- Istituto Nazionale di Fisica Nucleare – Laboratori Nazionali di Legnaro, Viale dell’Università 2, Legnaro, Italy
Different types of radiation cause varying levels of biological damage, even when the physical dose is the same, due to the unique way energy is distributed at the subcellular level. The MUSICA project is focused on creating an innovative detector that not only provides quantitative dosimetric data but also qualitative insights. These qualitative insights are derived from measuring physical parameters that are linked to biological effectiveness. This approach, particularly in proton therapy, aims to enhance treatment precision and improve clinical outcomes. Radiation damage occurs through processes at multiple scales, from the DNA level (2 nm) to the entire cell nucleus (10 μm). The stochastic spatial distribution of energy deposition can be studied using microdosimetric techniques with tissue-equivalent gas proportional counters (TEPC). While many studies employing TEPCs have examined sites smaller than 2 μm (such as chromosomes), characterization at the 10 μm scale has typically been performed useing solid-state detectors. However, gas microdosimeters provide benefits in terms of sensitivity, geometry, and tissue equivalence, making it highly advantageous to conduct multi-site characterization using a single detector. The project has developed a TEPC with two charge collection zones, allowing for the definition of two different volume sizes (e.g., 1 and 10 μm) without changing the gas pressure. This will enable dual-dimension microdosimetric characterization in a single measurement session without switching detectors. The resulting bi-dimensional data will be integrated into new radiobiological models that link physical measurements to biological outcomes, enhancing our understanding of how ionizing radiation affects living tissue.
1 Introduction and state of the art
The correlation between biological damage induced by ionizing radiation and the physical characteristics of the radiation field is crucial for improving the outcomes of hadron therapy treatments. Although the reference quantity for treatment planning systems (TPS) is the absorbed dose, it is known that different ionizing radiation types cause varying degrees of biological damage at the same dose level (Blakely et al., 1984; Durante and Paganetti, 2016). This variation in effectiveness is due to the fact that biological effects depend not only on the average absorbed energy (dose) but also on its microscopic spatial distribution, which can be highly heterogeneous (Goodhead, 1994). The physical dose is weighted by the relative biological effectiveness (RBE), which is the ratio of the dose of the radiation field under study to that of a reference field (photons) that produces the same biological effect. Modern Treatment Planning Systems (TPS) for hadron therapy aim to optimize radiation dose delivery while accounting for the variable Relative Biological Effectiveness (RBE) within the patient. Unlike conventional radiotherapy, hadron therapy exhibits spatial variations in RBE due to complex interactions of charged particles with biological tissues. Radiobiological measurements of RBE, while fundamental for precise treatment modeling, are often time-consuming, resource-intensive, and experimentally challenging. They require extensive in vitro and in vivo studies under controlled conditions, making their routine use in clinical settings impractical. A more feasible alternative is to leverage measurable physical characteristics of the radiation field to predict RBE using validated biophysical models.
Microdosimetry offers valuable tools for assessing the biological impact of ionizing radiation by capturing the stochastic nature of energy deposition within a defined microscopic volume. Unlike macroscopic dosimetric parameters, which provide average values over a bulk tissue volume, microdosimetry enables the characterization of the highly heterogeneous energy deposition patterns that drive biological effects (Booz et al., 1983; Braby et al., 2023). This is particularly relevant in hadron therapy, where radiation quality varies significantly along the particle track, leading to complex spatial variations in RBE. Microdosimetric quantities, such as the specific energy (z) and lineal energy (y), depend on the size of the sensitive volume, making them highly effective for studying correlations between radiation exposure and biological damage across different spatial scales. These scales range from the nanometer level (where DNA damage occurs) to tens of microns (encompassing entire cell nuclei or small tissue structures). By analyzing microdosimetric spectra, researchers can gain insights into the probability distributions of energy deposition events, which are more predictive of biological effects than mean dose alone. The integration of microdosimetric data into radiobiological models enhances their predictive power by providing physical input parameters that account for stochastic fluctuations in energy deposition. Microdosimetric-based RBE models can more accurately reflect the spatial variations in biological response. Furthermore, advancements in silicon-based microdosimeters and tissue-equivalent proportional counters (TEPCs) facilitate real-time in vivo and in vitro measurements, improving the feasibility of microdosimetry-informed treatment planning.
However, most experimental studies have focused on diameters of 1–2 μm, the size of a mammalian chromosome. Studies at smaller dimensions in the range of 50–300 nm have been conducted with specialized avalanche confinement TEPCs (Bortot et al., 2018; Mazzucconi et al., 2019). Beyond these small-scale investigations, ionizing radiation effects extend beyond individual chromosomes to the entire cell nucleus and even multicellular structures. To capture radiation interactions at larger biological sites, microdosimetric studies have been performed using sensitive volumes in the range of 15–20 μm, which approximate the dimensions of a whole cell nucleus. These studies have been conducted with solid-state detectors (SSDs) as reported in (Agosteo et al., 2010; Verona et al., 2020; Rosenfeld, 2016).
Studying radiation interactions across multiple scales—from nanometers to microns—enables a more comprehensive understanding of the relationship between physical energy deposition and biological outcomes. This multi-scale approach is essential for refining radiobiological models and improving treatment planning in hadron therapy. By integrating microdosimetric data from various spatial scales, TPS can better predict biological effectiveness and optimize dose delivery strategies to maximize tumor control while minimizing damage to healthy tissues.
The ability to modify the simulated site size to perform a multi-site microdosimetric characterization is a significant advantage of gas-based detectors over solid-state detectors (SSDs). In tissue-equivalent proportional counters (TEPCs), the simulated volume size is adjusted by varying the gas density and pressure, allowing flexibility in experimental setups. For instance, in a 1 mm diameter spherical cavity, propane gas must be maintained at approximately 41 kPa to simulate a 1 μm tissue site. To simulate a larger site, such as 10 μm, either an overpressure approach must be applied or a detector with a larger cavity must be used. However, this adaptability comes with operational challenges. When altering the gas pressure in TEPCs, a rigorous cleaning and conditioning process is necessary to maintain gain stability and response reproducibility. This process can take at least a week, as the detector must reach equilibrium to ensure reliable measurements. An alternative approach is to continuously flush the detector with fresh gas, but this method is impractical in clinical environments due to logistical constraints. To overcome these limitations, two separate detectors with different gas pressures or cavity sizes can be employed, though this introduces additional complexity in detector calibration, poistioning and data interpretation. Despite these challenges, the capability to adjust the simulated site size remains a crucial feature of gas-based microdosimetry, enabling more detailed investigations into radiation interactions at different spatial scales. To overcome these issues, an innovative tissue-equivalent proportional counter (TEPC) has been developed, capable of measuring at two different dimensional scales almost simultaneously. This design eliminates the need to change the experimental setup or rely on gas flow adjustments, thus retaining all the advantages associated with using a single detector—such as consistent positioning, uniform materials, stable gas conditions, and reliable response reproducibility. This novel TEPC system ensures bi-dimensional characterization of clinical beams within a single measurement session, significantly improving efficiency and accuracy in microdosimetric assessments. The ability to measure at two dimensions concurrently enhances the resolution of the data collected, allowing for a more comprehensive understanding of radiation interactions at varying scales. Additionally, the information obtained from such measurements will be integrated into emerging multi-dimensional radiobiological models, contributing to more precise and personalized radiation therapy planning. This advancement has the potential to improve the effectiveness of proton and heavy-ion therapies by providing a more detailed characterization of their microdosimetric behavior.
The development of the new multi-site TEPC has been carried out at the Legnaro National Laboratories of the Italian National Institute for Nuclear Physics (INFN-LNL) where the microdosimetry group have decades of expertise in experimental microdosimetry and the development of advanced TEPCs for hadron therapy (De Nardo et al., 2004; Conte et al., 2019; Bianchi et al., 2021a; Selva et al., 2020; Bianchi et al., 2024).
2 Methodology
The concept behind developing a multi-site TEPC is to use a single TEPC at a fixed pressure to simulate two different sensitive volumes of interest for multi-site radiobiological models. This is achieved by inserting a third helical electrode coaxial to the anode within the cylindrical TEPC volume. The electric field between the cathode and the helix controls the drift of electrons in this region, determining whether the external volume functions as a sensitive region. This concept is schematically illustrated in Figure 1: when the field directs electrons toward the helix, the external region collects electrons and becomes active (left side of Figure 1); when it directs them toward the cathode, the external region remains inactive (right side of Figure 1). The radius of the inner region confined by the helix is a factor of ten smaller than the cathode radius. The concept behind the multi-site TEPC has an Italian industrial patent pending.
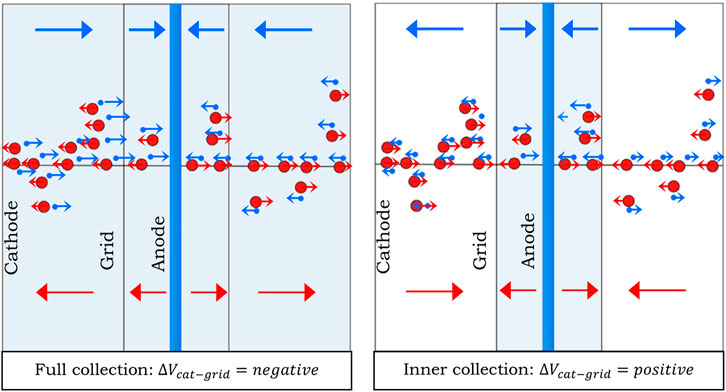
Figure 1. Graphical representation of the measurement conditions: on the left all charges are collected to the anode, on the right only those produced inside the helix. Red dots represent ions while blue dots represent electrons. The arrows indicate the moving direction of ions and electrons in the same colour coding.
Based on this concept, the detector developed in the MUSICA project is a cylindrical TEPC with three electrodes: a150 cathode wall of 2 cm of diameter and height and 2.5 mm thick, a helical electrode with a diameter of 2 mm made of a 100 μm thick gold-plated tungsten wire, and a central anode wire made of a 25 μm thick gold-plated tungsten wire. The A150 cylinder is enclosed within a 2.5 mm thick Rexolite shell and a 0.25 mm thick outer aluminium cap. Additionally, two A150 disks are positioned on the top and bottom of the sensitive volume, as illustrated in Figure 2. Two guard tubes are placed at the extremities of the anode wire to better define the electric field and, consequently, the sensitive volume from which free electrons are collected at the anode. Additionally, by keeping these electrodes at the same potential as the anode, they prevent the collection of electrons from parasitic gaseous regions outside the sensitive volume. This electrode configuration was first introduced for the first time in the mini-TEPCs developed at INFN-LNL, and more details can be found in (Bianchi et al., 2024). The optimization of specific aspects of the detector design, such as the size and position of the guard tubes, has been performed through simulations carried out with COMSOL Multiphysics software. The water equivalent thickness of the multi-site TEPC has been evaluated as equal to 6.5 mm, considering both the density and the stopping power of the different materials. A schematic representation of the detector is shown in Figure 2. Following this design, a first prototype of the detector has been constructed and will be tested in the future.
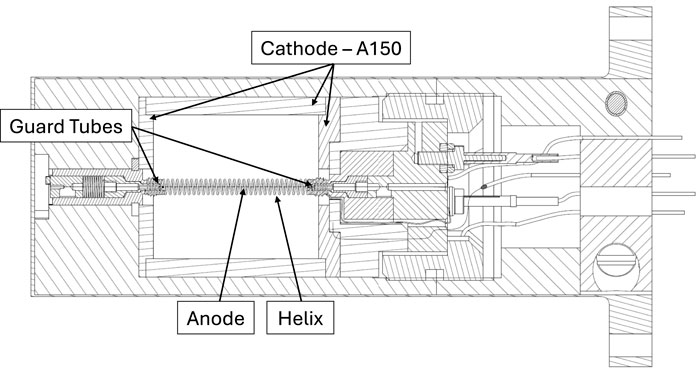
Figure 2. Section of a technical drawing of the multi-site TEPC. The components surrounding the sensitive volume are mainly cosntructed in Rexolite.
The detector operates in a sealed mode, complying with most safety regulations that prohibit the use of gas-flow systems with propane bottles in clinical facilities. Before each measurement campaign, the detector is kept 1 week in vacuum to reach a level of vacuum of at least 10–5 hPa. Once this level is achieved, the detector undergoes a series of filling and evacuation cycles with propane at a pressure higher than the operational level. Each phase lasts approximately 1 hour and is repeated at least four times. The final filling is performed at operational pressure, after which the detector is left to stabilize for at least 2 days before radiation measurements in the field. During this stabilization period, the pressure is monitored to verify the success of the preparation process.
3 Discussion and future perspectives
The device developed within the MUSICA project provides a comprehensive spectrum of information regarding the physical quantities that characterize the radiation field. By combining microdosimetric spectra from multiple sites with the trajectories of primary particles within the Tissue-Equivalent Proportional Counter (TEPC), the system offers a rich dataset that can be integrated into innovative multi-site radiobiological models. These models establish correlations with the extent of biological damage considering the stochastic of energy depositions at different cellular scales, enabling precise mapping of the biological effectiveness of the investigated radiation field. An example of such models is the GSM2 recently developed, more information can be found in (Bordieri et al., 2025; Battestini et al., 2023). Such a model holds great potential for integration into clinical treatment planning systems. By incorporating point-by-point radiation field effectiveness, treatment plans could evolve from traditional methods to more biologically optimized approaches. This shift would result in improved clinical outcomes by maximizing tumor control while minimizing the risk of secondary effects resulting from irradiation of surrounding healthy tissues.
Furthermore, as emphasized throughout the previous section, the methodology developed within MUSICA is highly innovative. Prior to this experiment, no study had utilized a TEPC with charge collection on two distinct coaxial cylindrical volumes. This novel approach opens up new avenues for the precise measurement and characterization of radiation interactions at a detailed level, offering an innovative experimental technique for model validation.
The detector developed in the MUSICA project contributes to a deeper understanding of the relationship between physical radiation fields and biological effects, offering a step forward in personalizing and optimizing cancer treatments. By utilizing the multi-site information in new radiobiological models, future clinical practices could enhance the ability to tailor radiation therapy, aiming to improve therapeutic outcomes while reducing the potential for unintended damage to surrounding healthy tissue.
The next phase of the project will begin with an extensive series of tests designed to evaluate the performance of the detector in reference fields, specifically with neutron and gamma rays. These fields are chosen because they provide a controlled environment where the operational parameters of the detector, such as its sensitivity and response to different types of radiation, can be assessed under well-understood conditions. In particular, the multi-site technique employed by the detector will be scrutinized. This technique relies on switching the electric field in the detector to select the charge collection volume, and testing in these reference fields will provide valuable data to confirm the effectiveness of this method. This is crucial because validating the technique in a controlled setting will ensure that the detector functions as intended when exposed to more complex radiation fields, like those encountered in hadron therapy. These tests will be conducted to establish the correct voltages for both charge collection and to assess the real simulated site size in the internal collection. The confinement of the charge will be solely performed by the helix structure, which requires detailed examination to ensure that the internal collection is accurately simulated. This phase is critical for fine-tuning of the sensitivity and precision at small scales of the detector, ensuring it can handle the variations in radiation field interactions at the microscopic level.
Once the detector has been thoroughly characterized and its performance has been validated in reference fields, it will be deployed at the proton therapy center for further clinical testing. Here, measurements of both internal and external collection volumes will be compared with reference detectors, such as the mini-TEPCs developed at LNL-INFN at the same site sizes. This comparison will ensure that the data gathered by the new detector is reliable and consistent with established measurement techniques, validating its use in clinical environments.
Finally, the proton beam itself will be systematically characterized across various site sizes, enabling a comprehensive analysis of the physical characteristics of the radiation field. The data obtained from these measurements will be analyzed in terms of key quantities useful for determining the Relative Biological Effectiveness (RBE), which is vital for refining treatment planning in hadron therapy and, especially, in proton therapy. Additionally, this data will play a crucial role in enhancing quality assurance (QA) procedures, helping to ensure that treatment is both effective and safe by minimizing damage to surrounding healthy tissues while maximizing tumor control.
Data availability statement
The raw data supporting the conclusions of this article will be made available by the authors, without undue reservation.
Author contributions
AB: Conceptualization, Data curation, Formal Analysis, Funding acquisition, Investigation, Validation, Visualization, Writing – original draft, Writing – review and editing. AS: Investigation, Validation, Writing – review and editing. MR: Resources, Writing – review and editing. VC: Investigation, Methodology, Supervision, Validation, Writing – review and editing.
Funding
The author(s) declare that financial support was received for the research and/or publication of this article. This work has been funded by the fifth scientific commission of INFN.
Conflict of interest
The authors declare that the research was conducted in the absence of any commercial or financial relationships that could be construed as a potential conflict of interest.
Generative AI statement
The author(s) declare that no Generative AI was used in the creation of this manuscript.
Publisher’s note
All claims expressed in this article are solely those of the authors and do not necessarily represent those of their affiliated organizations, or those of the publisher, the editors and the reviewers. Any product that may be evaluated in this article, or claim that may be made by its manufacturer, is not guaranteed or endorsed by the publisher.
References
Agosteo, S., Cirrone, G. A. P., Colautti, P., Cuttone, G., D’Angelo, G., Fazzi, A., et al. (2010). Study of a silicon telescope for solid state microdosimetry: preliminary measurements at the therapeutic proton beam line of CATANA. Radiat. Meas. 45 (10), 1284–1289. doi:10.1016/j.radmeas.2010.06.051
Battestini, M., Missiaggia, M., Attili, A., Tommasino, F., La Tessa, C., Cordoni, F. G., et al. (2023). Across the stages: a multiscale extension of the generalized stochastic microdosimetric model (MS-GSM2) to include the ultra-high dose rate. Front. Phys. 11. doi:10.3389/fphy.2023.1274064
Bianchi, A., Selva, A., Colautti, P., Petringa, G., Cirrone, P., Reniers, B., et al. (2021a). Repeatability and reproducibility of microdosimetry with a mini-TEPC. Front. Phys. 9. doi:10.3389/fphy.2021.727816
Bianchi, A., Selva, A., Pasquato, F., Rossignoli, M., Minarello, A., Fazzi, A., et al. (2024). Microdosimetric measurements for LET monitoring in proton therapy. The development of engineered mini-TEPCs for clinical applications: first results. Radiat. Meas. 177, 107271. doi:10.1016/j.radmeas.2024.107271
Blakely, E. A., Ngo, F. Q. H., Curtis, S. B., and Tobias, C. A. (1984). Heavy-ion radiobiology: cellular studies. Adv. Radiat. Biol. 11, 295–389. doi:10.1016/B978-0-12-035411-5.50013-7
Booz, J., Braby, L., Coyne, J., Kliauga, P., Lindborg, L., Menzel, H.-G., et al. (1983). ICRU report 36. J. Int. Comm. Radiat. Units Meas. 19 (1).
Bordieri, G., Missiaggia, M., Cartechini, G., Battestini, M., Bronk, L., Guan, F., et al. (2025). Validation of the generalized stochastic microdosimetric model (GSM) over a broad range of LET and particle beam type: a unique model for accurate description of (therapy relevant) radiation qualities. Phys. Med. and Biol. 70 (1), 015005. doi:10.1088/1361-6560/ad9dab
Bortot, D., Mazzucconi, D., Bonfanti, M., Agosteo, S., Pola, A., Pasquato, S., et al. (2018). A novel TEPC for microdosimetry at nanometric level: response against different neutron fields. Radiat. Prot. Dosim. 180 (1-4), 172–176. doi:10.1093/rpd/ncx198
Braby, L. A., Conte, V., Dingfelder, M., Goodhead, D. T., Pinsky, L. S., Rosenfeld, A. B., et al. (2023). ICRU Report 98, Stochastic nature of radiation interactions: microdosimetry. J. Int. Comm. Radiat. Units Meas. 23 (1), 1–168. doi:10.1177/14736691231211380
Conte, V., Bianchi, A., Selva, A., Petringa, G., Cirrone, G. A. P., Parisi, A., et al. (2019). Microdosimetry at the CATANA 62 MeV proton beam with a sealed miniaturized TEPC. Phys. Medica 64, 114–122. doi:10.1016/j.ejmp.2019.06.011
De Nardo, L., Cesari, V., Donà, G., Magrin, G., Colautti, P., Conte, V., et al. (2004). Mini-TEPCs for radiation therapy. Radiat. Prot. Dosim. 108 (4), 345–352. doi:10.1093/rpd/nch023
Durante, M., and Paganetti, H. (2016). Nuclear physics in particle therapy: a review. Rep. Prog. Phys. 79 (9), 096702. doi:10.1088/0034-4885/79/9/096702
Goodhead, D. T. (1994). Initial events in the cellular effects of ionizing radiations: clustered damage in DNA. Int. J. Radiat. Biol. 65 (1), 7–17. doi:10.1080/09553009414550021
Mazzucconi, D., Bortot, D., Pola, A., Fazzi, A., Colautti, P., Conte, V., et al. (2019). Nano-microdosimetric investigation at the therapeutic proton irradiation line of CATANA. Radiat. Meas. 123, 26–33. doi:10.1016/j.radmeas.2019.02.012
Rosenfeld, A. B. (2016). Novel detectors for silicon-based microdosimetry, their concepts and applications. Nucl. Instrum. Methods Phys. Res. Sect. A Accel. Spectrom. Detect. Assoc. Equip. 809, 156–170. doi:10.1016/j.nima.2015.08.059
Selva, A., Bianchi, A., Colautti, P., and Conte, V. (2020). Towards a compact experimental setup for gas-based microdosimetry. J. Phys. Conf. Ser. 1662, 012030. doi:10.1088/1742-6596/1662/1/012030
Verona, C., Cirrone, G. A. P., Magrin, G., Marinelli, M., Palomba, S., Petringa, G., et al. (2020). Microdosimetric measurements of a monoenergetic and modulated Bragg Peaks of 62 MeV therapeutic proton beam with a synthetic single crystal diamond microdosimeter. Med. Phys. 47 (11), 5791–5801. doi:10.1002/mp.14466
Keywords: microdosimetry, TEPC, multi-site microdosimetry, radiation quality, proton therapy, hadron therapy
Citation: Bianchi A, Selva A, Rossignoli M and Conte V (2025) A multi-site microdosimeter for clinical beam characterization. Front. Sens. 6:1587646. doi: 10.3389/fsens.2025.1587646
Received: 04 March 2025; Accepted: 24 March 2025;
Published: 09 April 2025.
Edited by:
Vincenzo Guidi, University of Ferrara, ItalyReviewed by:
Stefano Agosteo, Polytechnic University of Milan, ItalyCopyright © 2025 Bianchi, Selva, Rossignoli and Conte. This is an open-access article distributed under the terms of the Creative Commons Attribution License (CC BY). The use, distribution or reproduction in other forums is permitted, provided the original author(s) and the copyright owner(s) are credited and that the original publication in this journal is cited, in accordance with accepted academic practice. No use, distribution or reproduction is permitted which does not comply with these terms.
*Correspondence: Anna Bianchi, YW5uYS5iaWFuY2hpQGxubC5pbmZuLml0