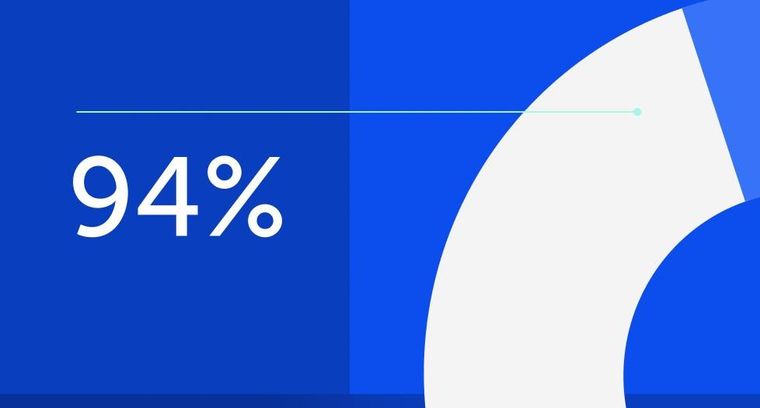
94% of researchers rate our articles as excellent or good
Learn more about the work of our research integrity team to safeguard the quality of each article we publish.
Find out more
PERSPECTIVE article
Front. Sens., 13 October 2023
Sec. Lab-on-a-Chip Devices
Volume 4 - 2023 | https://doi.org/10.3389/fsens.2023.1283402
This article is part of the Research TopicThe Future of Sensing Research: Innovators and Early Career Scientists' PerspectiveView all 6 articles
Over the past few decades, Lab-on-a-chip (LoC) devices have made health diagnostics easier and cheaper by enabling rapid, hassle-free, and inexpensive measurements of different biochemical markers, properties of epidermally retrievable biofluids (e.g., sweat and interstitial fluids), etc. The miniaturization of these sensing technologies along with the integration of flexible frameworks (microfluidic assemblies and electronics) and user-friendly software have paved the way for autonomous and continuous tracking of human health parameters. However, with emerging new technologies, there are new and complex challenges, especially in the case of these miniature LoC devices. Although microfluidics-specific challenges such as analyte contamination, flow rate variability, improving detection specificity, etc. are being worked upon, the bigger question is: how reliable are these wearable devices in the long run? This perspective piece initially talks about the state-of-the-art LoC technologies that have been incorporated into wearable devices. Next, the perspective identifies some of the reliability studies conducted (as well as overlooked) on the integrated wearable electronics used. Finally, the paper discusses the challenges and future directions of research in the field of reliability of LoC-based wearable electronics.
With the emergence of technology, there has been massive emphasis on continuous healthcare monitoring and diagnostics through the use of wearable electronics. One of the earliest endeavors was by NASA (United States), which introduced the first wearable sensors for health monitoring of the astronauts in the 1960s. For public usage, a wireless EKG (Electrocardiogram) was introduced in 1977, while GlucoWatch, for monitoring blood glucose levels, was introduced in 2002 (Lezen et al., 2002). At present, several wearable technologies are commercially available, but these technologies are limited to gravitational, acoustic, electrical impulse, and environment stimuli sensing, i.e., they are primarily used for monitoring body temperature, heart rate, calories burnt, etc. These technologies, however, often fail to provide point-of-care diagnostics and detection of the onset of diseases. To fill this gap, in the past decade, there has been a substantial growth of Lab-on-a-Chip (LoC) devices in the form of wearables, which have brought health monitoring and diagnostics much closer to the consumer. These devices are capable of sensing biofluids (blood, sweat, saliva, mucus, etc.) (Heikenfeld, 2016) and provide immediate information about body temperature (Webb et al., 2013), pH (Curto et al., 2012), hydration (Huang et al., 2013), and other relevant biomarkers (that indicate the possibility of the onset of a disease in the near future) (Kwon et al., 2023). These devices are certainly revolutionizing the modern-day healthcare management and there is a continuous impetus to reduce the cost and increase the longevity of these devices. A critical aspect to meet such cost and longevity goals is to ensure that these devices are robust and reliable and can withstand different environmental stresses. Testing such reliability is critical for all electronic devices; however, there has been very little progress in the reliability analysis of the wearable electronics LoC devices. Therefore, almost no study proposing a new design of a wearable LoC electronic device ever consider studying the reliability of such a device against environmental stresses. This perspective is written in order to highlight the importance of the reliability analysis of such wearable LoC electronic devices.
Recent developments have focused on designing wearable LoC electronics that are capable of employing non-invasive methods for sensing epidermally retrievable biofluids (such as sweat) (Alizadeh et al., 2018; Lin et al., 2020; Vinoth et al., 2021; Zhao et al., 2021; Kunnel and Demuru, 2022; Hu et al., 2023). Considering the variable flow rate of sweat collection at the epidermis, Lin et al. (2020) devised a programmable microfluidic valve that decouples the influence of flow rate variability at the sensor level and leverages valving to provide biofluid routing and compartmentalization at the system level, eventually enabling an integration with consumer electronics for context-based sensor selection. Apart from the detection of basic parameters such as pH and hydration levels in sweat, Vinoth et al. (2021) devised a high throughput sweat sampling device for detecting the concentrations of lactate, Na+, and K+ ions. The sensors were physically separated in the microfluidic network in order to prevent the mixing of the electrode components. In addition, the sensors reported long term stability and reproducibility for sensing in test conditions. Kunnel and Demuru (2022) developed a microfluidic device that uses a potentiometric Cl− and Ca2+ ion sensing module to enable simultaneous extraction, sampling, and storage of sweat and interstitial fluid along with the monitoring of the Ca2+ and Cl− counterions. Another simplistic approach towards measuring sweat rate was studied by Zhao et al. (2020a), wherein the authors used swellable hydrogels in a geometric pattern, and measured the local sweat rate by studying the extent of swelling of the hydrogels using colorimetric analysis. Similar colorimetric analysis was employed by Zhao et al. (2021) for the detection of pH, chloride, and glucose (based on color hues) in generated sweat using a wearable microfluidic thread/fabric-based analytical device (μTFAD) that was mounted on the skin. This device consisted of hydrophilic dot-patterns on a hydrophobic surface (achieved by embroidering thread into fabric) and these hydrophilic threads transported sweat to the thread-like detection zones.
LoC devices are in their nascent stage of development and the research has been focused towards improving the specificity of detection, increasing the types of ions that can be detected, and enabling optimal methods for simultaneous detection of multiple body fluids through the development of complex and stable microfluidic networks. To enable higher control of the microfluidic network and facilitate conversion of the detected data into a form that is not abstruse to the consumer, flexible electronics are crucial in wearable devices. The development of flexible electronics in the form of foldable and stretchable integrated circuits on “wavy” silicon ribbons on silicon rubber was introduced by Kim et al. (2008). Over the years, different prototypes of flexible electronics have been introduced and studied (Ko et al., 2008; Kim et al., 2009; Cheng et al., 2010; Viventi et al., 2010; Trinh and Lee, 2022), with micro-printed electronics on flexible polymer substrates leading the way for integration with microfluidic patches. These polymer-based substrates facilitate higher degree of conformal adhesion to the non-linear skin surface and enable seamless integration of various electronic circuits with microfluidic devices. Although this new frontier in analyzing biomarkers through the highly sophisticated LoC wearable electronic systems is undergoing rapid developments, unfortunately, most of the studies are merely proof-of-concept investigations, with insignificant emphasis on the related reliability analysis that will ensure stable, reproducible, and robust performance data (over a long time duration of use of the devices across widely varying environmental conditions) that will enable a transition of these devices into full commercialization. This article highlights the need for such reliability studies. In Section 4, we discuss some related (and limited) reliability studies performed on these devices. In the following section, we discuss the future directions of research on reliability of wearable electronics LoC devices.
The reliability of a device is crucial to determining its lifetime and its functions under different conditions and is essential for its success in the consumer market. Major reliability issues that can be associated to wearable electronic LoC devices include 1) reduced specificity of detection, 2) unreliable adhesion of LoC devices or sensors to skin, and 3) unstable biochemical measurements.
Detection of the biomolecules (by the LoC devices) depends on the coupling of a biological detection element (such as enzyme, antibody, oligonucleoid, etc.) and a suitable transducer that monitors the degree of the interaction between the former (detection element) and the to-be-detected biomolecules, thereby furnishing detection specific information (Economou et al., 2018). A reduced specificity of targeted biomolecule detection can be attributed to multiple failures (such as contamination of analyte concentration, failure of the detection module, malfunctioning of the electrical circuits, etc.) in the device, which can provide invalid biomarker detection data.
Adhesion of the LoC devices to skin is crucial for sample collection, and to ensure the continuity of the detection process. If a device is loosely attached, the analyte collection module of the LoC device will fail to function properly. Thus, it is imperative that device specific peel tests (Zhao et al., 2020b; Hojaiji et al., 2020) are conducted to validate the adhesion of these layers among themselves and to the human skin.
Unstable biochemical measurements refer to the variable and non-repeatable biomarker detection results in presence of the same input test conditions. Possible causes can point to the presence of an unstable biological or chemical detection element (Sim et al., 2022); furthermore, such unstable measurements will affect the functioning of the device and might lead to eventual failure. It is therefore of prime importance to understand the root cause of these failures, as it will define the failure modes of these LoC devices in different conditions. Subsequently, the failures can simply be modeled as a function of the stress variables (such as moisture ingress, temperature variations, shock or vibrational loads, and conformal deformations), and the major failure modes can be identified as electromigration, crack propagation, delamination of layers, and contamination of microfluidics (see Figure 1). Further discussions on the effect of these stresses on the device have been provided in the following section.
FIGURE 1. A schematic representing the various environmental stresses acting on a wearable and flexible Lab-on-a-Chip device (exploded view of the various stacking layers of an LoC device shown on top-right), and the possible failure modes corresponding to long-term usage of these devices.
Other possible reliability issues for the wearable electronic LoC devices include large chemical impedance for non-invasive methods of sample collection (Cunnigham, 2010), mechanical impedance (Moore and Mundie, 1972) and mechanical noise from skin and contaminants (Atalay et al., 2015), electrical impedance of electrode-skin-body interface (Yao and Zhu, 2016), etc. These issues can be addressed at the design level (by using suitable materials) and the noise caused by these cases can be corrected by using suitable data processing algorithms and other methods (Gandhi et al., 2011).
As pointed out earlier, even the most careful studies on designing novel LoC wearable electronic devices rarely test and establish the reliability of these devices. For example, the robustness (in terms of prolonged adhesion reliability) and performance consistency over multiple loading and unloading cycles has not been explored for the swellable hydrogel patch developed for sweat detection (Zhao et al., 2020b). Similarly, other studies on sweat sensing (Zhao et al., 2021; Kunnel and Demuru, 2022) report repeatable results on the device performance, but the long-term stability and failure-free operation of such devices in out-of-lab settings have not been discussed.
Since wearable LoC devices are prone to continuous stretching, bending, twisting, and folding deformations, the chances that these devices will fail (on various modes, such as delamination, electrical damage, etc.) are relatively high. Considering the mutual dependance of an integrated multi-layered conformal patch consisting of various microfluidic and electrical sensing modules, the adhesion of these layers to themselves and the non-linear surface of the skin is of crucial importance. Kim et al. (2017) studied the adhesion of anisotropic conductive films and their effect on the bending reliability of chip-in-Flex packages. They used oxygen plasma treatment to improve the adhesion between the conductive films and flexible printed circuit substrates and demonstrated stable adhesion up to 160,000 bending cycles without any electrical failures. Similar air plasma treatment helped improve the adhesion of PLA layers, as shown in a study by Lopresti et al. (2022). Using a different approach, Yeo et al. (2016) devised a eutectic gallium indium based thin film microfluidic tactile sensor and reported its robustness in withstanding more than 2,500 loading and unloading cycles. The highly sensitive deformation detection was enabled by the fluid displacement which corresponded to a change in electrical resistance. Cheng et al. (2010) devised a flexible 900 MHz RF (radio frequency) radiation sensor consisting of an elastic antenna and a cluster of conventional rigid circuit for RF power detection that could withstand planar stretching up to 15% elongation and demonstrate reliable performance against folding and twisting deformations. Hojaiji et al. (2020) developed an autonomous wearable system that employs iontophoretic actuation to activate modules for sweat detection and analysis. They performed 180o peeling tests to characterize the adhesion strength of the device to human skin and between different layers of the device. However, the adhesion reliability has not been explored for long-term use of the devices. In retrospect, there are fewer studies available that delve into the long-term reliability of these wearable LoC technologies.
In simpler terms, a wearable device for the consumer needs to be effectively functioning (i.e., the performance degradation of these devices over time should stay above a defined threshold value) for a few years in the presence of standard operating conditions. It should cause no discomfort to the user and should be medically safe. In case of a microfluidic skin patch, for example, qualitatively it should be compatible with human skin, and most importantly, must not fall off from the user (i.e., a sustainable adhesion of the device to the skin should be ensured). In addition, the wearable device is exposed to various environmental stresses such as hygroscopic conditions (i.e., moisture from skin and environmental humidity), temperature variations, shock and/or vibrations from the user and/or environment, and contamination from the body and environment (i.e., bacteria from skin, sweat minerals or protein accumulation on skin from dead skin cells, etc.) (Boysen et al., 1984; Grice and Segre, 2011) as shown in Figure 1. Moisture is a major deterrent to functional electronic circuits and upon exposure can cause electromigration and localized electrical overstress leading to partial or complete failure of the circuit. Since electronics integration is a significant aspect of continuous data retrieval from the microfluidics sensing hardware and if coupled with the process of sample collection, sensing and manipulation, is integral to the basic functioning of the device, the probable failure of these electronics is of prime concern. Furthermore, these circuits are prone to being subjected to frequent stresses corresponding to stretching, folding, bending, and twisting deformations which increase their susceptibility to failure through various modes such as crack initiation and propagation in printed traces, delamination of the conformal layers, and contamination with the electro-chemical networks in the microfluidic channels (Figure 1).
For example, consider a simple wearable LoC device that samples human sweat, detects the concentration of specific ions, and reports back to a memory storage or a server through RF modes. To evaluate the reliability of the device based on stresses due to temperature and humidity, a suitable Temperature-Humidity Bias (THB) accelerated stress testing standard can be used. Based on the standard, a given sample size of devices will be subjected to multiple cycles of stresses (type and profile of stresses can be designed as per requirement). The key performance indicators (KPIs) for the device can be the percentage accuracy of biomarker detection (or any electrical or chemical parameter that defines the detection sensitivity), volume of analyte extracted, detection agent concentration, electrical continuity in the circuits, specified voltage gradients (if any), RF transmission efficiency, etc. The KPIs should be checked before, during, and after the devices have been subjected to accelerated testing: this will help to portray the performance degradation observed in the device. A device can be said to have failed if the respective KPIs drop below a specified threshold value. Subsequent analyses will help to find the root cause of these failures and can point towards one or more of the failure modes mentioned in this article or suggest a new failure mode. Similarly, for a device whose reliability is to be evaluated based on conformal deformations, the device can be subjected to multiple cycles of bending, stretching, and twisting deformations and its failure profile subsequently measured. Based on the root causes observed specific to different stress conditions, suitable design/operational modifications can be deployed to ensure the creation of a highly reliable LoC device.
Further research should be conducted in the domain of creating more reliable LoC devices. A deeper understanding of the failures and the factors causing them are necessary to resolve these issues, and well-designed reliability tests can provide substantial insight into the physics behind these failures. Considering, for example, a case of the delamination of a layer that is caused by higher stress concentrations at the adhesive surfaces, a suitable approach will be to use a material that has higher adhesion strength [as shown by Hojaiji et al. (2020)] or redesign the device to allow for lower stresses at that interface. Similarly, if moisture ingress is found out to be the major source of failures, the device should have either hydrophobic materials or materials with low water absorption properties [such materials have been developed in the studies by Younas et al. (2019) and Ghosh and Ahn (2019)] on the exterior. It is a matter of concern that substantial research on the reliable functioning of LoC devices is lacking in literature.
Therefore, reliability studies on the performance degradation of these devices are of prime importance. Such studies should include investigations on different failure modes observed, the effectiveness of analyte sampling and detection after prolonged usage (conditions mimicking prolonged usage are reproduced, over a much short period of time, by applying accelerated stress testing conditions), device-specific electrical characteristics, the adhesion consistency between the multiple layers of the device, and the adhesion to the skin. Our perspective aims at providing insights and future research directions to research groups and commercial entities to develop reliable wearable devices for health monitoring.
The original contributions presented in the study are included in the article/supplementary material, further inquiries can be directed to the corresponding author.
SS: Writing–original draft, Writing–review and editing. SD: Writing–original draft, Writing–review and editing.
The authors declare that no financial support 6was received for the research, authorship, and/or publication of this article.
The authors declare that the research was conducted in the absence of any commercial or financial relationships that could be construed as a potential conflict of interest.
The author SD declared that they were an editorial board member of Frontiers at the time of submission. This had no impact on the peer review process and the final decision.
All claims expressed in this article are solely those of the authors and do not necessarily represent those of their affiliated organizations, or those of the publisher, the editors and the reviewers. Any product that may be evaluated in this article, or claim that may be made by its manufacturer, is not guaranteed or endorsed by the publisher.
Alizadeh, A., Burns, A., Lenigk, R., Gettings, R., Ashe, J., Porter, A., et al. (2018). A wearable patch for continuous monitoring of sweat electrolytes during exertion. Lab. Chip 18, 2632–2641. doi:10.1039/C8LC00510A
Atalay, O., Kennon, W. R., and Demirok, E. (2015). Weft-knitted strain sensor for monitoring respiratory rate and its electro-mechanical modeling. IEEE Sens. J. 15, 110–122. doi:10.1109/JSEN.2014.2339739
Boysen, T. C., Yanagawa, S., Sato, F., and Sato, K. (1984). A modified anaerobic method of sweat collection. J. Appl. Physiol. Respir. Environ. Exerc. Physiol. 56, 1302–1307. doi:10.1152/jappl.1984.56.5.1302
Cheng, S., and Wu, Z. (2010). Microfluidic stretchable RF electronics. Lab. Chip 10, 3227–3234. doi:10.1039/C005159D
Curto, V. F., Fay, C., Coyle, S., Byne, R., O’Toole, C., Barry, C., et al. (2012). Real-time sweat pH monitoring based on a wearable chemical barcode micro-fluidic platform incorporating ionic liquids. Sens. Actuators B Chem. 171-172, 1327–1334. doi:10.1016/j.snb.2012.06.048
Economou, A., Kokkinos, C., and Prodromidis, M. (2018). Flexible plastic, paper and textile lab-on-a chip platforms for electrochemical biosensing. Lab. Chip 18, 1812–1830. doi:10.1039/c8lc00025e
Gandhi, N., Khe, C., Chung, D., Chi, Y. M., and Cauwenberghs, G. (2011). Properties of dry and non-contact electrodes for wearable physiological sensors. in 2011 International Conference on Body Sensor Networks. Dallas, TX, USA, 23-25 May 2011, IEEE, 107–112. doi:10.1109/BSN.2011.39
Ghosh, S., and Ahn, C. H. (2019). Lyophilization of chemiluminescent substrate reagents for high-sensitive microchannel-based lateral flow assay (MLFA) in point-of-care (POC) diagnostic system. Analyst 144, 2109–2119. doi:10.1039/C8AN01899E
Grice, E. A., and Segre, J. A. (2011). The skin microbiome. Nat. Rev. Microbiol. 9, 244–253. doi:10.1038/nrmicro2537
Heikenfeld, J. (2016). Non-invasive analyte access and sensing through eccrine sweat: challenges and outlook circa 2016. Electronanalysis 28, 1242–1249. doi:10.1002/elan.201600018
Hojaiji, H., Zhao, Y., Gong, M. C., Mallajosyula, M., Tan, J., Lin, H., et al. (2020). An autonomous wearable system for diurnal sweat biomarker data acquisition. Lab. Chip 20, 4582–4591. doi:10.1039/d0lc00820f
Hu, Y., Wang, L., Li, J., Yang, Y., Zhao, G., Liu, Y., et al. (2023). Thin, soft, skin-integrated electronics for real-time and wireless detection of uric acid in sweat. Int. J. Smart Nano Mat. 1, 1–14. doi:10.1080/19475411.2023.2236997
Huang, X., Cheng, H., Chen, K., Zhang, Y., Zhang, Y., Liu, Y., et al. (2013). Epidermal impedance sensing sheets for precision hydration assessment and spatial mapping. IEEE Trans. Biomed. Eng. 60, 2848–2857. doi:10.1109/TBME.2013.2264879
Kim, D.-H., Ahn, J.-H., Choi, W. M., Kim, H.-S., Song, J., et al. (2008). Stretchable and foldable silicon integrated circuits. Science 320, 507–511. doi:10.1126/science.1154367
Kim, D.-H., Kim, Y.-S., Wu, J., Liu, Z., Song, J., Kim, H.-S., et al. (2009). Ultrathin silicon circuits with strain-isolation layers and mesh layouts for high-performance electronics on fabric, vinyl, leather, and paper. Adv. Mat. 21, 3703–3707. doi:10.1002/adma.200900405
Kim, J.-H., Lee, T.-I., Kim, T.-S., and Paik, K.-W. (2017). The effect of anisotropic conductive films adhesion on the bending reliability of chip-in-flex packages for wearable electronics applications. IEEE Trans. Compon. Packag. Manuf. 7, 1583–1591. doi:10.1109/TCPMT.2017.2718186
Ko, H. C., Stoykovich, M. P., Song, J., Malyarchuk, V., Choi, W. M., Yu, C.-J., et al. (2008). A hemispherical electronic eye camera based on compressible silicon optoelectronics. Nature 454, 748–753. doi:10.1038/nature07113
Kunnel, B. P., and Demuru, S. (2022). An epidermal wearable microfluidic patch for simultaneous sampling, storage, and analysis of biofluids with counterion monitoring. Lab. Chip 22, 1793–1804. doi:10.1039/d2lc00183g
Kwon, W.-K., Yoo, C.-M., Kim, J. H., Kim, T.-W., Lim, A.-G., Hwang, M.-H., et al. (2023). Role of human dural fibroblasts in the angiogenic responses of human endothelial cells: an in vitro dural model and the application of lab-on-a-chip for EDAS. Bioeng. Transl. Med. 2023, e10589. doi:10.1002/btm2.10589
Lezen, H., Barrow, B. A., White, S., and Holman, R. R. (2002). A non-invasive frequent home blood glucose monitor. Pract. Diabetes. 19, 101–103. doi:10.1002/pdi.312
Lin, H., Tan, J., Zhu, J., Lin, S., Zhao, Y., Yu, W., et al. (2020). A programmable epidermal microfluidic valving system for wearable biofluid management and contextual biomarker analysis. Nat. Comm. 11, 4405. doi:10.1038/s41467-020-18238-6
Lopresti, F., Patella, B., Divita, V., Zanca, C., Botta, L., Radacsi, N., et al. (2022). Green and integrated wearable electrochemical sensor for chloride detection in sweat. Sensors 22, 8223. doi:10.3390/s22218223
Moore, T. J., and Mundie, J. R. (1972). Measurement of specific mechanical impedance of the skin: effects of static force, site of stimulation, area of probe, and presence of a surround. J. Acoust. Soc. Am. 52, 577–584. doi:10.1121/1.1913148
Sim, D., Brothers, M. C., Slocik, J. M., Islam, A. E., Maruyama, B., Grigsby, C. C., et al. (2022). Biomarkers and detection platforms for human health and performance monitoring: a review. Adv. Sci. 9, 2104426. doi:10.1002/advs.202104426
Trinh, K. T. L., and Lee, N. Y. (2022). Fabrication of wearable PDMS device for rapid detection of nucleic acids via recombinase polymerase amplification operated by human body heat. Biosensors 12, 72. doi:10.3390/bios12020072
Vinoth, R., Nakagawa, T., Mathiyarasu, J., and Vinu Mohan, A. M. (2021). Fully printed wearable microfluidic devices for high-throughput sweat sampling and multiplexed electrochemical analysis. ACS Sens. 6, 1174–1186. doi:10.1021/acssensors.0c02446
Viventi, J., Kim, D.-H., Moss, J. D., Kim, Y.-S., Blanco, J. A., Annetta, N., et al. (2010). A conformal, bio-interfaced class of silicon electronics for mapping cardiac electrophysiology. Sci. Transl. Med. 2, 24ra22. doi:10.1126/scitranslmed.3000738
Webb, R. C., Bonifas, A. P., Behnaz, A., Zhang, Y., Yu, K. J., Cheng, H., et al. (2013). Ultrathin conformal devices for precise and continuous thermal characterization of human skin. Nat. Mat. 12, 938–944. doi:10.1038/nmat3755
Yao, S., and Zhu, Y. (2016). Nanomaterial-enabled dry electrodes for electrophysiological sensing: a review. JOM 68, 1145–1155. doi:10.1007/s11837-016-1818-0
Yeo, J. C., Yu, J., Koh, Z. M., Wang, Z., and Lim, C. T. (2016). Wearable tactile sensor based on flexible microfluidics. Lab. Chip 16, 3244–3250. doi:10.1039/C6LC00579A
Younas, M., Maryam, A., Khan, M., Nawaz, A. A., Jaffery, S. H. I., Anwar, M. N., et al. (2019). Parametric analysis of wax printing technique for fabricating microfluidic paper-based analytic devices (μPAD) for milk adulteration analysis. Microfluid. Nanofluidics. 23, 38. doi:10.1007/s10404-019-2208-z
Zhao, F. J., Bonmarin, M., Chen, Z. C., Larson, M., Fay, D., Runnoe, D., et al. (2020a). Ultra-simple wearable local sweat volume monitoring patch based on swellable hydrogels. Lab. Chip 20, 168–174. doi:10.1039/c9lc00911f
Zhao, F. J., Wang, B., Hojaiji, H., Wang, Z., Lin, S., Yeung, C., et al. (2020b). A wearable freestanding electrochemical sensing system. Sci. Adv. 6, eaaz0007. doi:10.1126/sciadv.aaz0007
Keywords: reliability, failures, lab-on-a-chip devices, wearable electronics, sensors
Citation: Subudhi SK and Das S (2023) Reliability of lab-on-a-chip technologies for wearable electronics: a perspective. Front. Sens. 4:1283402. doi: 10.3389/fsens.2023.1283402
Received: 26 August 2023; Accepted: 03 October 2023;
Published: 13 October 2023.
Edited by:
Suman Chakraborty, Indian Institute of Technology Kharagpur, IndiaReviewed by:
Tonghathai Phairatana, Prince of Songkla University, ThailandCopyright © 2023 Subudhi and Das. This is an open-access article distributed under the terms of the Creative Commons Attribution License (CC BY). The use, distribution or reproduction in other forums is permitted, provided the original author(s) and the copyright owner(s) are credited and that the original publication in this journal is cited, in accordance with accepted academic practice. No use, distribution or reproduction is permitted which does not comply with these terms.
*Correspondence: Siddhartha Das, c2lkZEB1bWQuZWR1
Disclaimer: All claims expressed in this article are solely those of the authors and do not necessarily represent those of their affiliated organizations, or those of the publisher, the editors and the reviewers. Any product that may be evaluated in this article or claim that may be made by its manufacturer is not guaranteed or endorsed by the publisher.
Research integrity at Frontiers
Learn more about the work of our research integrity team to safeguard the quality of each article we publish.