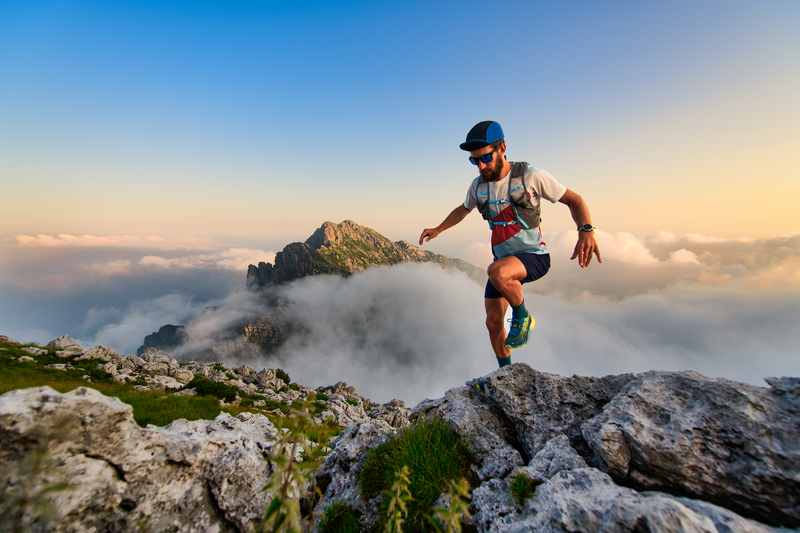
95% of researchers rate our articles as excellent or good
Learn more about the work of our research integrity team to safeguard the quality of each article we publish.
Find out more
REVIEW article
Front. Sens. , 31 May 2022
Sec. Biosensors
Volume 3 - 2022 | https://doi.org/10.3389/fsens.2022.789771
Conventional detection of cancer involves highly invasive and expensive diagnostic procedures, often leading to non-compliance from patients. Therefore, there is a strong requirement for the development of non-invasive techniques that can facilitate rapid and timely diagnosis of the disease. The tumor-immune interaction often leads to anomalous expression of different soluble immune signaling molecules like cytokines and chemokines, thus making them promising candidates for sensing disease development and progression. Furthermore, differential expression of soluble isoforms of several immune-checkpoint molecules like PD-L1, CTLA-4 etc., has been found to have strong correlation with tissue-specific tumor development, disease progression and in many cases, disease prognosis. Therefore, development of biosensors, to rapidly detect and analyze the levels of these soluble immune molecules in different body fluids, requiring minimal sample volume, has the potential to be a game-changer in the field of cancer diagnosis. In addition, real time monitoring of these soluble immune checkpoint molecules in patient-derived biofluids may serve as decision support tools for patient selection for immunotherapeutic interventions. Majority of the biosensors designed to detect the soluble immune biomarkers, have used a two-antibody based sandwich system to capture the target analyte. However, new technologies using bioreceptors like the aptamers or nano-yeast scFv antibody fragments have made possible multiplexed detection of several analytes simultaneously. The use of gold nanoparticles or carbon nanotubes on the electrode surface serves to increase the sensitivity of detection, due to their high electrical conductivity. Further, fabrication of the biosensors on microfluidic platforms enable the detection of these analytes at ultra-low levels. This review discusses the recent advances made in the development of biosensors for specific and selective detection of these immune-markers that can be successfully translated to the clinics as a new paradigm in disease diagnosis and monitoring.
Tumor development and progression is a complex interplay between immune system and cancer cells. The tumor microenvironment (TME) is uniquely shaped by tumor cells, stromal cells and immune-cell infiltrates, which negatively or positively regulate tumorigenesis (Lei et al., 2020). These infiltrating immune cells communicate with each other as well as the tumor cells via various immunoregulatory molecules such as cytokines that are intricately involved in cancer pathogenesis (Figure 1A). Cytokines are low molecular weight, soluble messenger proteins produced by the immune cells to facilitate crosstalk between themselves and surrounding cells. During an immune response against invading pathogens, cytokines participate in the concerted effort of the immune system to eliminate the threat, as well as mitigate inflammatory response once the immediate threat is overcome, and mediate repair of damaged tissue (Owen et al., 2013). Likewise, in the initial phase of cancer development, tumor growth is inhibited by various pro-inflammatory cytokines released by the tumor-infiltrating immune cells (Gonzalez et al., 2018). Eventually these neoplastic cells start producing cytokines that stimulate themselves in an autocrine fashion, as well as stromal cells and blood vessels to support cancer development. Notably, some pro-inflammatory cytokines that are predominantly involved in anti-tumor activities, can also have pro-tumor effects under specific conditions, like sustained release during chronic inflammation (Mojic et al., 2018). Cytokines released from the tumor cells help recruit and differentiate immunosuppressive cells like tumor-associated macrophages (TAMs) or Myeloid-derived suppressor cells into the TME, that establish an immunosuppressive milieu to counter the anti-tumor immune responses of various immune cells, like T cells, NK cells etc,. leading to successful immune evasion (Yang and Zhang, 2017; Pérez-Romero et al., 2020).
FIGURE 1. Schematic representation of biosensor-mediated detection of tumor-derived soluble immune molecules present in biofluids, for disease diagnosis and monitoring. (A) Interaction between tumor cells and T cells in the tumor microenvironment (TME) via membrane bound molecules (MHC: TCR; B7: CTLA4; PD-L1:PD1; CD40:CD40L), as well as soluble proteins like growth factors, cytokines and the soluble isoforms of immune-check point molecules (sPD-L1, sPD-1, sLAG-3, sCD40L). (B) These soluble immune molecules often escape the TME and are released in the circulation. (C) Due to their diagnostic as well as prognostic values, non-invasive or minimally invasive sampling of these soluble immune molecules, can lead to the development of novel biosensor based POCT devices for disease diagnosis and monitoring. For rapid and ultra-sensitive detection of these soluble immune molecules, various biosensors have been developed which mainly consists of three parts: (D) bioreceptors like monoclonal antibodies, aptamer (Wu et al., 2019; Yan et al., 2020), scFv fragments (Reza et al., 2019), enzymes (Nguyen et al., 2019), nucleic acid, nanobodies (Goode et al., 2016) and ligand (Niedziałkowski et al., 2021) that interacts with the target; (E) a transducer for the production of signals and (F) a signal amplification and processing unit for the quantification of the transduced signal.
In parallel, the checkpoints of the immune system, that guard against aberrant immune response to self, are now exploited by the tumor cells to bypass immune surveillance. The tumor cells often show high expression of several immune checkpoint molecules that are involved in the physiological role of T cell inhibition as part of peripheral immune tolerance mechanisms (Marcucci et al., 2017). For example, tumor cells in various tissue-specific cancer express Programmed Cell Death Ligand 1 (PD-L1), which interacts with its receptor PD-1 on T cells, to induce inhibition of activated T cells (Zheng et al., 2019) (Figure 1A). Furthermore, sustained signaling through PD-1 on T cells by the tumor cell-expressed PD-L1, often leads to an “exhausted” phenotype of the tumor-infiltrating T cell, leading to their de-activation inside TME (Blank and Mackensen, 2007). Importantly, some of these immune checkpoint molecules undergo alternative splicing to generate soluble isoforms that lack the membrane-anchors, which can then be released from the TME (Oaks et al., 2000; Nielsen et al., 2005). While the interplay between tumor cells and immune-checkpoint molecules is well established, the role of these soluble isoforms in cancer progression or immune-evasion is still a work-in-progress. Several studies have demonstrated the correlation of serum-levels of these soluble checkpoint molecules with cancer progression as well as prognosis, which has been reviewed extensively by our group (Chakrabarti et al., 2019) as well as others (Gu et al., 2018). These soluble immune checkpoint molecules, therefore, are good candidates as disease biomarkers. Given the intimate correlation of cytokines and soluble isoforms of the checkpoint molecules with cancer pathogenesis, it is important to integrate the detection of these molecules in different stages of disease progression, with conventional diagnostic modalities. Furthermore, the field of patient care in cancer is now steadily progressing to personalized therapeutic strategies, especially in the light of immunotherapeutic advancements (Kakimi et al., 2017), which necessitate a better understanding of the cancer-immune interplay. Parallel detection of several of these molecules in patient sera or other body fluids can reveal crucial information about disease pathogenesis, which in addition to having prognostic values; can be used as an endpoint marker for novel therapeutic interventions. Conventional and gold standard detection procedures like ELISA (Leng et al., 2008), to monitor the levels of multiple soluble immune molecules will be time-consuming and also will require large sample volumes, which may impediment their inclusion in routine clinical examinations. Therefore, it is imperative to design and fabricate highly specific biosensors that require minimal sample volumes, for their rapid detection in the serum as well as other bio-fluids. Such sensors have been developed by several groups, many of which have been tested extensively using patient-derived fluids, or validated with bio-mimicking fluids. A schematic representation of the different components of biosensor-mediated detection of tumor-derived soluble immune markers is given in Figure 1. Briefly, cytokines or soluble immune checkpoint molecules are produced in the tumor microenvironment as a result of tumor-immune interactions (Figure 1A). These soluble immune molecules can be released into the circulation and therefore are easily traceable in the serum and other body fluids (Figure 1B) facilitating non-invasive sampling of the target biomarkers for biosensor-based detection and quantification (Figure 1C). The various biosensors designed for such detection use different types of bioreceptors [antibodies, aptamers, nanobodies, single-chain variable fragments of antibodies (scFv), enzymes, nucleic acids, receptors or ligands and proteins] for highly specific capture of target analyte (Figure 1D). The signal derived from the interaction of the bioreceptors with the target analyte is then transduced by different types of electrochemical, optical or mechanical transducers (Figure 1E), to a signal processing unit for quantitative interpretation of the interaction thereby computing the levels of the target analyte in the sampled biofluid (Figure 1F). The different types of bioreceptors and transducers have been discussed in details in later sections.
Several groups have extensively reviewed biosensor-based detection of several disease-relevant cytokines irrespective of the pathologies (Liu et al., 2016). These biosensors can be electrochemical (Dutta et al., 2021), optical (Singh et al., 2017), paper based electrochemical (Loo and Pui, 2020), or even a combination thereof (Chen et al., 2015; Razmi et al., 2018; Khan and Mujahid, 2020). In the current review, we present a focussed discussion of biosensor-mediated detection of cytokines, either individually or multiplexed, that have been unequivocally implicated in cancer pathogenesis. Only those biosensors that have been additionally validated in patient-derived samples have been chosen for discussion, to specifically highlight their efficacy in real-life situations. In addition, the current review also, for the first time, presents a detailed account of biosensor-mediated detection of soluble immune checkpoint molecules involved in cancer pathogenesis and immune evasion in an integrated manner. These molecules, either alone or as part of a panel, provide important diagnostic and prognostic clues, and can also be used for monitoring the efficacy of evolving immunotherapeutic strategies. An in-depth information about the biological significance of different soluble immune checkpoint molecules described in this review, will provide crucial impetus to research groups working on the development of ultra-sensitive biosensors for diagnostic purposes. At the same time, this review will also reveal a new diagnostic paradigm to clinicians, thus facilitating the inclusion of bio-sensing devices to clinical diagnostic and disease-monitoring approaches. Such complementary and interdisciplinary discussions are required for smooth transition of these devices from bench to bedside.
To this end, the current review presents a comprehensive account of different strategies to detect and monitor the cytokines and soluble immune-checkpoint molecules that can be applied in point of care testing (POCT) for clinical diagnosis.
Biosensors have emerged in the field of medical research as an alternative to the conventional diagnostic techniques. These analytical tools consist of a bio-recognition molecule that identifies and binds to the target (analyte), and a transducer for signal production followed by a signal-processing unit (Bhalla et al., 2016). While the signal processing unit is responsible for processing and amplification of transduced signals and their further conversion into digital form for quantification and interpretation of output data (Figure 1F); the major considerations that need to be undertaken during fabrication of a biosensor, are the transducers and the bio-receptors.
A transducer is a fundamental part of biosensor that converts biological interactions into a signal. Based on the transducer type, a biosensor can be generally categorized as electrochemical, optical and mechanical (Figure 1E). Electrochemical biosensors are the most extensively used biosensing platforms that are rapid, inexpensive, and small-sized portable devices (Hosu et al., 2018; Cho et al., 2020; Dutta et al., 2021). Amongst all other biosensing techniques, electrochemical biosensors are generally the most sensitive and can produce lower Detection limits (DLs) by utilizing smaller sample volume, and their efficiency remains unaltered by sample turbidity. However, the electrochemical biosensors suffer from limited shelf life (Menon et al., 2020). Depending on the mode of detection, electrochemical biosensors can be further classified as amperometric, voltammetric, impedimetric potentiometric or Field-effect transistors (FET) type (Menon et al., 2020) (Figure 1E). Notably, till date most cytokines have been detected by electrochemical biosensors (Berggren et al., 1998; Crowley et al., 1999; Bart et al., 2005). On the other hand, in a biosensor, interaction of the bio-receptor with target analytes leads to several changes in the optical field parameters such as phase, polarization, or frequency. These changes can be exploited in optical biosensing strategies (Purohit et al., 2020). Based on transduction mechanisms, optical biosensors can be grouped as fluorescence, luminescence, colorimetric, surface enhanced Raman scattering (SERS) and Surface Plasmon Resonance (SPR) based biosensors (Figure 1E). These biosensors have been used in the detection of different targets like pathogens, toxins, cancer biomarkers (Damborský et al., 2016) in addition to cytokines (Li et al., 2017; Alba-Patiño et al., 2020; Oh et al., 2014). Finally, mechanical biosensors analyze the mechano-physical changes, occurring due to the biomolecular interactions on the transducer surface (Tamayo et al., 2013). It provides certain advantages over other biosensing techniques, which include analyte detection in as low as zeptogram levels due to improved mass resolution and bioaffinity (Braun et al., 2009). In addition, it exhibits rapid, label-free and real-time detection of a wide range of analytes in both liquid and gaseous phases (Purohit et al., 2020). Based on their mechanical transduction methods, these biosensors can be classified as quartz microbalance, surface acoustic wave, and nano-mechanical systems (Figure 1E). Mechanical biosensors have shown great potential in the detection of different targets of cancer, genetic mutation and protein biomarkers of different diseases (Purohit et al., 2020) as well as cytokines (Krishnamoorthy et al., 2008; van den Hurk and Evoy, 2013; Pohanka, 2018).
While fabricating a biosensor, it is important to consider the bioreceptor component as well. Depending upon the type of biorecognition molecules used, a biosensor can be grouped as immunosensors (antibody-based or receptor/ligand-based), aptasensors and enzyme-based sensors (Figure 1D). Immunosensors take into account the antibody-antigen interaction for the detection of target analyte. Monoclonal antibodies (mAbs) are the most extensively used bioreceptors, and have been used for detection of cytokines for a long time (Ward et al., 1992). However, smaller antibody fragments have higher antigen-sequestering capacity and sensitivity in comparison to full-length antibodies (Figure 1D). To that end, monomeric Fab’ fragments were first reported as bioreceptors for detection of tumor necrosis factor-α (TNF-α) in a bioluminescent immunoassay (Erikaku et al., 1991). More recently, the discovery of single domain antibodies derived from the heavy-chain only antibodies of Camelids have garnered much attention in the field. These “nanobodies” facilitate easier chemical coupling and immobilization on the sensor surface and their small paratope enables interaction with less accessible epitopes when compared to full-length antibodies or smaller antibody fragments (Figure 1D). A nanobody-based impedimetric immunosensor was reported to detect IgG from rabbit sera (Goode et al., 2016). Furthermore, engineered antibody fragments like single-chain variable fragment (scFv) antibodies have also been used as bioreceptors for the detection of multiple soluble immune-checkpoint molecules like sPD-1, sPD-L1, & sLAG-3 (Figure 1D) (Reza et al., 2019).
Apart from the antibodies, recently the aptamers which are short, single-stranded, 20–100 nucleotides long DNA or RNA sequences, are garnering much attention as bioreceptors. (Figure 1D). These oligonucleotides possess stable tertiary structures, and can be designed to bind a broad range of targets with high specificity and affinity. Ligand-specific aptamers are selected from an initial pool consisting of a large number of oligonucleotides, based on their affinity towards the target molecule. This is then followed by elution of the bound candidate oligonucleotides and amplifying them using PCR. This enriched pool is subjected to the same selection steps repeatedly for several rounds to select the best binders. Using this Systematic Evolution of Ligands by Exponential Enrichment or SELEX procedure, both DNA and RNA aptamers with strongest possible affinity to the target molecule can be selected (Lakhin et al., 2013; van den Kieboom et al., 2015). Importantly, the affinity of these aptamers towards the target molecules is comparable to that of the antibodies. Using aptamers as the bioreceptors, several biosensors termed aptasensors, have been designed for the detection of different target proteins. For example, platelet-derived growth factor (PDGF), a neuroinflammatory biomarker, has been detected in an electrochemical impedance spectroscopy (EIS)-based aptasensor (Liao and Cui, 2007). Despite the advantages, aptamers are not as commonly used as antibodies as yet, and needs to be used more frequently as the bioreceptor of choice while fabricating biosensors, for more sensitive and specific detection of analytes. In enzyme-based biosensing techniques, the catalytic biochemical reaction between enzyme and substrate is transduced into measurable signals (Nguyen et al., 2019). High specificity of interaction between enzyme and substrate is the major advantage of enzyme-based biosensors. An enzyme-based amperometric biosensor was prepared using three enzymes namely, cholesterol esterase, cholesterol oxidase, and peroxidase for estimation of cholesterol levels in the serum of healthy individuals and patients at risk of cardiovascular disease (Lata et al., 2016). Apart from this, ligand molecules have also been used as bioreceptors for the detection of analyte in the sample (Figure 1D). For example, a small molecule inhibitory ligand, BMS-8, has been used in the fabrication of biosensor for the detection of soluble PD-L1 (Niedziałkowski et al., 2021).
Over the last several decades, biosensors have shown immense potential for the detection of diverse disease-relevant biomolecules. These include detection of cancer biomarkers like Prostate-specific antigen (PSA) in prostate cancer or HER2in breast cancer (Loo et al., 2011; Akbari jonous et al., 2019), or nucleic acid targets in infectious diseases like dengue (Baeumner et al., 2002) and tuberculosis (Ramos-Sono et al., 2020). Biosensors have also been fabricated to detect amyloid-beta derived diffusible ligands (ADDLs), a biomarker for Alzheimer’s disease (Haes et al., 2005) as well as autoantibodies in Rheumatoid Arthritis, an autoimmune disease (Guerrero et al., 2020). Moreover, biosensors to detect proinflammatory cytokines like IL-6, which is involved in the pathogenesis of life-threatening conditions like sepsis (Russell et al., 2019) and more recently in COVID-19 pathogenesis (Adrover-Jaume et al., 2021), has been developed as well.
In the current era of molecular medicine and personalised therapy, the relevance of detecting disease-specific biomarkers in patients and at different stages of disease progression is increasing. Biosensors, being ultrasensitive tools for detection of target molecules, need to be extensively tested in clinical samples and in different body fluids before being included as part of diagnostic modalities or for POCT. In the following section, a detailed and comparative account of biosensors for detection of relevant cytokines (single or multiplexed) in cancer patient-derived samples has been presented. Additionally, given the importance of these soluble immune checkpoint molecules in cancer pathogenesis, progression and prognosis, a comprehensive discussion about the biosensors capable of evaluating these molecules in bio-mimicking fluids is given below. The general construction of some of the key types of biosensing strategies has been depicted in Figure 2.
FIGURE 2. Schematic representation of the general biosensing strategies that are employed in the detection of cytokines and soluble immune-checkpoint molecules involved in cancer pathogenesis. (A) Most of the biosensors fabricated for the detection of cytokines have utilized the sandwich antibody-based biorecognition system. The detection antibodies are conjugated with various reporter molecules like enzyme labels (Chikkaveeraiah et al., 2009; Chikkaveeraiah et al., 2011; Valverde et al., 2020), metal ions (Yuan et al., 2015) or redox labels (Sardesai et al., 2011; Sardesai et al., 2013) for quantification of target proteins by electrochemical (Chikkaveeraiah et al., 2009; Chikkaveeraiah et al., 2011; Yuan et al., 2015; Valverde et al., 2020) or electro-chemiluminescence (Sardesai et al., 2011; Sardesai et al., 2013) methods. This sandwich-type detection approach has also been incorporated with surface-enhanced Raman scattering (SERS)-based immunoassay (Wang et al., 2019), where the electrode modified with SERS substrate and capture antibody, forms a sandwich structure with the SERS tag labelled detection antibody. (B) In contrast, single biorecognition based biosensors consist of the detection of the target protein by monoclonal antibodies (Lin et al., 2015), single-stranded nucleic acids (Pan et al., 2017) or target-specific small molecule ligands (Niedziałkowski et al., 2021), that are immobilized on the sensor surface. The common detection techniques used in such single antibody-based systems are electrochemical like DPV (Lin et al., 2015; Pan et al., 2017) or EIS (Niedziałkowski et al., 2021). (C) Lateral flow aptasensors for the detection of cytokines have been fabricated such that on the sample zone, either the analyte containing the target protein is added, or a biotinylated target-specific aptamer captures the free target. These complexes then flow to the conjugation zone where the biotinylated aptamer-target conjugate binds to a detection antibody complex or the free target binds to a capture aptamer-labelled SERS tag. Streptavidin or streptavidin-conjugated biotinylated detection aptamers are immobilized on the test zone, to which the aptamer: target complexes flow and bind, which can be visualized instantly due to the presence of reporter molecules (Cheng et al., 2020; Cao et al., 2021). The dotted lines in the figure depict the different studies that have used similar bio-sensing principles. The different biosensing strategies illustrated here have been further collated in Table 1.
Several cytokines have been shown to play important role in cancer development as well as immune evasion by cancer cells. While IL-2 mediates antitumor immunity by promoting the recruitment and activation of natural killer (NK) cells and tumor-specific cytotoxic T lymphocytes (CTLs), cytokines like IL-4, IL-10, TGF-β, etc.,. have been found to promote tumor growth and progression (Germano et al., 2008; Setrerrahmane and Xu, 2017). Interestingly IFN-γ, a proinflammatory cytokine with well-established anti-tumor activities, is found to exhibit pro-tumor effects as well, especially under sustained and chronic expression in the tumor microenvironment, when it selects for more aggressive tumor cells (Mojic et al., 2018). Only a few studies have employed the biosensors to detect the levels of different cytokines like IL-6, IL-8, Tumor Necrosis Factor-α (TNFα), Vascular Endothelial Growth Factor (VEGF) and Platelet-derived growth factor (PDGF) in cancer patient-derived sera, thus being validated in real-life scenarios.
Among the various cytokines involved in the tumor-mediated immune evasion, IL-6 is perhaps the best studied. Produced in the tumor microenvironment, IL-6 favors tumor growth and proliferation by promoting inhibition of apoptosis, tumor cell survival, angiogenesis and metastasis. Increased serum levels of IL-6 has been reported in different cancers like prostate cancer (Nakashima et al., 2000), colorectal carcinoma (Xu et al., 2016), and head and neck squamous cell carcinomas (HNSCC) (Riedel et al., 2005), thereby making it a highly relevant candidate for disease monitoring in cancer patients. Biosensor mediated detection of IL-6 in serum samples of patients with various tissue-specific malignancies can provide crucial information for early disease diagnosis as well as help monitor disease progression. Moreover, detection of IL-6 in different stages of the disease can lead to the better understanding of the role of this proinflammatory cytokine in cancer progression. While several biosensors have been designed and fabricated for the detection of IL-6 in biomimicking fluid samples, few have also been validated at the patient level.
Most (4 out of 5 studies collated in Table 1) of the IL6 specific biosensors employ a sandwich-based assay, where the analyte is sandwiched between a capture antibody that is attached to the sensor surface, and an enzyme-labeled detection antibody (Figure 2A). Electrochemical transducers can detect the formation of this ternary complex on the basis of the enzyme activity, and the signal generated is most commonly detected by amperometric methods. The primary capture antibody for IL-6 can be adsorbed on densely packed single-walled (SWNT) or multi-walled nanotubes (MWNT), forming nanoforests. The advantages of these carbon nanotube forests are that they are cheap, easy to manufacture, and offer a highly conductive platform for immunosensing. Such a SWNT-forest based immunosensor has been used to detect IL-6 in serum samples of prostate cancer patients with a DL for IL-6 (30 pg/mL) along with other prostate cancer specific biomarkers (Chikkaveeraiah et al., 2009). While the normal range of IL-6 in healthy controls is ∼2.91 ± 6.45 pg/mL (Kim et al., 2011), in primary head and neck squamous cell carcinoma patients it increases up to approximately 3-fold (Riedel et al., 2005). However, in prostate cancer patients, the increase is not as dramatic and has been recorded as 5.6 ± 6.7 pg/ml (Mean ± Standard deviation) as seen in a cohort of patients of mean age 74.5 years (Michalaki et al., 2004). The DLs of such SWNT-based sensors were the same with laboratory samples (Munge et al., 2009) and could achieve a modest 3-fold betterment of the DL with similar samples when s, densely packed glutathione modified gold-nanoparticles were used instead of SWNT (Munge et al., 2009). Increasing the number of enzyme labels on the secondary antibody, resulted in further increase in sensitivity of the IL-6 sensor. Conjugation of the secondary antibody to MWNTs containing 106 horse-radish peroxidase (HRP) molecules per 100 nm, increased the sensitivity drastically and showed a 60-fold lower DL of 0.5 pg/mL in calf serum as well as HNSCC cell lines (Malhotra et al., 2010), thus providing a breakthrough for ultra-low detection of IL-6. Finally, disease-relevant ultra-low detection limit of IL-6 (0.3 pg/ml) in prostate cancer patient sera could be achieved with an electrochemical biosensor fabricated on a microfluidic platform. The microfluidic system uses polydimethylsiloxane (PDMS) channels connected to a pump and sample injector. High concentration of analyte-specific antibodies were conjugated to superparamagnetic beads labeled densely with HRP molecules for high-efficiency off-line capture of IL-6. The bead-antibody bioconjugate was next captured by a second set of antibodies immobilized on an 8-electrode microarray system composed of glutathione-modified Au-NPs on the nano-structured electrode surface. Importantly, this device could simultaneously detect both IL-6 and PSA from the serum of prostate cancer patients in a short period of time; with the results showing excellent correlation with the more time-consuming ELISA (Chikkaveeraiah et al., 2011). Such microfluidic devices can therefore be envisioned for highly sensitive multiplexed detection of multiple cancer biomarkers, thus presenting a better holistic picture of the disease pathogenesis and progression, with possible prognostic values. In addition to the electrochemical biosensors, electro-chemiluminescence (ECL) based IL-6 sensors have also been devised where; a pyrolytic graphite chip was fabricated with microwells coated with SWNT and capture antibodies. Upon target binding, secondary antibody conjugated with RuBPY-silica labels are added for sandwich-based detection of the analytes. The electrochemical oxidation of tripropylamine in the microwells results in the generation of photoexcited [Ru(bpy)32+]* that emits light at 610 nm, which is then captured by a CCD camera. This non-microfluidic ECL-based biosensor was fabricated for multiplexed detection of IL-6 and PSA from prostate cancer patient sera, and had a DL of 0.25 pg/mL for IL-6 and 1 pg/mL for PSA (Sardesai et al., 2011). Built on the same principle, a similar carbon nanotube based ECL sensor was built on a microfluidic device, where the ECL was constructed in analytical microwells fabricated onto three PDMS channels. This device could achieve ultralow DL of 10 fg/mL for IL-6 when tested with pooled serum samples from prostate cancer patients (Sardesai et al., 2013).
TABLE 1. List of biosensors in detection of soluble immune molecules such as cytokines and soluble immune checkpoint molecules as biomarkers in cancer, in either patient derived fluids or biomimicking fluids.
The microfluidic based ECL sensor has multiple advantages over the non-microfluidic ECL device. In comparison to the non-microfluidic sensor, the microfluidic device is highly sensitive displaying a 25-fold lower DL than the non-microfluidic sensor. Moreover, it is significantly faster and could carry out rapid detection of the analytes in a period of 1.1 h, which the non-microfluidic array took about 3 h to complete. In addition to improved speed of detection, the microfluidic device could also work with smaller reagent volume and could easily detect the analyte in highly diluted samples, thus requiring much smaller sample volume as well. It is to be noted that both electrochemical and ECL based biosensors are especially suited for multiplexed detection of multiple analytes. While the electrochemical biosensors require multi-electrode chips for simultaneous detection of biomarker panels, ECL-based sensors do not require such multi-electrode chips and therefore may be cheaper to fabricate.
Apart from antibodies as biorecognition elements, DNA-aptamers have been used to fabricate a biosensor, which could detect IL-6 in colorectal cancer patient serum with a DL of 1.66 pg/mL (Tertis et al., 2019). This label-free aptasensor was developed based on electrochemical impedance technique using a glassy carbon electrode (GCE) modified with p-aminobenzoic acid, p-aminothiophenol and gold nanoparticles (AuNPs). The IL-6-specifc aptamer was thiolated to aid its immobilization on the modified electrode surface via gold-sulfur bonding. This method provides large surface area and active sites for immobilization of IL-6 specific aptamers. Such aptasensors are comparable in performance with the immunosensors, in addition to being cheaper to produce, without the need of production of monoclonal antibodies using hybridoma technology. Therefore, the application of aptasensors to detect multiplexed targets from patient sera and other biofluids need to be investigated in-depth.
In addition to IL-6, IL-8 also promotes growth, survival, angiogenesis and migration of endothelial and cancer cells in tumor environments (Waugh and Wilson, 2008), and can be detected in high levels in serum samples of breast cancer (Kozłowski et al., 2003) and oral cancer patients (Punyani and Sathawane, 2013). Due to the involvement of IL-8 in cancer pathogenesis as well as in other inflammatory conditions, few studies have ventured to detect and quantify this cytokine in human sera using biosensors. However, in contrast to the electrochemical or ECL-based signal transduction, these studies have utilized sandwich type SERS as their biosensing strategy. One such surface-enhanced Raman scattering immunoassays has used highly branched gold nanoparticles (AuNP) as the SERS substrate, on which the capture antibody is immobilized. The analyte (IL-8) is sandwiched between this SERS substrate and detector antibody-conjugated gold nanocages, which act as the SERS tag (Wang et al., 2019) (Figure 2A). Diatom biosilica or bimetallic Ag-Au surface has also been used as the SERS substrate to which the capture antibody was immobilized. Detection antibodies tagged with gold nanoparticles that bound to the captured IL-8 on the SERS substrate, constituted the SERS tag or reporter (Kamińska et al., 2017a; Kamińska et al., 2017b). Although all three studies could detect IL-8 from serum samples with almost similar detection limits (approx. 6–7 pg/mL), only the first one used gastric cancer patient and breast cancer patient sera in addition to healthy controls to validate the efficacy and range of detection. Using this sensor, the study found a serum concentration of 115 pg/mL, 708 pg/mL, and 437 pg/mL in healthy, breast cancer and gastric cancer patients respectively, which correlated very well with ELISA data (Wang et al., 2019). SERS-based multiplexed analysis of a panel of cytokines in a microfluidic platform, did not affect the limit of IL-8 detection (Kamińska et al., 2017b).
SERS is a powerful tool in the field of nanosensing, the sensitivity of which depends mostly on the substrate materials, which can be composed of noble metals or new nanocomposite materials and carbon-based nanomaterials like carbon quantum dots or carbon nanotubes. Therefore, the applicability of these more efficient SERS substrate in detecting the cancer-specific cytokines need to be investigated in depth.
Tumor Necrosis Factor-α (TNFα) is a pleiotropic cytokine that is known to exhibit anti-tumor activity by inducing tumor cell death and therefore has been used as a potential therapeutic molecule in cancer treatment. However, contrasting reports about its ability to promote tumor cell growth and proliferation and to induce angiogenesis and tumor metastasis has also been noted (Wang and Lin, 2008). Elevated levels of TNF-α are associated with lymphocytic leukemia (Ferrajoli et al., 2002) and prostate cancer (Michalaki et al., 2004). Importantly, decrease in serum levels of TNF-α has been noted in breast cancer patient’s post-chemotherapy (Berberoglu et al., 2004). Thus, it is an ideal candidate not only as a specific biomarker for hematological malignancies as well as solid tumors, but also as a readout of therapeutic efficacy of different treatment modalities. For the quantification of TNF-α, sandwich-type electrochemical biosensors using magnetic microbeads (MBs) have been the choice of the field. Sandwich type amperometric biosensors using magnetic MBs showed moderately low limits of detection (40 pg/mL) for IL-8 in serum-mimic solution (Bettazzi et al., 2013). Modification of the MBs with carboxyl group for capture-antibody immobilization, led to further refinement of the sensing technique and lowering of DL by almost 8-fold (5.8 pg/mL in spiked serum) (Eletxigerra et al., 2014). However, the best sensitivity was achieved with neutravidin-functionalized magnetic MBs and biotinylated capture antibodies that were set-up on screen-printed carbon electrodes (Valverde et al., 2020). Upon target binding to the capture antibody, HRP-labelled detector antibodies bind to form a ternary immunocomplex. The HRP label provide the required electrochemical signal in presence of the substrate H2O2. This platform produced fast and highly sensitive simultaneous detection of TNF-α and another cancer biomarker, Receptor Activator of Nuclear Factor-κB Ligand (RANKL) in serum from breast cancer patients, and had a DL of 3.0 pg/mL for TNF-α and 2.6 pg/mL for RANKL. Importantly, this sensor could detect both analytes within diagnostically relevant ranges of 40 and 48 pg/mL in HER2 negative and positive breast cancer patients respectively, in comparison to 11–14 pg/mL for healthy individuals. Similar to TNF-α, the RANKL readings in the different patient and healthy control cohorts were comparable to the gold-standard ELISA readings (Valverde et al., 2020). Thus, neutravidin-MBs provide a highly sensitive platform for the detection of analytes in patient sera at the picrogram level, especially for simultaneous detection of target proteins.
Vascular Endothelial Growth Factor (VEGF), a highly potent proinflammatory cytokine and a well-known inducer of angiogenesis, is exploited by tumor cells to form new blood vessels in and around the tumor for optimal supply of nutrients and oxygen to the proliferating tumor cells (Carmeliet, 2005). VEGF is a rational biomarker of most cancers and its upregulated expression has been observed in gastric cancer (Wang et al., 2016) and prostate cancer (Botelho et al., 2010). For the detection of VEGF from patient sera, graphene oxide (GO) based electrochemical biosensors have been used in all the studies, where either the anti-VEGF monoclonal antibody Avastin (Bevacizumab) (Lin et al., 2015), or VEGF-specific single stranded DNA (ssDNA) molecules (Pan et al., 2017) have been used as bioreceptors (Figure 2B). An aqueous solution of Avastin-magnetic graphene oxide (MGO) was applied on a gold electrode platform in the presence of a magnetic field, and the concentration of VEGF was measured by differential pulse voltammetry (DPV). This strategy demonstrated a DL of 31.25 pg/mL for VEGF in the plasma of brain tumor patients. Apart from the specificity and sensitivity, a unique advantage of this biosensor was its reusability (the activity was retained even after 50 uses), which makes it significantly cost-effective (Lin et al., 2015). Additionally, the detection ability of this sensor was well within diagnostic range (49–64 pg/mL in healthy controls versus 167–196 pg/mL in brain tumor patients). On the other hand, the ssDNA bioreceptor was immobilized on Au-electrode modified with GO as part of a sandwich system to capture the VEGF, which was further detected by detection antibodies coated on poly-L-lactide nanoparticles (PLLA NPs), and quantitated by DPV. The DL for VEGF was around 50 pg/mL in prostate cancer patient sera, when detected simultaneously with PSA (Pan et al., 2017), and could detect a concentration of 180–198 pg/mL VEGF in three prostate cancer patient sera, which falls in the diagnostic range. While both the biosensors developed for the detection of VEGF, showed pico-gram level DLs, it is intriguing to note the lower DL in the avastin-based system in comparison to the sandwich-based two-antibody system. The latter system may therefore need additional modification of detection strategy to increase its sensitivity. Furthermore, the DL of the VEGF biosensor, could be drastically lowered to ultra-low levels (6 fg/mL), by using reduced GOs coupled to AuNPs, which increased the conductivity of the electrode (Pourmadadi et al., 2020). However, this sensor has not been tested in clinical samples and therefore needs further investigations.
Platelet-derived growth factor (PDGF) promotes cancer development and progression by modulating cell growth, angiogenesis, metastasis and apoptosis. Increased expression of PDGF has been observed in breast cancer (Ariad et al., 1991) and esophageal cancer (Krzystek-Korpacka et al., 2011) Several groups have independently developed lateral flow aptasensors (LFAs) for the detection of PDGF in spiked serum samples (Liu et al., 2019) or patient sera (Cheng et al., 2020; Cao et al., 2021). LFAs have gained attention owing to their rapid, cost-effective and user-friendly properties. Detection of PDGF-BB in breast cancer patient serum was done using a LFA, where the target molecule is recognized by biotinylated aptamers present on the sample pad. The aptamer: PDGF-BB complex then migrates to the conjugate pad (CP) A, where it binds to a poly thymine-Cy3-AuNP-anti-PDGF-BB antibody complex. This aptamer: PDGF-BB: Cy3-AuNP-Ab ternary complex then migrates to CP B where it is coupled with AuNP-anti-Cy3 antibody. Finally, the captured analyte complex binds to streptavidin (SA) immobilized on the test zone of the biosensor, via the biotin-conjugated aptamer, to produce a visible red line. The antibodies that did not bind to PDGF-aptamer complexes migrate forward and are captured by the polyclonal anti-mouse antibodies immobilized on the control line. This biosensor exhibited a DL of 1 ng/mL in an assay time of 10 min (Cheng et al., 2020) (Figure 2C). The organization of the LFA was further improved by including a SERS-tag in the conjugate pad leading to lower DL (3.802 pg/mL) for PDGF-BB (Cao et al., 2021). This DL could be of diagnostic significance, as the serum concentration of PDGF level in healthy individual is ∼1.5 to 0.8 ng/mL (Kim et al., 2011). Plasma samples of prostate cancer patients were tested for simultaneous detection of thrombin and PDGF, where two different SERS tags (Au–Ag hollow nanoparticles) coated with target specific aptamers were used. The SERS tag: target complex was then individually captured on biotinylated target-specific aptamers immobilised on two separate test lines. Upon formation of a sandwich complex between the aptamers and the target on the test zone, a blue band was displayed. While more sensitive than the LFA, this LFA-SERS sensor required a slightly longer assay time of 30 min (Cao et al., 2021) (Figure 2C). The rapid assay times and user-friendliness of the LFAs make them an attractive method for the detection and quantification of other soluble biomarkers in cancer.
Due to the lack of regular testing of cytokine levels in different disease conditions or healthy individuals, as well as extreme variability in the cytokine levels studied, there are no established “normal” range or thresholds for these molecules. The cytokines are expressed at an almost undetectable level in healthy individuals under normal physiological state, but anomaly in their levels occur during various disease conditions, that range from pico-gram to nano-gram levels. The DLs of most of the biosensors (9 out of 11 studies on single cytokine detection collated in Table 1) used to detect the different cytokines in patient samples or laboratory samples, are most often at the pico-gram level and therefore can be considered as physiologically relevant. Additionally, some of the biosensors described here are able to detect ultra-low levels of cytokines in femto-gram range. The utility of such low-level detection in terms of disease-relevance need to be evaluated from a clinical perspective. Detection of such infinitesimal changes in cytokine levels and its implication in health and disease may open up new windows of opportunity for therapeutic and diagnostic purposes. The extreme patient-specific variations noted in several studies can be due to different parameters including stage of the disease and tissue specificity. Also, cohort age and ethnicity may have impact on the cytokine levels due to the differences in immune status and genetic polymorphisms. Therefore, in-depth investigations and validation of these biosensors considering the above-mentioned parameters are required, which will further help in the translation of these biosensors in the clinical settings, so that they can substitute for the conventional time-consuming diagnostic tests. Additionally, given the exclusive role the cytokines play in shaping the tumor microenvironment, it is essential to study the cytokine profile of the TME in its entirety to further understand the tumor milieu, which may lead to development of novel diagnostic and therapeutic strategies.
Moreover, it has been observed that most of the above-discussed biosensors for cytokine detection have adopted the two-antibody based sandwich approach to achieve signal amplification and lower DLs (Chikkaveeraiah et al., 2009; Chikkaveeraiah et al., 2011; Sardesai et al., 2011; Sardesai et al., 2013; Wang et al., 2019; Valverde et al., 2020) (Figure 2A) (Table 1). Despite the specificity, the use of multiple antibodies may increase the overall cost of biosensor production. In contrast, for VEGF quantification a single antibody-based approach was utilized for analyte recognition with comparable sensitivity as the two-antibody based systems (Lin et al., 2015) (Figure 2B). Given the high-cost of production of monoclonal antibodies, this single-antibody based strategy should be investigated further for development of more cost-effective biosensors with minimal resource requirement. In addition, multiplexing the sensor devices to detect the whole gamut of cytokines involved in the process of tumorigenesis will provide important clues to the disease pathogenesis, and may also lead to the discovery of new therapeutic targets. Furthermore, for the establishment of the biosensors as a reliable diagnostic tool in the POCT, improvisations are needed to develop kit-based platforms like LFA that can offer rapid diagnosis and instant visual results in a user-friendly manner. The cost-effectiveness, portability and ease of use makes the LFA-based biosensors highly promising that can be made available for POCT even in less accessible remote areas.
In order to be activated and differentiate into effector cells, T cells must receive three distinct signals from the antigen-presenting cells (APCs) with encoded instructions for activation. The first is triggered by the interaction of the T cell receptor (TCR) with its cognate peptide-MHC complex on the APC. This is followed by costimulatory signals provided by receptor-ligand interactions on the T cell and APC respectively, which acts as T cell activation signal. The third signal is provided by the cytokines secreted from activated APCs, which instruct the T cell on the differentiation status, post activation (Smith-Garvin et al., 2009). Notably, peripheral tolerance mechanisms of the immune system ensure the downregulation of activated T cells, by expressing several inhibitory receptors on the T cells, thereby protecting self from damage due to sustained T cell activity. Therefore, these receptors, along with their ligands expressed on APCs, serve as the checkpoints to maintain immune homeostasis (Mahoney et al., 2015).
In case of tumor cells, APCs infiltrating the tumor acquire tumor-associated antigens and present them to T cells in the draining lymph nodes (LN) to drive their activation. These activated T cells will then leave the LN and home to the tumor site, thus infiltrating the tumor in a fully activated form, ready to take out these abnormal cells from the system (Slaney et al., 2014). As part of immune evasion strategies, tumor cells often over-express checkpoint molecules, such as the T cell inhibitory ligand PD-L1. PD-L1 interacts with the inhibitory receptor programmed cell death protein-1 (PD-1) expressed on activated T cells. This interaction results in the de-activation of the infiltrating anti-tumor T cells or leads them towards an exhausted phenotype (Yamamoto et al., 2008; Jiang et al., 2015; Zahm et al., 2018). These “exhausted T” cells become non-responsive towards the tumor antigen-bearing cells, thus helping tumor cells to successfully evade anti-tumor immune responses (Iwai et al., 2002). Notably, immune checkpoint molecules have been found to undergo alternative splicing to generate soluble isoforms, which are then released into the serum and possibly other body fluids (Gu et al., 2018). These soluble isoforms have often been correlated to disease prognosis in cancer (Chakrabarti et al., 2019), thereby making them valuable biomarkers for screening and monitoring disease progression.
Soluble isoform of PD-L1 (sPD-L1) is reportedly detected in the serum of patients with malignant melanoma (Zhou et al., 2017) and nasal NK/T-cell lymphoma (Nagato et al., 2017) and an elevated serum concentration is associated with poor prognosis (Okuma et al., 2017). Hence, sPD-L1 may act as a biomarker in predicting the aggressiveness of tumor and outcome of therapy. In contrast, increase in serum concentration of sPD-1 is linked with increased rate of patient survival in non-small cell lung cancer (NSCLC) (Sorensen et al., 2016). sPD-1 may bind to the membrane associated PD-L1 on the tumor cell and block its interaction with PD-1 on T cells, thus restoring the T-cell mediated anti-tumor activity. Therefore, in-depth investigation of the levels of both sPD-1 and sPD-L1 in different cancers at different stages can offer important insights into their role in cancer-immune cross talk.
Given the importance of the sPD-1 and sPD-L1, a few immunosensors have been developed to detect these molecules. While most have not yet been tested in patient samples, these biosensors present a necessary first step towards integrating these modalities into routine disease diagnosis or disease staging. To this end, an ultrasensitive electrochemical biosensor has been reported where BMS-8, a small molecule that specifically interacts with sPD-L1, was immobilized on a gold electrode. This technique detected the concentration of sPD-L1 in buffer solution, with a DL of 2.5 fg/mL (Niedziałkowski et al., 2021) (Figure 2B). In contrast, anti-sPD-L1 monoclonal antibodies were used as the bio-receptor in a label-free localized surface plasmon resonance biosensor (LSPR), which reached a DL of 1 pg/mL in buffer (Luo et al., 2019). The sensor surface was decorated with excessively tilted fiber gratings (ExTFG) conjugated with large-sized gold nanoshells, on which the anti-sPD-L1 monoclonal antibodies were cross-linked. (Luo et al., 2019). Another biosensing strategy for the detection of sPD-L1 in buffer and spiked serum samples was to fabricate an electrochemical paper-based microfluidic aptasensor. The electrode is modified with nanocomposites consisting of amine-functionalized SWCNT. An aptamer having high affinity for PD-L1 was immobilised on the electrode along with an electroactive substance like methylene blue, for efficient electron transfer and signal detection. In addition, AuNPs promoted reduction of the electrode surface resistance and amplified the signal. The highly sensitive and accurate aptasensor produced a DL of 10 pg/mL. It is suitable for POCT because of its small size, low detection time, cost-effectiveness, and portability (Xing et al., 2021). An important caveat for the ultra-sensitive detection of the soluble immune checkpoint molecules is that most (6 out of 7 studies collated in Table 1) of the developed biosensors have been validated using biomimicking fluids like spiked serum or culture supernatants. Therefore, it is imperative to check their efficacy in patient sera, which may require further modifications to the biosensing platform. Recently, a study has used blood samples from cancer patients to detect soluble PD-L1 using magnetite nanorods with ordered mesocages (MNOM) and silver nanoclusters (AgNCs). The magneto-optical properties of the nanocomplexes resulted in highly sensitive detection of the target molecule. Highly specific dual biorecognition of the patient-derived sPD-L1 was achieved between the antibody immobilized on the gold chip and the aptamer on MNOM@AgNCs. This SPR-based sensor had a DL of 3.29 ng/mL (Huang et al., 2021). Since, the normal serum concentration of sPD-L1 is about 0.716 ng/mL (Wang et al., 2015), and the concentration of sPD-L1 in cancer patients may reach up to 25 ng/mL, this sensor can detect well within the diagnostic range of the analyte. Similar investigations with the above mentioned soluble isoforms of the checkpoint molecules is required to establish them for diagnostic procedures in the different types of cancers or other disease conditions.
In addition to the PD-L1: PD-1 interaction, there are other immune checkpoint molecules, which are as intensely involved in tumor-immune cross talk, and for which soluble isoforms also exist. These include the CD28/CTLA-4: B7 ligands axis, ICOS: ICOS-L axis, and the CD226/PVR: PVRL axis. (Khan et al., 2021; Her et al., 2009; Xu et al., 2009; Iguchi-Manaka et al., 2020). Other immune molecules belonging to the Tumor-necrosis factor superfamily like CD40, 4-1BB, OX-40, CD27 and GITR, along with their respective ligands are also involved in the anti-tumor immune responses (Ward-Kavanagh et al., 2016). These molecules also have soluble splice variants which correlate with poor disease prognosis in cancer (Taylor and Schwarz, 2001; Dimberg et al., 2006; Gan et al., 2013; Huang et al., 2013; Mu et al., 2015).
One such molecule, the sCD40L, is found to be increased in the serum of patients with lung (Roselli et al., 2004) and nasopharyngeal cancer (Caggiari et al., 2007). Normal serum level of sCD40L is ∼2.2 ng/mL, (Kim et al., 2011), whereas it increases to ∼18 ng/mL in cancer (Caggiari et al., 2007). This soluble splice variant competes with the membrane bound CD40L for engagement with CD40 receptors expressed on tumor cells. sCD40L is found to support tumor growth and stimulate angiogenesis and establishes an immunosuppressive environment by upregulating the suppressive function of myeloid derived suppressor cells, expression of PD-1 molecules and induces production of tumorigenic cytokines and proliferation of regulatory T cells (Huang et al., 2012). Electrochemical biosensors have been designed to detect sCD40L using monoclonal antibodies as bioreceptors immobilized on different types of fabricated materials on the sensor electrode surface. An ultra-low DL of 3 fg/mL could be achieved when the anti-CD40L mAb was immobilized on a nanocomposite made of AuNPs, branched polyethylenimine (b-PEI) and carboxylated Multiwalled Carbon Nanotubes (c-MWCNTs) on the sensor surface (Wu et al., 2017). Modification of a GCE with b-cyclodextrin (CD) and reduced graphene oxide tetraethylene pentamine (rGO-TEPA) along with coupling of a linker to the modified electrode led to accelerated immobilization of target-specific antibodies and detection of sCD40L with a DL of 83.3 fg/mL in human serum. (Zhao et al., 2015). For the simultaneous detection of CRP and sCD40L, a sandwich-based system was developed which used a different set of modifications on the GCE with AuNP nanospheres and bovine serum albumin (BSA) that helped to immobilize the target-specific capture antibodies. The detection antibodies for CRP and sCD40L were conjugated to rGO-TEPA further modified with metal ion labels. Two different metal ions (Cu2+ and Pb2+) were used for the detection of the two query proteins. This highly stable and accurate immunosensor exhibited a DL of 13.1 pg/mL for sCD40L (Yuan et al., 2015). It is important to note here, that sCD40L is an important biomarker in cardio-vascular diseases as well (Shami et al., 2020) and therefore, biosensor-mediated detection of soluble isoforms of immune-checkpoint molecules has broader applications in many diseases.
In addition to secreting soluble isoforms of the checkpoint molecules, tumor cells have been found to encapsulate them in exosomes and release them in the circulation. Exosomes are lipid-enclosed vesicles (30–150 nm) produced by many types of cells, which facilitate intercellular communication by transporting nucleic acids, proteins, and lipids from one cell to another (Yáñez-Mó et al., 2015; Tkach and Théry, 2016). Exosomes containing PD-L1 have been reported to be released by many types of tumor cells, especially in lung cancer (Kim et al., 2019). Detection of these PD-L1 expressing exosomes in the serum provides a unique opportunity for non-invasive diagnosis and assessment of cancer progression. Among various detection approaches, a highly sensitive and label-free SPR biosensor was used to detect the exosomal epidermal growth factor receptor (EGFR) and PD-L1 in cell culture medium and serum samples from lung cancer patients (Liu et al., 2018). Another SPR sensor was prepared by functionalizing graphene on a gold sensor chip and utilising M-Pep as the biorecognition molecule. This M-Pep is a multifunctional peptide consisting of assembly, binding and recognition domains and could detect PD-L1 ectodomain expressed on exosomes in spiked serum samples (Mao et al., 2021). Another biosensing device based on Fe3O4@TiO2 nanospheres was developed to capture the exosomes. Exosomal PD-L1 was further quantified by SERS tag-conjugated anti-PD-L1 antibody, in the serum of NSCLC patients. This technique could distinguish early and advanced stage NSCLC patients from healthy controls (Pang et al., 2020). These data suggest that the nanosensor-mediated detection of the soluble immune markers can be further extrapolated to study the exosomal contents secreted by the tumor cells, for greater clarity of understanding of the tumor-immune interaction, and as a marker for disease progression.
The above discussion about the different biosensors fabricated to detect the cancer-relevant cytokines and soluble immune checkpoint molecules, has revealed some interesting trends in the strategies adapted in these bio-sensing devices to detect the proteins of interest. In the following section, an overview of these trends is provided.
The design and principle of operation of biosensors rely heavily on the conductivity, physical and chemical properties of the transducer for ultra-sensitivity of detection, as well as biocompatibility. Nanoparticles (NPs) of metal conductors and semiconductors are the ideal choice for the efficient design of transducers. NPs exhibit better conductivity than the corresponding macrostructures owing to the high surface area-to-volume ratio, which enables an enhanced electron transfer rate mainly as a surface phenomenon. NPs of inert metals, such as gold (Au), silver (Ag), platinum (Pt), palladium (Pd), etc., are the most common ones used in electrochemical and optical biosensors. On the other hand, carbon-based NPs, such as graphene, CNTs, carbon nanoshells etc,. have gained much popularity in the past few decades owing to the specific characteristics such as high electrical conductivity due to the presence of mobile π-electrons, large surface area and the possibility of modification with a variety of functional groups (Jacobs et al., 2010; Suvarnaphaet and Pechprasarn, 2017). Many such NPs have been used, in varying composition, in the biosensors discussed so far, for the detection of soluble cytokines and immune checkpoint molecules.
AuNPs and other Au nanostructures show, in addition to excellent conductivity and biocompatibility, easy immobilisation of biomolecules through the widely explored gold-thiol (Au-S) binding chemistry (Vidotti et al., 2011). A biorecognition particle, such as an antibody or aptamer, can be directly immobilised either to the Au surface or via a linker with a thiol group. The aptasensors reported by Tertis et al. (2019), Cao et al. (2021), Xing et al. (2021) immobilised thiol-terminated aptamers onto the AuNP surface. On the other hand, thiol-terminated linkers were used by other groups, such as glutathione (GSH) (Munge et al., 2009; Chikkaveeraiah et al., 2011; Krause et al., 2015), the Raman reporters 4-mercaptobenzoic acid (4-MBA) (Wang et al., 2019) and 5,5’-dithiobis (2-ntrobenzoic acid) (DTNB) (Kamińska et al., 2017a), 11-mercaptoundecanoic acid (11-MUA) (Pourmadadi et al., 2020), and cysteamine (Luo et al., 2019). Antibodies were also reported to be loaded onto AuNPs-multi-walled carbon nanotube (MWNT) conjugates by adsorption (Wu et al., 2017), and via glutaraldehyde (GA) onto Au@BSA conjugates (Yuan et al., 2015). Such linkers usually contained one or more carboxyl groups (GSH, 4-MBA, DTNB, 11-MUA) (Munge et al., 2009; Chikkaveeraiah et al., 2011; Krause et al., 2015; Kamińska et al., 2017a; Wang et al., 2019; Pourmadadi et al., 2020) or an amine group (cysteamine) (Luo et al., 2019). Amide bond formation can be induced between these linkers and the corresponding amino- or carboxyl-groups on the antibodies/aptamers, respectively. The most commonly used catalysts for the consecutive amidization steps are (1-ethyl-3-(3-dimethylaminopropyl) carbodiimide (EDC) and N-hydroxysuccinimide (NHS). As a result, the antibodies/aptamers are covalently attached to the AuNPs. The AuNP conjugates may either be attached to the electrode, as part of the transducing interface, or freely dispersed in the electrolytic solution, forming a part of the signal generating label/reporter. Tertis et al. (2019) immobilised AuNPs on a glassy carbon electrode (GCE) with two linkers: p-aminobenzoic acid and p-aminothiophenol, via EDC/NHS catalysis and Au-S chemistry. The LSPR biosensor developed by Luo et al. (2019) on the other hand, used 3-mercaptopropyl trimethoxysilane (MPTMS) as a linker to attach AuNPs to the optical fiber. The SERS-based biosensors developed by Wang et al., Kamińska et al., 2017b; Cao et al. (2021) employed the AuNP conjugates as dispersed SERS tags bound to signal antibodies/aptamers. Another notable property of AuNPs is the pink/red coloration imparted by the dispersed solution, which has been utilised to design a colorimetric lateral flow aptasensor (LFA) capable of giving a naked eye colorimetric readout (Cheng et al., 2020). Localised AuNPs are capable of effecting the surface plasmon resonance phenomenon, brought by the coherent oscillation of surface electrons on the AuNP upon absorption at the resonant frequency. This phenomenon was explored by the LSPR biosensor reported by Luo et al. (2019).
Among the most extensively utilised NPs for the detection of cytokines and immune checkpoint molecules, carbon-based NPs closely follow AuNPs. Single-walled carbon nanotubes (SWNTs), graphene oxide (GO) and reduced graphene oxide (RGO) have been frequently reported to be used for enhancement of the conductivity of the transducing surface, as well as for bio functionalisation. The carboxyl groups present in GO and carboxyl functionalised SWNT and MWNT can be easily used to covalently immobilise antibodies via EDC/NHS treatment (Chikkaveeraiah et al., 2009; Malhotra et al., 2010; Sardesai et al., 2011; Sardesai et al., 2013; Lin et al., 2015). Yuan et al. (2015) used a different approach for immobilisation wherein they used glutaraldehyde as linkers between the amine groups of antibodies and that of tetraethylene pentamine (TEPA) in RGO-TEPA nanosheets. The linkage thus formed was an imine bond. Zhao et al. employed the thiol groups of 1,4-phenylene diisothiocyanate (PDITC C6H4[NCS]2) for crosslinking tetraethylene-RGO and antibodies via the amine groups. Thiol groups were also used by Pan et al. (2017) to immobilise thiolated aptamers on GO. The carboxyl groups of GO were first attached to branched polyethylenimine (b-PEI) via EDC/NHS treatment, followed by the addition of thiol groups via sulfosuccinimidyl-4-(N-maleimidomethyl)cyclohexane-1-carboxylate (Sulfo-SMCC) treatment. Apart from these, biotin-streptavidin affinity linkage has also been widely used for conjugating antibodies/aptamers to NPs, enzymes to antibodies, etc., (Chikkaveeraiah et al., 2009; Munge et al., 2009; Krause et al., 2015; Cheng et al., 2020; Cao et al., 2021) Valverde et al. (2020) employed neutravidin coated magnetic beads instead of streptavidin to capture biotinylated antibodies.
Cytokines and other immune checkpoint molecules (and other signal molecules) are present in ultra-low levels in blood and interstitial fluids. It is due to this reason that researchers often seek to concentrate the analyte molecules before the detection step. One simple approach is to use magnetic beads coated with biorecognition particles. This not only ensures the efficient capture of the analyte molecules, but also provides an off-electrode reaction site for the biological capture/complex formation. The magnetic beads can then be captured onto the electrode via a magnet. This approach has already been reported in the relevant field by many researchers (Bettazzi et al., 2013; Eletxigerra et al., 2014; Valverde et al., 2020).
Another important consideration when designing a biosensor is the selection of the bioreceptors. mAbs have been used as a bio-recognition element for analyte detection purposes because of their high selectivity and binding affinity to the target proteins. However, their exorbitant price, decreased stability in higher temperature and sub-optimal pH ranges, and complicated production procedure pose serious limitations for translation of the biosensors to the clinics. These problems can be circumvented with the emergence of Aptamers as bioreceptors owing to various advantages like structural stability, small size, as well as ease of production and modification. Chemical synthesis of aptamers in large scale, without involving animal models, reduces production time in addition to being cost-effective. Also, aptamers exhibit low immunogenicity and toxicity as compared to antibodies (Bouchard et al., 2010). Moreover, aptamers are highly stable at elevated temperatures, have long shelf life and possess regenerative abilities (Thiviyanathan and Gorenstein, 2012). Aptamers can be modified to prevent their degradation, and can be labelled with different functional probes easily without loss of activity. Furthermore, unlike antibodies, aptamers can be probed against non-immunogenic and toxic targets that may be of significance in different infectious disease pathogenesis (Hong et al., 2012). Due to these distinct and favorable advantages of aptamers, recently, the aptamer-based sensors have gained traction in the field of biosensing, especially in cytokine detection (Kim et al., 2021). These aptasensors have also been designed for clinical diagnostics, where they have been utilized in the detection of cancer biomarkers, cancer cells as well as infectious microorganisms (bacteria, virus and prion) (Hong et al., 2012). Therefore, while the mAbs are still preferred by most researchers for fabricating biosensors, the aptamers provide a better alternative for clinical translation and should be investigated as bioreceptors more frequently.
Although biosensors offer many advantages such as ultra-sensitivity, rapid and cost-effective detection, portability, etc., there are however some limitations that need to be addressed before they are brought to use at the clinical testing level. These limitations include low stability, specificity and the need of sample pre-processing steps, among others. Some of these limitations have been attempted to be solved at the design level. For instance, microfluidic devices and integrated systems can maintain a controlled flow rate, and provide separate chambers for sample pre-mixing and pre-processing steps (Chikkaveeraiah et al., 2011; Sardesai et al., 2013). Wuethrich et al. (2019) prepared a microfluidic platform for the parallel detection of three soluble immune checkpoint molecules, which employed alternating current to conduct electrohydrodynamic (ac-EHD) mixing in a single sample droplet.
Low sample volume is an advantage offered by multiplexed biosensors, which can monitor several biomarkers simultaneously within the same sample. Furthermore, multiplexed detection of several biomarkers from patient sera would allow the analysis of the tumor-immune interactions in a more integrative manner, for in-depth monitoring of disease development and progression. The multiplexed biosensing devices are generally made on microfluidic platform, to help maintain small sample volume. The multiplexed cytokine biosensors employed antibody-conjugated magnetic beads for on-line or off-line capture of the respective target proteins, to achieve femtogram level detection. Offline capture of the HNSCC biomarker proteins IL-6, IL-8, VEGF, VEGF-C from serum samples of oral cancer patients by antibody-coated magnetic beads, led to the detection of these proteins in unprecedented ultra-low levels of 5–50 pg/mL (Malhotra et al., 2012). The same group employed this strategy to design a more improved semi-automated modular microfluidic immunoarray for the capture and simultaneous detection of femtogram levels of different cytokines involved in oral cancer or cancer-related oral pathologies (Otieno et al., 2014; Krause et al., 2015). Here, enzyme-labeled antibody-coated magnetic beads were used for the capture of the target proteins in a capture-chamber. After washing these bioconjugates under magnetic control, the magnetic bead-antibody-analyte complexes were detected on a nanostructured eight-sensor array with a second set of antibodies. Electrochemical reactions between enzyme-labeled antibodies and the substrate, which was injected into the system, were then quantified by amperometric signal generation (Otieno et al., 2014; Krause et al., 2015).
In an interesting development, a nano-yeast based approach was adapted for the multiplexed detection of the soluble immune checkpoint molecules sPD-1, sPD-L1 and sLAG-3, which are involved in CD8 T cell exhaustion (Triebel et al., 2006; Blackburn et al., 2009), a hallmark of immune-evasion by tumor cells. Here hemagglutinin-tagged target-specific nano-yeast scFv was conjugated to anti-hemaglutinin antibody to separately functionalize the electrode surface. These multiplexed immune checkpoint biosensors (MICBs) involved application of a single drop of sample on electrodes followed by ac-EHD based mixing of samples. The analytes were detected with target-specific secondary antibodies, conjugated either with HRP (Wuethrich et al., 2019) or with a SERS-tag (Reza et al., 2019). While the SERS-based biosensor could detect femtogram levels of the soluble immune checkpoint molecules, the colorimetric one showed slightly lesser sensitivity at pico-gram levels, albeit within the disease relevant range (as shown in Table 1). In addition to successful multiplexed detection of the analytes, these studies established nano-yeast scFv as a high affinity and stable alternative to monoclonal antibodies, with long shelf life without compromising their sensitivity.
Another tool for rapid and user-friendly detection is the chip-based or paper-based lateral flow assays (Cheng et al., 2020; Cao et al., 2021). While such assay platforms are cheap and easily interpretable even by untrained personnel, these are qualitative or semi-quantitative at best, in contrast to the highly sensitive electrochemical sensors, which give measurable outputs.
These trends clearly show that all of these strategies collectively endeavour to overcome the limitations associated with biosensors, and make the bio-sensing platforms robust and suitable for point-of-care testing.
Cytokines and soluble immune checkpoint molecules present in patient sera, serve as potential biomarkers in a broad range of malignancies, and early detection of these candidate biomarkers can provide a larger window for timely medical intervention in cancer. In the current discourse, we have comprehensively discussed the different biosensing platforms that have been developed to specifically detect these molecules either directly from patient samples or as investigative studies using bio-mimicking fluids, in ultra-low levels (summarized in Table 1). While having immense promise, further testing and validation in different cohorts of cancer patients need to be undertaken, for clinical implementation.
Importantly, detection of the immune molecules under consideration goes beyond the monitoring of disease progression. Several of these molecules can, in fact, be used as readouts of patient response to therapeutic regimens and as a screening method to qualitatively and quantitatively monitor the efficacy of novel therapeutic strategies. For example, the immune checkpoint inhibitor mAbs have often shown variable efficacy in different cohorts of patients (Sharpe, 2017). Such variability could be due the elevated levels of the soluble isoforms in the serum, which could act as decoy receptors for the mAbs and inhibit their interactions with the membrane-bound checkpoint molecules. On the other hand, it has been shown that patients with higher serum concentration of sCTLA4 levels responded better to ipilimumab, the anti-CTLA4 mAb that resulted in improved survival rates (Leung et al., 2014). In this case, sCTLA4 in the serum binds to the costimulatory ligands on the APCs, thereby blocking the second costimulatory signal required for T cell activation, resulting in inhibition of the T cell-mediated immune response. Ipilimumab-mediated scavenging of the sCTLA-4 could therefore lead to a better anti-tumor response. These findings unequivocally establish the requirement of real-time monitoring of the soluble immune checkpoint molecules like sCTLA-4, thereby necessitating the integration of the biosensing devices in routine diagnostic and monitoring approaches in cancer patients. These devices could provide important clues for patient selection for checkpoint blockade immunotherapy, which can then be customized to the physiological heterogeneity of the levels of the soluble isoforms in individual patients.
While the biosensors developed so far have been able to detect very low levels of relevant soluble immune molecules in patient derived sera, it is imperative to investigate other easily collectible biofluids like urine, saliva, sweat and semen for similar monitoring. In various tissue specific cancers, these body fluids may reflect the pathology better than the peripheral blood serum. Additionally, the biochemical characteristics of these fluids may differ significantly from that of serum and among themselves, which warrants the fabrication of specific biosensors considering these parameters.
In addition to the aforementioned applications of the biosensors in cancer, these can also be employed in the detection and monitoring of several other ailments, like cardiovascular disease, autoimmune diseases and inflammatory bowel diseases. Moreover, highly sensitive and rapid detection of inflammatory markers like CRP would provide crucial time for medical intervention in infectious diseases like COVID-19 (Luo et al., 2020).
Overall, the utility of immunosensors that can detect femtogram or even lower levels of analytes in the body fluids transcends diagnostic application and can provide valuable information regarding disease pathogenesis for both clinical and basic research. In the clinics, these sensors can help monitor efficacy of therapeutic interventions, act as a decision-support tool to clinicians and help design most relevant personalized immunotherapeutic strategy. Furthermore, real-time monitoring of the soluble immune markers by the nano-sensing devices can provide crucial information for the identification of new therapeutic targets in cancer and beyond. In conclusion, for the implementation of the ultra-sensitive biomarkers from the bench to bedside, there is a requirement of close collaboration between biomedical engineers working on the development of these ultra-sensitive devices, and basic scientists like cancer biologists and immunologists as well as clinical experts to provide the most relevant targets. Such interdisciplinary approaches will help a holistic evolution and progress of the field to ultimately arrive at the goal of making these biosensors available for patients for early disease diagnosis and implementation of personalized therapeutic strategies.
NP performed literature review, wrote the manuscript, and designed figures and table. DB contributed to manuscript writing and designed figures. AH edited and reviewed the manuscript, and GM conceived the paper, supervised and performed writing and reviewing of the manuscript. ND contributed to writing the manuscript. GD critically read and reviewed the manuscript. All authors contributed to the article and approved the submitted version.
The authors declare that the research was conducted in the absence of any commercial or financial relationships that could be construed as a potential conflict of interest.
All claims expressed in this article are solely those of the authors and do not necessarily represent those of their affiliated organizations, or those of the publisher, the editors and the reviewers. Any product that may be evaluated in this article, or claim that may be made by its manufacturer, is not guaranteed or endorsed by the publisher.
Adrover-Jaume, C., Alba-Patiño, A., Clemente, A., Santopolo, G., Vaquer, A., et al. (2021). Paper Biosensors for Detecting Elevated IL-6 Levels in Blood and Respiratory Samples from COVID-19 Patients. Sens. Actuators B Chem. 330, 129333. doi:10.1016/j.snb.2020.129333
Aggarwal, S., Devaraja, K., Sharma, S. C., and Das, S. N. (2014). Expression of Vascular Endothelial Growth Factor (VEGF) in Patients with Oral Squamous Cell Carcinoma and its Clinical Significance. Clin. Chim. Acta 436, 35–40. doi:10.1016/j.cca.2014.04.027
Akbari jonous, Z., Shayeh, J. S., Yazdian, F., Yadegari, A., Hashemi, M., and Omidi, M. (2019). An Electrochemical Biosensor for Prostate Cancer Biomarker Detection Using Graphene Oxide-Gold Nanostructures. Eng. Life Sci. 19 (3), 206–216. doi:10.1002/elsc.201800093
Alba-Patiño, A., Russell, S. M., Borges, M., Pazos-Pérez, N., Álvarez-Puebla, R. A., and de la Rica, R. (2020). Nanoparticle-based Mobile Biosensors for the Rapid Detection of Sepsis Biomarkers in Whole Blood. Nanoscale Adv. 2 (3), 1253–1260. doi:10.1039/D0NA00026D
Ariad, S., Seymour, L., and Bezwoda, W. R. (1991). Platelet-derived Growth Factor (PDGF) in Plasma of Breast Cancer Patients: Correlation with Stage and Rate of Progression. Breast Cancer Res. Tr. 20 (1), 11–17. doi:10.1007/bf01833352
Baeumner, A. J., Schlesinger, N. A., Slutzki, N. S., Romano, J., Lee, E. M., and Montagna, R. A. (2002). Biosensor for Dengue Virus Detection: Sensitive, Rapid, and Serotype Specific. Anal. Chem. 74 (6), 1442–1448. doi:10.1021/ac015675e
Bart, M., Stigter, E. C. A., Stapert, H. R., De Jong, G. J., and Van Bennekom, W. P. (2005). On the Response of a Label-free Interferon-γ Immunosensor Utilizing Electrochemical Impedance Spectroscopy. Biosens. Bioelectron. 21 (1), 49–59. doi:10.1016/j.bios.2004.10.009
Berberoglu, U., Yildirim, E., and Celen, O. (2004). Serum Levels of Tumor Necrosis Factor Alpha Correlate with Response to Neoadjuvant Chemotherapy in Locally Advanced Breast Cancer. Int. J. Biol. Markers 19 (2), 130–134. doi:10.1177/172460080401900207
Berggren, C., Bjarnason, B., and Johansson, G. (1998). An Immunological Interleukine-6 Capacitive Biosensor Using Perturbation with a Potentiostatic Step. Biosens. Bioelectron. 13 (10), 1061–1068. doi:10.1016/S0956-5663(98)00058-X
Bettazzi, F., Enayati, L., Sánchez, I. C., Motaghed, R., Mascini, M., and Palchetti, I. (2013). Electrochemical Bioassay for the Detection of TNF-α Using Magnetic Beads and Disposable Screen-Printed Array of Electrodes. Bioanalysis 5 (1), 11–19. doi:10.4155/bio.12.293
Bhalla, N., Jolly, P., Formisano, N., and Estrela, P. (2016). Introduction to Biosensors. Essays Biochem. 60 (1), 1–8. doi:10.1042/EBC20150001
Blackburn, S. D., Shin, H., Haining, W. N., Zou, T., Workman, C. J., Polley, A., et al. (2009). Coregulation of CD8+ T Cell Exhaustion by Multiple Inhibitory Receptors during Chronic Viral Infection. Nat. Immunol. 10 (1), 29–37. doi:10.1038/ni.1679
Blank, C., and Mackensen, A. (2007). Contribution of the PD-L1/PD-1 Pathway to T-Cell Exhaustion: an Update on Implications for Chronic Infections and Tumor Evasion. Cancer Immunol. Immunother. 56 (5), 739–745. doi:10.1007/s00262-006-0272-1
Botelho, F., Pina, F., and Lunet, N. (2010). VEGF and Prostatic Cancer: a Systematic Review. Eur. J. Cancer Prev. 19 (5), 385–392. doi:10.1097/cej.0b013e32833b48e1
Bouchard, P. R., Hutabarat, R. M., and Thompson, K. M. (2010). Discovery and Development of Therapeutic Aptamers. Annu. Rev. Pharmacol. Toxicol. 50, 237–257. doi:10.1146/annurev.pharmtox.010909.105547
Braun, T., Ghatkesar, M. K., Backmann, N., Grange, W., Boulanger, P., Letellier, L., et al. (2009). Quantitative Time-Resolved Measurement of Membrane Protein-Ligand Interactions Using Microcantilever Array Sensors. Nat. Nanotech. 4 (3), 179–185. doi:10.1038/nnano.2008.398
Caggiari, L., Guidoboni, M., Vaccher, E., Barzan, L., Franchin, G., Gloghini, A., et al. (2007). High Serum Levels of Soluble CD40-L in Patients with Undifferentiated Nasopharyngeal Carcinoma: Pathogenic and Clinical Relevance. Infect. Agents Cancer 2 (1), 1–11. doi:10.1186/1750-9378-2-5
Cao, X., Song, Q., Sun, Y., Mao, Y., Lu, W., and Li, L. (2021). A SERS-LFA Biosensor Combined with Aptamer Recognition for Simultaneous Detection of Thrombin and PDGF-BB in Prostate Cancer Plasma. Nanotechnology 32 (44), 445101. doi:10.1088/1361-6528/ac1754
Carmeliet, P. (2005). VEGF as a Key Mediator of Angiogenesis in Cancer. Oncology 69 (Suppl. 3), 4–10. doi:10.1159/000088478
Chakrabarti, R., Kapse, B., and Mukherjee, G. (2019). Soluble Immune Checkpoint Molecules: Serum Markers for Cancer Diagnosis and Prognosis. Cancer Rep. 2 (4), e1160. doi:10.1002/cnr2.1160
Chang, K.-P., Kao, H.-K., Wu, C.-C., Fang, K.-H., Chang, Y.-L., Huang, Y.-C., et al. (2013). Pretreatment Interleukin-6 Serum Levels Are Associated with Patient Survival for Oral Cavity Squamous Cell Carcinoma. Otolaryngol. Head. Neck Surg. 148 (5), 786–791. doi:10.1177/0194599813478573
Chen, P., Huang, N. T., Chung, M. T., Cornell, T. T., and Kurabayashi, K. (2015). Label-free Cytokine Micro- and Nano-Biosensing towards Personalized Medicine of Systemic Inflammatory Disorders. Adv. Drug Deliv. Rev. 95, 90–103. doi:10.1016/j.addr.2015.09.005
Cheng, N., Liu, Y., Mukama, O., Han, X., Huang, H., Li, S., et al. (2020). A Signal-Enhanced and Sensitive Lateral Flow Aptasensor for the Rapid Detection of PDGF-BB. RSC Adv. 10 (32), 18601–18607. doi:10.1039/D0RA02662J
Chikkaveeraiah, B. V., Bhirde, A., Malhotra, R., Patel, V., Gutkind, J. S., and Rusling, J. F. (2009). Single-wall Carbon Nanotube Forest Arrays for Immunoelectrochemical Measurement of Four Protein Biomarkers for Prostate Cancer. Anal. Chem. 81 (21), 9129–9134. doi:10.1021/ac9018022
Chikkaveeraiah, B. V., Mani, V., Patel, V., Gutkind, J. S., and Rusling, J. F. (2011). Microfluidic Electrochemical Immunoarray for Ultrasensitive Detection of Two Cancer Biomarker Proteins in Serum. Biosens. Bioelectron. 26 (11), 4477–4483. doi:10.1016/j.bios.2011.05.005
Cho, I.-H., Kim, D. H., and Park, S. (2020). Electrochemical Biosensors: Perspective on Functional Nanomaterials for On-Site Analysis. Biomater. Res. 24 (1), 1–12. doi:10.1186/s40824-019-0181-y
Crowley, E., O'sullivan, C., and Guilbault, G. G. (1999). Amperometric Immunosensor for Granulocyte-Macrophage Colony-Stimulating Factor Using Screen-Printed Electrodes. Anal. Chim. acta 389 (1-3), 171–178. doi:10.1016/S0003-2670(99)00146-4
Damborský, P., Švitel, J., and Katrlík, J. (2016). Optical Biosensors. Essays Biochem. 60 (1), 91–100. doi:10.1042/EBC20150010
Dimberg, J., Hugander, A., and Wågsäter, D. (2006). Expression of CD137 and CD137 Ligand in Colorectal Cancer Patients. Oncol. Rep. 15 (5), 1197–1200. doi:10.3892/or.15.5.1197
Dutta, N., Lillehoj, P. B., Estrela, P., and Dutta, G. (2021). Electrochemical Biosensors for Cytokine Profiling: Recent Advancements and Possibilities in the Near Future. Biosensors 11 (3), 94. doi:10.3390/bios11030094
Eletxigerra, U., Martinez-Perdiguero, J., Merino, S., Villalonga, R., Pingarrón, J. M., and Campuzano, S. (2014). Amperometric Magnetoimmunoassay for the Direct Detection of Tumor Necrosis Factor Alpha Biomarker in Human Serum. Anal. Chim. acta 838, 37–44. doi:10.1016/j.aca.2014.05.047
Erikaku, T., Zenno, S., and Inouye, S. (1991). Bioluminescent Immunoassay Using a Monomeric Fab'-Photoprotein Aequorin Conjugate. Biochem. Biophys. Res. Commun. 174 (3), 1331–1336. doi:10.1016/0006-291X(91)91568-W
Ferrajoli, A., Keating, M. J., Manshouri, T., Giles, F. J., Dey, A., Estrov, Z., et al. (2002). The Clinical Significance of Tumor Necrosis Factor-α Plasma Level in Patients Having Chronic Lymphocytic Leukemia. Blood, J. Am. Soc. Hematol. 100 (4), 1215–1219. doi:10.1182/blood.v100.4.1215.h81602001215_1215_1219
Gan, X., Feng, X., Gu, L., Tan, W., Sun, X., Lv, C., et al. (2013). Correlation of Increased Blood Levels of GITR and GITRL with Disease Severity in Patients with Primary Sjögren's Syndrome. Clin. Dev. Immunol. 2013, 1–9. doi:10.1155/2013/340751
Germano, G., Allavena, P., and Mantovani, A. (2008). Cytokines as a Key Component of Cancer-Related Inflammation. Cytokine 43 (3), 374–379. doi:10.1016/j.cyto.2008.07.014
Gonzalez, H., Hagerling, C., and Werb, Z. (2018). Roles of the Immune System in Cancer: from Tumor Initiation to Metastatic Progression. Genes Dev. 32 (19-20), 1267–1284. doi:10.1101/gad.314617.118
Goode, J., Dillon, G., and Millner, P. A. (2016). The Development and Optimisation of Nanobody Based Electrochemical Immunosensors for IgG. Sens. Actuators B Chem. 234, 478–484. doi:10.1016/j.snb.2016.04.132
Gu, D., Ao, X., Yang, Y., Chen, Z., and Xu, X. (2018). Soluble Immune Checkpoints in Cancer: Production, Function and Biological Significance. J. Immunother. cancer 6 (1), 1–14. doi:10.1186/s40425-018-0449-0
Guerrero, S., Sánchez-Tirado, E., Martínez-García, G., González-Cortés, A., Yáñez-Sedeño, P., and Pingarrón, J. M. (2020). Electrochemical Biosensor for the Simultaneous Determination of Rheumatoid Factor and Anti-cyclic Citrullinated Peptide Antibodies in Human Serum. Analyst 145 (13), 4680–4687. doi:10.1039/D0AN00481B
Haes, A. J., Chang, L., Klein, W. L., and Van Duyne, R. P. (2005). Detection of a Biomarker for Alzheimer's Disease from Synthetic and Clinical Samples Using a Nanoscale Optical Biosensor. J. Am. Chem. Soc. 127 (7), 2264–2271. doi:10.1021/ja044087q
Her, M., Kim, D., Oh, M., Jeong, H., and Choi, I. (2009). Increased Expression of Soluble Inducible Costimulator Ligand (ICOSL) in Patients with Systemic Lupus Erythematosus. Lupus 18 (6), 501–507. doi:10.1177/0961203308099176
Hong, P., Li, W., and Li, J. (2012). Applications of Aptasensors in Clinical Diagnostics. Sensors 12 (2), 1181–1193. doi:10.3390/s120201181
Hosu, O., Selvolini, G., Cristea, C., and Marrazza, G. (2018). Electrochemical Immunosensors for Disease Detection and Diagnosis. Cmc 25 (33), 4119–4137. doi:10.2174/0929867324666170727104429
Huang, J., Jochems, C., Talaie, T., Anderson, A., Jales, A., Tsang, K. Y., et al. (2012). Elevated Serum Soluble CD40 Ligand in Cancer Patients May Play an Immunosuppressive Role. Blood, J. Am. Soc. Hematol. 120 (15), 3030–3038. doi:10.1182/blood-2012-05-427799
Huang, J., Jochems, C., Anderson, A. M., Talaie, T., Jales, A., Madan, R. A., et al. (2013). Soluble CD27-Pool in Humans May Contribute to T Cell Activation and Tumor Immunity. J. I. 190 (12), 6250–6258. doi:10.4049/jimmunol.1300022
Huang, X., Zhang, Z.-h., Chen, J., Mao, Z., Zhu, H., Liu, Y., et al. (2021). One Dimensional Magneto-Optical Nanocomplex from Silver Nanoclusters and Magnetite Nanorods Containing Ordered Mesocages for Sensitive Detection of PD-L1. Biosens. Bioelectron. 189, 113385. doi:10.1016/j.bios.2021.113385
Iguchi-Manaka, A., Okumura, G., Ichioka, E., Kiyomatsu, H., Ikeda, T., Bando, H., et al. (2020). High Expression of Soluble CD155 in Estrogen Receptor-Negative Breast Cancer. Breast Cancer 27 (1), 92–99. doi:10.1007/s12282-019-00999-8
Iwai, Y., Ishida, M., Tanaka, Y., Okazaki, T., Honjo, T., and Minato, N. (2002). Involvement of PD-L1 on Tumor Cells in the Escape from Host Immune System and Tumor Immunotherapy by PD-L1 Blockade. Proc. Natl. Acad. Sci. U.S.A. 99 (19), 12293–12297. doi:10.1073/pnas.192461099
Jablonska, E., Piotrowski, L., and Grabowska, Z. (1997). Serum Levels of IL-lβ, IL-6, TNF-α, sTNF-RI and CRP in Patients with Oral Cavity Cancer. Pathol. Oncol. Res. 3 (2), 126–129. doi:10.1007/BF02907807
Jacobs, C. B., Peairs, M. J., and Venton, B. J. (2010). Review: Carbon Nanotube Based Electrochemical Sensors for Biomolecules. Anal. Chim. Acta 662, 105–127. doi:10.1016/j.aca.2010.01.009
Jiang, Y., Li, Y., and Zhu, B. (2015). T-cell Exhaustion in the Tumor Microenvironment. Cell Death Dis. 6 (6), e1792. doi:10.1038/cddis.2015.162
Jin, J., Si, J., Liu, Y., Wang, H., Ni, R., and Wang, J. (2018). Elevated Serum Soluble Programmed Cell Death Ligand 1 Concentration as a Potential Marker for Poor Prognosis in Small Cell Lung Cancer Patients with Chemotherapy. Respir. Res. 19 (1), 1–9. doi:10.1186/s12931-018-0885-x
Kakimi, K., Karasaki, T., Matsushita, H., and Sugie, T. (2017). Advances in Personalized Cancer Immunotherapy. Breast Cancer 24 (1), 16–24. doi:10.1007/s12282-016-0688-1
Kamińska, A., Sprynskyy, M., Winkler, K., and Szymborski, T. (2017a). Ultrasensitive SERS Immunoassay Based on Diatom Biosilica for Detection of Interleukins in Blood Plasma. Anal. Bioanal. Chem. 409 (27), 6337–6347. doi:10.1007/s00216-017-0566-5
Kamińska, A., Winkler, K., Kowalska, A., Witkowska, E., Szymborski, T., Janeczek, A., et al. (2017b). SERS-based Immunoassay in a Microfluidic System for the Multiplexed Recognition of Interleukins from Blood Plasma: towards Picogram Detection. Sci. Rep. 7 (1), 1–11. doi:10.1038/s41598-017-11152-w
Khan, M. A., and Mujahid, M. (2020). Recent Advances in Electrochemical and Optical Biosensors Designed for Detection of Interleukin 6. Sensors 20 (3), 646. doi:10.3390/s20030646
Khan, M., Arooj, S., and Wang, H. (2021). Soluble B7-CD28 Family Inhibitory Immune Checkpoint Proteins and Anti-Cancer Immunotherapy. Front. Immunol. 12, 651634. doi:10.3389/fimmu.2021.651634
Kim, H. O., Kim, H.-S., Youn, J.-C., Shin, E.-C., and Park, S. (2011). Serum Cytokine Profiles in Healthy Young and Elderly Population Assessed Using Multiplexed Bead-Based Immunoassays. J. Transl. Med. 9 (1), 1–7. doi:10.1186/1479-5876-9-113
Kim, D. H., Kim, H., Choi, Y. J., Kim, S. Y., Lee, J.-E., Sung, K. J., et al. (2019). Exosomal PD-L1 Promotes Tumor Growth through Immune Escape in Non-small Cell Lung Cancer. Exp. Mol. Med. 51 (8), 1–13. doi:10.1038/s12276-019-0295-2
Kim, J., Noh, S., Park, J. A., Park, S.-C., Park, S. J., Lee, J.-H., et al. (2021). Recent Advances in Aptasensor for Cytokine Detection: A Review. Sensors 21 (24), 8491. doi:10.3390/s21248491
Kinoshita, T., Ito, H., and Miki, C. (1999). Serum Interleukin-6 Level Reflects the Tumor Proliferative Activity in Patients with Colorectal Carcinoma. Cancer 85 (12), 2526–2531. doi:10.1002/(sici)1097-0142(19990615)85:12<2526::aid-cncr6>3.0.co;2-3
Kozłowski, L., Zakrzewska, I., Tokajuk, P., and Wojtukiewicz, M. Z. (2003). Concentration of Interleukin-6 (IL-6), Interleukin-8 (IL-8) and Interleukin-10 (IL-10) in Blood Serum of Breast Cancer Patients. Rocz. Akad. Med. Bialymst 48, 82–84. PMID: 14737948.
Krause, C. E., Otieno, B. A., Bishop, G. W., Phadke, G., Choquette, L., Lalla, R. V., et al. (2015). Ultrasensitive Microfluidic Array for Serum Pro-inflammatory Cytokines and C-Reactive Protein to Assess Oral Mucositis Risk in Cancer Patients. Anal. Bioanal. Chem. 407 (23), 7239–7243. doi:10.1007/s00216-015-8873-1
Krishnamoorthy, S., Iliadis, A. A., Bei, T., and Chrousos, G. P. (2008). An Interleukin-6 ZnO/SiO2/Si Surface Acoustic Wave Biosensor. Biosens. Bioelectron. 24 (2), 313–318. doi:10.1016/j.bios.2008.04.011
Krzystek-Korpacka, M., Diakowska, D., Gamian, A., and Matusiewicz, M. (2011). Increase in Serum Platelet-Derived Growth Factor (PDGF)-BB Reflects Lymph Node Involvement in Esophageal Cancer Patients Independently from Platelet Count. Exp. Oncol. 33 (3), 140–144. PMID: 21956466.
Lakhin, A. V., Tarantul, V. Z., and Gening, L. V. (2013). Aptamers: Problems, Solutions and Prospects. Acta Naturae 5 (4), 34–43. PMID: 24455181. doi:10.32607/20758251-2013-5-4-34-43
Lata, K., Dhull, V., and Hooda, V. (2016). Fabrication and Optimization of ChE/ChO/HRP-AuNPs/c-MWCNTs Based Silver Electrode for Determining Total Cholesterol in Serum. Biochem. Res. Int. 2016, 1–11. doi:10.1155/2016/1545206
Lei, X., Lei, Y., Li, J.-K., Du, W.-X., Li, R.-G., Yang, J., et al. (2020). Immune Cells within the Tumor Microenvironment: Biological Functions and Roles in Cancer Immunotherapy. Cancer Lett. 470, 126–133. doi:10.1016/j.canlet.2019.11.009
Leng, S. X., McElhaney, J. E., Walston, J. D., Xie, D., Fedarko, N. S., and Kuchel, G. A. (2008). ELISA and Multiplex Technologies for Cytokine Measurement in Inflammation and Aging Research. J Gerontol Ser. A Biol. Sci. Med. Sci. 63 (8), 879–884. doi:10.1093/gerona/63.8.879
Leung, A. M., Lee, A. F., Ozao-Choy, J., Ramos, R. I., Hamid, O., O’Day, S. J., et al. (2014). Clinical Benefit from Ipilimumab Therapy in Melanoma Patients May Be Associated with Serum CTLA4 Levels. Front. Oncol. 4, 110. doi:10.3389/fonc.2014.00110
Li, J., Sun, K., Chen, Z., Shi, J., Zhou, D., and Xie, G. (2017). A Fluorescence Biosensor for VEGF Detection Based on DNA Assembly Structure Switching and Isothermal Amplification. Biosens. Bioelectron. 89, 964–969. doi:10.1016/j.bios.2016.09.078
Liao, W., and Cui, X. T. (2007). Reagentless Aptamer Based Impedance Biosensor for Monitoring a Neuro-Inflammatory Cytokine PDGF. Biosens. Bioelectron. 23 (2), 218–224. doi:10.1016/j.bios.2007.04.004
Lin, C.-W., Wei, K.-C., Liao, S.-s., Huang, C.-Y., Sun, C.-L., Wu, P.-J., et al. (2015). A Reusable Magnetic Graphene Oxide-Modified Biosensor for Vascular Endothelial Growth Factor Detection in Cancer Diagnosis. Biosens. Bioelectron. 67, 431–437. doi:10.1016/j.bios.2014.08.080
Liu, G., Qi, M., Hutchinson, M. R., Yang, G., and Goldys, E. M. (2016). Recent Advances in Cytokine Detection by Immunosensing. Biosens. Bioelectron. 79, 810–821. doi:10.1016/j.bios.2016.01.020
Liu, C., Zeng, X., An, Z., Yang, Y., Eisenbaum, M., Gu, X., et al. (2018). Sensitive Detection of Exosomal Proteins via a Compact Surface Plasmon Resonance Biosensor for Cancer Diagnosis. ACS Sens. 3 (8), 1471–1479. doi:10.1021/acssensors.8b00230
Liu, G., Gurung, A., and Qiu, W. (2019). Lateral Flow Aptasensor for Simultaneous Detection of Platelet-Derived Growth Factor-BB (PDGF-BB) and Thrombin. Molecules 24 (4), 756. doi:10.3390/molecules24040756
Loo, S. W., and Pui, T.-S. (2020). Cytokine and Cancer Biomarkers Detection: The Dawn of Electrochemical Paper-Based Biosensor. Sensors 20 (7), 1854. doi:10.3390/s20071854
Loo, L., Capobianco, J. A., Wu, W., Gao, X., Shih, W. Y., Shih, W.-H., et al. (2011). Highly Sensitive Detection of HER2 Extracellular Domain in the Serum of Breast Cancer Patients by Piezoelectric Microcantilevers. Anal. Chem. 83 (9), 3392–3397. doi:10.1021/ac103301r
Luo, B., Wang, Y., Lu, H., Wu, S., Lu, Y., Shi, S., et al. (2019). Label-free and Specific Detection of Soluble Programmed Death Ligand-1 Using a Localized Surface Plasmon Resonance Biosensor Based on Excessively Tilted Fiber Gratings. Biomed. Opt. Express 10 (10), 5136–5148. doi:10.1364/BOE.10.005136
Luo, X., Zhou, W., Yan, X., Guo, T., Wang, B., Xia, H., et al. (2020). Prognostic Value of C-Reactive Protein in Patients with Coronavirus 2019. Clin. Infect. Dis. 71 (16), 2174–2179. doi:10.1093/cid/ciaa641
Mahoney, K. M., Freeman, G. J., and McDermott, D. F. (2015). The Next Immune-Checkpoint Inhibitors: PD-1/PD-L1 Blockade in Melanoma. Clin. Ther. 37 (4), 764–782. doi:10.1016/j.clinthera.2015.02.018
Malhotra, R., Patel, V., Vaqué, J. P., Gutkind, J. S., and Rusling, J. F. (2010). Ultrasensitive Electrochemical Immunosensor for Oral Cancer Biomarker IL-6 Using Carbon Nanotube Forest Electrodes and Multilabel Amplification. Anal. Chem. 82 (8), 3118–3123. doi:10.1021/ac902802b
Malhotra, R., Patel, V., Chikkaveeraiah, B. V., Munge, B. S., Cheong, S. C., Zain, R. B., et al. (2012). Ultrasensitive Detection of Cancer Biomarkers in the Clinic by Use of a Nanostructured Microfluidic Array. Anal. Chem. 84 (14), 6249–6255. doi:10.1021/ac301392g
Mao, Z., Zhao, J., Chen, J., Hu, X., Koh, K., and Chen, H. (2021). A Simple and Direct SPR Platform Combining Three-In-One Multifunctional Peptides for Ultra-sensitive Detection of PD-L1 Exosomes. Sens Actuators B Chem. 346, 130496. doi:10.1016/j.snb.2021.130496
Marcucci, F., Rumio, C., and Corti, A. (2017). Tumor Cell-Associated Immune Checkpoint Molecules - Drivers of Malignancy and Stemness. Biochim Biophys. Acta (BBA) - Rev. Cancer 1868 (2), 571–583. doi:10.1016/j.bbcan.2017.10.006
Menon, S., Mathew, M. R., Sam, S., Keerthi, K., and Kumar, K. G. (2020). Recent Advances and Challenges in Electrochemical Biosensors for Emerging and Re-emerging Infectious Diseases. J. Electroanal. Chem. 878, 114596. doi:10.1016/j.jelechem.2020.114596
Michalaki, V., Syrigos, K., Charles, P., and Waxman, J. (2004). Serum Levels of IL-6 and TNF-α Correlate with Clinicopathological Features and Patient Survival in Patients with Prostate Cancer. Br. J. Cancer 90 (12), 2312–2316. doi:10.1038/sj.bjc.6601814
Mojic, M., Takeda, K., and Hayakawa, Y. (2018). The Dark Side of IFN-γ: Its Role in Promoting Cancer Immunoevasion. Ijms 19 (1), 89. doi:10.3390/ijms19010089
Mu, C.-Y., Qin, P.-X., Qu, Q.-X., Chen, C., and Huang, J.-A. (2015). Soluble CD40 in Plasma and Malignant Pleural Effusion with Non-small Cell Lung Cancer: A Potential Marker of Prognosis. Chronic Dis. Transl. Med. 1 (1), 36–41. doi:10.1016/J.CDTM.2015.02.010
Munge, B. S., Krause, C. E., Malhotra, R., Patel, V., Silvio Gutkind, J., and Rusling, J. F. (2009). Electrochemical Immunosensors for Interleukin-6. Comparison of Carbon Nanotube Forest and Gold Nanoparticle Platforms. Electrochem. Commun. 11 (5), 1009–1012. doi:10.1016/j.elecom.2009.02.044
Nagato, T., Ohkuri, T., Ohara, K., Hirata, Y., Kishibe, K., Komabayashi, Y., et al. (2017). Programmed Death-Ligand 1 and its Soluble Form Are Highly Expressed in Nasal Natural killer/T-Cell Lymphoma: a Potential Rationale for Immunotherapy. Cancer Immunol. Immunother. 66 (7), 877–890. doi:10.1007/s00262-017-1987-x
Nakashima, J., Tachibana, M., Horiguchi, Y., Oya, M., Ohigashi, T., Asakura, H., et al. (2000). Serum Interleukin 6 as a Prognostic Factor in Patients with Prostate Cancer. Clin. Cancer Res. 6 (7), 2702–2706. PMID: 10914713.
Nguyen, H. H., Lee, S. H., Lee, U. J., Fermin, C. D., and Kim, M. (2019). Immobilized Enzymes in Biosensor Applications. Materials 12 (1), 121. doi:10.3390/ma12010121
Niedziałkowski, P., Bojko, M., Ryl, J., Wcisło, A., Spodzieja, M., Magiera-Mularz, K., et al. (2021). Ultrasensitive Electrochemical Determination of the Cancer Biomarker Protein sPD-L1 Based on a BMS-8-Modified Gold Electrode. Bioelectrochemistry 139, 107742. doi:10.1016/j.bioelechem.2021.107742
Nielsen, C., Ohm-Laursen, L., Barington, T., Husby, S., and Lillevang, S. T. (2005). Alternative Splice Variants of the Human PD-1 Gene. Cell. Immunol. 235 (2), 109–116. doi:10.1016/j.cellimm.2005.07.007
Oaks, M. K., Hallett, K. M., Penwell, R. T., Stauber, E. C., Warren, S. J., and Tector, A. J. (2000). A Native Soluble Form of CTLA-4. Cell. Immunol. 201 (2), 144–153. doi:10.1006/cimm.2000.1649
Oh, B.-R., Huang, N.-T., Chen, W., Seo, J. H., Chen, P., Cornell, T. T., et al. (2014). Integrated Nanoplasmonic Sensing for Cellular Functional Immunoanalysis Using Human Blood. ACS Nano 8 (3), 2667–2676. doi:10.1021/nn406370u
Okuma, Y., Hosomi, Y., Nakahara, Y., Watanabe, K., Sagawa, Y., and Homma, S. (2017). High Plasma Levels of Soluble Programmed Cell Death Ligand 1 Are Prognostic for Reduced Survival in Advanced Lung Cancer. Lung Cancer 104, 1–6. doi:10.1016/j.lungcan.2016.11.023
Otieno, B. A., Krause, C. E., Latus, A., Chikkaveeraiah, B. V., Faria, R. C., and Rusling, J. F. (2014). On-line Protein Capture on Magnetic Beads for Ultrasensitive Microfluidic Immunoassays of Cancer Biomarkers. Biosens. Bioelectron. 53, 268–274. doi:10.1016/j.bios.2013.09.054
Owen, J. A., Punt, J., and Stranford, S. A. (2013). Kuby Immunology. New York, NY, USA: W. H. Freeman, 574.
Pan, L.-H., Kuo, S.-H., Lin, T.-Y., Lin, C.-W., Fang, P.-Y., and Yang, H.-W. (2017). An Electrochemical Biosensor to Simultaneously Detect VEGF and PSA for Early Prostate Cancer Diagnosis Based on Graphene oxide/ssDNA/PLLA Nanoparticles. Biosens. Bioelectron. 89, 598–605. doi:10.1016/j.bios.2016.01.077
Pang, Y., Shi, J., Yang, X., Wang, C., Sun, Z., and Xiao, R. (2020). Personalized Detection of Circling Exosomal PD-L1 Based on Fe3O4@TiO2 Isolation and SERS Immunoassay. Biosens. Bioelectron. 148, 111800. doi:10.1016/j.bios.2019.111800
Pérez-Romero, K., Rodríguez, R. M., Amedei, A., Barceló-Coblijn, G., and Lopez, D. H. (2020). Immune Landscape in Tumor Microenvironment: Implications for Biomarker Development and Immunotherapy. Ijms 21 (15), 5521. doi:10.3390/ijms21155521
Pohanka, M. (2018). Piezoelectric Biosensor for the Determination of Tumor Necrosis Factor Alpha. Talanta 178, 970–973. doi:10.1016/j.talanta.2017.10.031
Pourmadadi, M., Shayeh, J. S., Arjmand, S., Omidi, M., and Fatemi, F. (2020). An Electrochemical Sandwich Immunosensor of Vascular Endothelial Growth Factor Based on Reduced Graphene Oxide/gold Nanoparticle Composites. Microchem. J. 159, 105476. doi:10.1016/j.microc.2020.105476
Punyani, S. R., and Sathawane, R. S. (2013). Salivary Level of Interleukin-8 in Oral Precancer and Oral Squamous Cell Carcinoma. Clin. Oral Invest. 17 (2), 517–524. doi:10.1007/s00784-012-0723-3
Purohit, B., Vernekar, P. R., Shetti, N. P., and Chandra, P. (2020). Biosensor Nanoengineering: Design, Operation, and Implementation for Biomolecular Analysis. Sensors Int. 1, 100040. doi:10.1016/j.sintl.2020.100040
Ramos-Sono, D., Laureano, R., Rueda, D., Gilman, R. H., La Rosa, A., Ruiz, J., et al. (2020). An Electrochemical Biosensor for the Detection of Mycobacterium tuberculosis DNA from Sputum and Urine Samples. PloS one 15 (10), e0241067. doi:10.1371/journal.pone.0241067
Razmi, N., Baradaran, B., Hejazi, M., Hasanzadeh, M., Mosafer, J., Mokhtarzadeh, A., et al. (2018). Recent Advances on Aptamer-Based Biosensors to Detection of Platelet-Derived Growth Factor. Biosens. Bioelectron. 113, 58–71. doi:10.1016/j.bios.2018.04.048
Reza, K. K., Sina, A. A. I., Wuethrich, A., Grewal, Y. S., Howard, C. B., Korbie, D., et al. (2019). A SERS Microfluidic Platform for Targeting Multiple Soluble Immune Checkpoints. Biosens. Bioelectron. 126, 178–186. doi:10.1016/j.bios.2018.10.044
Riedel, F., Zaiss, I., Herzog, D., Götte, K., Naim, R., and Hörmann, K. (2005). Serum Levels of Interleukin-6 in Patients with Primary Head and Neck Squamous Cell Carcinoma. Anticancer Res. 25 (4), 2761–2765. PMID: 16080523.
Roselli, M., Mineo, T. C., Basili, S., Martini, F., Mariotti, S., Aloe, S., et al. (2004). Soluble CD40 Ligand Plasma Levels in Lung Cancer. Clin. Cancer Res. 10 (2), 610–614. doi:10.1158/1078-0432.ccr-0348-03
Russell, C., Ward, A. C., Vezza, V., Hoskisson, P., Alcorn, D., Steenson, D. P., et al. (2019). Development of a Needle Shaped Microelectrode for Electrochemical Detection of the Sepsis Biomarker Interleukin-6 (IL-6) in Real Time. Biosens. Bioelectron. 126, 806–814. doi:10.1016/j.bios.2018.11.053
Sardesai, N. P., Barron, J. C., and Rusling, J. F. (2011). Carbon Nanotube Microwell Array for Sensitive Electrochemiluminescent Detection of Cancer Biomarker Proteins. Anal. Chem. 83 (17), 6698–6703. doi:10.1021/ac201292q
Sardesai, N. P., Kadimisetty, K., Faria, R., and Rusling, J. F. (2013). A Microfluidic Electrochemiluminescent Device for Detecting Cancer Biomarker Proteins. Anal. Bioanal. Chem. 405 (11), 3831–3838. doi:10.1007/s00216-012-6656-5
Setrerrahmane, S., and Xu, H. (2017). Tumor-related Interleukins: Old Validated Targets for New Anti-cancer Drug Development. Mol. Cancer 16 (1), 1–17. doi:10.1186/s12943-017-0721-9
Shami, A., Edsfeldt, A., Shore, A. C., Natali, A., Khan, F., Nilsson, J., et al. (2020). CD40 Levels in Plasma Are Associated with Cardiovascular Disease and in Carotid Plaques with a Vulnerable Plaque Phenotype and Remodelling. Eur. Heart J. 41 (Suppl. ment_2), ehaa946–3782. doi:10.1093/ehjci/ehaa946.3782
Sharpe, A. H. (2017). Introduction to Checkpoint Inhibitors and Cancer Immunotherapy. Immunol. Rev. 276 (1), 5–8. doi:10.1111/imr.12531
Singh, M., Truong, J., Reeves, W., and Hahm, J.-i. (2017). Emerging Cytokine Biosensors with Optical Detection Modalities and Nanomaterial-Enabled Signal Enhancement. Sensors 17 (2), 428. doi:10.3390/s17020428
Slaney, C. Y., Kershaw, M. H., and Darcy, P. K. (2014). Trafficking of T Cells into Tumors. Cancer Res. 74 (24), 7168–7174. doi:10.1158/0008-5472.CAN-14-2458
Smith-Garvin, J. E., Koretzky, G. A., and Jordan, M. S. (2009). T Cell Activation. Annu. Rev. Immunol. 27, 591–619. doi:10.1146/annurev.immunol.021908.132706
Sorensen, S. F., Demuth, C., Weber, B., Sorensen, B. S., and Meldgaard, P. (2016). Increase in Soluble PD-1 Is Associated with Prolonged Survival in Patients with Advanced EGFR -mutated Non-small Cell Lung Cancer Treated with Erlotinib. Lung Cancer 100, 77–84. doi:10.1016/j.lungcan.2016.08.001
Suvarnaphaet, P., and Pechprasarn, S. (2017). Graphene-based Materials for Biosensors: A Review. Sensors 17, 2161. doi:10.3390/s17102161
Tamayo, J., Kosaka, P. M., Ruz, J. J., San Paulo, Á., and Calleja, M. (2013). Biosensors Based on Nanomechanical Systems. Chem. Soc. Rev. 42 (3), 1287–1311. doi:10.1039/C2CS35293A
Taylor, L., and Schwarz, H. (2001). Identification of a Soluble OX40 Isoform: Development of a Specific and Quantitative Immunoassay. J. Immunol. Methods 255 (1-2), 67–72. doi:10.1016/S0022-1759(01)00424-0
Tertis, M., Leva, P. I., Bogdan, D., Suciu, M., Graur, F., and Cristea, C. (2019). Impedimetric Aptasensor for the Label-free and Selective Detection of Interleukin-6 for Colorectal Cancer Screening. Biosens. Bioelectron. 137, 123–132. doi:10.1016/j.bios.2019.05.012
Thiviyanathan, V., and Gorenstein, D. G. (2012). Aptamers and the Next Generation of Diagnostic Reagents. Prot. Clin. Appl. 6 (11-12), 563–573. doi:10.1002/prca.201200042
Tkach, M., and Théry, C. (2016). Communication by Extracellular Vesicles: where We Are and where We Need to Go. Cell 164 (6), 1226–1232. doi:10.1016/j.cell.2016.01.043
Triebel, F., Hacene, K., and Pichon, M.-F. (2006). A Soluble Lymphocyte Activation Gene-3 (sLAG-3) Protein as a Prognostic Factor in Human Breast Cancer Expressing Estrogen or Progesterone Receptors. Cancer Lett. 235 (1), 147–153. doi:10.1016/j.canlet.2005.04.015
Valverde, A., Serafín, V., Garoz, J., Montero-Calle, A., González-Cortés, A., Arenas, M., et al. (2020). Electrochemical Immunoplatform to Improve the Reliability of Breast Cancer Diagnosis through the Simultaneous Determination of RANKL and TNF in Serum. Sens. Actuators B Chem. 314, 128096. doi:10.1016/j.snb.2020.128096
van den Hurk, R., and Evoy, S. (2013). Deflection Cantilever Detection of Interferon Gamma. Sens. Actuators B Chem. 176, 960–965. doi:10.1016/j.snb.2012.09.023
van den Kieboom, C. H., van der Beek, S. L., Mészáros, T., Gyurcsányi, R. E., Ferwerda, G., and de Jonge, M. I. (2015). Aptasensors for Viral Diagnostics. TrAC Trends Anal. Chem. 74, 58–67. doi:10.1016/j.trac.2015.05.012
Vidotti, M., Carvalhal, R. F., Mendes, R. K., Ferreira, D. C. M., and Kubota, L. T. (2011). Biosensors Based on Gold Nanostructures. J. Braz. Chem. Soc. 22, 3–20. doi:10.1590/S0103-50532011000100002
Wang, X., and Lin, Y. (2008). Tumor Necrosis Factor and Cancer, Buddies or Foes? Acta Pharmacol. Sin. 29 (11), 1275–1288. doi:10.1111/j.1745-7254.2008.00889.x
Wang, L., Wang, H., Chen, H., Wang, W.-d., Chen, X.-q., Geng, Q.-r., et al. (2015). Serum Levels of Soluble Programmed Death Ligand 1 Predict Treatment Response and Progression Free Survival in Multiple Myeloma. Oncotarget 6 (38), 41228–41236. doi:10.18632/oncotarget.5682
Wang, L., Chang, Y., Xu, J., and Zhang, Q. (2016). Predictive Significance of Serum Level of Vascular Endothelial Growth Factor in Gastric Cancer Patients. BioMed Res. Int. 2016, 1–6. doi:10.1155/2016/8103019
Wang, Z.-y., Li, W., Gong, Z., Sun, P.-r., Zhou, T., and Cao, X.-w. (2019). Detection of IL-8 in Human Serum Using Surface-Enhanced Raman Scattering Coupled with Highly-Branched Gold Nanoparticles and Gold Nanocages. New J. Chem. 43 (4), 1733–1742. doi:10.1039/C8NJ05353G
Ward, L. D., Shi, P. T., and Simpson, R. J. (1992). Binding of Anti-human-interleukin-6 Monoclonal Antibodies to Synthetic Peptides of Human Interleukin-6 Studied Using Surface Plasmon Resonance. Biochem. Int. 26 (3), 559–565. PMID: 1627166.
Ward-Kavanagh, L. K., Lin, W. W., Šedý, J. R., and Ware, C. F. (2016). The TNF Receptor Superfamily in Co-stimulating and Co-inhibitory Responses. Immunity 44 (5), 1005–1019. doi:10.1016/j.immuni.2016.04.019
Waugh, D. J. J., and Wilson, C. (2008). The Interleukin-8 Pathway in Cancer. Clin. Cancer Res. 14 (21), 6735–6741. doi:10.1158/1078-043210.1158/1078-0432.ccr-07-4843
Wu, J., He, J., Zhang, Y., Zhao, Y., Niu, Y., and Yu, C. (2017). Reusable Voltammetric Immunosensor for sCD40L, a Biomarker for the Acute Coronary Syndrome, Using a Glassy Carbon Electrode Modified with a Nanocomposite Consisting of Gold Nanoparticles, Branched Polyethylenimine and Carboxylated Multiwalled Carbon Nanotubes. Microchim. Acta 184 (6), 1837–1845. doi:10.1007/s00604-017-2192-5
Wu, Y., Belmonte, I., Sykes, K. S., Xiao, Y., and White, R. J. (2019). Perspective on the Future Role of Aptamers in Analytical Chemistry. Anal. Chem. 91 (24), 15335–15344. doi:10.1021/acs.analchem.9b03853
Wuethrich, A., Rajkumar, A. R., Shanmugasundaram, K. B., Reza, K. K., Dey, S., Howard, C. B., et al. (2019). Single Droplet Detection of Immune Checkpoints on a Multiplexed Electrohydrodynamic Biosensor. Analyst 144 (23), 6914–6921. doi:10.1039/C9AN01450K
Xing, Y., Liu, J., Sun, S., Ming, T., Wang, Y., Luo, J., et al. (2021). New Electrochemical Method for Programmed Death-Ligand 1 Detection Based on a Paper-Based Microfluidic Aptasensor. Bioelectrochemistry 140, 107789. doi:10.1016/j.bioelechem.2021.107789
Xu, Z., Zhang, T., Zhuang, R., Zhang, Y., Jia, W., Song, C., et al. (2009). Increased Levels of Soluble CD226 in Sera Accompanied by Decreased Membrane CD226 Expression on Peripheral Blood Mononuclear Cells from Cancer Patients. BMC Immunol. 10 (1), 1–8. doi:10.1186/1471-2172-10-34
Xu, J., Ye, Y., Zhang, H., Szmitkowski, M., Mäkinen, M., Li, P., et al. (2016). Diagnostic and Prognostic Value of Serum Interleukin-6 in Colorectal Cancer. Medicine 95 (2), e2502. doi:10.1097/md.0000000000002502
Yamamoto, R., Nishikori, M., Kitawaki, T., Sakai, T., Hishizawa, M., Tashima, M., et al. (2008). PD-1-PD-1 Ligand Interaction Contributes to Immunosuppressive Microenvironment of Hodgkin Lymphoma. Blood, J. Am. Soc. Hematol. 111 (6), 3220–3224. doi:10.1182/blood-2007-05-085159
Yan, S.-R., Foroughi, M. M., Safaei, M., Jahani, S., Ebrahimpour, N., Borhani, F., et al. (2020). A Review: Recent Advances in Ultrasensitive and Highly Specific Recognition Aptasensors with Various Detection Strategies. Int. J. Biol. Macromol. 155, 184–207. doi:10.1016/j.ijbiomac.2020.03.173
Yáñez-Mó, M., Siljander, P. R.-M., Andreu, Z., Bedina Zavec, A., Borràs, F. E., Buzas, E. I., et al. (2015). Biological Properties of Extracellular Vesicles and Their Physiological Functions. J. Extracell. Vesicles 4 (1), 27066. doi:10.3402/jev.v4.27066
Yang, L., and Zhang, Y. (2017). Tumor-associated Macrophages: from Basic Research to Clinical Application. J. Hematol. Oncol. 10 (1), 1–12. doi:10.1186/s13045-017-0430-2
Yuan, G., Yu, C., Xia, C., Gao, L., Xu, W., Li, W., et al. (2015). A Simultaneous Electrochemical Multianalyte Immunoassay of High Sensitivity C-Reactive Protein and Soluble CD40 Ligand Based on Reduced Graphene Oxide-Tetraethylene Pentamine that Directly Adsorb Metal Ions as Labels. Biosens. Bioelectron. 72, 237–246. doi:10.1016/j.bios.2015.04.088
Zahm, C. D., Colluru, V. T., McIlwain, S. J., Ong, I. M., and McNeel, D. G. (2018). TLR Stimulation during T-Cell Activation Lowers PD-1 Expression on CD8+ T Cells. Cancer Immunol. Res. 6 (11), 1364–1374. doi:10.1158/2326-6066.CIR-18-0243
Zhao, Y., He, J., Yuan, G., Xia, C., Li, Y., and Yu, C. (2015). Rapidly Accomplished Femtomole Soluble CD40 Ligand Detection in Human Serum: a “Green” Homobifunctional Agent Coupled with Reduced Graphene Oxide-Tetraethylene Pentamine as Platform. RSC Adv. 5 (107), 88392–88400. doi:10.1039/C5RA13440D
Zheng, Y., Fang, Y. C., and Li, J. (2019). PD-L1 Expression Levels on Tumor Cells Affect Their Immunosuppressive Activity. Oncol. Lett. 18 (5), 5399–5407. doi:10.3892/ol.2019.10903
Keywords: immunosensors, cancer, soluble immune checkpoint molecules, cytokines, diagnostic and prognostic markers, immunotherapy
Citation: Pandey N, Biswas D, Dutta N, Hansda A, Dutta G and Mukherjee G (2022) Sensing Soluble Immune Checkpoint Molecules and Disease-Relevant Cytokines in Cancer: A Novel Paradigm in Disease Diagnosis and Monitoring. Front. Sens. 3:789771. doi: 10.3389/fsens.2022.789771
Received: 05 October 2021; Accepted: 22 April 2022;
Published: 31 May 2022.
Edited by:
Juliana Cancino-Bernardi, University of São Paulo, BrazilReviewed by:
Kobra-Omidfar, Tehran University of Medical Sciences, IranCopyright © 2022 Pandey, Biswas, Dutta, Hansda, Dutta and Mukherjee. This is an open-access article distributed under the terms of the Creative Commons Attribution License (CC BY). The use, distribution or reproduction in other forums is permitted, provided the original author(s) and the copyright owner(s) are credited and that the original publication in this journal is cited, in accordance with accepted academic practice. No use, distribution or reproduction is permitted which does not comply with these terms.
*Correspondence: Gayatri Mukherjee, Z2F5YXRyaS5tdWtoZXJqZWVAc21zdC5paXRrZ3AuYWMuaW4=
†These authors have contributed equally to this work
Disclaimer: All claims expressed in this article are solely those of the authors and do not necessarily represent those of their affiliated organizations, or those of the publisher, the editors and the reviewers. Any product that may be evaluated in this article or claim that may be made by its manufacturer is not guaranteed or endorsed by the publisher.
Research integrity at Frontiers
Learn more about the work of our research integrity team to safeguard the quality of each article we publish.