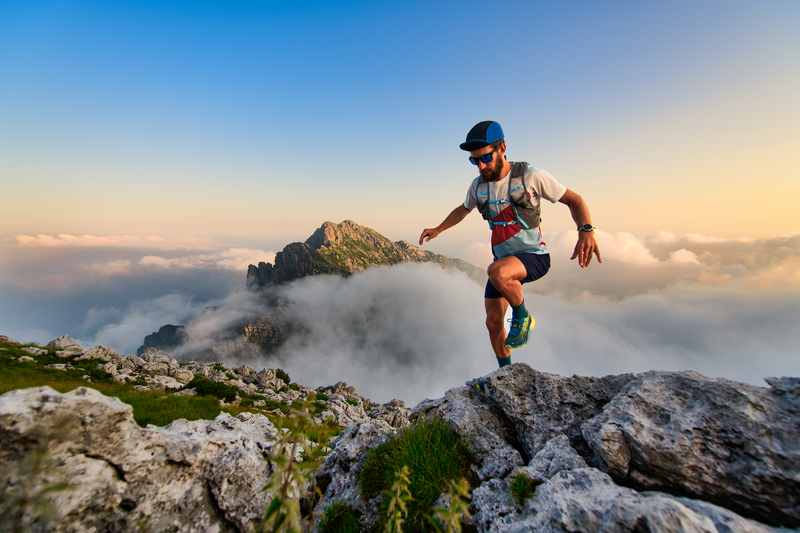
95% of researchers rate our articles as excellent or good
Learn more about the work of our research integrity team to safeguard the quality of each article we publish.
Find out more
ORIGINAL RESEARCH article
Front. Sens. , 10 August 2021
Sec. Chemical Sensors
Volume 2 - 2021 | https://doi.org/10.3389/fsens.2021.719161
This article is part of the Research Topic 2021 Retrospective: Frontiers in Sensors View all 7 articles
A modified carbon fibre yarn sensor was developed for the voltammetric determination of paracetamol and its interferents (dopamine and ascorbic acid). Reduced graphene oxide (rGO) was electrochemically deposited onto a carbon fibre yarn. Further modification was achieved using polypyrrole (PPy) coated onto the rGO carbon fibre yarn via electropolymerisation of pyrrole with cyclic voltammetry (CV). The surface of the rGO and PPy-rGO carbon fibre electrodes were characterised using Raman spectroscopy and scanning electron microscopy. The rGO and PPy-rGO carbon fibres had a 3.5-fold and 7-fold larger electrochemical surface area compared to bare carbon fibre (calculated using the Randles-Sevcik equation). Two clearly distinguished oxidation peaks at 0.49 and 0.25 V (vs. Ag/AgCl) were observed at the rGO fibre electrode during the simultaneous detection of paracetamol and dopamine, respectively, by CV. The detection limit (3σ S/N) of the rGO carbon fibre electrode for differential pulse voltammetry (DPV) determination of paracetamol was at 21.1 and 6.0 µM for dopamine. In comparison, the simultaneous determination of paracetamol and dopamine by CV at the PPy-rGO fibre electrode gave oxidation peaks of paracetamol and dopamine at 0.55 and 0.25 V (vs. Ag/AgCl), respectively. The detection limit (3σ S/N) for paracetamol was notably improved to 3.7 µM and maintained at 6.0 µM for dopamine at the PPy-rGO carbon fibre electrode during DPV.
Digital health devices that are capable of on-body drug detection are of interest to monitor therapeutic drugs and drugs of abuse as well as drugs in consumer goods (e.g. alcohol and caffeine). Electrochemical detection is possible for a wide range of drug molecules and would allow wearable sensing due to the ease of electronics integration with electrochemical detection. Paracetamol (acetaminophen) is a synthetic non-opioid analgesic and antipyretic drug, widely used to treat fever and relieve mild to moderate pain (Twycross et al., 2013; Dhanush et al., 2018; Atta et al., 2019). Paracetamol overdose results in toxicity, urinary problems and liver damage (Ghanbari and Bonyadi, 2018; Ponnaiah et al., 2018). The electrochemical detection of paracetamol is possible due to its oxidation to N-acetyl p-quinoneimine (Özcan and Şahin, 2007; Twycross et al., 2013). Interferents during paracetamol electrochemical analysis include dopamine, which coexists in human metabolic fluid and plays an essential role in neuronal physiology (Hao et al., 2019). Several neurological disorders, including schizophrenia, Alzheimer’s and Parkinson’s diseases are associated with insufficient levels of dopamine in the human body (Soltani et al., 2015; Ghanbari and Bonyadi, 2018; Farajikhah et al., 2019). In the central nervous system, dopamine functions to control neural interactions by decreasing permeability of gap junctions between adjoining neurons of the same type (Selvaraju and Ramaraj, 2003; Babaei and Taheri, 2013; Hasanzadeh et al., 2013).
Drug monitoring is necessary to maintain drug efficacy and safety when delivered to a patient. The co-occurrence of paracetamol and interferents in physiological fluids such as blood serum and human urine can be electrochemically determined and their concentrations monitored with selective and cost-effective sensors (Dhanush et al., 2018; Ghanbari and Bonyadi, 2018). Different substrate materials have been used to develop flexible and wearable electrodes in skin patches, yarns for textiles, and temporary tattoos (Bandodkar and Wang, 2014). It is essential that these types of sensors are durable and lightweight, which makes carbon fibres and yarns ideal as an electrode material due to its low density and flexible mechanical properties (Jost et al., 2013; Huang et al., 2019). Metal-based fibres have also received attention because of their high electrical conductivities but they are typically more brittle and prone to being oxidised under ambient conditions (Le et al., 2013; Abdul Bashid et al., 2017). Carbon-based fibres enjoy an excellent balance between electrical conductivity, corrosion resistance, strength and flexibility (Han and Kumar, 2008; Khan et al., 2012; Le et al., 2013; Gandara and Gonçalves, 2020). In addition, carbon yarns have a conductive 3D micro-structure, which may help to decrease the packing of graphene sheets during the deposition of reduced graphene oxide (rGO) to allow better exploitation of the properties of this 2D material (Almeida et al., 2019).
Surface modification of electrodes with nanomaterials shows significant improvement during electrochemical sensing of drugs compared to the non-modified electrode (Palakollu et al., 2020). Graphene and its composites have been the focus of much research due to its electrical conductivity, large surface area and high electrocatalytic activity. rGO and its hybrids are widely studied as potential electrode materials for electrochemical sensors and biosensors (Selvam et al., 2016; Yang et al., 2018; Ramezani et al., 2019; Shukla et al., 2020). rGO is produced by the reduction of graphene oxide (GO) from aqueous solutions, making it suitable for a wide variety of bio-based applications. Further enhancement of electrochemical properties of carbon hybrid materials can be achieved when in combination with conducting polymers. The ease of preparation, good electrical conductivity and ductility of conducting polymers means they are highly applicable for wearable devices (Ponnaiah et al., 2018; Zhang et al., 2018). Polypyrrole (PPy) has been extensively studied as a sensing electrode, due to its electrochemical switching and redox properties (Li et al., 2005; Ateh et al., 2006; Kaur et al., 2015; Tang et al., 2015; Huang et al., 2016; Kim et al., 2016). PPy electrodes can however suffer from low electrical performance and poor cycling stability. To tackle this challenge, considerable efforts have been made to combine PPy-based materials with graphene or its derivatives. (Liang et al., 2015; Abdul Bashid et al., 2017; Atikah Md Jani et al., 2017; Rasouli et al., 2018; Zhang et al., 2018). RGO is mainly made up of sp2 hybridized carbon atoms, decorated with small amounts of hydroxyl (‒OH), carboxy (‒COOH), or epoxide (‒O‒) groups. Oxygen-containing groups on rGO behave as sites to promote the surface activity of PPy.
This paper describes the optimisation of flexible rGO-coated and PPy-rGO-coated carbon-fibre yarns for drug sensing. While many publications deal with surface modification of bulk electrodes, there are few examples of flexible and fibre-based electrode modification that could be used for wearable applications. Puthongkham et al. (2018) describe nanohorn-modified carbon fiber microelectrodes for dopamine detection, while Farajikhah et al. (2019) have published rGO-modified stainless steel filaments for detection of dopamine. Here we optimise rGO and PPy-rGO deposition onto carbon fibre yarns for paracetamol detection. To demonstrate the ability of the rGO-coated and PPy-rGO-coated fibre electrodes to sense drugs, monitoring of model drug paracetamol was explored alongside the common interferent dopamine and ascorbic acid. The electrochemical behaviour of these drugs at the fibre electrodes was studied using CV and DPV. Additionally, the surface morphologies, areas, and chemistries of the fibre electrodes were analysed.
Analytical grade pyrrole (98%) was purchased from Sigma-Aldrich (United Kingdom), stored at 0°C and was freshly distilled before use. Sodium dodecyl sulfate (SDS), potassium ferricyanide (K3 [Fe(CN)6]), potassium chloride (KCl), paracetamol, dopamine hydrochloride and l-ascorbic acid were also purchased from Sigma-Aldrich (United Kingdom). Phosphate buffered saline (PBS) tablets were purchased from Fisher Scientific (United Kingdom). Carbon fibre (CAS nuber 7,440–44–0) was purchased from Alfa Aesar (United Kingdom).
Cyclic voltammetry (CV) was performed using an eDAQ EA161 potentiostat combined with e-corder 401 and EChem V2.1.16 software. Differential pulse voltammetry (DPV) was performed using a Palmsens4 potentiostat with PS Trace software. A Basi® Ag/AgCl (3.0 M NaCl internal solution) was used as a reference electrode and a platinum mesh was used as a counter electrode. Raman spectroscopy was performed using a Renishaw inVia confocal Raman microscope with a 785 nm laser. Scanning electron microscopy (SEM) images were obtained using a JEOL United States JSM-7100 F field emission electron microscope combined with Pathfinder 2.5 X-ray microanalysis software for SEM/EDS. X-ray photoelectron spectroscopy (XPS) data was obtained using a ThermoFisher Scientific Theta Probe spectrometer with a monochromated Al K X-ray source. An X-ray spot of ∼400 μm radius was employed in the acquisition of all spectra. Survey spectra were acquired employing a pass energy of 300 eV. High resolution, core level spectra for S2p were acquired with a pass energy of 50 eV.
Graphene Oxide (GO) dispersion was prepared using the modified Hummers method (Marcano et al., 2010; Zhu et al., 2012; Liu et al., 2015). Firstly, a mixture of concentrated H2SO4/H3PO4 (360:40 ml) was added to a mixture of graphite flakes (3.0 g, 1 wt. equiv.) and KMnO4 (18.0 g, 6 wt. equiv.). The reaction was heated to 55°C and stirred for 14.5 h, then cooled to room temperature, and poured onto ice (∼400 ml) with 30% H2O2 (20 ml). The mixture was then stirred for 30 min and centrifuged at 4,400 rpm for 20 min. The precipitate was washed and centrifuged with HCl solution (9:1 water/HCl by volume) twice and then dispersed in DI water and dialyzed for 7 days. The graphene oxide dispersion was finally obtained by probe sonicating (Branson Digital sonifier, 400 W, 38% amplitude) the purified graphite oxide dispersion for 1 h, with a pulse of 2 seconds on and one second off, totally 1.5 h. To prepare the dried GO powder, the prepared GO dispersion (12.3 mg/ml) was then put into Freeze-dryer Alpha 1-4 LDplus (48 h, −55°C) to make dried GO powder.
Dried GO powder was dissolved in an aqueous solution containing 0.1 M PBS solution used as an electrolyte, resulting in an electrodeposition solution at a concentration of 0.5 mg ml−1. Deposition techniques used in this study were potentiostatic deposition (a constant potential applied at −1.2 V vs. Ag/AgCl for 5 min) and CV (a deposition potential range of −1.4 to +1.4 V vs. Ag/AgCl at a scan rate of 50 mV s−1; the number of scans varied from 3 to 20). The electropolymerisation solution containing pyrrole (0.2 M) and SDS (0.2 M) were used to optimise the PPy-rGO sensing layer, performed by five scans CV (an electropolymerised potential range of −0.2 to +1.0 V vs. Ag/AgCl and a scan rate of 50 mV s−1). All of the as-prepared fibre electrodes were washed with deionised water and then dried under ambient conditions before further sensing.
The ECSA (A) of the sensing fibre was measured using CV at different scan rate, and 5 min N2 purged aqueous solution of 10 mM K3 [Fe(CN)6] in 0.1 M KCl supporting electrolyte, and calculated using the Randles - Sevcik equation as follows (Nayak and Shetti, 2016; Shetti et al., 2017):
where Ip is the anodic peak current, n refers to the number of electrons transferred during the electrode reaction (n = 1 in this study), D0 is the diffusion coefficient (7.6 × 10–6 cm2 s−1), ν is the potential scan rate, and C0 is the concentration of K3 [Fe(CN)6].
The rGO-coated and PPy-rGO-coated carbon fibre electrodes were examined in the presence of paracetamol, dopamine and ascorbic acid (an interferents commonly found in the blood). The current response of the fibre electrodes to paracetamol and interferents was tested (Ghanbari and Bonyadi, 2018). Stock solutions 1 mM analytes (drug or interferent) was prepared in 0.1 M PBS solution. The analyte solutions (0–500 µM) used in the electrochemical experiments were prepared by dilution of these stock solutions. CV was conducted with a potential range of −0.6 to +0.9 V vs. Ag/AgCl and a scan rate of 50 mV s−1. For DPV, the following parameters were used: pulse amplitude = 50 mV; pulse width = 40 ms; voltage step = 50 mV, and a scan rate = 10 mV s−1 (potential range uses was −1.0 to +1.0 V vs. Ag/AgCl).
The electrochemical reduction of GO was carried out using a neutral (pH = 7.4) aqueous GO dispersion (0.5 mg ml−1) by either potentiostatic deposition or cyclic voltammetry (CV). For potentiostatic deposition, an electrode potential of −1.2 V (vs Ag/AgCl) was applied for 5 min. During CV, a potential range of −1.4 to +1.4 V (vs Ag/AgCl) was scanned for 5, 10, 15 and 20 cycles to deposit rGO onto carbon fibres (Lin et al., 2019). The electrochemical reduction of GO is described by the equation below (Selvam et al., 2016):
Supplementary Figure S1A shows voltammograms during 20 cycles of GO reduction onto carbon fibres. Two cathodic peaks appeared at approximately −0.45 and 0.2 V (vs Ag/AgCl) and the increase in the peak currents were attributed to the deposition of rGO on the bare carbon fibre surfaces (Selvam et al., 2016). The reduction peak was at a similar potential to that previously reported for the electrochemical reduction of GO in PBS on a glassy carbon electrode (Chen et al., 2011) (approximately 0.30 V vs SCE which is equivalent to ca. 0.35 V vs Ag/AgCl). The reduction peak of the GO layer deposited onto a roughened Au electrode was observed at approximately −0.7 V (vs Ag/AgCl) (Olejnik et al., 2014). PPy was electrochemically deposited using CV onto a subset of rGO-coated carbon fibres (made using 20 CV scans) to give a PPy.SDS coating (main graph in Supplementary Figure S1B). CVs following polymerisation gave an oxidation peak potential at 0.2 V (vs. Ag/AgCl) for the PPy.SDS-coated rGO on carbon fibres, with no clear reduction peaks observed (Supplementary Figure S1B inset). These results compare to an oxidation peak potential of 0.09 V (vs. Ag/AgCl) found for a PPy.KNO3. SDS film reported by Nikoofard et al. (2014).
The Raman spectra of rGO-coated carbon fibres and PPy‒rGO-coated carbon fibres are shown in Figure 1. Characteristic D and G bands of the carbonaceous materials of bare carbon fibre appear at 1,350 and 1,587 cm−1 respectively. In comparison, the rGO-coated carbon fibres exhibit dominant peaks corresponding to D and G bands in the range of 1,348–1,363 cm−1 and 1,589–1,599 cm−1, respectively (Farajikhah et al., 2019; Shukla et al., 2020). The Raman spectra of potentiostatic deposition of rGO on carbon fibre and three cycles CV rGO on carbon fibre are displayed in Supplementary Figure S2. Potentiostatic deposition of rGO and three cycles CV rGO on carbon fibre also exhibit two dominant peaks corresponding to D and G bands at 1,337 and 1,592 cm−1, and 1,325 and 1,598 cm−1, respectively. The D band is attributed to disordered structural defects that relate to the conversion of sp2 hybridised carbon to sp3 hybridised carbon, whereas the G band is assigned to the vibrational mode of E2g phonon of sp2 carbon atoms (the stretching of the C=C bond) (Atikah Md Jani et al., 2017; Ramesh et al., 2018; Yang et al., 2018; Zhang et al., 2018). The Raman spectra of all samples show a shift for the D and G bands corresponding to the increase in the electrodeposition scans.
FIGURE 1. (A) Raman spectra (785 nm laser) of rGO coated carbon fibre using 5 CV scans (red), 10 CV scans (magenta), 15 CV scans (green), 20 CV scans (orange), and PPy‒rGO coated carbon fibre (blue, made using 5 CV scans and 0.2 M pyrrole and SDS solution with 20 CV scans rGO); (B) Raman spectra (785 nm laser) of a PPy.SDS coated carbon fibre (blue, made using 5 CV scans and 0.2 M SDS solution), and PPy‒rGO coated carbon fibre (grey, made using 5 CV scans and 0.2 M pyrrole and SDS solution electropolymerised on 20 CV scans rGO coated carbon fibre).
The ratio of D/G bands (ID/IG) was measured to be 0.97 for bare carbon fibre. The variation in ID/IG intensity with respect to the different electrodeposition conditions was investigated and an increase in the ID/IG ratio was found following 5, 10 and 15 cycles of rGO electrodeposition. This corresponds to the formation of new smaller graphitic domains, indicating a reduction of rGO (Ramesh et al., 2018; Farajikhah et al., 2019). In contrast, the ID/IG ratio of an rGO-coated carbon fibre made from 20 cycles decreased to 0.89 indicating that oxygen functional groups of GO were removed, which led to a decrease in the ID/IG ratio compared to the bare carbon fibre. This suggests that the initial layers of rGO deposited on carbon fibre have more structural defects (rGO character) than the subsequent electrodeposited layers. Johra et al. (2014) suggested that the ID/IG ratio decreased during the reduction of graphene oxide compared to GO, due to the repair of defects by recovery of aromatic structures.
Two additional peaks were observed following rGO depostion at approximately 2,700 and 2,900 cm−1, corresponding to the 2D band and (D + G) combination mode, respectively (Antony et al., 2015). The 2D band relates to the number of graphene layers and a broadened band is attributed to rGO consisting of few layers with some defects. The (D + G) combination mode is a second‒order peak derived from the combination of D‒G peak. The electrochemical reduction is accompanied by a lower oxygen content in graphene. The intensities of both peaks increased in the case of graphene compared to those of GO, indicating better graphitisation (Johra et al., 2014).
The Raman spectra of the PPy‒rGO coating at the surface of the fibre electrode (electropolymerised from 5 CV scans in 0.2 M pyrrole and 0.2 M SDS solution onto carbon fibre coated with rGO using 20 CV cycles) are depicted in Figure 1B. The growth of PPy at the surface of the modified rGO electrode is confirmed from the Raman spectra of the PPy‒rGO coated carbon fibre, showing peaks characteristic of PPy (grey line). The C=C stretching mode associated with the polymer backbone overlaps with the G-band of rGO at 1,578 cm−1. The skeletal and antisymmetrical C-N stretching modes are observed at 1,488 cm−1 and 1,382 cm−1, respectively. The peaks at 932 cm−1 and 982 cm−1 are associated with ring distortion, while the peaks at 1,052 cm−1 and 1,079 cm−1 are due to symmetric C-H in-plane bending modes. The ring distortion (932 cm−1) and C-H in-plane bending (1,079 cm−1) modes are related to the PPy bipolaron structure, while the peaks at 982 cm−1 and 1,052 cm−1 are related to the PPy polaron structure (Lynam et al., 2007; Garcia-Torres and Crean, 2018). The Raman spectra show that both polaron and bipolaron charge carriers are present in the PPy‒rGO coated carbon with relative bipolaron to polaron peak intensity ratios I932/I982 of 0.82 (peak intensities of 9,715 and 11,813 arb.) and I1079/I1052 of 0.8 (peak intensities of 9,131 and 11,409 arb.). This indicates that the polaron charge carriers dominate in these electropolymerised coatings.
The surface morphology is an important parameter of coatings used as electrode materials in sensors. SEM images (Figure 2A) illustrate how the electrodeposited rGO interphases vary with the deposition method and materials used (e.g., PPy-rGO fibre electrode). Bare carbon fibre has a clear and smooth surface. After electrodeposition, rGO has a loose structure on the outer surface of the carbon fibre. The surface morphology of the rGO material deposited potentiostatically and the rGO deposited after 10 CV scans (Supplementary Figure S3) show an area of the carbon fibre that is covered with rGO, while the deposition of rGO (from both 15 and 20 CV scans) shows an increase in the area coated with rGO due to the higher number of CV cycles. PPy-rGO coated carbon fibre has a very different surface structure showing microspheroid particles of PPy grown on the rGO surface. This suggests that the polymerisation of pyrrole occurred on the surface of rGO from the π-π interaction and hydrogen bond between rGO and PPy (Pruna et al., 2020).
FIGURE 2. (A) Scanning electron microscopy (SEM) images of: Bare carbon fibre, 15 CV scans rGO coated carbon fibre, 20 CV scans rGO coated carbon fibre, PPy-rGO coated carbon fibre; (B) Energy-dispersive X-ray spectroscopy (EDS) - mapping images of PPy‒rGO coated carbon fibre (made using 5 CV scans and 0.2 M pyrrole and SDS solution electropolymerised on 20 CV scans rGO coated carbon fibre) consisting of Carbon, Oxygen, Nitrogen, Sulfur, Sodium, Chlorine and Phosphorous.
Supplementary Figure S4 gives EDS elemental mapping of an SEM image of rGO coated onto carbon fibre from 20 CV scans; composed of C, O, Na, Cl, P and K elements. The C and O mapping suggest the successful formation of rGO onto the carbon fibre. The EDS results presented in Supplementary Table S1 allow comparison of deposition methods and show that carbon and oxygen are the major elements on the rGO electrode surfaces with sodium, chlorine, phosphorous and potassium present due to the PBS solution used. The existence of oxygen indicates the electrodeposition of rGO on the surface of the carbon fibre yarn. EDS results for PPy‒rGO coated on the carbon fibre electrode, show carbon, oxygen, nitrogen, sulphur, sodium and chlorine. An increase in N atomic composition was observed after PPy electropolymerisation with the presence of sulfur and increased O due to the sulfate counterion of PPy.SDS on the surface of the rGO fibre electrode. The EDS results confirm that the surface of the carbon fibre was modified with PPy and rGO.
Figure 2B shows EDS elemental mapping of an SEM image of PPy‒rGO-carbon fibre, composing of C, O, N, S, Na, Cl and P. The C and N mapping suggest the successful formation of PPy onto rGO coated carbon fibre after PPy electropolymerisation. The presence of sulfur shows the incorporation of the sulfate anion dopant in PPy.SDS on the surface of the fibre electrode. EDS elemental mapping results confirm the presence of carbon and oxygen groups of rGO and carbon, nitrogen and sulfur groups of PPy‒rGO which comprise the surface of the fibre electrode.
XPS spectra of rGO coated on carbon fibre (using 20 CV scans; Figure 3A) exhibit two major peaks in the wide survey scan of C1s at 285 eV and O1s at 533 eV. The characteristic C1s peak corresponds to sp2 carbon‒carbon bonding, while the O1s is the result of the different sp3 carbon‒oxygen bonding (Shin et al., 2014; Aunkor et al., 2016). The Na1s peak at 1,072 eV and Cl2p peak at 200 eV are the components of the electrolyte PBS solution used during rGO coating. High resolution spectra show characteristic C1s, O1s, Na1s and Cl2p core level peaks for rGO coated on carbon fibre (using 20 CV scans). The C1s peak shows an intense peak at 285 eV, corresponding to carbon atoms in different functional group which are proposed as C=C (sp2), C–C or C–H (sp3), C–O (epoxyl group), C–OH (hydroxyl group) and C=O or O–C=O (carboxyl group) (Shin et al., 2014; Kar et al., 2016). Such functionality, including epoxy, hydroxyl and carboxyl groups are present in graphene oxide and partially remain after incomplete reduction of graphene oxide (Hilder et al., 2011; Krishnamoorthy et al., 2013). The O1s peak represents oxygen in the form of C=O, C–O and O–C=O, due to the addition of oxygenated functional groups present in rGO (Aunkor et al., 2016).
FIGURE 3. (A) X-ray photoelectron spectra (XPSpectra) of C, O, Na and Cl in 20 CV scans rGO coated carbon fibre; (B) XPSpectra of C, O, N and S in PPy‒rGO coated carbon fibre (made using 5 CV scans and 0.2 M pyrrole and SDS solution electropolymerised on 20 CV scans rGO coated carbon fibre).
The XPS spectrum of PPy‒rGO coated carbon fibre is shown in Figure 3B and consists of four main peaks: C1s at 285 eV, O1s at 532 eV, N1s at 400 eV and S2p at 169 eV. Carbon and nitrogen elements result from the PPy backbone, whereas the S2p region is due to the SDS dopant anions that are incorporated into PPy during the polymerisation process (Abdul Bashid et al., 2017). The C1s peak from PPy and rGO correspond to C–C and C=C (the chemical reduction of GO to rGO), C–N (the existence of PPy), C–O, C=O, –COO–, C–S (possible bonding structures) (Zheng et al., 2014; Chen et al., 2017). The O1s peak represents oxygen in the form of C–O and COOH (rGO functional groups), C–O–C, C=O and C–OH (the possible occurrence bonding structures) and S–O (sulphur dopant from SDS) (Abdul Bashid et al., 2017). Whereas, the N1s peak is assigned to–NH structures which are from nitrogen in the PPy ring, including –NH+– (the polaron state) and =NH+– (the bipolaron state) (Chen et al., 2017; El Jaouhari et al., 2017). The S2p peak for PPy-rGO is attributed to the S–O–, SO2 and C4H4S species after the PPy polymerisation (Abdul Bashid et al., 2017).
After confirming using cyclic voltammetry, Raman spectroscopy, SEM-EDX and XPS results that the carbon fibre electrodes were functionalised with rGO and rGO-PPy, experiments to characterise the electrochemical performance of these fibre electrodes were carried out. Figure 4A shows cyclic voltammograms of rGO-coated fibre electrodes in aqueous solutions containing potassium ferricyanide (10 mM) and KCl (0.1 M) at a scan rate of 100 mV s−1. The rGO fibre electrodes (20 CV scans) give larger oxidation peak currents compared to bare carbon fibre electrodes that are shifted to a lower peak potential which indicate faster electron transfer kinetics and improved electrochemical properties (Kumar et al., 2019). Electrochemical surface area (ECSA) measurements were performed the on PPy-rGO coated carbon fibre and Figure 4B shows cyclic voltammograms of PPy-rGO fibre electrodes in aqueous solutions containing potassium ferricyanide. The ECSA of the fibre electrodes was calculated using the Randles - Sevcik equation. Figure 4C shows how the ECSA of the bare carbon fibre increased with rGO coating, likely due to the nanostructure imparted by the reduction of rGO, while retaining the original carbon fibril surface as shown in SEM results. The potentiostatic deposition rGO on carbon fibres were found to have the lowest ECSA value compared to rGO deposition on carbon fibres using CV. The ECSA value increased with increasing numbers of CV scans during rGO deposition. rGO deposited using 10, 15 and 20 scans gave a 2-fold, 3-fold and 3.5-fold increase in ECSA value compared to the bare carbon fibre, respectively. The PPy-rGO coating gave a 7-fold increase in ECSA value compared to the bare carbon fibre. The polymerisation of pyrrole occurred on the rGO surface owing to the π-π interaction and hydrogen bond between rGO and pyrrole. These interactions enhance the ECSA of the fibre electrode which gave the PPy-rGO fibre electrode a 2-fold increase in ECSA value compared to the 20 CV scans rGO carbon fibre electrode (Atikah Md Jani et al., 2017).
FIGURE 4. (A) Cyclic voltammograms obtained for the rGO caoted carbon fibre electrodes in 10 mM K3[Fe(CN)6] and 0.1 M KCl solution at a scan rate of 100 mV s−1; (B) Cyclic voltammograms obtained for the PPy-rGO coated carbon fibre electrodes in 10 mM K3[Fe(CN)6] and 0.1 M KCl solution at various scan rate (5–100 mV s−1); and (C) Surface area (cm2) of fibre electrodes as calculated by the Randles - Sevcik equation.
The electrochemical response of the rGO fibre electrode in the presence of paracetamol (as model drug) was investigated initially by cyclic voltammetry. The number of cycles for deposition by CV was varied from 3 to 20 to determine the effect this had on the ability of rGO fibre electrodes to sense paracetamol and interferents. As expected, increasing the number of cycles during deposition resulted in more rGO being reduced on the fibre surface, increasing the rGO domain on fibre electrodes. Cyclic voltammograms for rGO coated carbon fibres in the presence of 0–500 µM paracetamol in 0.1 M PBS were recorded (Figure 5). At neutral pH, paracetamol is oxidised to N-acetyl-p-quinoneimine (Özcan and Şahin, 2007). Increasing oxidation currents of paracetamol and a cathodic shift in oxidation peak potential were observed with increasing cycles for rGO deposition (3–20 deposition scans). CVs of rGO carbon fibre (15 cycles) give an oxidation peak of paracetamol at 0.54 V (vs Ag/AgCl), and the reduction peak was found at 0.03 V (vs Ag/AgCl) as shown in Supplementary Figure S5A. The oxidation peak potential of paracetamol is shifted compared to that previously reported using rGO on a glassy carbon electrode at 0.63 V (vs Ag/AgCl) (Ghanbari and Bonyadi, 2018). The oxidation peak current measured at the rGO-carbon fibre electrode (15 cycles) versus the concentration of paracetamol is presented in Supplementary Figure S5B, with a linear range between 50–500 µM (R2 = 0.9901) and a sensitivity of 0.32 μA cm−2 μmol−1 L.
FIGURE 5. (A) Cyclic voltammograms (50 mVs−1) of a rGO-coated carbon fibre (made using 20 CV scans) with various concentrations of paracetamol in aqueous 0.1 M PBS solution; (B) The resulting oxidation peak currents vs. paracetamol concentration (n = 3); (C) Differential pulse voltammograms of rGO-coated carbon fibres (made using 20 CV scans) with various concentrations of paracetamol in aqueous 0.1 M PBS solution; (D) The resulting oxidation peak currents vs. paracetamol concentration (n = 3); (E) Cyclic voltammograms (50 mVs−1) of uncoated carbon fibre and rGO-coated carbon fibres (made using potentiostatic and CV techniques) with the 500 µM paracetamol in aqueous 0.1 M PBS solution; (F) Differential pulse voltammograms of rGO-coated carbon fibres (made using potentiostatic and CV techniques) with the 500 µM paracetamol in aqueous 0.1 M PBS solution.
Well-defined redox peaks of paracetamol with increased peak currents were observed at rGO on carbon fibre deposited after 20 cycles as displayed in Figure 5A, suggesting a better electron transfer rate at the electrode surface compared to the 15 cycle equivalent (Palakollu et al., 2020). The oxidation and reduction peaks of paracetamol were observed at 0.49 and 0.11 V (vs Ag/AgCl), respectively. Figure 5B shows a calibration curve of the oxidation peak current measured at the rGO-carbon fibre electrode (20 cycles) versus the concentration of paracetamol with a linear region in the concentration range of 50–500 µM (R2 = 0.9961) and an improved sensitivity of 0.44 μA cm−2 μmol−1 L. The limits of detection for paracetamol of 15, and 20 CV scans of rGO-coated carbon fibre were 19.0 µM, and 17.5 µM (S/N = 3), respectively.
Differential pulse voltammetry (DPV) was examined in an effort to increase the fibre electrode sensitivity by reducing background capacitance observed during CV. Again an increase in peak current and a negative shift of the peak potential was found with increasing rGO deposition (Figure 5F). Figure S5c shows DPV detection of 10–500 µM paracetamol concentrations at the rGO-coated carbon fibre (15 cycles). Anodic current peaks were centred at 0.5 V (vs Ag/AgCl) and a linear correlation to paracetamol concentration was observed between 50–500 µM (R2 = 0.9946), as shown in Supplementary Figure S5D. In comparison, the 20-cycle rGO-coated carbon fibre (Figure 5C), shows a negative shift in the anodic peak of paracetamol at 0.45 V (vs Ag/AgCl). The corresponding plot of peak current vs paracetamol concentration shows two linear ranges between 20–100 µM (R2 = 0.9965) and 100–500 µM (R2 = 0.9933) as shown in Figure 5D. The limits of detection for paracetamol of 15 and 20 CV scans of rGO-coated carbon fibre were 38.6 and 21.1 µM (S/N = 3), respectively. The sensitivity of the calibration (20 cycles) is 0.3 μA cm−2 μmol−1 L, which is a slight improvement over the 15-cycle equivalent at 0.2 μA cm−2 μmol−1 L. These results demonstrate that the rGO coating on a carbon yarn electrode, after 20 deposition cycles, enhances the electro-oxidation of paracetamol.
Dopamine is a common interferent in biological fluids when measuring paracetamol (Farajikhah et al., 2019). As shown in Figure 6A, cyclic voltammograms of rGO carbon fibre (20 cycles) show an oxidation peak for dopamine at 0.25 V (vs Ag/AgCl), with two reduction peaks observed at −0.25 and 0.10 V (vs Ag/AgCl), respectively. The oxidised o-dopaminoquinone undergoes intramolecular cyclisation to form leucodopaminochrome, which exhibits two cathodic peaks related to the reduction of o-dopaminoquinone to dopamine and leucodopaminochrome to dopaminochrome (Daniel Arulraj et al., 2016). The oxidation peak current increased linearly with dopamine concentration over the concentration ranges of 0–50 µM with a correlation coefficient of 0.9927 and 50–500 µM with a correlation coefficient of 0.9955 (Figure 6B) and the detection limit (3σ) is 5.1 µM (S/N = 3) which is lower than that of paracetamol sensing. DPV responses of dopamine concentrations from 0–500 μM at the rGO carbon fibre (20 cycles) are displayed in Supplementary Figure S6A. Anodic peaks were centred at 0.2 V (vs. Ag/AgCl) and the peak current increased with increasing dopamine concentration. The corresponding calibration plot between the oxidation peak current and concentration of dopamine again shows two linear correlations with dopamine concentration observed between 0–50 µM (R2 = 0.9866) and 100–500 µM (R2 = 0.9930), as illustrated in Figure 6C. The detection limit (3σ S/N) of paracetamol with DPV was 9.0 µM, which is higher compared to CV detection. In addition, CV analysis gave a broader linear range for dopamine analysis with more sensitive detection (0.22 μA cm−2 μmol−1 L) compared to the detection using DPV (0.09 μA cm−2 μmol−1 L).
FIGURE 6. (A) Cyclic voltammograms (50 mVs−1) of a rGO-coated carbon fibre (made using 20 CV scans) with various concentrations of dopamine in aqueous 0.1 M PBS solution; (B) The resulting oxidation peak currents vs. dopamine concentration using CV technique (n = 3); (C) The resulting oxidation peak currents of a rGO-coated carbon fibre (made using 20 CV scans) vs. dopamine concentration using DPV technique (n = 3); (D) DPV curves recorded with 100 μM paracetamol (red), 100 μM paracetamol with the presence of 100 μM dopamine (blue), and 100 μM paracetamol with the presence of 100 μM dopamine and 100 μM ascorbic acid (yellow).
Generally, dopamine and ascorbic acid coexist as electro-active interferents in the electrochemical detection of paracetamol. Interestingly, when the rGO carbon fibre electrodes were studied in a solution containing both dopamine and ascorbic acid, only a response to dopamine was observed (results not shown). Good selectivity of paracetamol over interferents is demonstrated using DPV in Figure 6D, where simultaneous detection of 100 µM paracetamol in the presence of 100 µM dopamine (blue line) is shown. Two clearly distinguished anodic peaks are observed, with the oxidation peak of paracetamol at 0.49 V (vs. Ag/AgCl) separated from the oxidation peak of dopamine at 0.25 V (vs. Ag/AgCl), while ascorbic acid is not detected (yellow line). The sensitivity for paracetamol (calculated using DPV response) is higher at 0.3 μA cm−2 μmol−1 L compared to 0.09 μA cm−2 μmol−1 L for dopamine. These results indicate that the simultaneous determination of paracetamol and dopamine is possible at the rGO-coated carbon fibre electrode.
Polypyrrole has been shown to enhance the performance of carbon yarn electrodes towards sensing of paracetamol. PPy‒rGO coated carbon yarns were developed by electropolymerisation of PPy onto rGO-coated carbon fibre to further enhance the electrochemical surface area and therefore the performance of the fibre electrode. CV analysis using a PPy-rGO carbon fibre electrode (PPy deposited using 5 CV cycles from a 0.2 M pyrrole and 0.2 M dopant solution onto 20-scan-rGO fibre), gave an oxidation peak for paracetamol at 0.55 V vs. Ag/AgCl with the reduction peak obscured due to the background capacitance of PPy (Figure 7A). The oxidation peak was at a similar potential to that previously observed with PPy coated onto reduced graphene oxide/glassy carbon electrodes (0.57 V vs. Ag/AgCl) (Ghanbari and Bonyadi, 2018). A calibration curve of the oxidation peak current versus paracetamol concentration (0–500 µM) gives two linear regions in the range of 10–50 µM with a correlation coefficient of 0.9999 and 50–500 µM with a correlation coefficient of 0.9924 (Figure 7B). The detection limit (3σ S/N) is 9.1 µM. Figure 7C shows DPV detection of paracetamol at the PPy-rGO fibre electrode and the anodic peak current appeared at 0.45 V vs. Ag/AgCl. Two different linear correlations with paracetamol concentration were observed: the first between 0–50 µM (R2 = 0.9960) and the second between 100–500 µM (R2 = 0.9862) as shown in Figure 7D. Using DPV and the PPy-coated rGO carbon yarns, the detection limit of paracetamol sensing was notably improved (3σ S/N) to 3.7 µM. Table 1 lists a series of published electrochemical sensors based on rGO and PPy for the detection of various important drugs and compares them to the performance of the PPy-coated rGO carbon yarn reported here. Electrode material, synthesis approach, analyte, determination technique and LOD are given as well as the reference where the information is available.
FIGURE 7. (A) Cyclic voltammograms (50 mVs−1) of a PPy-rGO coated carbon fibre with various concentrations of paracetamol in aqueous 0.1 M PBS solution; (B) The resulting oxidation peak currents vs. paracetamol concentration using CV technique (n = 3); (C) Differential pulse voltammograms of a PPy-rGO coated carbon fibre with various concentrations of paracetamol in aqueous 0.1 M PBS solution; (D) The resulting oxidation peak currents vs. paracetamol concentration using DPV technique (n = 3).
TABLE 1. Comparison of the electrochemical sensors based on rGO and PPy electrodes for the determination of drugs.
Figure 8A shows cyclic voltammetric responses of dopamine (0–500 µM) at the PPy-rGO carbon fibre electrode, the oxidation peak of dopamine was found at 0.2 V (vs Ag/AgCl). The oxidation peak current increased linearly with dopamine concentration over the concentration ranges of 10–50 µM with a correlation coefficient of 0.9891 and 100–500 µM with a correlation coefficient of 0.9915 (Figure 8B). The detection limit is 6.7 µM (S/N = 3) which was lower than that of paracetamol sensing. DPV analysis of dopamine was also carried out at the PPy-rGO coated carbon fibre electrode (results not shown). The corresponding calibration plot between the oxidation peak current and concentration of dopamine shows two linear correlations with dopamine concentration observed between 2.5–50 µM (R2 = 0.9901) and 100–500 µM (R2 = 0.9974), as illustrated in Figure 8C. The detection limit (3σ S/N) of dopamine was 6.0 µM which was similar to the detection limit for dopamine sensing using CV. Table 2 shows a comparison of the limit of detection of various electrodes described in this study obtained using CV, DPV and amperometry for paracetamol and dopamine sensing.
FIGURE 8. (A) Cyclic voltammograms (50 mVs−1) of a PPy-rGO coated carbon fibre with various concentrations of dopamine in aqueous 0.1 M PBS solution; (B) The resulting oxidation peak currents vs. dopamine concentration using CV technique; (C) The resulting oxidation peak currents of a PPy-rGO coated carbon fibre vs. dopamine concentration using DPV technique; (D) DPV curves recorded with 100 μM paracetamol (red), 100 μM paracetamol with the presence of 100 μM dopamine (blue), and 100 μM paracetamol with the presence of 100 μM dopamine and 100 μM ascorbic acid (yellow).
Figure 8D shows good selectivity of paracetamol over dopamine and ascorbic acid using DPV, where two clearly distinguished oxidation peaks are observed during simultaneous detection of paracetamol (100 µM) and dopamine (100 μM; blue line). In addition, two anodic peaks were observed towards paracetamol (100 µM) and dopamine (100 µM) in the presence of ascorbic acid (100 μM; yellow line). These results indicate that the proposed PPy-rGO coated carbon fibre electrode enabled the simultaneous determination of paracetamol and dopamine.
The amperometric response of the rGO carbon yarn (20 cycles) to paracetamol (0–500 μM) was investigated at an applied potential of 0.6 V (vs. Ag/AgCl), as depicted in Figure 9A. The amperometric current shows a step increase after the addition of increasing concentrations of paracetamol. A linear relationship between the oxidation current and paracetamol concentration is obtained for concentrations ranging from 50 to 500 μM of paracetamol with a detection limit (3σ S/N) of 63.0 µM (Figure 9B). The PPy‒rGO coated carbon yarn shows a step increase with successive addition of 0–500 μM paracetamol (Figure 9C). From the calibration curve shown in Figure 9D, there is a linear region over a concentration range of 35–500 μM (R2 = 0.9914). The detection limit for the PPy‒rGO coated carbon fibre electrode (3σ S/N) is 48.0 μM.
FIGURE 9. (A) Amperometric response of a 20 CV scans carbon fibre in aqueous 0.1 M PBS solutions containing paracetamol (0 ˗ 500 μM) at an applied potential of 0.6 V (vs. Ag/AgCl); (B) Calibration plot, anodic peak current vs. concentration of paracetamol; (C) Amperometric response of a PPy‒rGO coated carbon fibre (made using 5 CV scans and 0.2 M pyrrole and SDS solution electropolymerised on 20 CV scans rGO coated carbon fibre) in aqueous 0.1 M PBS solutions containing paracetamol (0 ˗ 500 μM) at an applied potential of 0.6 V (vs. Ag/AgCl); (D) Calibration plot, anodic peak current vs. concentration of paracetamol.
A modified carbon fibre yarn electrode was developed for the determination of paracetamol and dopamine. The rGO-coated carbon fibre electrode was constructed using electrodeposition and CV with the fibre made from 20 CV cycles yielding an optimised electrode to detect paracetamol and dopamine. Subsequent electropolymerisation of PPy onto the rGO fibre electrode increased the electrochemical surface area on the fibre electrode. Using differential pulse voltammetry, the PPy-rGO carbon fibre electrode responded with a detection limit (3σ S/N) of 3.7 and 6.0 µM for paracetamol and dopamine, respectively. This flexible fibre-based sensor represents greatly enhanced electrochemical responses for paracetamol and dopamine during simultaneous detection without the overlapping peak potentials.
The original contributions presented in the study are included in the article/Supplementary Material, further inquiries can be directed to the corresponding author.
SS and CC carried out the rGO flexible electrode studies, participated in the sequence alignment and drafted the manuscript. JV participated in the sequence alignment and drafted the manuscript. SZ, GW and JC participated in rGO synthesis. All authours approved the final manuscript.
The authors declare that the research was conducted in the absence of any commercial or financial relationships that could be construed as a potential conflict of interest.
All claims expressed in this article are solely those of the authors and do not necessarily represent those of their affiliated organizations, or those of the publisher, the editors and the reviewers. Any product that may be evaluated in this article, or claim that may be made by its manufacturer, is not guaranteed or endorsed by the publisher.
The authors thank the Royal Thai Government Scholarship for providing funding for this work as well as EPSRC strategic equipment grant, EP/M022749/1.
The Supplementary Material for this article can be found online at: https://www.frontiersin.org/articles/10.3389/fsens.2021.719161/full#supplementary-material
Abdul Bashid, H. A., Lim, H. N., Kamaruzaman, S., Abdul Rashid, S., Yunus, R., Huang, N. M., et al. (2017). Electrodeposition of Polypyrrole and Reduced Graphene Oxide onto Carbon Bundle Fibre as Electrode for Supercapacitor. Nanoscale Res. Lett. 12 (1). doi:10.1186/s11671-017-2010-3
Adhikari, B.-R., Govindhan, M., and Chen, A. (2015). Carbon Nanomaterials Based Electrochemical Sensors/biosensors for the Sensitive Detection of Pharmaceutical and Biological Compounds. Sensors 15 (9), 22490–22508. doi:10.3390/s150922490
Adhikari, B.-R., Govindhan, M., and Chen, A. (2015). Sensitive Detection of Acetaminophen with Graphene-Based Electrochemical Sensor. Electrochim. Acta 162, 198–204. doi:10.1016/j.electacta.2014.10.028
Almeida, D. A. L., Couto, A. B., and Ferreira, N. G. (2019). Flexible Polyaniline/reduced Graphene Oxide/carbon Fiber Composites Applied as Electrodes for Supercapacitors. J. Alloys Compd. 788, 453–460. doi:10.1016/J.JALLCOM.2019.02.194
Antony, R. P., Preethi, L. K., Gupta, B., Mathews, T., Dash, S., and Tyagi, A. K. (2015). Efficient Electrocatalytic Performance of Thermally Exfoliated Reduced Graphene Oxide-Pt Hybrid. Mater. Res. Bull. 70, 60–67. doi:10.1016/j.materresbull.2015.04.015
Ateh, D. D., Navsaria, H. A., and Vadgama, P. (2006). Polypyrrole-based Conducting Polymers and Interactions with Biological Tissues. J. R. Soc. Interf. 3 (11), 741–752. doi:10.1098/rsif.2006.0141
Atikah Md Jani, N., Aidil Ibrahim, M., Ishak Tunku Kudin, T., Malik Marwan Ali, A., Osman, H., and Hasdinor Hassan, O. (2017). Morphological and Electrochemical Properties of Hybridized PPy/rGO Composites. Mater. Today Proc. 4 (4), 5138–5145. doi:10.1016/j.matpr.2017.05.019
Atta, N. F., Galal, A., Ahmed, Y. M., and El-Ads, E. H. (2019). Design Strategy and Preparation of a Conductive Layered Electrochemical Sensor for Simultaneous Determination of Ascorbic Acid, Dobutamine, Acetaminophen and Amlodipine. Sensors Actuators B: Chem. 297, 126648. doi:10.1016/j.snb.2019.126648
Aunkor, M. T. H., Mahbubul, I. M., Saidur, R., and Metselaar, H. S. C. (2016). The green Reduction of Graphene Oxide. RSC Adv. 6 (33), 27807–27828. doi:10.1039/c6ra03189g
Babaei, A., and Taheri, A. R. (2013). Nafion/Ni(OH)2 Nanoparticles-Carbon Nanotube Composite Modified Glassy Carbon Electrode as a Sensor for Simultaneous Determination of Dopamine and Serotonin in the Presence of Ascorbic Acid. Sensors Actuators B: Chem. 176, 543–551. doi:10.1016/J.SNB.2012.09.021
Bandodkar, A. J., and Wang, J. (2014). Non-invasive Wearable Electrochemical Sensors: A Review. Trends Biotechnol. 32 (7), 363–371. doi:10.1016/J.TIBTECH.2014.04.005
Chen, L., Tang, Y., Wang, K., Liu, C., and Luo, S. (2011). Direct Electrodeposition of Reduced Graphene Oxide on Glassy Carbon Electrode and its Electrochemical Application. Electrochem. Commun. 13 (2), 133–137. doi:10.1016/j.elecom.2010.11.033
Chen, J., Wang, Y., Cao, J., Liu, Y., Zhou, Y., Ouyang, J.-H., et al. (2017). Facile Co-electrodeposition Method for High-Performance Supercapacitor Based on Reduced Graphene Oxide/Polypyrrole Composite Film. ACS Appl. Mater. Inter. 9 (23), 19831–19842. doi:10.1021/acsami.7b03786
Chen, X., Li, D., Ma, W., Yang, T., Zhang, Y., and Zhang, D. (2019). Preparation of a Glassy Carbon Electrode Modified with Reduced Graphene Oxide and Overoxidized Electropolymerized Polypyrrole, and its Application to the Determination of Dopamine in the Presence of Ascorbic Acid and Uric Acid. Microchim Acta 186 (7), 407. doi:10.1007/s00604-019-3518-2
Daniel Arulraj, A., Arunkumar, A., Vijayan, M., Balaji Viswanath, K., and Vasantha, V. S. (2016). A Simple Route to Develop Highly Porous Nano Polypyrrole/Reduced Graphene Oxide Composite Film for Selective Determination of Dopamine. Electrochim. Acta 206, 77–85. doi:10.1016/j.electacta.2016.04.134
Demirkan, B., Bozkurt, S., Cellat, K., Arıkan, K., Yılmaz, M., Şavk, A., et al. (2020). Palladium Supported on Polypyrrole/reduced Graphene Oxide Nanoparticles for Simultaneous Biosensing Application of Ascorbic Acid, Dopamine, and Uric Acid. Sci. Rep. 10 (1), 1–10. doi:10.1038/s41598-020-59935-y
Dhanush, S., Sreejesh, M., Bindu, K., Chowdhury, P., and Nagaraja, H. S. (2018). Synthesis and Electrochemical Properties of Silver Dendrites and Silver dendrites/rGO Composite for Applications in Paracetamol Sensing. Mater. Res. Bull. 100, 295–301. doi:10.1016/j.materresbull.2017.12.044
El Jaouhari, A., El Asbahani, A., Bouabdallaoui, M., Aouzal, Z., Filotás, D., Bazzaoui, E. A., et al. (2017). Corrosion Resistance and Antibacterial Activity of Electrosynthesized Polypyrrole. Synth. Met. 226, 15–24. doi:10.1016/j.synthmet.2017.01.008
Farajikhah, S., Innis, P. C., Paull, B., Wallace, G. G., and Harris, A. R. (2019). Facile Development of a Fiber-Based Electrode for Highly Selective and Sensitive Detection of Dopamine. ACS Sens. 4 (10), 2599–2604. doi:10.1021/acssensors.9b01583
Gandara, M., and Gonçalves, E. S. (2020). Electroactive Composites: PANI Electrochemical Synthesis with GO and rGO for Structural Carbon Fiber Coating. Prog. Org. Coat. 138, 105399. doi:10.1016/j.porgcoat.2019.105399
Garcia-Torres, J., and Crean, C. (2018). Multilayered Flexible Fibers with High Performance for Wearable Supercapacitor Applications. Adv. Sustainable Syst. 2 (2), 1700143. doi:10.1002/adsu.201700143
Ghanbari, K., and Bonyadi, S. (2018). An Electrochemical Sensor Based on Reduced Graphene Oxide Decorated with Polypyrrole Nanofibers and Zinc Oxide-Copper Oxide P-N junction Heterostructures for the Simultaneous Voltammetric Determination of Ascorbic Acid, Dopamine, Paracetamol, and Tryptophan. New J. Chem. 42 (11), 8512–8523. doi:10.1039/c8nj00857d
Han, G. C., and Kumar, S. (2008). Materials Science: Making strong Fibers. Science 319 (5865), 908–909. doi:10.1126/science.1153911
Hao, W., Zhang, Y., Fan, J., Liu, H., Shi, Q., Liu, W., et al. (2019). Copper Nanowires Modified with Graphene Oxide Nanosheets for Simultaneous Voltammetric Determination of Ascorbic Acid, Dopamine and Acetaminophen. Molecules 24 (12), 2320–2413. doi:10.3390/molecules24122320
Hasanzadeh, M., Shadjou, N., and Omidinia, E. (2013). A Novel Electroanalytical Method for Simultaneous Detection of Two Neurotransmitter Dopamine and Serotonin in Human Serum. J. Neurosci. Methods 219 (1), 52–60. doi:10.1016/J.JNEUMETH.2013.07.007
Hilder, M., Winther-Jensen, B., Li, D., Forsyth, M., and MacFarlane, D. R. (2011). Direct Electro-Deposition of Graphene from Aqueous Suspensions. Phys. Chem. Chem. Phys. 13 (20), 9187–9193. doi:10.1039/c1cp20173e
Huang, Y., Li, H., Wang, Z., Zhu, M., Pei, Z., Xue, Q., et al. (2016). Nanostructured Polypyrrole as a Flexible Electrode Material of Supercapacitor. Nano Energy 22, 422–438. doi:10.1016/j.nanoen.2016.02.047
Huang, Y., Shen, C., Tang, Z., Shi, T., Zheng, S., and Lin, L. (2019). Mass Loading‐Independent Energy Storage with Reduced Graphene Oxide and Carbon Fiber. ChemElectroChem 6 (24), 6009–6015. doi:10.1002/celc.201901617
Johra, F. T., Lee, J.-W., and Jung, W.-G. (2014). Facile and Safe Graphene Preparation on Solution Based Platform. J. Ind. Eng. Chem. 20 (5), 2883–2887. doi:10.1016/j.jiec.2013.11.022
Jost, K., Stenger, D., Perez, C. R., McDonough, J. K., Lian, K., Gogotsi, Y., et al. (2013). Knitted and Screen Printed Carbon-Fiber Supercapacitors for Applications in Wearable Electronics. Energy Environ. Sci. 6 (9), 2698–2705. doi:10.1039/c3ee40515j
Kang, X., Wang, J., Wu, H., Liu, J., Aksay, I. A., and Lin, Y. (2010). A Graphene-Based Electrochemical Sensor for Sensitive Detection of Paracetamol. Talanta 81 (3), 754–759. doi:10.1016/j.talanta.2010.01.009
Kar, P., Sardar, S., Liu, B., Sreemany, M., Lemmens, P., Ghosh, S., et al. (2016). Facile Synthesis of Reduced Graphene Oxide-Gold Nanohybrid for Potential Use in Industrial Waste-Water Treatment. Sci. Technol. Adv. Mater. 17 (1), 375–386. doi:10.1080/14686996.2016.1201413
Kaur, G., Adhikari, R., Cass, P., Bown, M., and Gunatillake, P. (2015). Electrically Conductive Polymers and Composites for Biomedical Applications. RSC Adv. 5 (47), 37553–37567. doi:10.1039/c5ra01851j
Khan, U., Young, K., O'Neill, A., and Coleman, J. N. (2012). High Strength Composite Fibres from Polyester Filled with Nanotubes and Graphene. J. Mater. Chem. 22 (25), 12907–12914. doi:10.1039/c2jm31946b
Kim, S., Jang, L. K., Park, H. S., and Lee, J. Y. (2016). Electrochemical Deposition of Conductive and Adhesive Polypyrrole-Dopamine Films. Sci. Rep. 6, 1–8. doi:10.1038/srep30475
Krishnamoorthy, K., Veerapandian, M., Yun, K., and Kim, S.-J. (2013). The Chemical and Structural Analysis of Graphene Oxide with Different Degrees of Oxidation. Carbon 53, 38–49. doi:10.1016/j.carbon.2012.10.013
Kumar, Y., Pramanik, P., and Das, D. K. (2019). Electrochemical Detection of Paracetamol and Dopamine Molecules Using Nano-Particles of Cobalt Ferrite and Manganese Ferrite Modified with Graphite. Heliyon 5 (7), e02031. doi:10.1016/j.heliyon.2019.e02031
Le, V. T., Kim, H., Ghosh, A., Kim, J., Chang, J., Vu, Q. A., et al. (2013). Coaxial Fiber Supercapacitor Using All-Carbon Material Electrodes. ACS Nano 7 (7), 5940–5947. doi:10.1021/nn4016345
Li, C. M., Sun, C. Q., Chen, W., and Pan, L. (2005). Electrochemical Thin Film Deposition of Polypyrrole on Different Substrates. Surf. Coat. Technol. 198 (1-3), 474–477. doi:10.1016/J.SURFCOAT.2004.10.065
Li, J., Liu, J., Tan, G., Jiang, J., Peng, S., Deng, M., et al. (2014). High-sensitivity Paracetamol Sensor Based on Pd/graphene Oxide Nanocomposite as an Enhanced Electrochemical Sensing Platform. Biosens. Bioelectron. 54, 468–475. doi:10.1016/j.bios.2013.11.001
Liang, B., Qin, Z., Li, T., Dou, Z., Zeng, F., Cai, Y., et al. (2015). Poly(aniline-co-pyrrole) on the Surface of Reduced Graphene Oxide as High-Performance Electrode Materials for Supercapacitors. Electrochim. Acta 177, 335–342. doi:10.1016/j.electacta.2015.01.135
Lin, H.-Y., Chen, W.-H., and Huang, C.-H. (2019). Graphene in Electrochemical Biosensors. Amsterdam: Elsevier, 321–336. doi:10.1016/b978-0-12-815889-0.00015-5
Liu, Y., Weng, B., Razal, J. M., Xu, Q., Zhao, C., Hou, Y., et al. (2015). High-Performance Flexible All-Solid-State Supercapacitor from Large Free-Standing Graphene-PEDOT/PSS Films. Sci. Rep. 5, 17045. doi:10.1038/srep17045
Liu, Y., Zhang, B., Xu, Q., Hou, Y., Seyedin, S., Qin, S., et al. (2018). Development of Graphene Oxide/Polyaniline Inks for High Performance Flexible Microsupercapacitors via Extrusion Printing. Adv. Funct. Mater. 28 (21), 1706592. doi:10.1002/adfm.201706592
Lynam, C., Wallace, G. G., and Officer, D. L. (2007). Electrodeposition and Characterisation of Polypyrroles Containing Sulfonated Carbon Nanotubes. J. Nanosci. Nanotech. 7 (10), 3487–3494. doi:10.1166/jnn.2007.825
Marcano, D. C., Kosynkin, D. V., Berlin, J. M., Sinitskii, A., Sun, Z., Slesarev, A., et al. (2010). Improved Synthesis of Graphene Oxide. ACS Nano 4 (8), 4806–4814. doi:10.1021/nn1006368
Nayak, D. S., and Shetti, N. P. (2016). Electrochemical Oxidation of Provitamin B5, D-Panthenol and its Analysis in Spiked Human Urine. J. Anal. Sci. Technol. 7 (1), 1–8. doi:10.1186/s40543-016-0092-7
Nikoofard, H., Kalantar, Z., and Omidian, M. (2014). Electrochemical Preparation and Characterization of Polypyrrole Films in an Aqueous Solution Containing a Biocompatible Surfactant. Res. Rev. Electrochem. 5 (4), 101–108. https://www.tsijournals.com/articles/electrochemical-preparation-and-characterization-of-polypyrrole-films-in-an-aqueous-solution-containing-a-biocompatible-.pdf.
Olejnik, P., Świetlikowska, A., Gniadek, M., and Pałys, B. (2014). Electrochemically Reduced Graphene Oxide on Electrochemically Roughened Gold as a Support for Horseradish Peroxidase. J. Phys. Chem. C 118 (51), 29731–29738. doi:10.1021/jp507227z
Özcan, L., and Şahin, Y. (2007). Determination of Paracetamol Based on Electropolymerized-Molecularly Imprinted Polypyrrole Modified Pencil Graphite Electrode. Sens. Actuators B: Chem. 127 (2), 362–369. doi:10.1016/j.snb.2007.04.034
Palakollu, V. N., Chiwunze, T. E., Liu, C., and Karpoormath, R. (2020). Electrochemical Sensitive Determination of Acetaminophen in Pharmaceutical Formulations at Iron Oxide/graphene Composite Modified Electrode. Arabian J. Chem. 13 (2), 4350–4357. doi:10.1016/j.arabjc.2019.08.001
Ponnaiah, S. K., Prakash, P., and Vellaichamy, B. (2018). A New Analytical Device Incorporating a Nitrogen Doped Lanthanum Metal Oxide with Reduced Graphene Oxide Sheets for Paracetamol Sensing. Ultrason. Sonochem. 44, 196–203. doi:10.1016/j.ultsonch.2018.02.016
Pruna, A. I., Rosas-Laverde, N. M., and Busquets Mataix, D. (2020). Effect of Deposition Parameters on Electrochemical Properties of Polypyrrole-Graphene Oxide Films. Materials 13 (3), 624. doi:10.3390/ma13030624
Puthongkham, P., Yang, C., and Venton, B. J. (2018). Carbon Nanohorn-Modified Carbon Fiber Microelectrodes for Dopamine Detection. Electroanalysis 30 (6), 1073–1081. doi:10.1002/elan.201700667
Ramesh, A., Jeyavelan, M., and Leo Hudson, M. S. (2018). Electrochemical Properties of Reduced Graphene Oxide Derived through Camphor Assisted Combustion of Graphite Oxide. Dalton Trans. 47 (15), 5406–5414. doi:10.1039/c8dt00626a
Ramezani, M., Alibolandi, M., Nejabat, M., Charbgoo, F., Taghdisi, S. M., and Abnous, K. (2019). Graphene-Based Hybrid Nanomaterials for Biomedical Applications. Amsterdam: Elsevier, 119–141. doi:10.1016/b978-0-12-815889-0.00006-4
Rasouli, H., Naji, L., and Hosseini, M. G. (2018). 3D Structured Polypyrrole/reduced Graphene Oxide (PPy/rGO)-Based Electrode Ionic Soft Actuators with Improved Actuation Performance. New J. Chem. 42 (14), 12104–12118. doi:10.1039/c8nj00936h
Selvam, S., Balamuralitharan, B., Karthick, S. N., Hemalatha, K. V., Prabakar, K., and Kim, H.-J. (2016). Simultaneous Electrochemical Deposition of an E-rGO/β-CD/MnO2 Ternary Composite for a Self-Powered Supercapacitor Based Caffeine Sensor. Anal. Methods 8 (44), 7937–7943. doi:10.1039/c6ay02804g
Selvaraju, T., and Ramaraj, R. (2003). Simultaneous Determination of Ascorbic Acid, Dopamine and Serotonin at Poly(phenosafranine) Modified Electrode. Electrochem. Commun. 5 (8), 667–672. doi:10.1016/S1388-2481(03)00151-6
Shetti, N. P., Nayak, D. S., Malode, S. J., and Kulkarni, R. M. (2017). Electrochemical Sensor Based upon Ruthenium Doped TiO2Nanoparticles for the Determination of Flufenamic Acid. J. Electrochem. Soc. 164 (5), B3036–B3042. doi:10.1149/2.0031705jes
Shin, Y.-E., Sa, Y. J., Park, S., Lee, J., Shin, K.-H., Joo, S. H., et al. (2014). An Ice-Templated, pH-Tunable Self-Assembly Route to Hierarchically Porous Graphene Nanoscroll Networks. Nanoscale 6 (16), 9734–9741. doi:10.1039/c4nr01988a
Shukla, R. P., Cazelles, R., Kelly, D. L., and Ben-Yoav, H. (2020). A Reduced-Graphene Oxide-Modified Microelectrode for a Repeatable Detection of Antipsychotic Clozapine Using Microliters-Volumes of Whole Blood. Talanta 209, 120560. doi:10.1016/j.talanta.2019.120560
Soltani, N., Tavakkoli, N., Ahmadi, N., and Davar, F. (2015). Simultaneous Determination of Acetaminophen, Dopamine and Ascorbic Acid Using a PbS Nanoparticles Schiff Base-Modified Carbon Paste Electrode. Comptes Rendus Chim. 18 (4), 438–448. doi:10.1016/j.crci.2014.07.001
Tang, L., Han, J., Jiang, Z., Chen, S., and Wang, H. (2015). Flexible Conductive Polypyrrole Nanocomposite Membranes Based on Bacterial Cellulose with Amphiphobicity. Carbohydr. Polym. 117, 230–235. doi:10.1016/J.CARBPOL.2014.09.049
Thu, N. T. A., Duc, H. V., Hai Phong, N., Cuong, N. D., Hoan, N. T. V., and Quang Khieu, D. (2018). Electrochemical Determination of Paracetamol Using Fe3O4/Reduced Graphene-Oxide-Based Electrode. J. Nanomater. 2018, 1–15. doi:10.1155/2018/7619419
Twycross, R., Pace, V., Mihalyo, M., and Wilcock, A. (2013). Acetaminophen (Paracetamol). J. Pain Symptom Manage. 46 (5), 747–755. doi:10.1016/j.jpainsymman.2013.08.001
Yang, W., Chen, Y., Wang, J., Peng, T., Xu, J., Yang, B., et al. (2018). Reduced Graphene Oxide/Carbon Nanotube Composites as Electrochemical Energy Storage Electrode Applications. Nanoscale Res. Lett. 13, 181. doi:10.1186/s11671-018-2582-6
Zhang, W., Shen, C., Lu, G., Ni, Y., Lu, C., and Xu, Z. (2018). Synthesis of PPy/RGO-Based Hierarchical Material with Super-paramagnetic Behavior and Understanding its Robust Photo Current Driven by Visible Light. Synth. Met. 241, 17–25. doi:10.1016/j.synthmet.2018.03.018
Zheng, B., Huang, T., Kou, L., Zhao, X., Gopalsamy, K., and Gao, C. (2014). Graphene Fiber-Based Asymmetric Micro-supercapacitors. J. Mater. Chem. A. 2 (25), 9736–9743. doi:10.1039/c4ta01868k
Keywords: reduced grapheme oxide, polypyrrole, fibre electrode, cyclic voltammetry, wearable drug sensing
Citation: Sriprasertsuk S, Zhang S, Wallace G, Chen J, Varcoe JR and Crean C (2021) Reduced Graphene Oxide Carbon Yarn Electrodes for Drug Sensing. Front. Sens. 2:719161. doi: 10.3389/fsens.2021.719161
Received: 08 June 2021; Accepted: 19 July 2021;
Published: 10 August 2021.
Edited by:
Daniel S. Correa, Brazilian Agricultural Research Corporation (EMBRAPA), BrazilReviewed by:
Murilo Facure, Federal University of São Carlos, BrazilCopyright © 2021 Sriprasertsuk, Zhang, Wallace, Chen, Varcoe and Crean. This is an open-access article distributed under the terms of the Creative Commons Attribution License (CC BY). The use, distribution or reproduction in other forums is permitted, provided the original author(s) and the copyright owner(s) are credited and that the original publication in this journal is cited, in accordance with accepted academic practice. No use, distribution or reproduction is permitted which does not comply with these terms.
*Correspondence: Carol Crean, Yy5jcmVhbkBzdXJyZXkuYWMudWs=
Disclaimer: All claims expressed in this article are solely those of the authors and do not necessarily represent those of their affiliated organizations, or those of the publisher, the editors and the reviewers. Any product that may be evaluated in this article or claim that may be made by its manufacturer is not guaranteed or endorsed by the publisher.
Research integrity at Frontiers
Learn more about the work of our research integrity team to safeguard the quality of each article we publish.