- 1Nanoscience Program, Zewail City of Science and Technology, University of Science and Technology (UST), Giza, Egypt
- 2Biosensors Research Lab, Zewail City of Science and Technology, University of Science and Technology (UST), Giza, Egypt
Regardless of many years of research work and conducting numerous clinical studies, breast cancer (BC) continues to grow among women, threatening their lives and future. Currently, it affects more than 10% of women globally. Therefore, researchers have recently redirected their endeavors toward detecting BC at an early stage, recognizing its substantial impact on survival rates, and acknowledging its immense potential for providing effective cancer treatments at this early diagnosed disease stage. The utilization of biomarker-based diagnostic techniques significantly contributes to the advancement of early detection and precision. A wide range of biomarkers is linked to every newly diagnosed BC case. Traditionally, breast cancer was diagnosed using a variety of techniques such as mammography, molecular imaging, and ultrasonography. These techniques had several drawbacks, drawing the attention of many scientists to developing new methods and strategies. Among these strategies, electrochemical biosensors have proven to be excellent alternatives to more complex and traditional detection strategies in terms of performance, accuracy, robustness, and a tremendous range of applications. In this review, the use of conventional methods for detecting BC as well as their challenges and shortcomings have been stated. Moreover, a deep discussion was given to the newly developed nanocomposite-based electrochemical bio-sensing approaches that have been exploited for the effective detection and quantification of breast cancer biomarkers.
Introduction
Anciently, cancer has been known for millennia, dating back to the Ancient Egyptians. They mentioned several types of tumors on papyrus, including breast, uterine, and skin tumors. Cancer ranks among the leading causes of mortality worldwide (Barzaman et al., 2020). The rate of occurrence and death because of cancer are expected to increase as compared to the past due to many factors and it is predicted that cases of cancer will double globally in the next 50 years (Nicolini et al., 2018). In 2020, 19.3 million new cancer cases were positively diagnosed, and 9.9 million died, according to the International Agency for Research on Cancer (IARC) (Kashyap et al., 2022).
Among the different types of cancer, breast cancer (BC) has the highest mortality rate, hence, it hinders the lives of many people globally (Zhang et al., 2021). Specifically, women are more likely to have BC, and according to the recently updated global cancer statistics published by the IARC, the incidence of BC exceeded that of lung cancer in 2020. Therefore, it has become the most common type of cancer worldwide. It is not only in females, but also some incidences are reported on male breast cancer (MBC), which is not common and it just accounts for about 1% of all positively diagnosed breast cancer worldwide cases (Fox et al., 2022).
Since the early diagnosis supports the increase in the survival rates for many cancer types, targeting and identifying BC biomarkers will improve the speed and precision of detection (Najjar and Allison, 2022). There are several biomarkers associated with the presence of BC, such as human epidermal growth factor receptor 2, transmembrane glycoprotein Mucin 1, BC gene 1, TP53, estrogen (ER), BC gene 2, progesterone receptor (PR) and many other biomarkers. Several and various improvements in diagnostic techniques have occurred in the last few decades, leading to the present conventional diagnostic techniques.
Breast biopsy, molecular imaging, and ultrasonography are commonly part of the conventional techniques (Woolpert et al., 2024). These techniques, however, often require complex procedures, sophisticated equipment, and/or long and tedious analysis. Thus, it is essential to prioritize the development of efficient, straightforward, and widely accessible techniques for BC analysis, as they are considered as a critical factor in shaping the future of BC treatment (Seale and Tkaczuk, 2022; Tarighati et al., 2023).
To overcome the previous obstacles, numerous bio-sensing platforms have been designed and exploited for the detection of the designated biomarkers, such as physical, optical, and electrochemical biosensors (Magar and Hassan, 2021). Among these types, electrochemical biosensors have shown great efficacy in dealing with BC biomarkers as well as overcoming many other challenges. Biosensors are not only non-invasive techniques, but they also manage to overcome the issues regarding price, portability, and sensitivity. To better enhance the properties of electrochemical biosensors, different classes of nanomaterials were involved to improve the signal transduction and amplification. Moreover, they enhance the conductivity, the active electrochemical surface area, and the electrochemical bio-reactivity, leading to a great increase in the sensitivity and specificity of the sensor. Nanocomposites, over the other nanomaterial-based sensors, exhibited superior functions as electrode (sensor’s surface) modifiers used for cancer diagnosis. Since the conventional diagnostic techniques were subjected to considerable criticisms (e.g., their large, complicated, and expensive devices that require well-trained operators, specialized laboratories, and the long time required to perform the analysis), creating novel methods to overcome these obstacles is crucial for the future of bio-analytics and disease diagnosis. Accordingly, this review article is designed and devoted to discuss the principles, challenges, and limitations of most common types of conventional techniques employed in the process of BC diagnosis. Moreover, it sheds the light on the advanced diagnosis techniques, focusing on the electrochemical biosensor-based nanocomposites employed in the early detection of BC biomarkers.
Conventional techniques for the cancer diagnosis
Adequate and early screening of cancer is essential to control its prevalence and alleviate its resulting health complications. It also provides access to effective treatments in a short time and allows monitoring of the implemented therapies; thus, this will increase the survival rate. The most currently used conventional technique is mammography, as the gold standard for cancer detection (Nounou et al., 2015). It is conducted using low doses of x-rays to examine the whole cancer tissue horizontally and vertically in order to detect any changes, lesions, or lumps. It generates 2D mammograms because of superimposing multiple images, and it can identify any distortions or abnormal masses (Di Maria et al., 2022).
Physicians interpret the resulting mammograms according to Breast Imaging Reporting and Data System (BI-RADS) (Magny et al., 2024). Nevertheless, it still needs another confirmation or liquid biopsy to confirm the disease to avoid any misinterpretation resulting from the tissue deformities (Freitas et al., 2022). Furthermore, the use of mammography is limited, where young patients and ones with dense breast tissue cannot be accurately diagnosed. Accordingly, mammography sensitivity and specificity are deeply influenced by age, breast implants and dense tissues, where they can mask any cancerous tissue.
To overcome such drawbacks, scientists developed new modalities such as digital (full-field) mammography (DM). This modality exploits computer-aided diagnosis and algorithm-based software to construct 2D X-ray views of the tissue and interpret it by specialists (Medical Advisory Secretariat, 2010; Song et al., 2019). It is privileged in the lower radiation exposure and enhanced sensitivity and specificity of 70% and 92% respectively despite the young age and dense breast tissue.
Another advanced modality is digital breast tomosynthesis (DBT), where it is FDA-approved partial tomographic technique used to develop high-resolution 3D images of the tissue (Geras et al., 2019; Choudhery et al., 2021). It uses a rotating x-ray beam to generate multiple views; thus, it reduces the masking of diseased areas leading to higher detection rates and lower recall rates. However, this technique takes a longer time for data to be interpreted due to the large number of images taken and analyzed. In addition, it is expensive and still requires radiation and uncomfortable operation. Thus, computer-aided detection (CAD) was developed to surpass such pitfalls and reduce the interpretation duration (Magnuska et al., 2022; Kim et al., 2023). In CAD, the algorithms are tailored to identify any suspicious distortions in the tissue.
Further, machine learning (ML), and artificial intelligence (AI) are involved to create effective solutions and sophisticated programs to be used for high interpretation efficiency and low false negatives. However, ML, and AI applications are still under investigation (Pacilè et al., 2020; Sechopoulos et al., 2021).
Breast biopsy technique
Breast biopsy is a definitive invasive technique used for the cancer diagnosis when there are suspicious lumps in the breast tissues or abnormal breast shape and discharges (Kalinyak et al., 2011; Arshad et al., 2022; Lowry et al., 2022). It is performed mainly through needle biopsy; this method involves two ways to draw the sample from breast cells. The first one is fine needle aspiration cytology (FNAC); it is the least invasive and done by inserting hollow needles in the suspicious area to get the sample for further pathological analysis (Kazi et al., 2017). The second one is core needle biopsy (CNB), which uses a larger needle to draw cylinder-like samples from the breast tissue, and it is considered more invasive (Lewitowicz et al., 2015). Such types of needle biopsy can be assisted with either magnetic resonance imaging or ultrasonography to confirm the sampling area to avoid any errors. Another type of biopsy is the liquid biopsy; it is a promising complementary way for BC diagnosis (Freitas et al., 2022). It involves drawing a sample from the blood vessels near the cancerous tissue or other biological fluids that contain extracellular vesicles like exosomes, circulating tumor DNA, and cells (Lianidou and Markou, 2011; Azevedo et al., 2018). These molecules are low in concentration in normal subjects and high in concentration in case of autoimmune disorders and cancers. Therefore, they can be collected and analyzed in the process of cancer detection allowing early diagnosis. However, breast biopsies can be subjected to human error of sampling, and it is an invasive method; so, it limits the early monitoring and surveillance of cancer stages and continuous analysis of patients.
Molecular imaging technique
Magnetic resonance imaging (MRI), unlike mammography, is a highly sensitive technique that uses magnetic fields to analyze the lesions and lumps in the tissues (Wekking et al., 2023). It is not considered as the main imaging technique for breast cancer; nevertheless, it is used as a confirmation step especially in the early diagnosis. MRI depends on intravenous injection of contrast agents such as gadolinium and iron oxides nanostructures to generate high-resolution images (Di Corato et al., 2013). Owing to the fact that the vascularization of cancerous tissues has high permeability, the contrast agents can easily enter the target tissue and reside. Scientists used different contrasting agents to improve the efficiency and the resolution of the imaging process; however, these agents resulted in off-targeting effects in many cases. Moreover, some metal complexes can undergo trans-metallation causing toxicities reducing their efficiency. Therefore, researchers developed novel functionalized nanocarriers loaded with contrasting agents to enhance the targeting effects and reduce the toxicities. One of such nanosystems are superparamagnetic iron oxide nanoparticles (SPIONs); which were used as a carrier and can be manipulated easily with external magnetic fields (Vangijzegem et al., 2023). They were utilized as MRI contrast agents to aid in the detection of many cancer types with high targeting efficiency (Zheng et al., 2018; Talluri and Malla, 2019). Other modalities of MRI were developed such as chemical exchange saturation transfer MRI and dynamic contrast-enhanced MRI to enhance the imaging accuracy (Zhang et al., 2019; Onishi et al., 2020). Along with using conventional contrast agents, researchers examined radioactive chemicals to monitor the BC progression as a molecular breast imaging technique (Schillaci et al., 2013). However, MRI still requires complex equipment, invasive intervention, and exposure to radioactive tracers. This refrains it from spanning all healthcare systems and limits the follow-up of the patients. Moreover, there were several false positive results generated from MRI scans. Accordingly, this technique lacks high specificity and accuracy.
Ultrasonography technique
Ultrasonography (US) is an alternative imaging technique applied to confirm the diagnosis of BC using high-frequency sound waves. It is a powerful tool used to differentiate between the fluid cysts and solid lumps, and it does not require any ionizing radiation (Guo et al., 2018; Obeagu and Obeagu, 2024). In addition, ultrasounds can assess various distortions, lesions, and morphologies.
Previous studies showed that the ultrasonography could be used as a follow-up to mammography, where it has higher rates of detection especially in women with dense breasts. Three-dimensional US is a common technique, applied to generate 3D images either by reconstructing 2D views, or by using matrix array transducers to form 3D scans. Another type is elasto-sonography, where by applying slight non-invasive tissue deformations and using echo data. Hence, the instrument operator can receive data regarding the tissue stiffness using a hand-held probe (Tan et al., 2023). However, it cannot discriminate between the normal and cancerous tissues if they have the same elasticity. Advancements were further implemented on such techniques to overcome the fact that it is operator-dependent and consequently non-reproducible. Automated breast sonography is an advanced US technique used to surpass such drawbacks, where it employs a transducer paddle adjusted over the breast tissue to slightly compress it and start to scan. Furthermore, by using certain computer algorithms, 3D views could be generated without intervention of any specialist (Vourtsis, 2019).
Ultimately, the US technique is more sensitive than mammography in screening dense breast tissues as well as contrast-enhanced US, as it could distinguish between benign and malignant tumors. Some reports showed that combining US and MRI or US and mammography techniques could reduce time and cost and increase the efficiency of imaging. These fusions are more sensitive than MRI- and US-guided biopsies; so, they compensate for the pitfalls of each imaging technique alone (Smetherman, 2013; Fiorica, 2016).
Immunohistochemistry
Immunohistochemistry is a general bio-analytical technique, in which one ore multiple selected antibodies are used to identify the expression levels of specific antigens. Since there are several specific breast cancer biomarkers, targeting those biomarkers is considered as the most powerful tool for breast cancer detection. Human epidermal growth factor receptor 2 (HER2) is an essential protein involved in the progression of BC (Krishnamurti and Silverman, 2014; Marchiò et al., 2021). Along with HER2, there are protein-based biochemical markers in serum such as tetraspanin CD9 and CD82, Annexin-1, heat shock protein 70, and metalloprotease ADAM1 (Brzozowski et al., 2018). Also, there are nucleic acid-based BC biomarkers like microRNAs (miR), P53 gene mutations, and BRCA1 gene (Bonacho et al., 2020). The most common breast cancer biomarkers that have been intensively reported in the literature are collected and tabulated in Table 1.
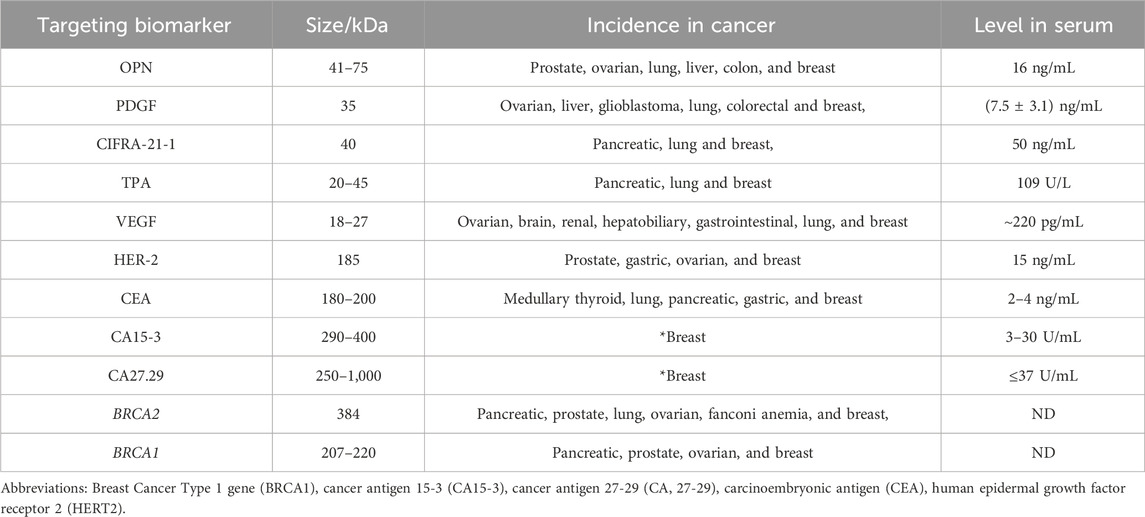
Table 1. Selected potential biomarkers for breast cancer (OPN, PDGF, CIFRA-21-1, tPA, VEGF, HER-2, CEA, CA 15-3, CA27.29, BRCA2, and BRCA1). Among the listed biomarkers (CA15-3 and CA27.29) are remarkable selective biomarkers for the BC diagnosis (Gajdosova et al., 2020).
Hannafon et al reported that concentration of miR-1246 and miR-21 in plasma were significantly increased in BC patients than healthy subjects (Hannafon et al., 2016). Such types of biomarkers were exploited to detect this disease with high sensitivity and specificity using the conventional immunoassays. Accordingly, enzyme-linked immunosorbent assay (ELISA) is used for the qualitative and quantitative diagnosis. ELISA uses antibodies specific to desired biomolecules or antigens. S. Mohan et al investigated N-hydroxy L-Arginine (NOHA) as a new biomarker in plasma to discriminate between patients with estrogen-negative and positive BC using ELISA technique (Mohan et al., 2018). They evaluated its sensitivity, specificity and precision using NOHA antibodies; where the assay reached ultra-high sensitivity with the limit of detection of 60 pg/mL. Therefore, this technique is widely exploited for biomarkers detection and selective diagnosis.
Advanced techniques for cancer diagnosis
Fast and accurate detection of various biomarkers at several molecular levels is a desired target for improving the breast cancer prognosis and patients’ health status. Conventional techniques for detecting cancer biomarkers are subjected to considerable criticisms due to their large samples, complicated, and expensive devices that require well-trained operators, specialized laboratories, and the long time required to perform the analysis. Consequently, developing alternative methods to overcome these obstacles is crucial for shaping the future of bio-analytics and disease diagnosis.
Biosensors represent a promising solution to the previously mentioned problems of conventional methods. Biosensors are non-invasive devices exhibiting extreme sensitivity and selectivity for specific biomarkers. They are characterized by having reasonable prices, fast assessment, and accurate measurement. Moreover, qualitative and quantitative measurements could be carried out using bio-sensing systems facilitating studying and explaining various cellular phenomena (Hassan, 2022). Biosensors are tailored-based platforms, in which specific approaches could be tailored and designed, for the direct tracking of the levels and concentrations of certain types of analytes in different biological or environmental matrices without any sample pre-treatments. As shown in Figure 1A, common bio-sensing approaches (techniques) comprise three main components: (1)-Bio-recognition element(s) (the essential element for identification, recognition, and determination of targeting analyte(s)). (2)-transducers (that generates measurable signals). (3)-Signal processing unit(s) (Magar and Hassan, 2021; Arafa et al., 2022). Biosensors are generally categorized, according to the transducer type, as an optical biosensor, electrochemical biosensor, field effect transistor (FET), optical, gravimetric and colorimetric sensing or mass-based biosensors (Hussein et al., 2020).
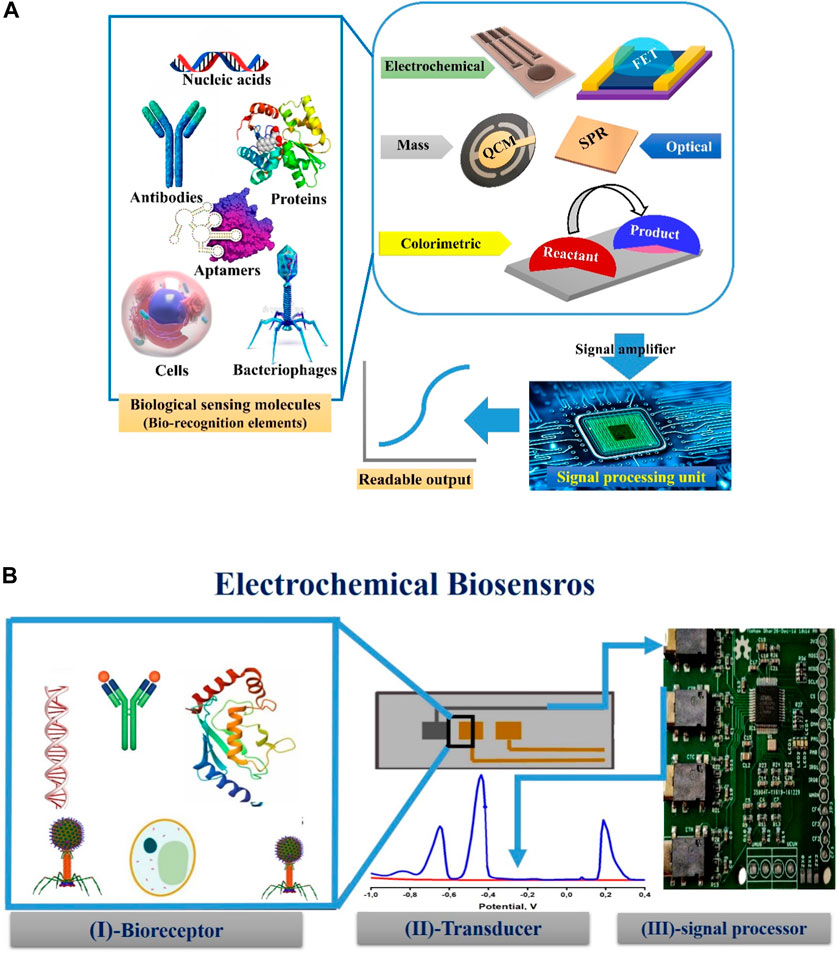
Figure 1. (A) General assembly of biosensors techniques (Electrochemical gravimetric and colorimetric) showing the most common types of biorecognition elements (nucleic acids, antibodies, protein, aptamers, cells, and bacteriophages) that could be immobilized on different transducers to generate readable and amplified signals through signal processing units. QCM, FET, and SPR stand for quartz crystal microbalance, field effect transistor (FET), and surface plasmon resonance, respectively. (B) Three main components of the electrochemical biosensor’s setup: On the active surface area of the transducer (working electrode), a selective sensing element (bio-receptor(s)) could be physically or chemically immobilized. The outcome of the selective interaction is processed and amplified through the third component (signal processing unit).
Electrochemical biosensors
Electrochemical biosensors engage the advantages of selective recognition of targeting analyte(s) and their electrochemical responses (e.g., voltammetric, amperometric, potentiometric or impedimetric signals) to be perfectly used for the rapid, and quantitative analysis in complex matrices. Electrochemical biosensing platforms comprise two or three-electrode setup including working, reference, and auxiliary (counter) electrodes. However, all electrochemical reactions (i.e., bio-catalysis enzymatic reactions, physical attachments and binding affinities, or redox reactions) are carried out on the working electrode surface (Hussein et al., 2020). Accordingly, chemical and physical immobilization of bio-receptors (e.g., proteins, antibodies, aptamers, or enzymes) are strongly influenced by the working electrode materials and their electro-active architecture (Anusha et al., 2023).
Several electrochemical assays, including the electrochemical impedance spectroscopy (EIS), differential pulse voltammetry (DPV), cyclic voltammetry (CV), and square wave voltammetry (SWV) have been developed and intensively used for disease diagnosis and biomarker determination (Rostamabadi and Heydari-Bafrooei, 2019; Freitas et al., 2020; Gajdosova et al., 2020). As shown in Figure 1B, three main individual compartments (sensing elements, transducer, and signal processing unit) are involved in the biosensor setup. Thus, to construct an effective electrochemical approach, certain considerations must be taken into account. First, a designed bio-receptor(s) or bio-sensing element(s) must be identified and selected to be immobilized onto the sensor surface for the selective detection of the analyte(s) (e.g., cells, biological molecules, protein biomarkers, etc.,…).
Second, physical entrapping and attachments of the bio-sensing elements could be simply applied onto the transducer surface, however chemical immobilization is strongly recommended for securing the orientation and vertical alignments of the selected bio-sensing elements. Indeed, this chemical immobilization will ensure the correct direction of bio-sensing active sites, increase the binding affinity and enable the high and fast recognition. Subsequently, this will decrease the possible non-specific (interferences), and increase the overall bio-sensing performance.
Third concern to be considered, controlling the charge (signal) transfer from the active bio-recognition/binding site(s) to the transducer surface is necessary to enable a robust and reliable electrochemical signal. Add to these considerations, blocking non-specific binding sites is necessary to minimize the false-positive feedback of the constructed biosensors. Figure 2 is drawn to summarize the main biosensor considerations that are highly needed for establishing high performance biosensing systems.
First is the selection and correct immobilization of bio-recognition element(s). Second is the understanding and optimizing of the signal transfer mechanism(s) including the direct and mediated electron transfer. In the mediated electron transfer, redox reaction(s) are taking place through the use of artificial electron shuttles. Third stage is the blocking of non-specific binding sites through the use of certain blockers (e.g., bovine serum albumin (BSA)).
Impact of nanomaterials on the biosensor performance
The overall performance of electrochemical biosensors is regulated by the materials used for the fabrication, functionalization, and/or modification of working electrodes and their electro-active architecture (Anusha et al., 2023).
Due to their great improvements in detection sensitivity and ability to conduct accurate analysis, a vast range of nanomaterials are used as reporting molecules, capture probes, nano-carriers, catalysts, and electrode modification materials in the electrochemical biosensors. Moreover, research on various types of electrochemical biosensors utilizing nanocomposites for the detection of transcriptomic, proteomic, and genomic breast cancer biomarkers has grown rapidly in recent years. Nanomaterials are used for sensor surface functionalization, and modification to add certain important electrochemical features including the increase in the electric conductivity, electro-catalytic activity, and support the direct fast electron transfer (Hussein et al., 2023; Duraia et al., 2024). In addition to those electrochemical features, nanomaterials are also used to expand the active surface area of the sensor surface allowing effective loading of bio-recognition elements, supporting the chemical and physical immobilization of the sensing materials, and increasing the sensor life time and signal stability (Ahirwar, 2021; Barhoum et al., 2023; Song et al., 2023). Therefore, in the next subsections, the important roles of nanomaterials and nanocomposites on the performance of different bio-sensing approaches will be intensively discussed.
Immunosensors: antibody-based electrochemical biosensors
Antibodies are the most commonly used bio-receptors in the biosensing process. Combining the properties of antibodies with the biosensors creates a special type called immune-sensors, using a certain antibody (Ab) as a recognition element. Based on the selective binding affinity between the antibody and its targeting antigen on the transducer surface (i.e., the immune-binding affinity resulted from antibody-antigen interactions), immunosensors exhibit superior specificity and sensitivity (Hussein et al., 2023). Sensor transducer transforms this immune-binding affinity into a reasonable electrochemical response which is dependent on the available concentration of free targeting antigen in the introduced samples (Hussein et al., 2023). Several studies have been applied successfully to the detection of breast cancer biomarkers and achieved promising results. In this regard, R. Sakthivel et al designed a superior sandwich-type electrochemical immunosensor by modifying a disposable gold working electrode with gold nanoparticles attached to a transition metal carbide MXene (Ti3C2TX) to support the chemical immobilization of HER2-ECD antibody and to decrease the charge transfer resistances through enhancing the electron transport (Sakthivel et al., 2024). Moreover, they synthesized a nanocomposite made of nitrogen-doped graphene/metal-organic framework together with HER2-ECD antibody to amplify the signal for effective determination of HER2 in patient serum samples. This immunoassay exhibited a linear range of 0.0001–50.0 ng/mL, good stability, and reproducibility; furthermore, it achieved great specificity with a low detection limit of 0.757 pg/mL.
Other examples of the electrochemical immunosensors were developed for the detection of the breast cancer biomarkers. For example, Kazerouni et al presented an innovative method for the electrochemiluminescence (ECL) identification of HER2 antigen. Interestingly, the sensor consists of 18 electrochemical cells, enabling it to simultaneously measure 18 different samples. They depend on the sandwich immunoassay by immobilizing HER2 antibody on the screen-printed carbon electrode modified with Ti3C2 MXenes to facilitate the electron transfer and the attachment of the antibodies. Moreover, they introduced an impressive nanocomposite containing gold nanoprisms for the ECL signal amplification, leading to better analyte detection and an anti-CD63 antibody for making the sandwich structure. The system achieved an excellent LOD of 5.0 pg/mL and showed superior adaptability in measuring other different analytes by utilizing different antibodies, giving room for the detection of several analytes by using the same design with different antibodies (Fathi Kazerouni et al., 2024).
Worth mentioning here that the ECL working principles relied on the electrogenerated chemiluminescence signal produced from bi-products (excited intermediates) generated at the surface of sensor transducers. As a result, a dose of light (luminescence) is emitted due to the relaxation of the excited molecules. Thus, the labeled ECL-based sensors are representing a combination of visual luminescence detection caused from electrochemical reactions. This kind of hybrid optical-electrochemical system can afford high sensitivity in many clinical applications (Cao et al., 2022; Cao et al., 2023; Yuan et al., 2023).
Additionally, N. Kumar et al introduced a platform to detect BC antigen Cluster of Differentiation-44 (CD44) by modifying the glassy carbon electrode with nanocomposites made of graphene quantum dots and gold nanoparticles (GQDs-GNPs). The nanocomposite was made through a simple and eco-friendly electrochemical exfoliation technique. Moreover, the nanocomposite provided excellent electrochemical stability, a large surface area, and several carboxylic functionalities, enhancing the immobilization of anti-CD44 antibodies. The sensor showed high specificity and selectivity for the CD44 biomarker and achieved an excellent LOD of 1.17 fg/mL in phosphate buffer saline (PBS). However, the serum sample showed a LOD of 3.17 fg/mL (Kumar et al., 2023).
Nucleic acid-based electrochemical biosensors (NBEB)
The presence of breast is accompanied by the existence of various nucleic acid-based biomarkers, allowing for its detection and monitoring through various techniques. Fortunately, nucleic acid-based materials are extensively utilized in various fields due to their diversity and their various benefits. Several types of nucleic acid-based substances are extensively used in biosensing because nucleic acids are superior bio-receptors proficient in the detection and recognition of various analytes. Subsequently, incorporating nucleic acid-based materials in biosensors, especially the electrochemical type, represents a promising solution for the process of diagnosis for breast cancer and other types of cancers. Specifically, the resultant NBEB comprises a probe DNA capable of binding to its complementary DNA upon the self-hybridization onto the sensor surface, resulting in an electrochemical signal that varies with the concentration of the target biomarker (Jing et al., 2022).
Various nucleic acid-based materials can be used as sensing probes in electrochemical biosensors. For instance, aptamers are oligonucleotide synthetic short single-stranded DNA or RNA ligands. They showed exceptional binding abilities (via intermolecular forces), outstanding selectivity, and extraordinary sensitivity towards a wide and diverse range of targets such as metabolites, proteins, and whole cells. Numerous aptamer-based electrochemical biosensors have been introduced in recent years (Senel et al., 2019; Zhang et al., 2023a). In this regard, Liangliang Bi et al has detected HER2 in the serum of a BC patient via electrochemical impedance spectroscopy (EIS) by modifying the GCE of an aptasensor with a nanocomposite made of carbon nanotubes decorated with palladium nanoparticles to enhance the conductivity and the attachment of the aptamer (Bi et al., 2023). The sensor showed simplicity, admissible specificity, good stability, and an acceptable LOD of 8.0 pg/mL. Moreover, it exhibited a linearity range of 0.03 ng/mL to 9.0 ng/mL. Moreover, Ya-Zhang et al fabricated an outstanding aptasensor by incorporating a nanocomposite into a screen-printed carbon electrode (Zhang et al., 2023b). The nanocomposite consisted of a metal organic framework, zeolitic imidazolate framework-67 (ZIF-67), and antimonene (AMNF). The presence of antimonene not only provides a large surface area, increases stability, and enhances biocompatibility, but also helps to strongly attach the single-stranded aptamer embedding Cd+2 as signal labels to the working electrode. The aptasensor demonstrates a good LOD of about 5.0 fg/mL and a detection range of 0–1,000 pg/mL. Furthermore, Ya-Zhang et al presented another electrochemical aptasensor for the detection of HER2 (Zhang et al., 2023c). They incorporated ZIF-67, polydopamine (PDA), and HER2 aptamer (ZIF-67-PDA + Apt) to provide high electrochemical redox activity and a large surface area. In addition, another nanocomposite used as signal labeling was made of Cu/UiO-66 metal organic framework, HER2 aptamer, and 3, 3′, 5, 5′-tetramethylbenzidine (TMB). Once the ZIF-67-PDA + Apt probe captured HER2, the conductivity of the electrode was weakened, followed by the addition of Cu/UiO-66+Apt, resulting in an enhancement of the electrochemical signal. The sensor showed a LOD of 45 fg/mL and a detection range of 0.75–40 pg/mL.
Another example of nucleic acid-based nanomaterials used as the sensitive probe of the working electrode is single-stranded DNA (ssDNA). Sara Ranjbari et al has conducted a good modification to the glassy carbon electrode with a nanocomposite consisting of hierarchical flower-like gold and MXene nanoparticles to elevate the conductivity of the sensor to detect miRNA-122 in serum samples of 20 BC patients (Ranjbari et al., 2023). Moreover, the nanocomposite aided in the attachment of the antisense ssDNA. The resultant electrochemical biosensor achieved a limit of detection of 11.4 fg/mL, exceeding the LOD of the traditional methods of detection, and a linear range of 32.6 fzg/mL to 32.6 ng/mL. Furthermore, it showed superior selectivity, sensitivity, and reproducibility and maintained excellent stability for 32 days. Another form of ssDNA used as a probe on the working electrode surface is complementary DNA. Sinan Fu et al has modified a GCE nanocomposite of a creative composition to enhance the electrode properties to detect miRNA-21 in the serum of BC patients (Fu et al., 2023). The nanocomposite consisted of tungsten disulfide, Co9S8, double-walled carbon nanotubes, and silver nanoparticles, Co9S8@WS2/DWCNT-AgNPs. The use of this newly developed nanocomposite led to a string enhancement in the conductivity, intensified the electrochemical signal, and offered expandable binding sites for larger attachment of the cDNA probe through Ag-S bonding. The sensor achieved sensitivity, selectivity, and reproducibility with a good LOD of 5.5 ag/mL and a concentration range of 3.6 ag/mL to 36.07 pg/mL.
In the detection of BC biomarkers, miRNA is not only used as a biomarker but also as a sensing probe on the working electrode of the electrochemical biosensor. Sadrabadi et al enhanced the properties of a bare carbon paste electrode by incorporating a nanocomposite on its surface in the presence of an electro-active label to detect microRNA-155 in the samples of BC patients (Sadrabadi et al., 2024). The nanocomposite was carbon nanofibers, a metal organic framework based on copper (CuMOF), and iron-modified graphene oxide nano-sheets (Fe@rGO). The use of this nanocomposite enhanced the electron transfer, provided binding sites for the attachment of the probe miRNA, and facilitated the fixation of the probe on the electrode surface. The sensor offered good selectivity, sensitivity, and reproducibility; moreover, it exhibited a low LOD of 31.6 fg/mL and a wide range of 0.79 fg/mL-80 pg/mL.
Although the use of DNA probes in electrochemical biosensors is impressively beneficial, they suffer from some drawbacks. They suffer from surface perturbations, inhibiting the attachment of the target molecules to the probe. In addition, non-specific interactions occur to reduce detection sensitivity and produce several background signals. Moreover, the complexity of the process of synthesizing these probes hinders their use in clinical diagnosis. Therefore, numerous research projects have been conducted using different alternatives to DNA probes with similar properties (Yu et al., 2024).
Peptide-based nucleic acid (PNA) represents a promising alternative to DNA probes, overcoming their obstacles and enhancing the process of diagnosing breast cancer. PNAs are similar to nucleotides in structure except for the presence of N-(2-aminoethyl)-glycine joined via a peptide bond instead of the negatively charged phosphate groups, leading to modifications in the interaction between the PNA and the target nucleic acid. Moreover, PNAs can effectively make duplexes at ionic strengths very close to physiological conditions (Lavecchia di Tocco et al., 2024). Various articles using PNAs as probes on the working electrodes of electrochemical biosensors for BC have been released. R. Liu et al improved the efficiency of glassy carbon electrode using a magnetic nanocomposite to detect the TP53 gene in the samples of BC patients using PNA as a probe (Zhang et al., 2024). The nanocomposite was made of Fe3O4/α-Fe2O3 and gold nanoparticles to increase the sensitivity of the electrochemical sensor, improve the conductivity, and to intensify the electrochemical signals. Due to the high specificity of the PNA probe and its high affinity towards the target DNA molecules, the sensor managed to detect the single-base mismatched DNA without any further DNA amplification steps. The electrochemical detection of the target TP53 on the surface of MGCE was achieved by applying a magnetic field after the self-hybridization between the PNA and the target DNA, leading to different and several rates of electron transfer resulting in current changes. Then, the previous process was followed by the removal of that field, leading to rapid regeneration of the modified electrode. The system achieved a remarkable LOD of 0.56 pg/mL and a broad linear range from 2.2 pg/mL to 2.2 μg/mL.
Mixed method-based electrochemical biosensors
Through the previous detection probes showed promising results and the ability to replace conventional methods, several modifications were conducted to achieve better results. For instance, adding catalytic enzymes to the detection method as a common label attracts the attention of many scientists. The enzymes convert definite substrates into active redox signals under certain conditions to better enhance the sensitivity and selectivity of the electrochemical biosensors. Therefore, Y. Zhang et al used the previous approach to develop electrochemical biosensor to detect the soluble programmed death ligand 1PD-L1 in the samples of BC patients (Zhang et al., 2023d). A glassy carbon electrode modified with polyethyleneimine (PEI), gold nanoparticles, and multi-walled carbon nanotubes (MWCNTs) nanocomposites was exploited to enhance the electrochemical signal, electrode surface conductivity and improve the attachment of the programmed death ligand 1PD-L1 aptamer as a sensing probe. Moreover, they added covalent organic frameworks, gold nanoparticles, antibodies, and horseradish peroxidase (COFs-AuNPs-Ab-HRP) to their electrochemical sensors to enhance the sensing performance through facilitating the catalytic oxidation in the presence of hydroquinone (HQ) and H2O2. Once the sensor encounters sPD-L1, hydrogen peroxide converts HRP to its oxidized form, leading to the oxidation of HQ to benzoquinone (BQ), to amplify the electrochemical readouts. The sensor achieved a remarkable LOD of 0.143 pg/mL and was effectively implemented in clinical and cellular samples, achieving great results in comparison with the ELISA kit. Furthermore, the sensor showed high selectivity and sensitivity, giving the chance for early detection of sPD-L1 in BC serum.
Employing aptamers with electrochemical immunosensors in the presence of enzymes is also a promising approach to enhancing the properties of the electrochemical sensor. Another research group has modified the glassy carbon electrode with SWCNTs to enhance the detection efficiency of the HER2 in the serum of BC patients. They utilized the SWNTs to facilitate the attachment of the anti-HER2 antibody, increase the binding sites of the antibodies, and improve the conductivity and electrochemical signal. Once the anti-HER2 antibody bound to the target HER2, biotin-labeled HER2-specific aptamers were used to bind to the target HER2, followed by the catalytic activity of the streptavidin-labeled alkaline phosphatase (AP) via binding to biotin, generating the electrochemical signal. Accordingly, a remarkable LOD of 0.23 pg/mL and a linear range from 1.0 pg/mL to 100 ng/mL were obtained with an acceptable recovery rate in the analysis of serum samples.
Additionally, Chihong Ma et al benefited from clustered regularly interspaced short palindromic repeats (CRISPR) to develop an outstanding electrochemical biosensor to detect miR-21 in BC patients (Ma et al., 2024). They merged the advantages of primer exchange reaction (PER) and CRISPR/Cas13a for the detection of the target biomarker. The detection process was conducted as follows: a sample containing the target biomarker was mixed with Cas13a/crRNA, leading to the recognition of the target miR-21. Consequently, it induces the trans-cleavage activity of CRISPR/Cas13a and breaks the target ribonucleotide site in hairpin 1 (HP1), resulting in the unfolding of HP1. Hence, the previous mixture is subjected to the surface of the gold electrode modified with Hairpin 2 (HP2), resulting in hybridization between the exposed segments of HP1 and HP2 and establishing binding sites for PER concatemers. Therefore, PER concatemers bind to these binding sites, followed by the attachment of multiple short single-stranded DNAs labeled with methylene blue (ssDNA-MB), leading to the electrochemically amplified signal. The sensors showed good reproducibility, with a LOD of 0.11 pg/mL, a linear range from 0.36 pg/mL to 0.36 μg/mL, and a practical performance in plasma samples. Table 2 is presented here to collect the most relative nanocomposite that have been developed and applied for electrochemical sensing of breast cancer biomarkers.
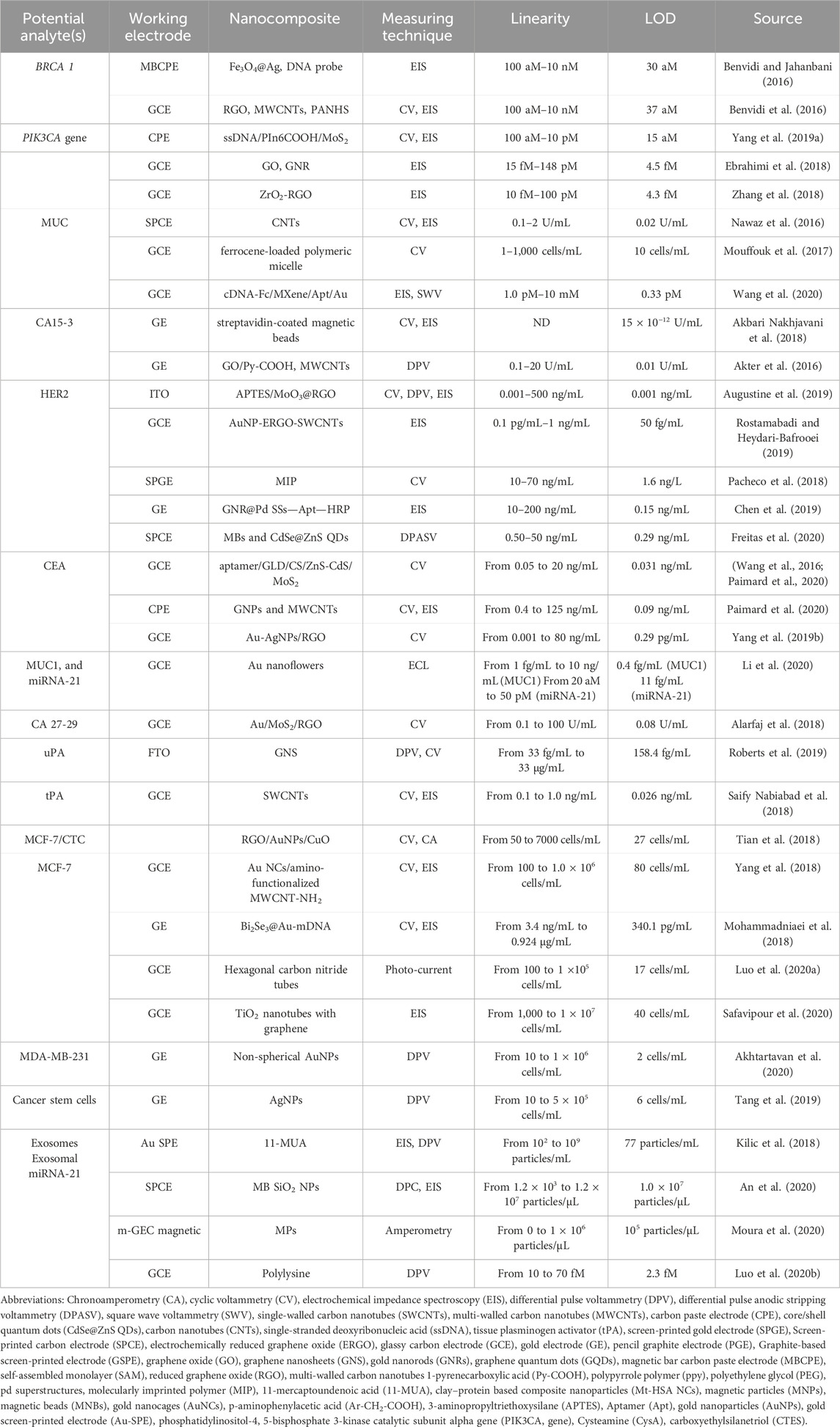
Table 2. A short list of reported electrochemical bio-sensing approaches for the BC diagnosis using developed nanocomposites (Gajdosova et al., 2020).
Conclusion
Ultimately, later diagnosis of breast cancer results in lower rates of human survival than early diagnosis, which can be achieved by detecting specific biomarkers associated with the breast cancer disease. Detecting those targeting multiple biomarkers can be done using several techniques such as PCR, ELISA, MRI, mammography, and other techniques. Despite tendering promising results, those diagnostic approaches are hindered by several drawbacks including long time of analysis, expert data acquisition, expensiveness, experienced supervision, and need of multiplexing capacity. Consequently, biosensor-based techniques for cancer detection gained considerable attention due to their portability, disposability and onsite analysis. In addition to other advantageous aspects such as low cost, noninvasive nature, and their multiplexing monitoring (i.e., the ability to determine the concentration of several targets simultaneously in a single drop of patient sample).
A new generation of electrochemical biosensors was released due to the involvement of nanostructured materials in the fabrication, functionalization, and modification of the sensor surfaces. Nanomaterials were incorporated into these sensors to achieve superior sensitivity and reliability. The use of nanostructured materials is necessary to support the effective immobilization of bio-receptors, to enhance the orientation and direction of those immobilized molecules and to increase their sensing lifetime. Another important feature, nanomaterials are implemented in the bio-sensing system to accelerate the charge/electron transfer due to their acquired electro-catalytic functions. Eventually, the incorporation of nanocomposites will afford high sensitivity, and high selectivity. Since there is always room for innovation, and optimization, more research studies will be sponsored to resolve some challenges regarding the stability and cross-reactivity.
Finding new potential biomarkers with higher stability and specificity is highly needed to resolve the technical limitations of the described biomarkers. Additionally, due to the presence of more than one type of biomarker in BC cancer, platforms capable of detecting multiple biomarkers simultaneously in one clinical sample are urgently needed in order to achieve high accuracy. Frequent biosensing measurements and continuous monitoring will generate vast amounts of results and confusing data. Analyzing this data manually is time-consuming and prone to errors. Machine learning (ML) and artificial intelligence (AI) algorithms can process large datasets efficiently, identify patterns, and extract relevant information. Hence, faster and more accurate data analysis will be obtained, leading to timely interventions and improved cancer diagnosis outcomes. Therefore, computational models, ML, and AI should be involved to support the large-scale production, commercialization and global marketing of biosensors in biomedical diagnosis and achieve remarkable simplicity and accuracy.
Author contributions
OG: Writing–original draft. ME: Writing–original draft, Writing–review and editing. AR: Writing–original draft, Writing–review and editing. RH: Writing–original draft, Writing–review and editing, Conceptualization, Funding acquisition, Project administration, Supervision.
Funding
The author(s) declare financial support was received for the research, authorship, and/or publication of this article. This work is funded by the Science, Technology and Innovation Funding Authority (STDF). The grant ID number is (STDF: 46218).
Acknowledgments
Authors acknowledge the research fund received from the Science, Technology and Innovation Funding Authority (STDF, Cairo, Egypt) to support the research project (Multiplex Nano-biosensor for the rapid breast cancer biomarkers detection and anti-cancer agents screening. Project ID: 46218, Applied Science, Call 1).
Conflict of interest
The authors declare that the research was conducted in the absence of any commercial or financial relationships that could be construed as a potential conflict of interest.
Publisher’s note
All claims expressed in this article are solely those of the authors and do not necessarily represent those of their affiliated organizations, or those of the publisher, the editors and the reviewers. Any product that may be evaluated in this article, or claim that may be made by its manufacturer, is not guaranteed or endorsed by the publisher.
References
Ahirwar, R. (2021). Recent advances in nanomaterials-based electrochemical immunosensors and aptasensors for HER2 assessment in breast cancer. Mikrochim. Acta 188 (10), 317. doi:10.1007/s00604-021-04963-2
Akbari Nakhjavani, S., Khalilzadeh, B., Samadi Pakchin, P., Saber, R., Ghahremani, M. H., and Omidi, Y. (2018). A highly sensitive and reliable detection of CA15-3 in patient plasma with electrochemical biosensor labeled with magnetic beads. Biosens. Bioelectron. 122, 8–15. doi:10.1016/j.bios.2018.08.047
Akhtartavan, S., Karimi, M., Sattarahmady, N., and Heli, H. (2020). An electrochemical signal-on apta-cyto-sensor for quantitation of circulating human MDA-MB-231 breast cancer cells by transduction of electro-deposited non-spherical nanoparticles of gold. J. Pharm. Biomed. Analysis 178, 112948. doi:10.1016/j.jpba.2019.112948
Akter, R., Jeong, B., Choi, J. S., and Rahman, M. (2016). Ultrasensitive Nanoimmunosensor by coupling non-covalent functionalized graphene oxide platform and numerous ferritin labels on carbon nanotubes. Biosens. Bioelectron. 80, 123–130. doi:10.1016/j.bios.2016.01.035
Alarfaj, N. A., El-Tohamy, M. F., and Oraby, H. (2018). New label-free ultrasensitive electrochemical immunosensor-based Au/MoS2/rGO nanocomposites for CA 27-29 breast cancer antigen detection. New J. Chem. 42 (13), 11046–11053. doi:10.1039/c8nj01388h
An, Y., Li, R., Zhang, F., and He, P. (2020). Magneto-mediated electrochemical sensor for simultaneous analysis of breast cancer exosomal proteins. Anal. Chem. 92 (7), 5404–5410. doi:10.1021/acs.analchem.0c00106
Anusha, T., Bhavani, K. S., Hassan, R. Y., and Brahman, P. K. (2023). Ferrocene tagged primary antibody generates electrochemical signal: an electrochemical immunosensing platform for the monitoring of vitamin D deficiency in clinical samples. Int. J. Biol. Macromol. 239, 124269. doi:10.1016/j.ijbiomac.2023.124269
Arafa, K. K., Ibrahim, A., Mergawy, R., El-Sherbiny, I. M., Febbraio, F., and Hassan, R. Y. A. (2022). Advances in cancer diagnosis: bio-electrochemical and biophysical characterizations of cancer cells. Micromachines 13, 1401. doi:10.3390/mi13091401
Arshad, R., Kiani, M. H., Rahdar, A., Sargazi, S., Barani, M., Shojaei, S., et al. (2022). Nano-based theranostic platforms for breast cancer: a review of latest advancements. Bioeng. (Basel) 9 (7), 320. doi:10.3390/bioengineering9070320
Augustine, S., Kumar, P., and Malhotra, B. D. (2019). Amine-functionalized MoO3@RGO nanohybrid-based biosensor for breast cancer detection. ACS Appl. Bio Mater. 2 (12), 5366–5378. doi:10.1021/acsabm.9b00659
Azevedo, R., Soares, J., Peixoto, A., Cotton, S., Lima, L., Santos, L. L., et al. (2018). Circulating tumor cells in bladder cancer: emerging technologies and clinical implications foreseeing precision oncology. Urol. Oncol. 36 (5), 221–236. doi:10.1016/j.urolonc.2018.02.004
Barhoum, A., Altintas, Z., Devi, K. S., and Forster, R. J. (2023). Electrochemiluminescence biosensors for detection of cancer biomarkers in biofluids: principles, opportunities, and challenges. Nano Today 50, 101874. doi:10.1016/j.nantod.2023.101874
Barzaman, K., Karami, J., Zarei, Z., Hosseinzadeh, A., Kazemi, M. H., Moradi-Kalbolandi, S., et al. (2020). Breast cancer: biology, biomarkers, and treatments. Int. Immunopharmacol. 84, 106535. doi:10.1016/j.intimp.2020.106535
Benvidi, A., and Jahanbani, S. (2016). Self-assembled monolayer of SH-DNA strand on a magnetic bar carbon paste electrode modified with Fe3O4@Ag nanoparticles for detection of breast cancer mutation. J. Electroanal. Chem. 768, 47–54. doi:10.1016/j.jelechem.2016.02.038
Benvidi, A., Tezerjani, M. D., Jahanbani, S., Mazloum Ardakani, M., and Moshtaghioun, S. M. (2016). Comparison of impedimetric detection of DNA hybridization on the various biosensors based on modified glassy carbon electrodes with PANHS and nanomaterials of RGO and MWCNTs. Talanta 147, 621–627. doi:10.1016/j.talanta.2015.10.043
Bi, L., Teng, Y., Baghayeri, M., and Bao, J. (2023). Employing Pd nanoparticles decorated on halloysite nanotube/carbon composite for electrochemical aptasensing of HER2 in breast cancer patients. Environ. Res. 237, 117030. doi:10.1016/j.envres.2023.117030
Bonacho, T., Rodrigues, F., and Liberal, J. (2020). Immunohistochemistry for diagnosis and prognosis of breast cancer: a review. Biotech. Histochem. 95 (2), 71–91. doi:10.1080/10520295.2019.1651901
Brzozowski, J. S., Bond, D. R., Jankowski, H., Goldie, B. J., Burchell, R., Naudin, C., et al. (2018). Extracellular vesicles with altered tetraspanin CD9 and CD151 levels confer increased prostate cell motility and invasion. Sci. Rep. 8 (1), 8822. doi:10.1038/s41598-018-27180-z
Cao, Y., Wang, J., Lin, C., Geng, Y., Ma, C., Zhu, J., et al. (2023). Zwitterionic electrochemiluminescence biointerface contributes to label-free monitoring of exosomes dynamics in a fluidic microreaction device. Adv. Funct. Mater. 33 (18), 2214294. doi:10.1002/adfm.202214294
Cao, Y., Zhou, J. L., Ma, Y., Zhou, Y., and Zhu, J. J. (2022). Recent progress of metal nanoclusters in electrochemiluminescence. Dalton Trans. 51 (23), 8927–8937. doi:10.1039/d2dt00810f
Chen, D., Wang, D., Hu, X., Long, G., Zhang, Y., and Zhou, L. (2019). A DNA nanostructured biosensor for electrochemical analysis of HER2 using bioconjugate of GNR@Pd SSs—apt—HRP. Sensors Actuators B Chem. 296, 126650. doi:10.1016/j.snb.2019.126650
Choudhery, S., Anderson, T., and Valencia, E. (2021). Digital breast tomosynthesis (DBT)-guided biopsy of calcifications: pearls and pitfalls. Clin. Imaging 72, 83–90. doi:10.1016/j.clinimag.2020.11.035
Di Corato, R., Gazeau, F., Le Visage, C., Fayol, D., Levitz, P., Lux, F., et al. (2013). High-resolution cellular MRI: gadolinium and iron oxide nanoparticles for in-depth dual-cell imaging of engineered tissue constructs. ACS Nano 7 (9), 7500–7512. doi:10.1021/nn401095p
Di Maria, S., Vedantham, S., and Vaz, P. (2022). X-ray dosimetry in breast cancer screening: 2D and 3D mammography. Eur. J. Radiol. 151, 110278. doi:10.1016/j.ejrad.2022.110278
Duraia, E.-s.M., Adebiyi, B. M., Das, S., Magar, H. S., Beall, G. W., and Hassan, R. Y. (2024). Single-step synthesis of carbon nanotubes-nickel cobaltite (CNT-NiCo2O4) by thermal decomposition of cyanide compounds for electrochemical sensing applications. Phys. E Low-dimensional Syst. Nanostructures 159, 115902. doi:10.1016/j.physe.2024.115902
Ebrahimi, A., Nikokar, I., Zokaei, M., and Bozorgzadeh, E. (2018). Design, development and evaluation of microRNA-199a-5p detecting electrochemical nanobiosensor with diagnostic application in Triple Negative Breast Cancer. Talanta 189, 592–598. doi:10.1016/j.talanta.2018.07.016
Fathi Kazerouni, M., Shirzad, H., Firoozbakhtian, A., Hosseini, M., Rabbani, H., Amin Mousavinezhad, S., et al. (2024). A Ti3C2 modified single electrode electrochemical system for the gold nanoprism-enhanced ECL detection of HER2. J. Electroanal. Chem. 956, 118103. doi:10.1016/j.jelechem.2024.118103
Fiorica, J. V. (2016). Breast cancer screening, mammography, and other modalities. Clin. Obstet. Gynecol. 59 (4), 688–709. doi:10.1097/grf.0000000000000246
Fox, S., Speirs, V., and Shaaban, A. M. (2022). Male breast cancer: an update. Virchows Arch. 480 (1), 85–93. doi:10.1007/s00428-021-03190-7
Freitas, A. J. A., Causin, R. L., Varuzza, M. B., Calfa, S., Hidalgo Filho, C. M. T., Komoto, T. T., et al. (2022). Liquid biopsy as a tool for the diagnosis, treatment, and monitoring of breast cancer. Int. J. Mol. Sci. 23 (17), 9952. doi:10.3390/ijms23179952
Freitas, M., Nouws, H. P. A., Keating, E., Fernandes, V. C., and Delerue-Matos, C. (2020). Immunomagnetic bead-based bioassay for the voltammetric analysis of the breast cancer biomarker HER2-ECD and tumour cells using quantum dots as detection labels. Microchim. Acta 187 (3), 184. doi:10.1007/s00604-020-4156-4
Fu, S., Ning, Z., Li, Q., He, Y., Xie, C., Cheng, J., et al. (2023). Sulfur source-mediated in situ growth of highly conductive nanocomposites for sensitive detection of miRNA-21. Sensors Actuators B Chem. 392, 134086. doi:10.1016/j.snb.2023.134086
Gajdosova, V., Lorencova, L., Kasak, P., and Tkac, J. (2020). Electrochemical nanobiosensors for detection of breast cancer biomarkers. Sensors (Basel) 20 (14), 4022. doi:10.3390/s20144022
Geras, K. J., Mann, R. M., and Moy, L. (2019). Artificial intelligence for mammography and digital breast tomosynthesis: current concepts and future perspectives. Radiology 293 (2), 246–259. doi:10.1148/radiol.2019182627
Guo, R., Lu, G., Qin, B., and Fei, B. (2018). Ultrasound imaging technologies for breast cancer detection and management: a review. Ultrasound Med. Biol. 44 (1), 37–70. doi:10.1016/j.ultrasmedbio.2017.09.012
Hannafon, B. N., Trigoso, Y. D., Calloway, C. L., Zhao, Y. D., Lum, D. H., Welm, A. L., et al. (2016). Plasma exosome microRNAs are indicative of breast cancer. Breast Cancer Res. 18 (1), 90. doi:10.1186/s13058-016-0753-x
Hassan, R. Y. A. (2022). Advances in electrochemical nano-biosensors for biomedical and environmental applications: from current work to future perspectives. Sensors 22, 7539. doi:10.3390/s22197539
Hussein, H. A., Hassan, R. Y. A., Chino, M., and Febbraio, F. (2020). Point-of-Care diagnostics of COVID-19: from current work to future perspectives. Sensors 20, 4289. doi:10.3390/s20154289
Hussein, H. A., Kandeil, A., Gomaa, M., and Hassan, R. Y. A. (2023). Double-antibody-based nano-biosensing system for the onsite monitoring of SARS-CoV-2 variants. Microsystems Nanoeng. 9 (1), 105. doi:10.1038/s41378-023-00578-0
Jing, L., Xie, C., Li, Q., Yang, M., Li, S., Li, H., et al. (2022). Electrochemical biosensors for the analysis of breast cancer biomarkers: from design to application. Anal. Chem. 94 (1), 269–296. doi:10.1021/acs.analchem.1c04475
Kalinyak, J. E., Schilling, K., Berg, W. A., Narayanan, D., Mayberry, J. P., Rai, R., et al. (2011). PET-guided breast biopsy. Breast J. 17 (2), 143–151. doi:10.1111/j.1524-4741.2010.01044.x
Kashyap, D., Pal, D., Sharma, R., Garg, V. K., Goel, N., Koundal, D., et al. (2022). Global increase in breast cancer incidence: risk factors and preventive measures. Biomed. Res. Int. 2022, 1–16. doi:10.1155/2022/9605439
Kazi, M., Suhani, , Parshad, R., Seenu, V., Mathur, S., and Haresh, K. P. (2017). Fine-needle aspiration cytology (FNAC) in breast cancer: a reappraisal based on retrospective review of 698 cases. World J. Surg. 41 (6), 1528–1533. doi:10.1007/s00268-017-3906-x
Kilic, T., Valinhas, A. T. D. S., Wall, I., Renaud, P., and Carrara, S. (2018). Label-free detection of hypoxia-induced extracellular vesicle secretion from MCF-7 cells. Sci. Rep. 8 (1), 9402. doi:10.1038/s41598-018-27203-9
Kim, H., Choi, J. S., Kim, K., Ko, E. S., Ko, E. Y., and Han, B. K. (2023). Effect of artificial intelligence-based computer-aided diagnosis on the screening outcomes of digital mammography: a matched cohort study. Eur. Radiol. 33 (10), 7186–7198. doi:10.1007/s00330-023-09692-z
Krishnamurti, U., and Silverman, J. F. (2014). HER2 in breast cancer: a review and update. Adv. Anat. Pathol. 21 (2), 100–107. doi:10.1097/pap.0000000000000015
Kumar, N., Sadique, M. A., Khan, R., Gowri, V. S., Kumar, S., Ashiq, M., et al. (2023). Immunosensor for breast cancer CD44 biomarker detection based on exfoliated graphene quantum dots integrated gold nanoparticles. Hybrid. Adv. 3, 100065. doi:10.1016/j.hybadv.2023.100065
Lavecchia di Tocco, F., Botti, V., Cannistraro, S., and Bizzarri, A. R. (2024). Detection of miR-155 using peptide nucleic acid at physiological-like conditions by surface plasmon resonance and bio-field effect transistor. Biosensors 14, 79. doi:10.3390/bios14020079
Lewitowicz, P., Wincewicz, A., Horecka-Lewitowicz, A., Matykiewicz, J., Koziel, D., Gluszek, S., et al. (2015). Breast cancer cells are in biopsy channel in postoperative material from mastectomies preformed 3 days after core needle biopsy (CNB) but they do not persist at biopsy channel apart from the tumor after 10 Days from the CNB procedure-a study of seven case. Breast J. 21 (5), 558–561. doi:10.1111/tbj.12460
Li, J., Liu, J., Bi, Y., Sun, M., Bai, J., and Zhou, M. (2020). Ultrasensitive electrochemiluminescence biosensing platform for miRNA-21 and MUC1 detection based on dual catalytic hairpin assembly. Anal. Chim. Acta 1105, 87–94. doi:10.1016/j.aca.2020.01.034
Lianidou, E. S., and Markou, A. (2011). Circulating tumor cells in breast cancer: detection systems, molecular characterization, and future challenges. Clin. Chem. 57 (9), 1242–1255. doi:10.1373/clinchem.2011.165068
Lowry, K. P., Bissell, M. C. S., Miglioretti, D. L., Kerlikowske, K., Alsheik, N., Macarol, T., et al. (2022). Breast biopsy recommendations and breast cancers diagnosed during the COVID-19 pandemic. Radiology 303 (2), 287–294. doi:10.1148/radiol.2021211808
Luo, J., Liang, D., Li, X., Deng, L., Wang, Z., and Yang, M. (2020a). Aptamer-based photoelectrochemical assay for the determination of MCF-7. Microchim. Acta 187 (5), 257. doi:10.1007/s00604-020-04239-1
Luo, L., Wang, L., Zeng, L., Wang, Y., Weng, Y., Liao, Y., et al. (2020b). A ratiometric electrochemical DNA biosensor for detection of exosomal MicroRNA. Talanta 207, 120298. doi:10.1016/j.talanta.2019.120298
Ma, C., Zhou, Q., Shi, J., Gao, H., Huang, D., Xue, H., et al. (2024). CRISPR-empowered electrochemical biosensor for target amplification-free and sensitive detection of miRNA. Talanta 266, 125125. doi:10.1016/j.talanta.2023.125125
Magar, H. S., and Hassan, R. Y. A. (2021). Mulchandani electrochemical impedance spectroscopy (EIS): principles, construction, and biosensing applications. Sensors 21, 6578. doi:10.3390/s21196578
Magnuska, Z. A., Theek, B., Darguzyte, M., Palmowski, M., Stickeler, E., Schulz, V., et al. (2022). Influence of the computer-aided decision support system design on ultrasound-based breast cancer classification. Cancers (Basel) 14 (2), 277. doi:10.3390/cancers14020277
Magny, S. J., Shikhman, R., and Keppke, A. L. (2024). “Breast imaging reporting and data system,” in StatPearls (Treasure Island: StatPearls Publishing Copyright © 2024).
Marchiò, C., Annaratone, L., Marques, A., Casorzo, L., Berrino, E., and Sapino, A. (2021). Evolving concepts in HER2 evaluation in breast cancer: heterogeneity, HER2-low carcinomas and beyond. Semin. Cancer Biol. 72, 123–135. doi:10.1016/j.semcancer.2020.02.016
Medical Advisory Secretariat (2010). Cancer screening with digital mammography for women at average risk for breast cancer, magnetic resonance imaging (MRI) for women at high risk: an evidence-based analysis. Ont. Health Technol. Assess. Ser. 10 (3), 1–55.
Mohammadniaei, M., Yoon, J., Lee, T., Bharate, B. G., Jo, J., Lee, D., et al. (2018). Electrochemical biosensor composed of silver ion-mediated dsDNA on Au-encapsulated Bi2Se3 nanoparticles for the detection of H2O2 released from breast cancer cells. Small 14 (16), 1703970. doi:10.1002/smll.201703970
Mohan, S., Patel, S., Greenstein, I., Ng, C., Frazier, K., Nguyen, G., et al. (2018). Metabolic relevance for N-hydroxy L-arginine reduction in estrogen-negative breast cancer cells. Amino Acids 50 (11), 1629–1636. doi:10.1007/s00726-018-2603-x
Mouffouk, F., Aouabdi, S., Al-Hetlani, E., Serrai, H., Alrefae, T., and Leo Chen, L. (2017). New generation of electrochemical immunoassay based on polymeric nanoparticles for early detection of breast cancer. Int. J. Nanomedicine 12, 3037–3047. doi:10.2147/ijn.s127086
Moura, S. L., Martín, C. G., Martí, M., and Pividori, M. I. (2020). Electrochemical immunosensing of nanovesicles as biomarkers for breast cancer. Biosens. Bioelectron. 150, 111882. doi:10.1016/j.bios.2019.111882
Najjar, S., and Allison, K. H. (2022). Updates on breast biomarkers. Virchows Arch. 480 (1), 163–176. doi:10.1007/s00428-022-03267-x
Nawaz, M. A., Rauf, S., Catanante, G., Nunes, G., and Marty, J. (2016). One step assembly of thin films of carbon nanotubes on screen printed interface for electrochemical aptasensing of breast cancer biomarker. Sensors (Basel) 16 (10), 1651. doi:10.3390/s16101651
Nicolini, A., Ferrari, P., and Duffy, M. J. (2018). Prognostic and predictive biomarkers in breast cancer: past, present and future. Semin. Cancer Biol. 52 (Pt 1), 56–73. doi:10.1016/j.semcancer.2017.08.010
Nounou, M. I., ElAmrawy, F., Ahmed, N., Abdelraouf, K., Goda, S., and Syed-Sha-Qhattal, H. (2015). Breast cancer: conventional diagnosis and treatment modalities and recent patents and technologies. Breast Cancer (Auckl) 9 (Suppl. 2), 17–34. doi:10.4137/BCBCR.S29420
Obeagu, E. I., and Obeagu, G. U. (2024). Breast cancer: a review of risk factors and diagnosis. Med. Baltim. 103 (3), e36905. doi:10.1097/md.0000000000036905
Onishi, N., Sadinski, M., Hughes, M. C., Ko, E. S., Gibbs, P., Gallagher, K. M., et al. (2020). Ultrafast dynamic contrast-enhanced breast MRI may generate prognostic imaging markers of breast cancer. Breast Cancer Res. 22 (1), 58. doi:10.1186/s13058-020-01292-9
Pacheco, J. G., Rebelo, P., Freitas, M., Nouws, H. P., and Delerue-Matos, C. (2018). Breast cancer biomarker (HER2-ECD) detection using a molecularly imprinted electrochemical sensor. Sensors Actuators B Chem. 273, 1008–1014. doi:10.1016/j.snb.2018.06.113
Pacilè, S., Lopez, J., Chone, P., Bertinotti, T., Grouin, J. M., and Fillard, P. (2020). Improving breast cancer detection accuracy of mammography with the concurrent use of an artificial intelligence tool. Radiol. Artif. Intell. 2 (6), e190208. doi:10.1148/ryai.2020190208
Paimard, G., Shahlaei, M., Moradipour, P., Akbari, H., Jafari, M., and Arkan, E. (2020). An Impedimetric Immunosensor modified with electrospun core-shell nanofibers for determination of the carcinoma embryonic antigen. Sensors Actuators B Chem. 311, 127928. doi:10.1016/j.snb.2020.127928
Ranjbari, S., Rezayi, M., Arefinia, R., Aghaee-Bakhtiari, S. H., Hatamluyi, B., and Pasdar, A. (2023). A novel electrochemical biosensor based on signal amplification of Au HFGNs/PnBA-MXene nanocomposite for the detection of miRNA-122 as a biomarker of breast cancer. Talanta 255, 124247. doi:10.1016/j.talanta.2022.124247
Roberts, A., Tripathi, P. P., and Gandhi, S. (2019). Graphene nanosheets as an electric mediator for ultrafast sensing of urokinase plasminogen activator receptor-A biomarker of cancer. Biosens. Bioelectron. 141, 111398. doi:10.1016/j.bios.2019.111398
Rostamabadi, P. F., and Heydari-Bafrooei, E. (2019). Impedimetric aptasensing of the breast cancer biomarker HER2 using a glassy carbon electrode modified with gold nanoparticles in a composite consisting of electrochemically reduced graphene oxide and single-walled carbon nanotubes. Microchim. Acta 186 (8), 495. doi:10.1007/s00604-019-3619-y
Sadrabadi, E. A., Benvidi, A., Azimzadeh, M., Asgharnejad, L., Dezfuli, A. S., and Khashayar, P. (2024). Novel electrochemical biosensor for breast cancer detection, based on a nanocomposite of carbon nanofiber, metal–organic framework, and magnetic graphene oxide. Bioelectrochemistry 155, 108558. doi:10.1016/j.bioelechem.2023.108558
Safavipour, M., Kharaziha, M., Amjadi, E., Karimzadeh, F., and Allafchian, A. (2020). TiO2 nanotubes/reduced GO nanoparticles for sensitive detection of breast cancer cells and photothermal performance. Talanta 208, 120369. doi:10.1016/j.talanta.2019.120369
Saify Nabiabad, H., Piri, K., Kafrashi, F., Afkhami, A., and Madrakian, T. (2018). Fabrication of an immunosensor for early and ultrasensitive determination of human tissue plasminogen activator (tPA) in myocardial infraction and breast cancer patients. Anal. Bioanal. Chem. 410 (16), 3683–3691. doi:10.1007/s00216-018-1005-y
Sakthivel, R., Lin, Y. C., Yu, M. C., Dhawan, U., Liu, X., Chen, J. C., et al. (2024). A sensitive sandwich-type electrochemical immunosensor using nitrogen-doped graphene/metal-organic framework-derived CuMnCoOx and Au/MXene for the detection of breast cancer biomarker. Colloids Surfaces B Biointerfaces 234, 113755. doi:10.1016/j.colsurfb.2024.113755
Schillaci, O., Spanu, A., Danieli, R., and Madeddu, G. (2013). Molecular breast imaging with gamma emitters. Q. J. Nucl. Med. Mol. Imaging 57 (4), 340–351.
Seale, K. N., and Tkaczuk, K. H. R. (2022). Circulating biomarkers in breast cancer. Clin. Breast Cancer 22 (3), e319–e331. doi:10.1016/j.clbc.2021.09.006
Sechopoulos, I., Teuwen, J., and Mann, R. (2021). Artificial intelligence for breast cancer detection in mammography and digital breast tomosynthesis: state of the art. Semin. Cancer Biol. 72, 214–225. doi:10.1016/j.semcancer.2020.06.002
Senel, M., Dervisevic, M., and Kokkokoğlu, F. (2019). Electrochemical DNA biosensors for label-free breast cancer gene marker detection. Anal. Bioanal. Chem. 411 (13), 2925–2935. doi:10.1007/s00216-019-01739-9
Smetherman, D. H. (2013). Screening, imaging, and image-guided biopsy techniques for breast cancer. Surg. Clin. North Am. 93 (2), 309–327. doi:10.1016/j.suc.2013.01.004
Song, F. X., Xu, X., Ding, H., Yu, L., Huang, H., Hao, J., et al. (2023). Recent progress in nanomaterial-based biosensors and theranostic nanomedicine for bladder cancer. Biosens. (Basel) 13 (1), 106. doi:10.3390/bios13010106
Song, S. Y., Park, B., Hong, S., Kim, M. J., Lee, E. H., and Jun, J. K. (2019). Comparison of digital and screen-film mammography for breast-cancer screening: a systematic review and meta-analysis. J. Breast Cancer 22 (2), 311–325. doi:10.4048/jbc.2019.22.e24
Talluri, S., and Malla, R. R. (2019). Superparamagnetic iron oxide nanoparticles (SPIONs) for diagnosis and treatment of breast, ovarian and cervical cancers. Curr. Drug Metab. 20 (12), 942–945. doi:10.2174/1389200220666191016124958
Tan, T., Rodriguez-Ruiz, A., Zhang, T., Xu, L., Beets-Tan, R. G. H., Shen, Y., et al. (2023). Multi-modal artificial intelligence for the combination of automated 3D breast ultrasound and mammograms in a population of women with predominantly dense breasts. Insights Imaging 14 (1), 10. doi:10.1186/s13244-022-01352-y
Tang, Y., Dai, Y., Huang, X., Li, L., Han, B., Cao, Y., et al. (2019). Self-assembling peptide-based multifunctional nanofibers for electrochemical identification of breast cancer stem-like cells. Anal. Chem. 91 (12), 7531–7537. doi:10.1021/acs.analchem.8b05359
Tarighati, E., Keivan, H., and Mahani, H. (2023). A review of prognostic and predictive biomarkers in breast cancer. Clin. Exp. Med. 23 (1), 1–16. doi:10.1007/s10238-021-00781-1
Tian, L., Qi, J., Qian, K., Oderinde, O., Liu, Q., Yao, C., et al. (2018). Copper (II) oxide nanozyme based electrochemical cytosensor for high sensitive detection of circulating tumor cells in breast cancer. J. Electroanal. Chem. 812, 1–9. doi:10.1016/j.jelechem.2017.12.012
Vangijzegem, T., Lecomte, V., Ternad, I., Van Leuven, L., Muller, R. N., Stanicki, D., et al. (2023). Superparamagnetic iron oxide nanoparticles (SPION): from fundamentals to state-of-the-art innovative applications for cancer therapy. Pharmaceutics 15 (1), 236. doi:10.3390/pharmaceutics15010236
Vourtsis, A. (2019). Three-dimensional automated breast ultrasound: technical aspects and first results. Diagn Interv. Imaging 100 (10), 579–592. doi:10.1016/j.diii.2019.03.012
Wang, H., Sun, J., Lu, L., Yang, X., Xia, J., Zhang, F., et al. (2020). Competitive electrochemical aptasensor based on a cDNA-ferrocene/MXene probe for detection of breast cancer marker Mucin1. Anal. Chim. Acta 1094, 18–25. doi:10.1016/j.aca.2019.10.003
Wang, Y.-L., Cao, J. T., Chen, Y. H., and Liu, Y. M. (2016). A label-free electrochemiluminescence aptasensor for carcinoembryonic antigen detection based on electrodeposited ZnS–CdS on MoS2 decorated electrode. Anal. Methods 8 (26), 5242–5247. doi:10.1039/c6ay01114d
Wekking, D., Porcu, M., De Silva, P., Saba, L., Scartozzi, M., and Solinas, C. (2023). Breast MRI: clinical indications, recommendations, and future applications in breast cancer diagnosis. Curr. Oncol. Rep. 25 (4), 257–267. doi:10.1007/s11912-023-01372-x
Woolpert, K. M., Ahern, T. P., Lash, T. L., O’Malley, D. L., Stokes, A. M., and Cronin-Fenton, D. P. (2024). Biomarkers predictive of a response to extended endocrine therapy in breast cancer: a systematic review and meta-analysis. Breast Cancer Res. Treat. 203 (3), 407–417. doi:10.1007/s10549-023-07149-x
Yang, J., Yin, X., and Zhang, W. (2019a). Electrochemical determination of PIK3CA gene associated with breast cancer based on molybdenum disulfide nanosheet-supported poly(indole-6-carboxylic acid). Anal. Methods 11 (2), 157–162. doi:10.1039/c8ay02425a
Yang, Y., Fu, Y., Su, H., Mao, L., and Chen, M. (2018). Sensitive detection of MCF-7 human breast cancer cells by using a novel DNA-labeled sandwich electrochemical biosensor. Biosens. Bioelectron. 122, 175–182. doi:10.1016/j.bios.2018.09.062
Yang, Y., Jiang, M., Cao, K., Wu, M., Zhao, C., Li, H., et al. (2019b). An electrochemical immunosensor for CEA detection based on Au-Ag/rGO@PDA nanocomposites as integrated double signal amplification strategy. Microchem. J. 151, 104223. doi:10.1016/j.microc.2019.104223
Yu, X., Ding, S., Zhao, Y., Xu, M., Wu, Z., and Zhao, C. (2024). A highly sensitive and robust electrochemical biosensor for microRNA detection based on PNA-DNA hetero-three-way junction formation and target-recycling catalytic hairpin assembly amplification. Talanta 266, 125020. doi:10.1016/j.talanta.2023.125020
Yuan, S., Xie, G., Yang, X., Chen, Y., and Zhang, H. (2023). Portable paper-based electrochemiluminescence test incorporating lateral-flow immunosensors for detection of interferon-γ levels. Front. Bioeng. Biotechnol. 11, 1131840. doi:10.3389/fbioe.2023.1131840
Zhang, K., Zhang, N., Zhang, L., Wang, H., Shi, H., and Liu, Q. (2018). Label-free impedimetric sensing platform for microRNA-21 based on ZrO2-reduced graphene oxide nanohybrids coupled with catalytic hairpin assembly amplification. RSC Adv. 8 (29), 16146–16151. doi:10.1039/c8ra02453g
Zhang, M., Horvat, J. V., Bernard-Davila, B., Marino, M. A., Leithner, D., Ochoa-Albiztegui, R. E., et al. (2019). Multiparametric MRI model with dynamic contrast-enhanced and diffusion-weighted imaging enables breast cancer diagnosis with high accuracy. J. Magnetic Reson. Imaging 49 (3), 864–874. doi:10.1002/jmri.26285
Zhang, Y., Chen, S., Ma, J., Zhou, X., Sun, X., Jing, H., et al. (2023d). Enzyme-catalyzed electrochemical aptasensor for ultrasensitive detection of soluble PD-L1 in breast cancer based on decorated covalent organic frameworks and carbon nanotubes. Anal. Chim. Acta 1282, 341927. doi:10.1016/j.aca.2023.341927
Zhang, Y., Chen, S., Sun, X., Jing, H., and Zhou, X. (2023a). Electrochemical biosensors for the non-invasive diagnosis of breast cancer. Electrochimica Acta 468, 143190. doi:10.1016/j.electacta.2023.143190
Zhang, Y., Wang, J., Liu, M., Ni, Y., Yue, Y., He, D., et al. (2024). Magnetically induced self-assembly electrochemical biosensor with ultra-low detection limit and extended measuring range for sensitive detection of HER2 protein. Bioelectrochemistry 155, 108592. doi:10.1016/j.bioelechem.2023.108592
Zhang, Y., Xu, Y., Liu, X., Ma, Y., and Huang, Z. (2023b). A novel electrochemical biosensor based on AMNFs@ZIF-67 nano composite material for ultrasensitive detection of HER2. Bioelectrochemistry 150, 108362. doi:10.1016/j.bioelechem.2022.108362
Zhang, Y., Xu, Y., Qi, N., Peng, L., and Yang, M. (2023c). An ultrasensitive dual-signal ratio electrochemical aptamer biosensor for the detection of HER2. Colloids Surfaces B Biointerfaces 222, 113118. doi:10.1016/j.colsurfb.2022.113118
Zhang, Y. N., Xia, K. r., Wei, B. l., and Zhang, B. (2021). Review of breast cancer pathologigcal image processing. Biomed. Res. Int. 2021, 1–7. doi:10.1155/2021/1994764
Keywords: cancer diagnosis, breast cancer biomarkers, electrochemical biosensors, nanocomposites, smart biosensors
Citation: Gamal O, Eldin MH, Refaat AA and Hassan RYA (2024) Advances in nanocomposites-based electrochemical biosensors for the early diagnosis of breast cancer. Front. Sens. 5:1399441. doi: 10.3389/fsens.2024.1399441
Received: 11 March 2024; Accepted: 25 April 2024;
Published: 30 May 2024.
Edited by:
Mohammed M. Rahman, King Abdulaziz University, Saudi ArabiaReviewed by:
Mihaela Tertis, University of Medicine and Pharmacy Iuliu Hatieganu, RomaniaYue Cao, Nanjing University of Posts and Telecommunications, China
Copyright © 2024 Gamal, Eldin, Refaat and Hassan. This is an open-access article distributed under the terms of the Creative Commons Attribution License (CC BY). The use, distribution or reproduction in other forums is permitted, provided the original author(s) and the copyright owner(s) are credited and that the original publication in this journal is cited, in accordance with accepted academic practice. No use, distribution or reproduction is permitted which does not comply with these terms.
*Correspondence: Rabeay Y. A. Hassan, cnlvdW5lc0B6ZXdhaWxjaXR5LmVkdS5lZw==