- Department of Forensic Science, Environmental and Life Science Program, Trent University, Peterborough, ON, Canada
Microplastics (MPs) are a part of our daily lives and persist in the environment all across the globe. As a recently recognized emerging pollutant, there is a call to action to mitigate and monitor microplastics. Despite traditional remediation and characterization methodologies, MP-related challenges still exist. Electrochemical strategies for microplastic remediation have been reported in recent years, but very few reports exist on using electrochemical sensors for monitoring microplastics. Therefore, this minireview highlights the opportunities within the existing electrochemical remediation platforms towards sensor design and development, and elaborates on microplastic electrochemical sensors so far.
Microplastics chemistry and relevance
An estimated 8 million tons of plastic waste enter the ocean each year, affecting close to 700 marine species (Piazza et al., 2022). Without proper management, the undesired release of microplastics (MPs) into the environment can contribute to environmental stressors and negative effects for such ecosystems, plants, and further down the food chain. With the current trend, the accumulation of secondary MPs in the environment is predicted to rise 155–265 million tons by the year 2060, with 13.2% of this being contributed by MPs (Lebreton and Andrady, 2019; Sobhani et al., 2020).
Microplastics are made from synthetic organic polymers such as polyethylene (PE), polypropylene (PP), polystyrene (PS), poly(ethylene terephthalate) (PET), and poly(vinyl chloride) (PVC), among others (Andrady, 2011; Cole et al., 2011; Oliveira et al., 2022). Plastics can be characterized based on size, namely macro-plastics (>25 mm), mesoplastics (5–25 mm), microplastics (MPs) (0.1 μm-10 mm) or nanoplastics (<100 nm) (Cole et al., 2011; Sobhani et al., 2020; Oliveira et al., 2022). MPs vary in polymer type, size, shape, persistence and matrix, and thus are challenging to accurately measure (Cole et al., 2011; Sobhani et al., 2020; Kiendrebeogo et al., 2021; Oliveira et al., 2022). MPs are generally produced directly from industrial sources or indirectly generated from larger plastics (Cole et al., 2011; Sobhani et al., 2020; Kannan and Vimalkumar, 2021).
The main concerns surrounding the ubiquity of MPs in the environment is regarding their impact on human and marine health (Prata et al., 2020; Sobhani et al., 2020). Considering that MPs are not visible to the naked eye due to their small sizes, they can readily enter marine ecosystems through runoff, as industrial waste and direct emissions from populated areas without being detected (Andrady, 2011; Smith et al., 2018; Kiendrebeogo et al., 2021; Oliveira et al., 2022) and have been found in many of our everyday foods (Prata et al., 2020; Smith et al., 2018; Sobhani et al., 2020). MPs also have a high adsorption capacity and act as a matrix/carrier for other harmful environmental pollutants (Cole et al., 2011; Sobhani et al., 2020; Oliveira et al., 2022). MP exposure through ingestion, inhalation or dermal contact, introduces adverse effects on health. Reports of hepatic stress, formation of granulomas, bronchial reactions, chronic pneumonia or bronchitis, pneumothorax, respiratory lesions, changes in gut microbe composition and metabolism and cognitive impairment have all been associated with MP exposure in humans (Prata et al., 2020; Prata, 2018).
Current strategies for MP remediation are mainly based on biodegradation using fungi, bacteria and enzymes (Corona et al., 2020). However, photodegradation, thermal treatments and chemical treatments have also been applied to MP removal (Hermanová and Pumera, 2022; Wu et al., 2017; Hu et al., 2021;, 2022). More recently, the photocatalysis, and plasma-based oxidation, amongst others, have been developed as an alternative low toxicity generating strategy for MP remediation (Wu et al., 2017; Hu et al., 2022). However, MPs are generally highly resistant to bio- and photo-degradation resulting in low efficiency removal (Cole et al., 2011; Sobhani et al., 2020; Hu et al., 2021). These characteristics make monitoring, and removal of MP waste important but a challenging task. Thus, for improving monitoring MP transport and regulatory practices, the detection of MPs is of utmost importance.
Other reviews on the topic of monitoring and measuring MPs have been covered in recent publications (Guo et al., 2022; Ivleva, 2021; Yu et al., 2022). The electrochemical review on using boron-doped diamond electrodes for detection of plastic leachates, small organic molecules, has also been published (Oliveira et al., 2022). In this mini-review, we present recent discoveries on using electrochemical methodologies for remediating and monitoring MPs directly, and the technological opportunities for MP pollutants.
Electrochemical remediation of microplastics
Remediation of MPs includes removal, degradation or recycling from the environment. All of these strategies can be achieved by using electrochemistry. For example, removal of MPs has been achieved through electrocoagulation. Electrocoagulation is a low-cost and environmentally-friendly solution for removing pollutants and contaminants from water (Zeboudji et al., 2013). The technique involves a process in which a metal electrode produces cations (anodic dissolution) under an electric field which then bind to MPs to coagulate them into larger precipitates that can be removed by filtration (Elkhatib et al., 2021; Kim and Park, 2021; Perren et al., 2018; Sharma et al., 2021). During this process, the selection of the optimized applied electric field is crucial for facilitating electrocoagulation to maximize remediation efficiency, while minimizing the electrical energy consumption (Shen et al., 2022). The power of electric field strength and its ranges for electrocoagulation are dependent on the interelectrode distance between the anode/cathode. The closer the electrodes, the stronger the electric field. In the electrocoagulation of total organic carbon of municipal wastewater, the strength of the electric field was highest with an interelectrode distance of 0.5 cm and lowest with 2 cm. Hence, it can also be said that the DEP (dielectrophoresis) force is also highest at 0.5 cm. In general, the positively charged metal ions and their complexes electrostatically interact with negatively charged MPs leading to MP aggregation (Figure 1A). Using a bench-scale stir-tank batch reactor and wastewater, two electrodes (anode and cathode made from aluminum) were used in parallel. The effects of wastewater pH and conductivity, and applied current density were operational parameters tested to determine the effectiveness of the method in wastewater treatment of MPs. The MPs were substantially removed up to 90–100% efficiency in simulated wastewater using electrocoagulation. The process was also applicable in a wide range of wastewater pH values (3–10) demonstrating that the low-cost technique has the potential to remove other forms of MPs in a variety of domestic wastewaters and industrial effluents (Perren et al., 2018). In this process, the anode/cathode material plays a critical role in remediation efficiency. For example, the aluminum electrodes were found to be more effective than iron electrodes (Ozyonar and Karagozoglu, 2011). Using the Al electrode, removal efficiency of MPs was >90% compared to that achieved using the Fe electrode, which ranged from 59–85% for PE, PMMA, cellulose acetate (CA) and PP MPs (Shen et al., 2022). Additionally, the MP flocs produced by iron anode had dense precipitation and fast sedimentation, which may have been contributed as a result of iron corrosion. Hence, faster corrosion of iron compared to aluminum leads to less effective application. The flocs produced by aluminum anode had a small floc size which was attributed to a larger surface area for MP remediation, resulting in fast speed, and strong adsorption capacity. The phosphate removal efficiency using electrocoagulation was 100 and 84.7% using Al and Fe electrodes respectively. The electrode mass depletion was also observed to be lower for the Al electrode compared to the Fe electrode under the same experimental conditions (Behbahani et al., 2011). The aluminum electrode was preferred to iron since it had a higher removal rate of COD, turbidity, and phosphate. The optimum operating conditions were found for original pH (7.8), 100 A/m2, and 10 min EC time and obtained removal efficiencies of COD, turbidity, and phosphorus (72, 98, and 98%), respectively (Ozyonar and Karagozoglu, 2011). The comparison of anode/cathode material, electrolyte type, reaction time and efficiency for various electrocoagulation methods are compared in Table 1. Clearly, efficiency is lower when Fe and Cu were used as anode and cathode materials, respectively. Using Al as an electrode material afforded the most efficient electrocoagulation.
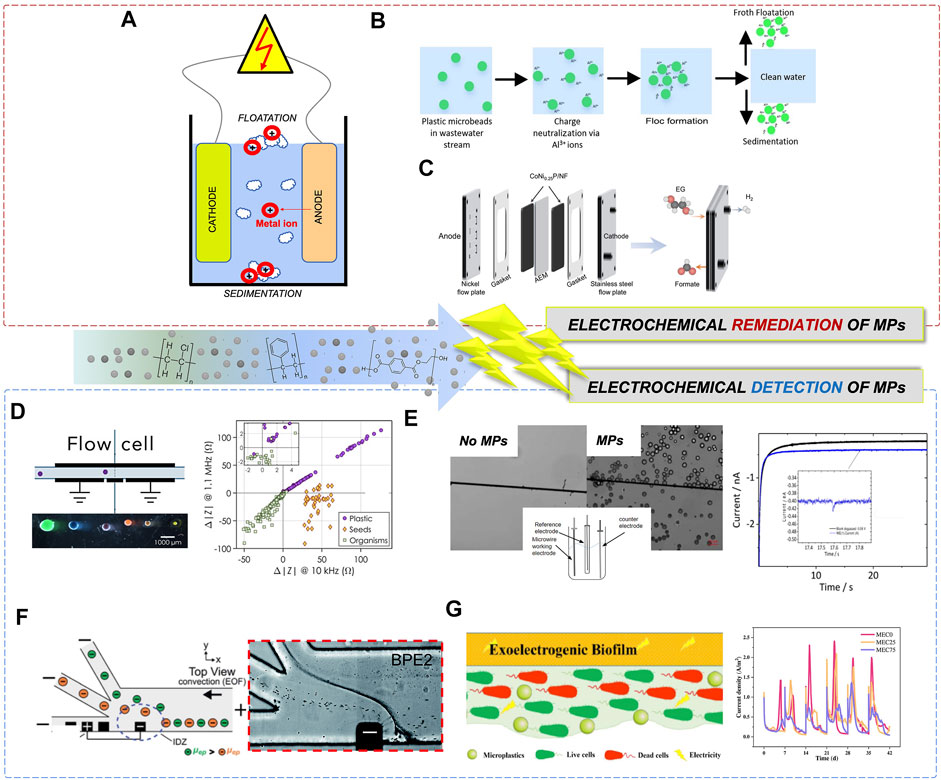
FIGURE 1. Illustration of electrochemical remediation and monitoring approaches for MPs. (A) Schematic of microplastic removal from water by electrocoagulation process. (B) Schematic of electrocoagulation of microbeads from waste water (Perren et al., 2018). (C) Membrane-electrode assembly (MEA) for electrooxidation of polymer breakdown products, specifically ethylene glycol oxidation into formate (Zhou et al., 2021). (D) The schematic of MPs passing through a flow cell for impedance measurements (left). Change in impedance as a function of particle type: organism, seeds, or plastic (inset shows little overlap between plastic and organism indicating excellent differentiation abilities) (Colson and Michel, 2021). (E) Optical microscopy images of the carbon fiber electrode in the absence and presence of MPs (left). The chronoamperogram (right) of the PE MPs (blue line) and in the absence of MPs (black line) (the inset provides an enlarged view of a transient current-time signal resulting from a collision event between MP and electrode (Shimizu et al., 2017). (F) Schematic of a device for continuous MP sorting based on MP electrophoretic mobilities, showing BPE across microchannel. Optical image (left) of two types of MPs (different sizes) being nearly qualitatively sorted into the middle and top outlet channels according to their electrophoretic mobility (Davies and Crooks, 2020). (G) Schematic of exoelectrogenic biofilm on electrode surface and its interactions with MPs (left). Current density output in MEC as a function of increasing MP concentrations (right) (Wang et al., 2022).

TABLE 1. The comparison of electrode material, electrolyte, reaction time and efficiency of various electrocoagulation setups.
The MP flocs produced by iron anode had dense precipitation and fast sedimentation, while the flocs produced by aluminum anode had fast speed, and strong adsorption capacity. Electrocoagulation was further expanded for removal of PE, PP, PMMA and cellulose MPs (Figure 1A) (Shen et al., 2022). The removal rates also varied from 50 - 99%, depending on the MP types, and more efficient rates with PP compared to the slowest rates with PMMA. Using the electrocoagulation removal process for MP, it is important to consider energy consumption required for the actual operation of this remediation strategy. During electrocoagulation the plastic MPs are charge neutralized by metal ions, such as Al(III) (Figure 1B), which in turn form flocs leading to froth floatation or sedimentation (Perren et al., 2018). The precipitates or floats formed during electrocoagulation may be used as indicators of MP presence, and exploited as sensor signaling probes in the future.
A degradation of MPs is also possible by carrying out electrochemical oxidation of polymeric materials. The diverse reactive oxygen species formed at high concentrations, including hydroxyl radical and hydrogen peroxide, during an electrochemical process react with polymeric chemical bonds in MPs (Kiendrebeogo et al., 2021). The electrooxidation of MP requires harsh oxidation mediators such as silver, cobalt or cerium ions, all of which are characterized with redox potentials above 1.7 V (Weber and Ramasamy, 2020). Unlike metallic electrodes, the boron-doped diamond electrode (BDD) has been a popular choice due to its capability for degradation and detection of various pollutants (Fernandez et al., 2020). Furthermore, the degradation of MPs in water, specifically PS MPs, was achieved by using BDD and electrooxidation processes. Within several hours of electrolysis, MPs were degraded directly (∼90%) into gaseous products such as CO2 rather than smaller particles (Kiendrebeogo et al., 2021). The mechanism of the degradation of PS can be described as the formation of hydroxyl radicals generated from water at the anode surface, followed by the radical-initiated breaking the polymer C-H bond, leading to the formation of a C-O bond. The polymeric C-C bonds of PS were effectively broken as well.
Further oxidation of the polymeric chain eventually led to its decomposition into CO2 and water. The subsequent CO2 production may be used in a sensor development to detect the presence of MPs. To improve MP degradation, various operating parameters were investigated such as a current intensity, anode surface, electrolyte type, electrolyte concentration, and reaction time to optimize the electrooxidation process. Other anode electrode materials, such as metal oxide (iridium oxide) and titanium electrode as the cathode were also tested for breaking down of MPs (Kiendrebeogo et al., 2021). The BDD electrode exhibited higher oxidation potentials compared to other anode materials. The reaction rate for the degradation of MPs was also observed to be higher using the BDD compared to the above-mentioned anode electrodes. BDD electrodes were observed to have a better MP removal efficiency (%) compared to mixed metal oxide and iridium oxide anodes. The higher removal efficiency was attributed to the BDD’s ability to generate a higher rate of hydroxyl radicals. Alongside MPs, nanoplastics (NPs) are also persistent plastics in the environment and may stem from weathering of MPs. The hydroxyl radicals from water discharge and sulfate radicals from electrolyte were active agents for NP degradation. The process had a higher degradation efficiency (86.6%) and was 2.6 times more effective than the traditional (sulfate radical free) process. Indeed, the sulfate radical remediated oxidation has been reported to be more effective than hydroxyl radical mediated oxidation (Divyapriya and Nidheesh, 2021; Nidheesh and Rajan, 2016). The NPs were also degraded by using BDD or carbon felt electrodes with over 85% efficiency (Kiendrebeogo et al., 2022). This approach was also successfully applied for anodic oxidation of PS as well (Kiendrebeogo et al., 2021). Other electrochemical transformations such as oxidative dehydrogenation of alkanes may provide additional avenues for MP remediation (Kiendrebeogo et al., 2022; Kiendrebeogo et al., 2021; Weber and Ramasamy, 2020). However, such new chemistries for electrochemical degradation of plastics remain largely underexplored.
In addition to anodic oxidation, cathode reduction treatments have also been explored to degrade MPs. The electrocatalytic treatment of MPs using titanium dioxide/graphite (TiO2/C) cathode resulted in high dechlorination efficiency of polyvinyl chloride (PVC) at 75% after 6 h at constant applied potential of −0.7 V vs. Ag/AgCl. The degradation pathway began with the dechlorination of PVC via cathodic reduction and the anodic oxidation of the hydroxyl radicals simultaneously (Miao et al., 2020). Unlike anodic oxidation, this process allows for removal of halogenated polymers. The formation of halogenated side products, such as HCl or perchlorates may be measured to indirectly monitor MP presence in a sensor application.
Although removal and degradation are normally the main goals of MP remediation, product conversion is also a viable solution. Recycling and repurposing of MPs can also generate new value-added chemicals while simultaneously removing MPs from the environment. The electrocatalytic upcycling of polyethylene terephthalate (PET) to chemicals such as potassium diformate, terephthalic acid and hydrogen gas - which have valuable industrial application - has been recently achieved (Zhou et al., 2021). The membrane-electrode assembly (MEA) based on the nickel plate anode and stainless steel cathode was employed to evaluate ethylene glycol oxidation to formate using nickel-modified cobalt phosphide (CoNixP) as the electrocatalyst (Figure 1C). While the polymer required initial chemical treatment to form monomers, such as terephthalic acid and ethylene glycol, the use of electrocatalyst resulted in high current densities at 1.7 V vs. RHE, with high selectivity (>80%) and faradaic efficiency (>80%) for formate product. In this work, the value-added chemical formation from MPs was the focus, however, one could imagine using formic acid, hydrogen gas or terephthalic acid as indicators in sensor design and development to detect MPs present in a sample of interest.
Overall, electrochemical processes for remediation of MPs are on the order of hours compared to traditional methodologies, which makes these electrochemical methods advantageous for practical applications. Although the electrochemical remediation strategies have shown great potential in solving the MP crisis, there are still challenges associated with MP. Current electrochemical processes may require high applied potentials or chemical oxidation which may lead to undesirable side reactions. The current procedures for isolation, characterization, and measurement are poorly defined which make MP remediation difficult. Future directions for electrochemical remediation of MPs may include standardized procedures, multi-faceted platform capable of simultaneous removal, degradation and recycling, more rapid and scalable MP remediation process, design and development of nanomaterials/electrode materials and catalysts, and development of novel electrochemical methods (Chellasamy et al., 2022; Goh et al., 2022). It is clear however that electrochemical remediation yields a variety of chemical entities, as indirect indicators of MPs, which may be utilized as sensing probes for the presence of MPs. The detection of MPs by using electrochemical sensors is critical for environmental monitoring, understanding transport and the fate of MPs and is discussed in the next section.
Electrochemical sensors for microplastics
The electrochemical sensors have been widely applied for detection of environmental pollutants, including leachates from MPs (Oliveira et al., 2022), but their uses for MP detection are rather scarce, and are only now gaining attention. The development of electrochemical sensors for MPs are of particular interest because of the short response time, simple operation, portability, and low cost associated with electrochemical devices. Unlike other conventional methods, the electrochemical methods are also easily extended to infield testing of a wide range of sample types, without a need for prior MP purification or isolation. Up-to-date, the MP monitoring and sensing has been achieved by using a label free electrochemical impedance spectroscopy (EIS), amperometry and voltammetry.
Sensing of PE MPs was achieved with an EIS-based sensor in conjunction with flow cytometry (Colson and Michel, 2021). The sensor was made up of a flow cell with Au-plated circuit boards, containing all Au-plated electrodes, for EIS detection, and the flow cytometry for particle detection (Figure 1D). This method was based on the concept that the change in real part of the impedance at low frequency should be proportional to particle volume. Hence, the change in impedance is a result of the MP particles flowing over electrodes, and at the low frequency, the change in impedance is proportional to the MP particle volume (Figure 1D). Using this system, the plastic and biological particles could be distinguished at any frequency measured. Interestingly, the biological particles induced negative change in impedance, while MPs induced a positive change, allowing this platform to be used for MPs detection in complex media. The clean water flow was tested in real-time and compared to water spiked with known MPs to determine the sensitivity of the sensor and its ability to measure and differentiate various sizes of the MPs. If MP was present in solution tested, the impedance would change, causing peaks, and allowing for quantification of particle size. To link impedance change to the particle size, the linear relationship between particle diameter and the cube root of the real impedance change was used. Ultimately, this sensor was capable of quantifying and sizing the MP beads (including biological structures within the 210—1200 µm size range), and the PE MPs (212—1000 µm). The sensor had a recovery rate >90% for MPs in the 100–300 um size range, and a 1% false positive rate for detecting biological materials as MPs (Colson and Michel, 2021). While EIS was successfully used as a label free method for detection of particles including MPs, the next generation sensor should aim at distinguishing between other types of MP particles, beyond PE, as well as MP detection in field.
Particle impact electrochemistry has also been utilized in the detection of spherical PE MPs (1–22 µm) (Shimizu et al., 2017). The particle-electrode impact method is widely used to study particles suspended in solution. The particle impact with the carbon fiber microwire electrode resulted in a rapid current response during chronoamperometry measurements. The electrochemical analysis set up consisted of an undivided three-electrode setup, held at a specific potential to observe a desirable reaction, and the signal change measured was a result of particle-electrode collision. The collision of particles to the electrode produced a transient current response, or spike, which was analyzed to detect MP (Figure 1E). The current spike in chronoamperogram was due to MP collision with the working electrode due to the reduction of oxygen in PE MP particles. Using this method, an excellent correlation was established between the frequency of the spikes and MP particle concentrations, with an R2 value of 0.96 (Shimizu et al., 2017). Overall, this method of detection provided a more reliable and accurate measurement of MP compared to conventional methods. While the method was applied to detection of electrically insulating MPs, detection of conductive particles is also possible.
The alternative method for MP detection is a serial faradaic ion concentration polarization (fICP) technique (Davies and Crooks, 2020). The fICP sorts the MPs based on the electrophoretic mobilities. The electrophoretic mobilities of the MPs affect the interaction with the electric field gradients (EFG). Particles with larger electrophoretic mobility focus at a position with a low electric field. Whereas smaller electrophoretic mobilities are focused in a higher electric field. A trifurcated trunk was used to sort MPs into different chambers by placing the bipolar electrodes (BPE) in proximity to the trunk. The positioning of the BPE places the IDZ and EFG across the opening of the bifurcated channel. Thus, providing a sorting mechanism for the MPs, moving the particles with the larger electrophoretic mobility into the lower channel, and the smaller into the upper channel. The fICP was utilized to control the flow of PS MP in a trifurcated microchannel. This method allowed for continuous focusing, sorting, and separating MPs. Sorting of MPs based on their electrophoretic mobilities was possible due to their interactions with electric field gradients, and being ultimately detected using optical and fluorescence microscopy. As the MPs moved toward the cathodic end of the bipolar electrodes, the electric field gradients directed the trajectory of the MPs toward the trifurcated microchannels, which sorted the MPs based on their electrophoretic mobilities and size (Figure 1F). This approach makes it possible to continuously and in real-time monitor MPs in water systems, as well as isolate or preconcentrate various MPs on demand without a need for membrane-based separation, which further simplifies this technology. Importantly, interfacing of fICP with other techniques would allow for additional flexibility in sensor design and development, but this is an opportunity that has not been exploited yet for MP detection. More recently, a low-cost and high-throughput method was developed based on the multiuse resistive pulse sensor (Pollard, et al., 2020). This microfluidic sensor, based on silver wire, monitored a change in current by the translocation of an analyte (MP) through a narrow constriction. The device was used to monitor MPs in tea bags, as well as algae detection, in order to demonstrate the utility of this method for counting and monitoring MPs in the presence of biological particles, which currently remains a challenge.
In addition to impedimetric and amperometric sensors for MPs, other examples of dual-mode sensors also have been reported. Using voltammetry and impedance, a sensor for detection of PE MP by using exoelectrogenic biofilms has been developed (Wang et al., 2022). Electroactive bacteria film used in microbial electrochemical systems showed a great promise as an energy efficient approach for wastewater MP detection. The biofilms were exposed to MPs to determine the response and investigate the electrochemical biofilm properties, biofilm morphology, extracellular polymeric substances (EPS), and microbial structure in response to MPs. A three-electrode setup consisted of a carbon fiber brush anode as a working electrode, Ag/AgCl as a reference electrode, and a titanium, woven wire mesh as a cathode counter electrode. The increased internal resistance was due to PE-MP binding to biofilm (Wang et al., 2022). EIS was performed to measure the impedance of the MFCs and MECs, using Nyquist plots, before and after binding. The resistance of the cells increased due to the presence of PE-MP. Most of the resistance, calculated from the equivalent circuit model, was due to the resistance to charge transfer, Rct. In addition, the toxic effects of the PE-MPs can also result in increased population of dead cells, causing a significant increase in the Rct. The current density did not change in microbial fuel cells when PE MP was present. However, in the microbial electrolysis cell, there was a decrease in current signal with increased MP concentrations, a trend which was stable for over 42 days (Figure 1G). The decrease in signal was due to the increased internal resistance due to PE MP binding to biofilm (Wang et al., 2022). Therefore, for sensing of PE MP using exoelectrogenic biofilms, the microbial electrolysis cells would have to be utilized to quantify the concentration of the MP present. In future, a microbial electrolysis cell may also be used for differentiation of MP types and size to extend its application to a real-life setting. In addition, the identical method may be repurposed for the remediation of MP in the microbial electrochemical systems for treating wastewater.
While electrochemical methodologies have been applied to MP remediation, the sensors for MPs remain under discovered. The evidence of successful electrochemical sensors for MP exists, but additional sensor design and development in MP monitoring is needed. Several of MP remediation strategies represent perfect opportunities for developing new testing tools. For example, MP remediation may be indirectly used for sensing purposes such as detection of MP coagulation, sensing of MP byproducts by using anodic oxidation or reduction of MP, detection of MP interactions with biofilms or other biologicals, etc. To advance electrochemical sensors for MPs, the current challenges include MP identification and differentiation, standardized procedures for MP isolation, characterization and environmental monitoring and tracking, and MP detection in a variety of sample types (soil, soil runoffs, various watersheds and pools, wastewaters and industrial effluents, agricultural watersheds, atmosphere, etc.). The electrochemical devices could also provide means of continuous monitoring of transport and fate of MPs through the environment in real-time. Important aspect of MP chemistry is its degradation into smaller NPs and hence detection and monitoring of plastic particles downstream from MP is also required. The MP easily interacts with and adsorbs other biomolecules and using electrochemical methodologies to gain insight into their fundamental interactions will provide us with new avenues for MP monitoring, and remediation.
Conclusion and outlook
MP is a part of our everyday lives and can be found all over our planet Earth, from the Arctic to the ocean floor. The challenges associated with end-of-life of these materials still need to be resolved, and MP monitoring and remediation are the immediate calls to action to mitigate this pollutant. The electrochemical methods have opened a great door of opportunities for fundamental discoveries about MPs, remediation tools, and fabrication of sensors for their monitoring. Hence, electrochemical methodologies are powerful tools which can transform our understanding of MPs in the environment and facilitate their monitoring and remediation.
Author contributions
All authors listed have made a substantial, direct, and intellectual contribution to the work and approved it for publication.
Funding
This work was supported by NSERC Discovery Grant.
Conflict of interest
The authors declare that the research was conducted in the absence of any commercial or financial relationships that could be construed as a potential conflict of interest.
Publisher’s note
All claims expressed in this article are solely those of the authors and do not necessarily represent those of their affiliated organizations, or those of the publisher, the editors and the reviewers. Any product that may be evaluated in this article, or claim that may be made by its manufacturer, is not guaranteed or endorsed by the publisher.
References
Andrady, A. L. (2011). Microplastics in the marine environment. Mar. Pollut. Bull. 62 (8), 1596–1605. doi:10.1016/j.marpolbul.2011.05.030
Behbahani, M., Alavi Moghaddam, M. R., and Arami, M. (2011). A comparison between aluminum and iron electrodes on removal of phosphate from aqueous solutions by electrocoagulation process. Int. J. Environ. Res. 5 (2), 403–412.
Chellasamy, G., Kiriyanthan, R. M., Maharajan, T., Radha, A., and Yun, K. (2022). Remediation of microplastics using bionanomaterials: A review. Environ. Res. 208, 112724. doi:10.1016/j.envres.2022.112724
Cole, M., Lindeque, P., Halsband, C., and Galloway, T. S. (2011). Microplastics as contaminants in the marine environment: A review. Mar. Pollut. Bull. 62 (12), 2588–2597. doi:10.1016/j.marpolbul.2011.09.025
Colson, B. C., and Michel, A. P. M. (2021). Flow-through quantification of microplastics using impedance spectroscopy. ACS Sens. 6 (1), 238–244. doi:10.1021/acssensors.0c02223
Corona, E., Martin, C., Marasco, R., and Duarte, C. M. (2020). Passive and active removal of marine microplastics by a mushroom coral (danafungia scruposa). Front. Mar. Sci. 7, 128. doi:10.3389/fmars.2020.00128
Davies, C., and Crooks, R. (2020). Focusing, sorting, and separating microplastics by serial faradaic ion concentration polarization. Chem. Sci. 11, 5547–5558. doi:10.1039/D0SC01931C
Divyapriya, G., and Nidheesh, P. V. (2021). Electrochemically generated sulfate radicals by boron doped diamond and its environmental applications. Curr. Opin. Solid State Mat. Sci. 25 (3), 100921. doi:10.1016/j.cossms.2021.100921
Elkhatib, D., Oyanedel-Craver, V., and Carisimmi, E. (2021). Electrocoagulation applied for the removal of microplastics from wastewater treatment facilities. Sep. Purif. Technol. 276, 118877. doi:10.1016/j.seppur.2021.118877
Fernandez, A., Pereira, C., Koziol, V., Pacheco, M. J., Ciriaco, L., and Lopes, A. (2020). Emerging contaminants removal from effluents with complex matrices by electrooxidation. Sci. Total Environ. 740, 140153. doi:10.1016/j.scitotenv.2020.140153
Goh, P. S., Kang, H. S., Ismail, A. F., Khor, W. H., Quen, L. K., and Higgins, D. (2022). Nanomaterials for microplastic remediation from aquatic environment: Why nano matters? Chemosphere 299, 134418. doi:10.1016/j.chemosphere.2022.134418
Guo, X., Lin, H., Xu, S., and He, (2022). Recent advances in spectroscopic techniques for the analysis of microplastics in food. J. Agric. Food Chem. 70 (5), 1410–1422. doi:10.1021/acs.jafc.1c06085
Hermanová, S., and Pumera, M. (2022). Micromachines for microplastics treatment. ACS Nanosci. Au 2, 225–232. doi:10.1021/acsnanoscienceau.1c00058
Hu, K., Tian, W., Yang, Y., Nie, G., Zhou, P., Wang, Y., et al. (2021). Microplastics remediation in aqueous systems: strategies and technologies. Water Res. 198, 117144. doi:10.1016/j.watres.2021.117144
Hu, K., Zhou, P., Yang, Y., Hall, T., Nie, G., Xao, Y., et al. (2022). Degradation of microplastics by a thermal fenton reaction. ACS Est. Eng. 2 (1), 110–120. doi:10.1021/acsestengg.1c00323
Ivleva, N. P. (2021). Chemical analysis of microplastics and nanoplastics: challenges, advanced methods, and perspectives. Chem. Rev. 121 (19), 11886–11936. doi:10.1021/acs.chemrev.1c00178
Kannan, K., and Vimalkumar, K. (2021). A review of human exposure to microplastics and insights into microplastics as obesogens. Front. Endocrinol. 12, 724989. doi:10.3389/fendo.2021.724989
Kiendrebeogo, M., Karimi Estahbanati, M. R., Khosravanipour Mostafazadeh, A., Drougi, P., and Tyagi, R. D. (2021). Treatment of microplastics in water by anodic oxidation: a case study for polystyrene. Environ. Pollut. 269, 116168. doi:10.1016/j.envpol.2020.116168
Kim, K. T., and Park, S. (2021). Enhancing microplastics removal from wastewater using electro-coagulation and granule-activated carbon with thermal regeneration. Processes 9, 617. doi:10.3390/pr9040617
Lebreton, L., and Andrady, A. (2019). Future scenarios of global plastic waste generation and disposal. Palgrave Commun. 5, 6. doi:10.1057/s41599-018-0212-7
Miao, F., Liu, Y., Gao, M., Yin, X., Xiao, P., Wang, M., et al. (2020). Degradation of polyvinyl chloride microplastics via an electro-Fenton-like system with a TiO2/graphite cathode. J. Hazard. Mat. 399, 123023. doi:10.1016/j.jhazmat.2020.123023
Nidheesh, P. V., and Rajan, R. (2016). Removal of rhodamine B from a water medium using hydroxyl and sulphate radicals generated by iron loaded activated carbon. RSC Adv. 6, 5330–5340. doi:10.1039/C5RA19987E
Oliveira, T. M. B. F., Ribeiro, F. W. P., Morais, S., de Lima-Neto, P., and Correia, A. N. (2022). Removal and sensing of emerging pollutants released from (micro)plastic degradation: strategies based on boron-doped diamond electrodes. Curr. Opin. Electrochem. 31, 100866. doi:10.1016/j.coelec.2021.100866
Ozyonar, F., and Karagozoglu, B. (2011). Operating cost analysis and treatment of domestic wastewater by electrocoagulation using aluminum electrodes. Pol. J. Environ. Stud. 20, 173–179.
Perren, W., Wojtasik, A., and Cai, Q. (2018). Removal of microbeads from wastewater using electrocoagulation. ACS Omega 3 (3), 3357–3364. doi:10.1021/acsomega.7b02037
Piazza, V., Uheida, A., Gambardella, C., Garaventa, F., Faimali, M., and Dutta, J. (2022). Ecosafety screening of photo-fenton process for the degradation of microplastics in water. Front. Mar. Sci. 8, 791431. doi:10.3389/fmars.2021.791431
Pollard, M., Hunsicker, E., and Platt, M. (2020). A tunable three-dimensional printed microfluidic resistive pulse sensor for the characterization of algae and microplastics. ACS Sens. 5, 2578–2586. doi:10.1021/acssensors.0c00987
Prata, J. C., da Costa, J. P., Lopes, I., Duarte, A. C., and Rocha-Santos, T. (2020). Environmental exposure to microplastics: an overview on possible human health effects. Sci. Total Environ. 702, 134455. doi:10.1016/j.scitotenv.2019.134455
Prata, J. C. (2018). Airborne microplastics: consequences to human health? Environ. Pollut. 234, 115–126. doi:10.1016/j.envpol.2017.11.043
Sharma, L., Prabhakar, S., Tiwari, V., Dhar, A., and Halder, A. (2021). Optimization of EC parameters using Fe and Al electrodes for hydrogen production and wastewater treatment. Environ. Adv. 3, 100029. doi:10.1016/j.envadv.2020.100029
Shen, M., Zhang, Y., Almatrafi, E., Hu, T., Zhou, C., Song, B., et al. (2022). Efficient removal of microplastics from wastewater by an electrocoagulation process. Chem. Eng. J. 428, 131161. doi:10.1016/j.cej.2021.131161
Shimizu, K., Sokolv, S., Katelhon, E., Holter, J., Young, N., and Compton, R. (2017). In situ detection of microplastics: single microparticle-electrode impacts. Electroanalysis 29 (10), 2200–2207. doi:10.1002/elan.201700213
Smith, M., Love, D. C., Rochman, C. M., and Neff, R. A. (2018). Microplastics in seafood and the implications for human health. Curr. Environ. Health Rep. 5, 375–386. doi:10.1007/s40572-018-0206-z
Sobhani, Z., Lei, Y., Tang, Y., Wu, L., Zhang, X., Naidu, R., et al. (2020). Microplastics generated when opening plastic packaging. Sci. Rep. 10, 4841. doi:10.1038/s41598-020-61146-4
Wang, S., Xu, M., Jin, B., Wünsch, U. B., Su, Y., and Hang, Y. (2022). Electrochemical and microbiological response of exoelectrogenic biofilm to polyethylene microplastics in water. Water. Res. 211, 118046. doi:10.1016/j.watres.2022.118046
Weber, R. S., and Ramasamy, K. K. (2020). Electrochemical oxidation of lignin and waste plastic. ACS Omega 5 (43), 27735–27740. doi:10.1021/acsomega.0c03989
Wu, W. M., Yang, J., and Criddle, C. S. (2017). Microplastics pollution and reduction strategies. Front. Environ. Sci. Eng. 11, 6. doi:10.1007/s11783-017-0897-7
Yu, C., Takhistov, P., Alocilja, E., Reyes de Corcuera, J., Frey, M. W., Gomes, C. L., et al. (2022). Bioanalytical approaches for the detection, characterization, and risk assessment of micro/nanoplastics in agriculture and food systems. Anal. Bioanal. Chem. 414, 4591–4612. doi:10.1007/s00216-022-04069-5
Zeboudji, B., Drouiche, N., Louinici, H., Mameri, N., and Ghaffour, N. (2013). The influence of parameters affecting boron removal by electrocoagulation process. Sep. Sci. Technol. 48 (8), 1280–1288. doi:10.1080/01496395.2012.731125
Keywords: microplastics, electrochemistry, oxidation, reduction, remediation, sensors, monitoring
Citation: Martic S, Tabobondung M, Gao S and Lewis T (2022) Emerging electrochemical tools for microplastics remediation and sensing. Front. Sens. 3:958633. doi: 10.3389/fsens.2022.958633
Received: 31 May 2022; Accepted: 04 August 2022;
Published: 26 August 2022.
Edited by:
Kagan Kerman, University of Toronto, CanadaReviewed by:
Zhuoqing Yang, Shanghai Jiao Tong University, ChinaMeissam Noroozifar, University of Toronto Scarborough, Canada
Copyright © 2022 Martic, Tabobondung, Gao and Lewis. This is an open-access article distributed under the terms of the Creative Commons Attribution License (CC BY). The use, distribution or reproduction in other forums is permitted, provided the original author(s) and the copyright owner(s) are credited and that the original publication in this journal is cited, in accordance with accepted academic practice. No use, distribution or reproduction is permitted which does not comply with these terms.
*Correspondence: Sanela Martic, c2FuZWxhbWFydGljQHRyZW50dS5jYQ==