- 1Sao Carlos Institute of Physics, University of São Paulo, São Carlos, Brazil
- 2Embrapa Instrumentação, Nanotechnology National Laboratory for Agriculture (LNNA), São Carlos, Brazil
An electronic tongue was developed for the detection of lactose content in commercial foods. This was accomplished by employing optimized detection units comprised of gelatin films and information visualization methods for data analysis. The films incorporating gelatin, tannic acid, and zein, served as the basis for the sensors, whose electrodes were screen printed using carbon black ink. Self-supporting films were produced using various combinations of these materials, some of which had limited solubility in water (from 33% to 36%). They were hydrophobic and yielded reproducible electrical impedance spectra to be used as sensing units. Lactose detection experiments were conducted using various standard concentrations and commercial food samples. Capacitance decreased with lactose concentration at low frequencies, with films lacking a hydrophobic coating showing higher capacitance signals (exceeding 200 nF). Low limits of detection were obtained for the most sensitive films, as low as 2.03 × 10−19 mol/L, comparable to existing biosensors to detect lactose. Combining data from four sensing units in an electronic tongue allowed for the differentiation of lactose concentrations ranging from 1 × 10−20 mol/L to 1 × 10−6 mol/L using the interactive document mapping (IDMAP) projection technique, leading to a silhouette coefficient of 0.716. The discriminatory power of the electronic tongue was validated by distinguishing between lactose-containing and lactose-free food products. These findings highlight the potential of electronic tongues made with sustainable materials for applications in food quality assessment and lactose intolerance management.
1 Introduction
Lactose detection is important for both food quality control and the health of lactose-intolerant individuals. Ideally, this detection should occur at the point of use or within the food industry on a routine basis, which requires portable and cost-effective technology. The main methodology fulfilling these requirements is based on biosensors (Bondancia et al., 2022) that can be used with portable instruments (Buscaglia et al., 2021). While biosensors offer high sensitivity and selectivity, they suffer from the drawback of requiring enzyme immobilization, which compromises device robustness and shelf life. An alternative to biosensors is electronic tongues, also used in food quality control (Coatrini Soares et al., 2022; Soares et al., 2023). Electronic tongues operate on the principle of global selectivity; however, they tend to exhibit lower performance than biosensors owing to the absence of specific analyte interactions. This limitation can be mitigated through careful selection of sensing unit materials and the application of statistical and computational methods to process electrical response data (Neto et al., 2021; Coatrini Soares et al., 2022; Soares et al., 2023).
In this paper, we present an electronic tongue capable of highly sensitive lactose detection, including in food samples. Our goal was to develop sensing units made of sustainable materials that are both cost-effective and biodegradable, meeting essential requirements for routine monitoring devices. To achieve this, we utilized substrates derived from natural polymers suitable for biomedical devices and food packaging due to their biodegradability and biocompatibility (Song et al., 2020; Soares et al., 2023). The materials employed included gelatin, zein, and tannic acid, with which wearable sensors can be produced (Leite et al., 2020). Gelatin, derived from the hydrolyzation of animal collagen, forms continuous, homogeneous, and self-supporting films (Rattaya et al., 2009). Tannic acid, a polyphenol extracted from oak trees, acts as a protein crosslinker, enhancing film mechanical properties (Jia et al., 2020). Zein, the principal protein found in corn, possesses a hydrophobic character and can be used as an external layer for films, thereby increasing moisture resistance and affinity to carbon black (Soares et al., 2023). The sensing units of the electronic tongue featured printed electrodes made from carbon ink on substrates fabricated with various combinations of the materials mentioned. By employing optimized materials and impedance spectroscopy as the principle of detection, the electronic tongue achieved high sensitivity in lactose detection, particularly when data were processed using information visualization methods.
2 Materials and methods
Self-supporting films were produced with gelatin (Sigma Aldrich), tannic acid (1,701.20 g.mol−1) and glycerol (92.09 g.mol−1) (Sigma Aldrich), diluted in ultrapure water. We employed the bench casting technique which consists in placing solutions in containers for evaporating the solvent at room temperature and in an air circulation oven (Zhang et al., 2010; Keller et al., 2011; Otoni et al., 2017). The gelatin film-forming solution was prepared as follows: gelatin powder at a concentration of 5% (by total volume) and glycerol 20% (by weight of dry gelatin) were hydrated with ultrapure water for 25 min at 80°C under mechanical stirring. Then, tannic acid (4% by weight of dry gelatin) was added, followed by mechanical stirring for 20 min, and elimination of bubbles by allowing the solution to rest. The solutions were then placed on a polyester substrate for solvent evaporation, forming a self-sustaining polymeric film. Some of the films were coated with a layer of zein and tannic acid deposited using the Solution Blow Spinning (SBS) technique in the aspersion mode of 20% zein in glacial acetic during 40 min, feed speed 0.45 mL.min−1, and a nozzle-collector distance of 35 cm. For the films containing a layer of zein and tannic acid, the concentration was 15% (by weight of dry zein). The purpose of this coating was to improve the moisture resistance of the films. The films produced were named as follows: Film 1, containing 5% gelatin + 4% tannic acid, prepared at room temperature; Film 2, 5% gelatin + 4% tannic acid, dried at 80°C; Film 3, 5% gelatin + 4% tannic acid + zein and tannic acid coating, dried at 80°C; and Film 4, 5% gelatin + 4% tannic acid + zein coating, dried at 80°C. The solubility of the self-supporting films in water was measured in triplicate, using samples with 0.05 g ± 0.01 g. The samples were placed in falcon tubes with 50 mL of ultrapure water and left to rest for 3 h. The falcon tubes were shaken every half an hour for 3 h. The non-solubilized material was separated using a sieve, and dried in an air circulation oven at 80°C for 30 min. These dry samples were weighed to estimate the soluble mass percentage, with the soluble mass calculated with Eq. 1 (Liu et al., 2020), where Mi is the initial mass and Mf is the final mass of the sample, weighed after drying in the oven.
The film morphology and the coating materials were assessed using a scanning electron microscope (SEM, JEOL 6510) operating at an acceleration voltage of 5 kV after sputter-coating the samples with gold. The hydrophilic/hydrophobic character of the self-supporting films was estimated with contact angle measurements, using a CAM 200 goniometer (KSV Instruments, Finland), with ultrapure water as a probe liquid. Ultrapure water was dropped on the self-supporting films, followed by image capture every second, with a total time of 60 s. The interdigitated electrodes shown in Figure 1 were printed on the self-supporting films using the silk-screen technique. The carbon ink made of carbon black Loctite EDAG 423 A (Henkel, United States) was forced through by a screen that contains the electrode shape. Before printing, the ink was diluted in 25 µL ethyl acetate to adjust its viscosity and to pass through the screen. Ink curing was carried out in an air circulation oven at 80°C for 30 min.
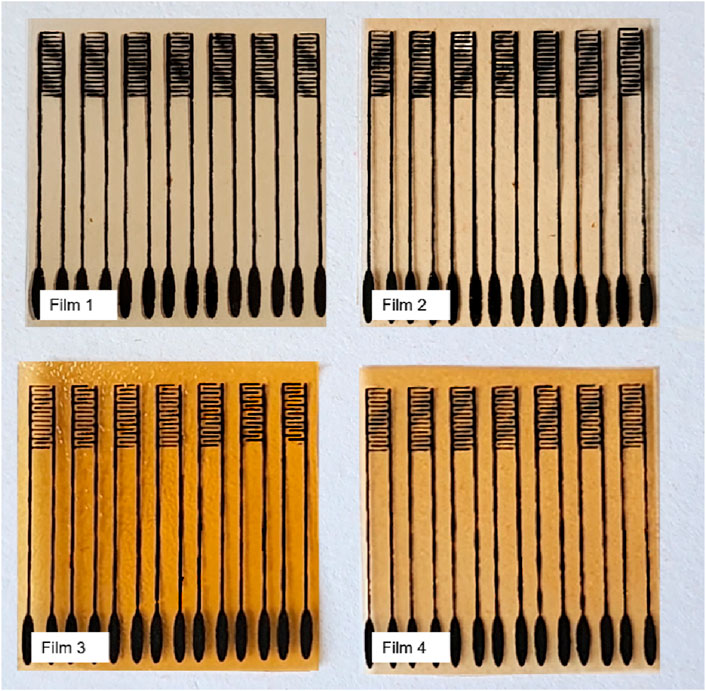
Figure 1. Interdigitated electrodes printed on: Film 1: 5% gelatin + 4% tannic acid; Film 2: 5% gelatin + 4% tannic acid + 80°C; Film 3: 5% gelatin + 4% tannic acid + zein and tannic acid coating + 80°C; Film 4: 5% gelatin + 4% tannic acid + zein coating + 80°.
2.1 Lactose detection
Lactose was chosen as analyte in samples diluted in a saline buffer using an electronic tongue comprising an array of electrodes printed on gelatin and tannic acid films, with and without hydrophobic coating. Impedance spectroscopy measurements were performed with a Solartron model 1260 A, in the frequency range between 1 Hz and 1 MHz, with AC potential 50 mV and DC potential 0 mV (see Supplementary Figure S1). Since the materials employed in the sensing units were selected to be as biodegradable as possible, robustness may be a problem with the ensuing difficulty of reproducibility of the electrical properties. We have therefore tested 10 sensing units with distinct compositions, four of which were found to be sufficiently reproducible for an electronic tongue. These electrodes, listed in Table 1, exhibited reproducible electrical responses from triplicate measurements when subjected to 10 µL ultrapure water. Lactose detection was performed in aqueous solutions, by measuring the capacitance spectra with these 4 sensing units for the concentrations: 1 × 10⁻2⁰, 1 × 10⁻1⁸, 1 × 10⁻1⁶, 1 × 10⁻1⁴, 1 × 10⁻12, 1 × 10⁻1⁰, 1 × 10⁻⁸ and 1 × 10⁻⁶ mol/L. Samples of commercial food were also measured: milk cream (Piracanjuba, Brazil), with and without lactose; milk (Piracanjuba, Brazil), with and without lactose; yoghurt (Itambé, Brazil) with lactose and whipped cream with lactose, whose capacitance spectra were analysed using information visualization techniques (Coatrini Soares et al., 2022).

Table 1. Films selected for electrode printing and assembly of the electronic tongue for lactose detection: Film 1: 5% gelatin + 4% tannic acid, prepared at room temperature; Film 2: 5% gelatin + 4% tannic acid dried at 80°C; Film 3: 5% gelatin + 4% tannic acid + zein and tannic acid coating and dried at 80°C; Film 4: 5% gelatin + 4% tannic acid + zein coating dried at 80°C.
3 Results and discussion
The solubility of the self-supporting films was a significant concern since lactose detection was performed in aqueous solutions, and maintaining the substrate integrity is crucial for preserving the physical properties, selectivity, and sensitivity of the electrodes. The average solubility of the gelatin and tannin films, listed in Table 1, ranged between 33% and 36%, as depicted in Supplementary Figure S2. The application of the zein layer was achieved through solution blowing, resulting in a thin coating on the gelatin films (Liu et al., 2020). Although minimal changes in solubility were observed in our study, possibly due to the deposition method, previous studies have reported significant decreases in solubility for films containing both zein and gelatin (Ahammed et al., 2020). To prevent potential interactions between these polymers during solution preparation for casting or spinning, a coating was applied to the gelatin. Such interactions could enhance hydrophobicity due to differing polymer interactions (Ahammed et al., 2020). Nevertheless, the zein-coated films yielded satisfactory results during electrical measurements.
The wettability of the self-supporting films was assessed by measuring the contact angle for water (Supplementary Figure S3). Comparing Films 1 and 2, a higher drying temperature favored surface hydrophobization of the films, as evidenced by an increase in the contact angle from 76° to 93°. The temperature can alter the arrangement of the protein, leading to the opening of the chain and exposure of nonpolar groups (Grossmann and McClements, 2023). For Films 3 and 4, the presence of tannic acid in the zein coating increased the contact angle from 67° to 90°. Tannic acid acts as a crosslinker between the amine and hydroxyl groups of protein chains, rendering the polar groups unavailable to interact with water, thus decreasing the surface hydrophilicity (Firdous et al., 2023). Films without a zein coating appeared to be the most hydrophobic. However, one should note that the spray deposition of zein results in an uneven surface, which can affect the contact angle (Wang et al., 2020). All films exhibited a considerable hydrophobic character (Ɵ > 60°) (Pulla-Huillca et al., 2021), with minor variations in the contact angle within a 60-s period, making them suitable for detecting lactose in aqueous solutions. Films with a hydrophobic coating may offer advantages due to rapid drying, allowing for a larger number of consecutive measurements within a specific time frame. Zein has a hydrophobic character, but its wettability may be even higher than hydrophilic biopolymers due to its microporous structure (Chen et al., 2019). Previous work in our group has also shown that the solvent used during zein deposition (glacial acetic acid) can cause defects on the surface of the protein film that contribute to water adsorption and mask the hydrophobic effect of zein. The SEM images in Supplementary Figure S4 for films without (films 1 and 2) and with zein deposition (films 3 and 4) showed a rough, thin, and non-uniform zein coating that could affect water deposition/adsorption. This led to a high hydrophobic character compared to natural zein.
Figure 2 shows the capacitance spectra obtained with the 4 sensing units corresponding to Film 1 through Film 4. In all cases, the capacitance tends to decrease with the lactose concentration, especially at low frequencies. A higher distinguishing power at this spectral region was expected because it is related to double-layer effects that predominate in organic film/water interfaces (Riul et al., 2003; Barsoukov and Macdonald, 2005; Riul et al., 2010; Lvovich, 2012; Elamine et al., 2019; Braunger et al., 2020; Facure et al., 2021; Rodrigues et al., 2021). Evidence from the literature (Riul et al., 2003; Barsoukov and Macdonald, 2005; Riul et al., 2010; Lvovich, 2012; Elamine et al., 2019; Braunger et al., 2020; Facure et al., 2021; Rodrigues et al., 2021) exists that at low frequencies, double-layer effects are dominant. In particular, in sensing the double-layer capacitance varies depending on how the sample properties are changed. Also worth noting is that Films 3 and 4 had considerably higher capacitances, thus indicating that the self-sustaining films coated with SBS fibers increased electrical charge accumulation.
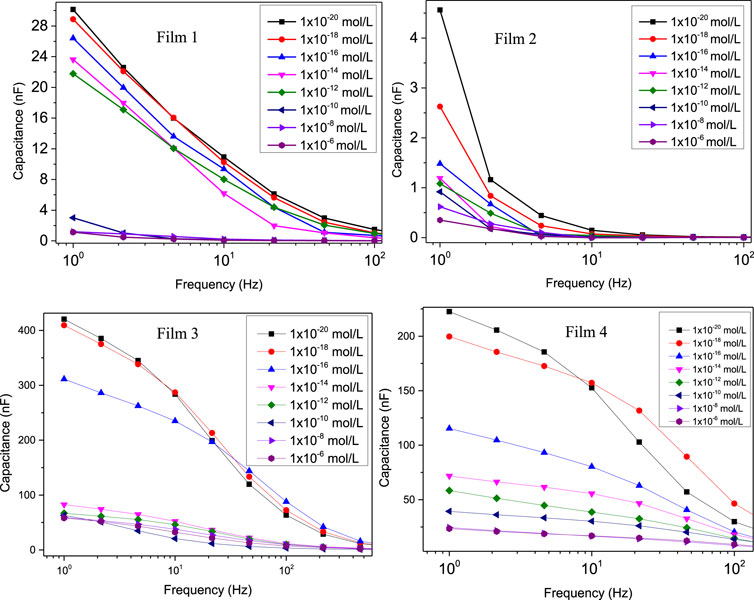
Figure 2. Capacitance spectra to detect different lactose concentrations obtained with sensing units fabricated with: Film 1: 5% gelatin + 4% tannic acid; Film 2: 5% gelatin + 4% tannic acid + 80°C; Film 3: 5% gelatin + 4% tannic acid + zein and tannic acid coating + 80°C; Film 4: 5% gelatin + 4% tannic acid + zein coating + 80°. Note the higher capacitances measured for Films 3 and 4.
To generate calibration curves, we employed the parallel coordinates technique (Lvovich, 2012) to identify frequencies where the differentiation between various lactose concentrations is most pronounced. The parallel coordinate graphs representing data from the four sensing units are presented in Supplementary Figure S5. These graphs consist of equally spaced parallel axes, determined by the intersection of the frequency axis at specific points of the measurement (Soares et al., 2015a; Soares et al., 2020; Coatrini Soares et al., 2022). From these graphs, one can discern the distinguishing ability at each frequency; blue boxes indicate that capacitance values at certain frequencies differ among the samples, while white boxes suggest that capacitance values at these frequencies do not aid in sample differentiation. Frequencies where using capacitance is detrimental to spectral differentiation are marked with red boxes. The calibration curves in Figure 3 were obtained at the most suitable frequencies, indicated in the figure. The limit of detection (LoD) and the limit of quantification (LoQ) were determined using IUPAC method (Currie, 1995; Soares et al., 2020) using Eqs 2, 3, in which SD is the standard deviation of the control samples, S0 is the capacitance signal in the 0 mol/L, SLOD is the capacitance value for limit of detection (read on the calibration curve), SLOQ is the capacitance value for limit of quantification (read on the calibration curve) and 3 or 10 is a constant that depends on the confidence level. Therefore, LoD and LoQ are the lactose concentration values at the SLoD and SLoq point, read from the calibration curves. The LoDs for Films 1, 2, 3, and 4 were 3.07 × 10−12, 9.06 × 10−19, and 3.25 × 10−19; and 2.03 × 10−19 mol/L. The LoQ were 9.21 × 10−12, 27.28 × 10−19, 10.72 × 10−19, and 6.09 × 10−19 mol/L. The most sensitive units have superior performance to biosensors to detect lactose in the literature (Tkáč et al., 2000; Yang et al., 2007; Portaccio and Lepore, 2017; Gursoy et al., 2018; Gursoy et al., 2020; De Brito et al., 2021).
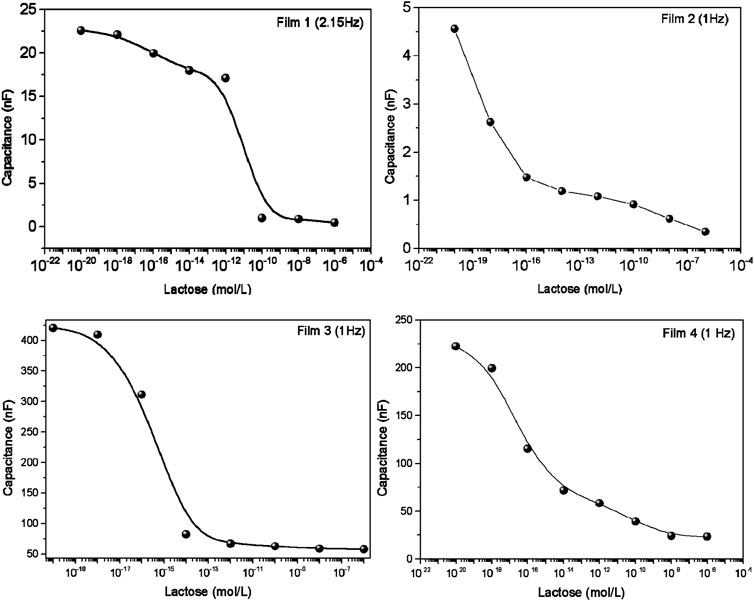
Figure 3. Calibration curves for the sensing units by taking the capacitance versus lactose concentrations at the most suitable frequencies for distinguishing the spectra. For Film 1 (5% gelatin + 4% tannic acid), the frequency was 2.15 Hz; for the other films the frequency used was 1 Hz: Film 2 (5% gelatin + 4% tannic acid + 80°C); Film 3 (5% gelatin + 4% tannic acid + zein and tannic acid coating + 80°C); Film 4 (5% gelatin + 4% tannic acid + zein coating + 80°C).
The ability of the sensing units to distinguish among the various lactose concentrations was confirmed by projecting the capacitance spectra on a 2-D map in Figure 4 using the IDMAP technique (Minghim et al., 2006; Paulovich et al., 2011). This map is obtained by calculating the Euclidean distances between the spectra in the original space, and them projecting them on the 2D space (Minghim et al., 2006; Paulovich et al., 2011). These projections are calculated using Eq. 4 where δmax and δmin are maximum and minimum Euclidean distances between the data instances, respectively. The silhouette coefficient (S) varies between −1 and 1, where values close to 1 indicate excellent distinguishing power, whereas S close to 0 or -1 are not helpful to distinguish the different spectra (Paulovich et al., 2011; Soares et al., 2015b). For the 4 sensing units, the Silhouette coefficient (S) was 0.397, 0.655, 0.511 and 0.409 for Films 1, 2, 3, 4, respectively, showing a high selectivity power for some of the sensing units.
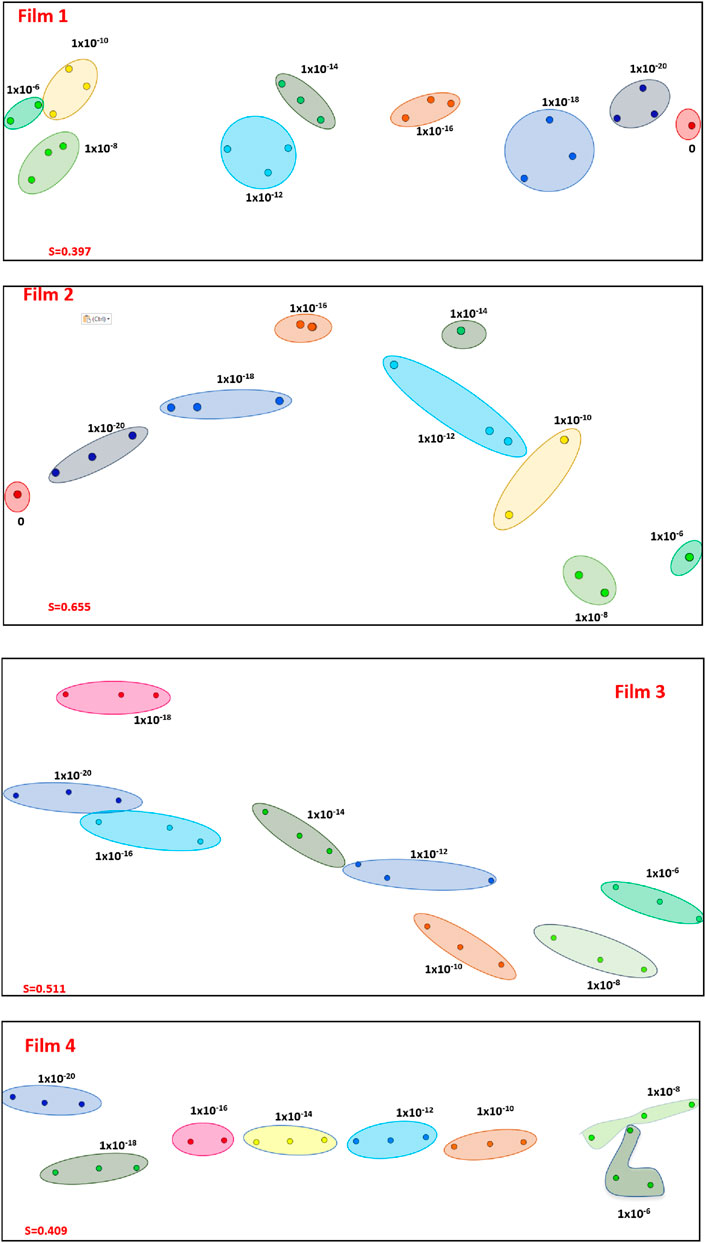
Figure 4. IDMAP projections of the capacitance spectra obtained for various lactose concentrations using the sensing units corresponding to: Film 1: 5% gelatin + 4% tannic acid; Film 2: 5% gelatin + 4% tannic acid + 80°C; Film 3: 5% gelatin + 4% tannic acid + zein and tannic acid coating + 80°C; Film 4: 5% gelatin + 4% tannic acid + zein coating + 80°C. The Silhouette Coefficients are 0.397, 0.655, 0.511 and 0.409 for Films 1, 2, 3 and 4, respectively.
The data acquired with the 4 sensing units were combined, thus forming an electronic tongue. Taking advantage from the parallel coordinates technique, we opted not to utilize the entire capacitance spectra; instead, we employed a feature selection procedure and included only the data corresponding to the frequencies marked with blue boxes. The resulting IDMAP projection in Figure 5 demonstrates complete separation of samples with different lactose concentrations, with increasing concentrations shifting towards the left of the map. The Silhouette Coefficient of 0.716 confirms the excellent distinguishing power.
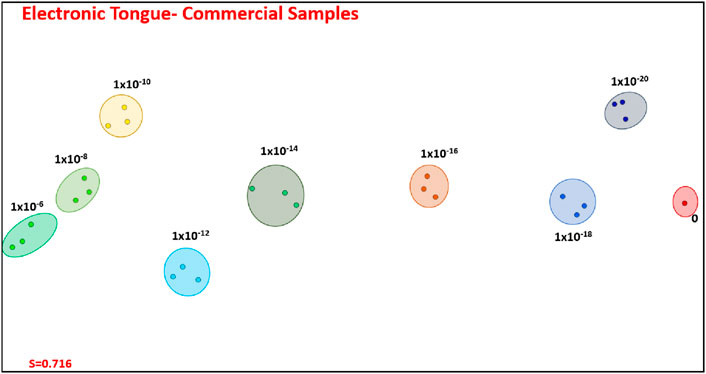
Figure 5. IDMAP plot for the capacitance spectra with feature selection for amalgamated data obtained with the four sensing units, thus corresponding to an electronic tongue: Film 1 through Film 4. Note that the high distinguishing power of this electronic tongue leads to a silhouette coefficient of 0.716.
The electronic tongue can be employed with commercial foods readily available in supermarkets. To demonstrate this capability, we selected various items, and the capacitance data—with feature selection where only the most suitable frequencies were chosen in Supplementary Figure S4—are projected in Figure 6. It is evident that food samples devoid of lactose could be easily distinguished from those containing lactose. Furthermore, lactose-free milk and lactose-free milk cream were distinguishable from their lactose-containing counterparts. While specific measurements to quantify lactose concentration were not conducted, it is apparent that food samples with higher lactose concentrations were positioned to the left of the IDMAP plot. Despite some overlap observed, particularly in clusters for milk and milk cream with added lactose, the electronic tongue exhibits a high discriminating power, with a silhouette coefficient of 0.549. The electrodes obtained showed performance comparable to regular electrodes, generally made of synthetic material (Tkáč et al., 2000; Yang et al., 2007; Portaccio and Lepore, 2017; Gursoy et al., 2018; Gursoy et al., 2020; De Brito et al., 2021). Due to the low toxicity, biocompatibility, and low cost of fabrication, these electrodes have the potential to be applied in the food, clinical, and environmental areas. Furthermore, using a hydrophobic zein coating helped with the stability of the electrodes in an aqueous medium, as they could be reused, and improved the affinity between the hydrophilic gelatin support and the carbon black ink. Normally, the electrodes are disposable, leading to electronic waste generation. Therefore, the use of biobased electrodes is an alternative to this problem.
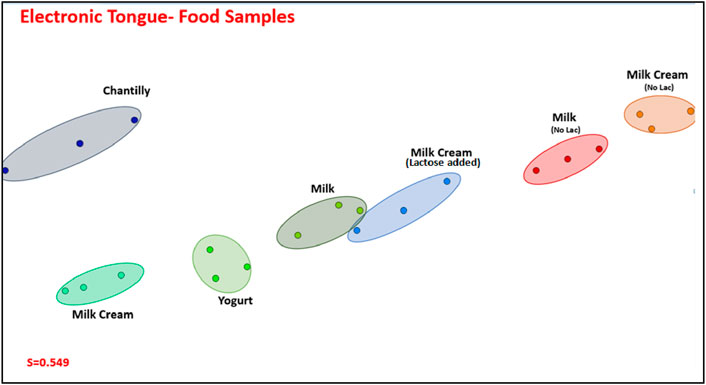
Figure 6. IMAP projection of the capacitance spectra, with feature selection, for the electronic tongue comprising Films 1, 2, 3, and 4.
4 Conclusion
We have shown that an electronic tongue consisting of self-supporting gelatin films, on which electrodes from carbon ink were printed, could detect lactose across various food samples, exhibiting the lowest reported limits of detection and quantification in existing literature. When the capacitance spectra were projected using IDMAP with feature selection, the discriminating power of the electronic tongue was confirmed with high silhouette coefficients. These coefficients were 0.716 for distinction of lactose concentrations from 1 × 10−20 up to 1 × 10−6 mol/L, and 0.549 in distinguishing among lactose-free and lactose-containing foods. Though no attempts were made to verify the biodegradability of the sensing units, one may expect that gelatin-based films will be biodegradable and even edible. Once the feasibility of lactose detection was demonstrated, further avenues to pursue in research on this type of electronic tongue may explore surface functionalization. Applications beyond lactose detection can be envisaged, particularly with the expected biocompatibility of the self-supporting gelatin films. There is potential for application in implantable sensors and biosensors, broadening the scope of this research into healthcare and biomedical fields.
Data availability statement
The raw data supporting the conclusion of this article will be made available by the authors, without undue reservation.
Author contributions
AP: Conceptualization, Data curation, Formal Analysis, Investigation, Methodology, Project administration, Validation, Visualization, Writing–original draft, Writing–review and editing. AC-S: Conceptualization, Data curation, Formal Analysis, Investigation, Methodology, Project administration, Validation, Visualization, Writing–original draft, Writing–review and editing. GF: Formal Analysis, Investigation, Methodology, Validation, Visualization, Writing–original draft, Writing–review and editing. TB: Formal Analysis, Investigation, Methodology, Validation, Visualization, Writing–original draft, Writing–review and editing. JC-S: Formal Analysis, Investigation, Methodology, Validation, Visualization, Writing–original draft, Writing–review and editing. OO: Formal Analysis, Funding acquisition, Investigation, Methodology, Project administration, Supervision, Validation, Visualization, Writing–original draft, Writing–review and editing. LM: Writing–review and editing, Formal Analysis, Funding acquisition, Investigation, Methodology, Project administration, Supervision, Validation, Visualization, Writing–original draft.
Funding
The author(s) declare that financial support was received for the research, authorship, and/or publication of this article. This work was supported by FIPAI, CAPES (001), CNPq (151567/2022-0, 406925/2022-4) and FAPESP (2018/22214-6, 2018/18953-8, 2023/11140-0 and 2020/09835-1).
Acknowledgments
The authors thanks INEO, Rede Agronano, SISNANO-MCTI.
Conflict of interest
The authors declare that the research was conducted in the absence of any commercial or financial relationships that could be construed as a potential conflict of interest.
Publisher’s note
All claims expressed in this article are solely those of the authors and do not necessarily represent those of their affiliated organizations, or those of the publisher, the editors and the reviewers. Any product that may be evaluated in this article, or claim that may be made by its manufacturer, is not guaranteed or endorsed by the publisher.
Supplementary material
The Supplementary Material for this article can be found online at: https://www.frontiersin.org/articles/10.3389/fsens.2024.1401077/full#supplementary-material
References
Ahammed, S., Liu, F., Khin, M. N., Yokoyama, W. H., and Zhong, F. (2020). Improvement of the water resistance and ductility of gelatin film by zein. Food Hydrocoll. 105, 105804. doi:10.1016/j.foodhyd.2020.105804
Barsoukov, E., and Macdonald, J. R. (2005) Impedance spectroscopy theory, experiment, and applications. Hoboken, N.J.: Wiley-Interscience. Available at: http://site.ebrary.com/id/10114201 (Accessed June 22, 2021).
Bondancia, T. J., Soares, A. C., Popolin-Neto, M., Gomes, N. O., Raymundo-Pereira, P. A., Barud, H. S., et al. (2022). Low-cost bacterial nanocellulose-based interdigitated biosensor to detect the p53 cancer biomarker. Mater. Sci. Eng. C 134, 112676. doi:10.1016/j.msec.2022.112676
Braunger, M. L., Fier, I., Shimizu, F. M., De Barros, A., Rodrigues, V., and Riul, A. (2020). Influence of the flow rate in an automated microfluidic electronic tongue tested for sucralose differentiation. Sensors 20, 6194. doi:10.3390/s20216194
Buscaglia, L. A., Oliveira, O. N., and Carmo, J. P. (2021). Roadmap for electrical impedance spectroscopy for sensing: a tutorial. IEEE Sensors J. 21, 22246–22257. doi:10.1109/JSEN.2021.3085237
Chen, X., Cui, F., Zi, H., Zhou, Y., Liu, H., and Xiao, J. (2019). Development and characterization of a hydroxypropyl starch/zein bilayer edible film. Int. J. Biol. Macromol. 141, 1175–1182. doi:10.1016/j.ijbiomac.2019.08.240
Coatrini Soares, A., Coatrini Soares, J., Popolin-Neto, M., Scarpa de Mello, S., Dos Santos Cinelli Pinto, D., Araújo Carvalho, W., et al. (2022). Microfluidic E-tongue to diagnose bovine mastitis with milk samples using machine learning with decision tree models. Chem. Eng. J. 451, 138523. doi:10.1016/j.cej.2022.138523
Currie, L. A. (1995). Nomenclature in evaluation of analytical methods including detection and quantification capabilities (IUPAC Recommendations 1995). Pure Appl. Chem. 67, 1699–1723. doi:10.1351/pac199567101699
De Brito, A. R., De Jesus, R. S., Tavares, I. M. D. C., Silva, F. N., Santana, N. B., Barbosa Ferrão, S. P., et al. (2021). Application of the electrochemical biosensor in the detection of lactose in skimmed milk. Surfaces Interfaces 22, 100839. doi:10.1016/j.surfin.2020.100839
Elamine, Y., Inácio, P. M. C., Lyoussi, B., Anjos, O., Estevinho, L. M., Da Graça Miguel, M., et al. (2019). Insight into the sensing mechanism of an impedance based electronic tongue for honey botanic origin discrimination. Sensors Actuators B Chem. 285, 24–33. doi:10.1016/j.snb.2019.01.023
Facure, M. H. M., Braunger, M. L., Mercante, L. A., Paterno, L. G., Riul, A., and Correa, D. S. (2021). “Electrical impedance-based electronic tongues: principles, sensing materials, fabrication techniques and applications,” in Reference module in biomedical sciences (Germany: Elsevier), B9780. doi:10.1016/B978-0-12-822548-6.00091-1
Firdous, S. O., Arif, K., Wong, S. Y., Li, X., and Arafat, M. T. (2023). In situ crosslinked electrospun gelatin matrix with unoxidized tannic acid through stable hydrogen bonding. J Appl. Polym. Sci 140, e54572. doi:10.1002/app.54572
Grossmann, L., and McClements, D. J. (2023). Current insights into protein solubility: a review of its importance for alternative proteins. Food Hydrocoll. 137, 108416. doi:10.1016/j.foodhyd.2022.108416
Gursoy, O., Sen Gursoy, S., Cogal, S., and Celik Cogal, G. (2018). Development of a new two-enzyme biosensor based on poly(pyrrole-co-3,4-ethylenedioxythiophene) for lactose determination in milk. Polym. Eng. Sci 58, 839–848. doi:10.1002/pen.24632
Gursoy, S. S., Yildiz, A., Cogal, G. C., and Gursoy, O. (2020). A novel lactose biosensor based on electrochemically synthesized 3,4-ethylenedioxythiophene/thiophene (EDOT/Th) copolymer. Open Chem. 18, 974–985. doi:10.1515/chem-2020-0100
Jia, C., Cao, D., Ji, S., Zhang, X., and Muhoza, B. (2020). Tannic acid-assisted cross-linked nanoparticles as a delivery system of eugenol: the characterization, thermal degradation and antioxidant properties. Food Hydrocoll. 104, 105717. doi:10.1016/j.foodhyd.2020.105717
Keller, S. S., Gammelgaard, L., Jensen, M. P., Schmid, S., Davis, Z. J., and Boisen, A. (2011). Deposition of biopolymer films on micromechanical sensors. Microelectron. Eng. 88, 2297–2299. doi:10.1016/j.mee.2011.02.093
Leite, L. S. F., Bilatto, S., Paschoalin, R. T., Soares, A. C., Moreira, F. K. V., Oliveira, O. N., et al. (2020). Eco-friendly gelatin films with rosin-grafted cellulose nanocrystals for antimicrobial packaging. Int. J. Biol. Macromol. 165, 2974–2983. doi:10.1016/j.ijbiomac.2020.10.189
Liu, C., Huang, J., Zheng, X., Liu, S., Lu, K., Tang, K., et al. (2020). Heat sealable soluble soybean polysaccharide/gelatin blend edible films for food packaging applications. Food Packag. Shelf Life 24, 100485. doi:10.1016/j.fpsl.2020.100485
Lvovich, V. F. (2012) Impedance spectroscopy: applications to electrochemical and dielectric phenomena. Hoboken, N.J: Wiley.
Minghim, R., Paulovich, F. V., and de Andrade Lopes, A. (2006). in Content-based text mapping using multi-dimensional projections for exploration of document collections. Editors R. F. Erbacher, J. C. Roberts, M. T. Gröhn, K. Borner, and C. A. San Jose, 60600S. doi:10.1117/12.650880
Neto, M. P., Soares, A. C., Oliveira, O. N., and Paulovich, F. V. (2021). Machine learning used to create a multidimensional calibration space for sensing and biosensing data. BCSJ 94, 1553–1562. doi:10.1246/bcsj.20200359
Otoni, C. G., Avena-Bustillos, R. J., Azeredo, H. M. C., Lorevice, M. V., Moura, M. R., Mattoso, L. H. C., et al. (2017). Recent advances on edible films based on fruits and vegetables—a review. Comp. Rev. Food Sci. Food Safe 16, 1151–1169. doi:10.1111/1541-4337.12281
Paulovich, F. V., Moraes, M. L., Maki, R. M., Ferreira, M., Oliveira, O. N., and de Oliveira, M. C. F. (2011). Information visualization techniques for sensing and biosensing. Analyst 136, 1344. doi:10.1039/c0an00822b
Portaccio, M., and Lepore, M. (2017). Determination of different saccharides concentration by means of a multienzymes amperometric biosensor. J. Sensors 2017, 1–8. doi:10.1155/2017/7498945
Pulla-Huillca, P. V., Gomes, A., Quinta Barbosa Bittante, A. M., Lourenço, R. V., and Sobral, P. J. D. A. (2021). Wettability of gelatin-based films: the effects of hydrophilic or hydrophobic plasticizers and nanoparticle loads. J. Food Eng. 297, 110480. doi:10.1016/j.jfoodeng.2021.110480
Rattaya, S., Benjakul, S., and Prodpran, T. (2009). Properties of fish skin gelatin film incorporated with seaweed extract. J. Food Eng. 95, 151–157. doi:10.1016/j.jfoodeng.2009.04.022
Riul, A., Gallardo Soto, A. M., Mello, S. V., Bone, S., Taylor, D. M., and Mattoso, L. H. C. (2003). An electronic tongue using polypyrrole and polyaniline. Synth. Met. 132, 109–116. doi:10.1016/S0379-6779(02)00107-8
Riul, A., Dantas, C. A. R., Miyazaki, C. M., and Oliveira, O. N. (2010). Recent advances in electronic tongues. Analyst 135, 2481. doi:10.1039/c0an00292e
Rodrigues, D. R., Fragoso, W. D., and Lemos, S. G. (2021). Electronic tongue based on a single impedimetric sensor and complex numbers-supervised pattern recognition. Electrochimica Acta 397, 139312. doi:10.1016/j.electacta.2021.139312
Soares, A. C., Soares, J. C., Dos Santos, D. M., Migliorini, F. L., Popolin-Neto, M., Dos Santos Cinelli Pinto, D., et al. (2023). Nanoarchitectonic E-tongue of electrospun zein/curcumin carbon dots for detecting Staphylococcus aureus in milk. ACS Omega 8, 13721–13732. doi:10.1021/acsomega.2c07944
Soares, A. C., Soares, J. C., Rodrigues, V. C., Oliveira, O. N., and Capparelli Mattoso, L. H. (2020). Controlled molecular architectures in microfluidic immunosensors for detecting Staphylococcus aureus. Analyst 145, 6014–6023. doi:10.1039/D0AN00714E
Soares, A. C., Soares, J. C., Shimizu, F. M., Melendez, M. E., Carvalho, A. L., and Oliveira, O. N. (2015a). Controlled film architectures to detect a biomarker for pancreatic cancer using impedance spectroscopy. ACS Appl. Mater. Interfaces 7, 25930–25937. doi:10.1021/acsami.5b08666
Soares, J. C., Shimizu, F. M., Soares, A. C., Caseli, L., Ferreira, J., and Oliveira, O. N. (2015b). Supramolecular control in nanostructured film architectures for detecting breast cancer. ACS Appl. Mater. Interfaces 7, 11833–11841. doi:10.1021/acsami.5b03761
Song, J., Winkeljann, B., and Lieleg, O. (2020). Biopolymer-based coatings: promising strategies to improve the biocompatibility and functionality of materials used in biomedical engineering. Adv. Mater. Inter 7, 2000850. doi:10.1002/admi.202000850
Tkáč, J., Šturdík, E., and Gemeiner, P. (2000). Novel glucose non-interference biosensor for lactose detection based on galactose oxidase–peroxidase with and without co-immobilised β-galactosidase. Analyst 125, 1285–1289. doi:10.1039/b001432j
Wang, J., Wu, Y., Cao, Y., Li, G., and Liao, Y. (2020). Influence of surface roughness on contact angle hysteresis and spreading work. Colloid Polym. Sci. 298, 1107–1112. doi:10.1007/s00396-020-04680-x
Yang, W., Pang, P., Gao, X., Cai, Q., Zeng, K., and Grimes, C. A. (2007). Detection of lactose in milk samples using a wireless multi-enzyme biosensor. Sen. Lett. 5, 405–410. doi:10.1166/sl.2007.212
Keywords: polysaccharides, sensors, electronic tongue, silk-screen, gelatin films
Citation: Piccinin ACV, Coatrini-Soares A, Franco GT, Bondancia TJ, Coatrini-Soares J, Oliveira ON Jr and Mattoso LHC (2024) Electronic tongue made of gelatin self-supporting films on printed electrodes to detect lactose. Front. Sens. 5:1401077. doi: 10.3389/fsens.2024.1401077
Received: 14 March 2024; Accepted: 16 May 2024;
Published: 04 June 2024.
Edited by:
Antonio Riul Jr, State University of Campinas, BrazilReviewed by:
Ashlesha Bhide, Roche, United StatesMaria Luisa Braunger, National Center for Research in Energy and Materials, Brazil
Leonardo Paterno, University of Brasilia, Brazil
Copyright © 2024 Piccinin, Coatrini-Soares, Franco, Bondancia, Coatrini-Soares, Oliveira and Mattoso. This is an open-access article distributed under the terms of the Creative Commons Attribution License (CC BY). The use, distribution or reproduction in other forums is permitted, provided the original author(s) and the copyright owner(s) are credited and that the original publication in this journal is cited, in accordance with accepted academic practice. No use, distribution or reproduction is permitted which does not comply with these terms.
*Correspondence: Osvaldo N. Oliveira, Y2h1QGlmc2MudXNwLmJy