heterochromatin‐like Domains Promote Transcriptional Memory and Silence Parasitic Genetic Elements in Bacteria. EMBO J. 41, e108708. doi:10.15252/EMBJ.2021108708
Azam, T. A., Hiraga, S., and Ishihama, A. (2000). Two Types of Localization of the DNA-Binding Proteins within the Escherichia coli Nucleoid. Genes. Cells 5, 613–626. doi:10.1046/J.1365-2443.2000.00350.X
Ball, C. A., Osuna, R., Ferguson, K. C., and Johnson, R. C. (1992). Dramatic Changes in Fis Levels upon Nutrient Upshift in Escherichia coli. J. Bacteriol. 174, 8043–8056. doi:10.1128/JB.174.24.8043-8056.1992
Bannister, A. J., and Kouzarides, T. (2011). Regulation of Chromatin by Histone Modifications. Cell. Res. 21 (3 21), 381–395. doi:10.1038/cr.2011.22
Berg, H. C. (1993). Random Walks in Biology: New and Expanded Edition, 152. Available at:https://www.abebooks.it/9780691000640/Random-Walks-Biology-New-Expanded-0691000646/plp (Accessed April 6, 2022).
Brackley, C. A., Taylor, S., Papantonis, A., Cook, P. R., and Marenduzzo, D. (2013). Nonspecific Bridging-Induced Attraction Drives Clustering of DNA-Binding Proteins and Genome Organization. Proc. Natl. Acad. Sci. U.S.A. 110, E3605–E3611. doi:10.1073/PNAS.1302950110
Bradley, M. D., Beach, M. B., de Koning, A. P. J., Pratt, T. S., and Osuna, R. (2007). Effects of Fis on Escherichia coli Gene Expression during Different Growth Stages. Microbiol. (N Y) 153, 2922–2940. doi:10.1099/MIC.0.2007/008565-0
Brangwynne, C. P., Tompa, P., and Pappu, R. v. (2015). Polymer Physics of Intracellular Phase Transitions. Nat. Phys. 11, 899–904. doi:10.1038/nphys3532
Callegari, A., Sieben, C., Benke, A., Suter, D. M., Fierz, B., Mazza, D., et al. (2019). Single-molecule Dynamics and Genome-wide Transcriptomics Reveal that NF-kB (P65)-DNA Binding Times Can Be Decoupled from Transcriptional Activation. PLoS Genet. 15, e1007891. doi:10.1371/journal.pgen.1007891
Clauß, K., Popp, A. P., Schulze, L., Hettich, J., Reisser, M., Escoter Torres, L., et al. (2017). DNA Residence Time Is a Regulatory Factor of Transcription Repression. Nucleic Acids Res. 45, 11121–11130. doi:10.1093/NAR/GKX728
Cremer, T., and Cremer, C. (2001). Chromosome Territories, Nuclear Architecture and Gene Regulation in Mammalian Cells. Nat. Rev. Genet. 2 (4 2), 292–301. doi:10.1038/35066075
de Jonge, W. J., Brok, M., Lijnzaad, P., Kemmeren, P., and Holstege, F. C. (2020). Genome‐wide Off‐rates Reveal How DNA Binding Dynamics Shape Transcription Factor Function. Mol. Syst. Biol. 16, e9885. doi:10.15252/MSB.20209885
de Koning, L., Savignoni, A., Boumendil, C., Rehman, H., Asselain, B., Sastre‐Garau, X., et al. (2009). Heterochromatin Protein 1α: a Hallmark of Cell Proliferation Relevant to Clinical Oncology. EMBO Mol. Med. 1, 178–191. doi:10.1002/EMMM.200900022
Dillon, S. C., and Dorman, C. J. (2010). Bacterial Nucleoid-Associated Proteins, Nucleoid Structure and Gene Expression. Nat. Rev. Microbiol. 8, 185–195. doi:10.1038/NRMICRO2261
Dorman, C. J. (2013). Co-operative Roles for DNA Supercoiling and Nucleoid-Associated Proteins in the Regulation of Bacterial Transcription. Biochem. Soc. Trans. 41, 542–547. doi:10.1042/BST20120222
Elgin, S. C. R., and Grewal, S. I. S. (2003). Heterochromatin: Silence Is Golden. Curr. Biol. 13, R895–R898. doi:10.1016/J.CUB.2003.11.006
Erbaş, A., and Marko, J. F. (2019). How Do DNA-Bound Proteins Leave Their Binding Sites? the Role of Facilitated Dissociation. Curr. Opin. Chem. Biol. 53, 118–124. doi:10.1016/J.CBPA.2019.08.007
Gibb, B., Ye, L. F., Gergoudis, S. C., Kwon, Y., Niu, H., Sung, P., et al. (2014). Concentration-Dependent Exchange of Replication Protein A on Single-Stranded DNA Revealed by Single-Molecule Imaging. PLOS ONE 9, e87922. doi:10.1371/JOURNAL.PONE.0087922
Graham, J. S., Johnson, R. C., and Marko, J. F. (2011). Concentration-dependent Exchange Accelerates Turnover of Proteins Bound to Double-Stranded DNA. Nucleic Acids Res. 39, 2249–2259. doi:10.1093/NAR/GKQ1140
Gruszka, D. T., Xie, S., Kimura, H., and Yardimci, H. (2020). Single-molecule Imaging Reveals Control of Parental Histone Recycling by Free Histones during DNA Replication. Sci. Adv. 6, eabc0330. doi:10.1126/SCIADV.ABC0330
Gurdon, J. B., Javed, K., Vodnala, M., and Garrett, N. (2020). Long-Term Association of a Transcription Factor with its Chromatin Binding Site Can Stabilize Gene Expression and Cell Fate Commitment. Proc. Natl. Acad. Sci. U.S.A. 117, 15075–15084. doi:10.1073/pnas.2000467117
Hadizadeh, N., Johnson, R. C., and Marko, J. F. (2016). Facilitated Dissociation of a Nucleoid Protein from the Bacterial Chromosome. J. Bacteriol. 198, 1735–1742. doi:10.1128/JB.00225-16
Halford, S. E., and Marko, J. F. (2004). How Do Site-specific DNA-Binding Proteins Find Their Targets? Nucleic Acids Res. 32, 3040–3052. doi:10.1093/NAR/GKH624
Hammel, M., Amlanjyoti, D., Reyes, F. E., Chen, J. H., Parpana, R., Tang, H. Y., et al. (2016). HU Multimerization Shift Controls Nucleoid Compaction. Sci. Adv. 2, e1600650. doi:10.1126/SCIADV.1600650
Hancock, S. P., Stella, S., Cascio, D., and Johnson, R. C. (2016). DNA Sequence Determinants Controlling Affinity, Stability and Shape of DNA Complexes Bound by the Nucleoid Protein Fis. PLOS ONE 11, e0150189. doi:10.1371/JOURNAL.PONE.0150189
Joshi, C. P., Panda, D., Martell, D. J., Andoy, N. M., Chen, T.-Y., Gaballa, A., et al. (2012). Direct Substitution and Assisted Dissociation Pathways for Turning off Transcription by a MerR-Family Metalloregulator. Proc. Natl. Acad. Sci. U.S.A. 109, 15121–15126. doi:10.1073/PNAS.1208508109
Kamar, R. I., Banigan, E. J., Erbas, A., Giuntoli, R. D., Olvera de la Cruz, M., Johnson, R. C., et al. (2017). Facilitated Dissociation of Transcription Factors from Single DNA Binding Sites. Proc. Natl. Acad. Sci. U.S.A. 114, E3251–E3257. doi:10.1073/PNAS.1701884114
Kilic, S., Bachmann, A. L., Bryan, L. C., and Fierz, B. (2015). Multivalency Governs HP1α Association Dynamics with the Silent Chromatin State. Nat. Commun. 6 (1 6), 1–11. doi:10.1038/ncomms8313
Koşar, Z., Attar, A. G., and Erbaş, A. (2022). Facilitated Dissociation of Nucleoid-Associated Proteins from DNA in the Bacterial Confinement. Biophysical J. 121, 1119–1133. doi:10.1016/J.BPJ.2022.03.002
Kotlajich, M. v., Hron, D. R., Boudreau, B. A., Sun, Z., Lyubchenko, Y. L., and Landick, R. (2015). Bridged Filaments of Histone-like Nucleoid Structuring Protein Pause RNA Polymerase and Aid Termination in Bacteria. Elife 4, e04970. doi:10.7554/ELIFE.04970
Lanctôt, C., Cheutin, T., Cremer, M., Cavalli, G., and Cremer, T. (2007). Dynamic Genome Architecture in the Nuclear Space: Regulation of Gene Expression in Three Dimensions. Nat. Rev. Genet. 8 (2 8), 104–115. doi:10.1038/nrg2041
Li, P., Banjade, S., Cheng, H.-C., Kim, S., Chen, B., Guo, L., et al. (2012). Phase Transitions in the Assembly of Multivalent Signalling Proteins. Nature 483 (7389 483), 336–340. doi:10.1038/nature10879
Lickwar, C. R., Mueller, F., Hanlon, S. E., McNally, J. G., and Lieb, J. D. (2012). Genome-wide Protein-DNA Binding Dynamics Suggest a Molecular Clutch for Transcription Factor Function. Nature 484 (7393 484), 251–255. doi:10.1038/nature10985
Lin, J., Chen, H., Dröge, P., and Yan, J. (2012). Physical Organization of DNA by Multiple Non-specific DNA-Binding Modes of Integration Host Factor (IHF). PLOS ONE 7, e49885. doi:10.1371/JOURNAL.PONE.0049885
Lioy, V. S., Cournac, A., Marbouty, M., Duigou, S., Mozziconacci, J., Espéli, O., et al. (2018). Multiscale Structuring of the E. coli Chromosome by Nucleoid-Associated and Condensin Proteins. Cell. 172, 771–783. e18. doi:10.1016/J.CELL.2017.12.027
Lucchini, S., Rowley, G., Goldberg, M. D., Hurd, D., Harrison, M., and Hinton, J. C. D. (2006). H-NS Mediates the Silencing of Laterally Acquired Genes in Bacteria. PLoS Pathog. 2, e81. doi:10.1371/JOURNAL.PPAT.0020081
Macvanin, M., Edgar, R., Cui, F., Trostel, A., Zhurkin, V., and Adhya, S. (2012). Noncoding Rnas Binding to the Nucleoid Protein HU in Escherichia coli. J. Bacteriol. 194, 6046–6055. doi:10.1128/JB.00961-12
Marshall, W. F. (2003). Gene Expression and Nuclear Architecture during Development and Differentiation. Mech. Dev. 120, 1217–1230. doi:10.1016/J.MOD.2003.05.001
Popp, A. P., Hettich, J., and Gebhardt, J. C. M. (2021). Altering Transcription Factor Binding Reveals Comprehensive Transcriptional Kinetics of a Basic Gene. Nucleic Acids Res. 49, 6249–6266. doi:10.1093/NAR/GKAB443
Remesh, S. G., Verma, S. C., Chen, J.-H., Ekman, A. A., Larabell, C. A., Adhya, S., et al. (2020). Nucleoid Remodeling during Environmental Adaptation Is Regulated by HU-dependent DNA Bundling. Nat. Commun. 11 (1 11), 1–12. doi:10.1038/s41467-020-16724-5
Schneider, R., Travers, A., and Muskhelishvili, G. (2001). DNA Supercoiling and Transcription in Escherichia coli: The FIS Connection. Biochimie 83, 213–217. doi:10.1016/S0300-9084(00)01217-7
Shao, Y., Feldman-Cohen, L. S., and Osuna, R. (2008). Functional Characterization of the Escherichia coli Fis-DNA Binding Sequence. J. Mol. Biol. 376, 771–785. doi:10.1016/J.JMB.2007.11.101
Sing, C. E., Olvera de la Cruz, M., and Marko, J. F. (2014). Multiple-binding-site Mechanism Explains Concentration-dependent Unbinding Rates of DNA-Binding Proteins. Nucleic Acids Res. 42, 3783–3791. doi:10.1093/NAR/GKT1327
Singh, S. S., Singh, N., Bonocora, R. P., Fitzgerald, D. M., Wade, J. T., and Grainger, D. C. (2014). Widespread Suppression of Intragenic Transcription Initiation by H-NS. Genes. Dev. 28, 214–219. doi:10.1101/GAD.234336.113
Skoko, D., Yoo, D., Bai, H., Schnurr, B., Yan, J., McLeod, S. M., et al. (2006). Mechanism of Chromosome Compaction and Looping by the Escherichia coli Nucleoid Protein Fis. J. Mol. Biol. 364, 777–798. doi:10.1016/J.JMB.2006.09.043
Stojkova, P., Spidlova, P., and Stulik, J. (2019). Nucleoid-associated Protein Hu: A Lilliputian in Gene Regulation of Bacterial Virulence. Front. Cell. Infect. Microbiol. 9, 159. doi:10.3389/FCIMB.2019.00159
Stracy, M., Schweizer, J., Sherratt, D. J., Kapanidis, A. N., Uphoff, S., and Lesterlin, C. (2021). Transient Non-specific DNA Binding Dominates the Target Search of Bacterial DNA-Binding Proteins. Mol. Cell. 81, 1499–1514. doi:10.1016/J.MOLCEL.2021.01.039
Strom, A. R., Emelyanov, A. v., Mir, M., Fyodorov, D. v., Darzacq, X., and Karpen, G. H. (2017). Phase Separation Drives Heterochromatin Domain Formation. Nature 547 (7662 547), 241–245. doi:10.1038/nature22989
Trojanowski, J., Frank, L., Rademacher, A., Mücke, N., Grigaitis, P., and Rippe, K. (2022). Transcription Activation Is Enhanced by Multivalent Interactions Independent of Phase Separation. Mol. Cell. 82, 1878–1893. doi:10.1016/J.MOLCEL.2022.04.017
Tsai, M.-Y., Zhang, B., Zheng, W., and Wolynes, P. G. (2016). Molecular Mechanism of Facilitated Dissociation of Fis Protein from DNA. J. Am. Chem. Soc. 138, 13497–13500. doi:10.1021/JACS.6B08416
van Noort, J., Verbrugge, S., Goosen, N., Dekker, C., and Dame, R. T. (2004). Dual Architectural Roles of HU: Formation of Flexible Hinges and Rigid Filaments. Proc. Natl. Acad. Sci. U.S.A. 101, 6969–6974. doi:10.1073/PNAS.0308230101
Verma, S. C., Qian, Z., and Adhya, S. L. (2019). Architecture of the Escherichia coli Nucleoid. PLoS Genet. 15, e1008456. doi:10.1371/JOURNAL.PGEN.1008456
Wang, W., Li, G.-W., Chen, C., Xie, X. S., and Zhuang, X. (2011). Chromosome Organization by a Nucleoid-Associated Protein in Live Bacteria. Sci. (1979) 333, 1445–1449. doi:10.1126/SCIENCE.1204697
Wilky, H., Chari, S., Govindan, J., and Amodeo, A. A. (2019). Histone Concentration Regulates the Cell Cycle and Transcription in Early Development. Development 146, dev177402. doi:10.1242/DEV.177402
Winardhi, R. S., Yan, J., and Kenney, L. J. (2015). H-NS Regulates Gene Expression and Compacts the Nucleoid: Insights from Single-Molecule Experiments. Biophysical J. 109, 1321–1329. doi:10.1016/J.BPJ.2015.08.016
Keywords: gene expression, facilitated dissociation, transcriptional regulation, DNA-binding proteins, transcription factors, nucleoid-associated proteins, chromosome organization
Citation: Koşar Z and Erbaş A (2022) Can the Concentration of a Transcription Factor Affect Gene Expression?. Front. Soft. Matter 2:914494. doi: 10.3389/frsfm.2022.914494
Received: 06 April 2022; Accepted: 10 June 2022;
Published: 01 July 2022.
Edited by:
Jay X. Tang, Brown University, United StatesReviewed by:
Vladimir N. Uversky, University of South Florida, United StatesCopyright © 2022 Koşar and Erbaş. This is an open-access article distributed under the terms of the Creative Commons Attribution License (CC BY). The use, distribution or reproduction in other forums is permitted, provided the original author(s) and the copyright owner(s) are credited and that the original publication in this journal is cited, in accordance with accepted academic practice. No use, distribution or reproduction is permitted which does not comply with these terms.
*Correspondence: Zafer Koşar, emFmZXIua29zYXJAYmlsa2VudC5lZHUudHI=; Aykut Erbaş, YXlrdXQuZXJiYXNAdW5hbS5iaWxrZW50LmVkdS50cg==