- Department of Molecular and Medical Genetics, Oregon Health and Science University, Portland, OR, United States
Ribosome biogenesis is essential for cell growth, proliferation, and animal development. Its deregulation leads to various human disorders such as ribosomopathies and cancer. Thus, tight regulation of ribosome biogenesis is crucial for normal cell homeostasis. Emerging evidence suggests that posttranslational modifications such as ubiquitination and SUMOylation play a crucial role in regulating ribosome biogenesis. Our recent studies reveal that USP36, a nucleolar deubiquitinating enzyme (DUB), acts also as a SUMO ligase to regulate nucleolar protein group SUMOylation, thereby being essential for ribosome biogenesis. Here, we provide an overview of the current understanding of the SUMOylation regulation of ribosome biogenesis and discuss the role of USP36 in nucleolar SUMOylation.
Introduction
Ribosome biogenesis is a complex multi-step cellular process for making the ribosome, including the synthesis of ribosomal RNA (rRNA) and ribosomal proteins, rRNA processing, and the assembly of the mature ribosome subunits in the nucleolus and subsequent transport into the cytoplasm (Rodnina and Wintermeyer, 2009). It requires coordinated and fine-tuned transcription catalyzed by all three RNA polymerases (RNA Pol I, II and III). RNA Pol I-driven transcription of the rRNA precursor (pre-rRNA) from multiple copies of the rDNA genes is considered as a rate-limiting step (Moss and Stefanovsky, 2002; Grummt, 2003; Chedin et al., 2007). Pre-rRNA contains 18S, 5.8S, and 28S rRNA species separated by internal transcribed spacers, ITS1 and ITS2, and flanked by 5′ and 3′ external transcribed spacers (5′-ETS and 3′ETS). These transcribed regions are removed by a series of cleavage steps in the nucleolus to generate mature rRNA species that are also extensively modified by methylation and pseudouridylation. These processing steps are meticulously regulated by a series of ribosome biogenesis factors, including small nucleolar RNAs (snoRNAs) and rRNA processing enzymes, ensuring accurate processing of pre-rRNA (Ashcroft et al., 2000; Baßler and Hurt, 2019; Bohnsack and Bohnsack, 2019; Singh et al., 2021; Dorner et al., 2023; Vanden Broeck and Klinge, 2023). 5S rRNA is transcribed by RNA pol III in the nucleoplasm and translocated into the nucleolus to be assembled into the large ribosome subunit. Concurrently, ribosomal proteins are assembled into ribosomal subunits by interacting with rRNA molecules through sequential and intricate assembly processes, which also require auxiliary factors such as chaperones and transport factors for accurate assembly and quality control. Once assembled, ribosomal subunits are ferried to the cytoplasm through nuclear pore complexes, where large and small subunits reunite to form functional ribosomes (Greber, 2016).
Ribosome biogenesis is essential for normal cell growth and proliferation. Defects in ribosome biogenesis are associated with a group of diseases collectively called ribosomopathies, including Diamond-Blackfan anemia (DBA), Dyskeratosis congenita (DKC), Treacher Collins syndrome (TCS), etc (Dai and Lu, 2008; Teng et al., 2013; Raiser et al., 2014; Danilova and Gazda, 2015; Mills and Green, 2017), whereas aberrant over-activation of ribosome biogenesis and translation is tightly linked to human cancer, thus being a therapeutic target (Bywater et al., 2012; Quin et al., 2014; Brighenti et al., 2015; Gentilella et al., 2015; Derenzini et al., 2017; Sulima et al., 2017; Pelletier et al., 2018). Therefore, it is crucial to understand how ribosome biogenesis is regulated during normal cell homeostasis and how it is deregulated in human diseases. Indeed, diverse cell growth or suppression signaling pathways have been shown to regulate ribosome biogenesis, such as mTOR, RAS, MYC oncogenic signaling, and p53, RB, and PTEN tumor suppressors (Dai et al., 2007; Dai and Lu, 2008). Recent studies including ours have shown that SUMOylation plays a critical role in regulating ribosome biogenesis. In this mini-review, we summarize the current understanding of SUMO regulation of ribosome biogenesis.
SUMOylation and its role in ribosome biogenesis
SUMOylation is a posttranslational modification of proteins by covalent conjugation of small ubiquitin-like modifiers (SUMOs), which plays a crucial role in the regulation of many cellular processes, including transcription, RNA splicing and processing, chromatin dynamics, DNA replication, DNA damage repair, cell cycle control, as well as ribosome biogenesis (Geiss-Friedlander and Melchior, 2007; Bergink and Jentsch, 2009; Finkbeiner et al., 2011a; Cubenas-Potts and Matunis, 2013; Jentsch and Psakhye, 2013; Raman et al., 2013; Chymkowitch et al., 2015a; Eifler and Vertegaal, 2015; Enserink, 2015; Nuro-Gyina and Parvin, 2015; Sarangi and Zhao, 2015). Mammals express three main SUMO isoforms, SUMO1-SUMO3. SUMO2 and SUMO3 are 97% identical (referred to as SUMO2/3) and each shares 45% sequence identity with SUMO1 (Kamitani et al., 1997; Kamitani et al., 1998a; Kamitani et al., 1998b; Muller et al., 2001; Geiss-Friedlander and Melchior, 2007). Like ubiquitination, SUMOylation occurs through sequential reactions involving a heterodimeric SUMO-activating enzyme SAE1/SAE2 (E1), a single SUMO-conjugating enzyme Ubc9 (E2) and a SUMO ligase (E3) in an ATP-dependent manner (Muller et al., 2001; Geiss-Friedlander and Melchior, 2007; Hay, 2013). In the final step, Ubc9 transfers SUMO to substrate acceptor lysine (Lys, K) residues via an isopeptide linkage, which is facilitated by SUMO E3s (Muller et al., 2001; Geiss-Friedlander and Melchior, 2007; Hay, 2013) (Figure 1). The SUMO acceptor Lys is often present within a conserved ΨKxE motif, where Ψ is a large hydrophobic amino acid and x is any amino acid (Rodriguez et al., 2001; Sampson et al., 2001; Geiss-Friedlander and Melchior, 2007; Gareau and Lima, 2010). Unlike ubiquitination which requires a ubiquitin (Ub) E3, SUMO E1 and Ubc9 can mediate SUMOylation, albeit with less efficiency, in the absence of SUMO E3. Also, while there are over 600 ubiquitin E3s in human (Deshaies and Joazeiro, 2009; Metzger et al., 2012), only a small number of SUMO E3 have been identified that are classified into three categories: a family of SP-RING-containing proteins including PIAS/siz family members (PIAS1, PIAS3, PIASxα, PIASxβ and PIASy (Kahyo et al., 2001; Sachdev et al., 2001; Nakagawa and Yokosawa, 2002; Nishida and Yasuda, 2002; Schmidt and Muller, 2002)) and Nse2 (Andrews et al., 2005; Potts and Yu, 2005; Berkholz et al., 2014), the nuclear pore Ran Binding Protein 2 (RanBP2) (Pichler et al., 2002; Reverter and Lima, 2005), and the ZNF451 family proteins (ZNF451-1, ZNF451-2, ZNF451-3) (Cappadocia et al., 2015; Eisenhardt et al., 2015; Varejao et al., 2020). In addition, a few other proteins possess SUMO E3-like activity such as Pc2, RSUME, TRIM28, SLX4 (Kagey et al., 2003; Morita et al., 2005; Weger et al., 2005; Carbia-Nagashima et al., 2007; Pungaliya et al., 2007; Oh et al., 2010; Pelisch et al., 2010; Chu and Yang, 2011; Yang et al., 2011; Oh and Chung, 2013; Guervilly et al., 2015; Neo et al., 2015; Ouyang et al., 2015; Yamashita et al., 2016) and Cajal body marker protein Coilin (Lett et al., 2023). SUMOylation is also a reversible modification. A group of deSUMOylating enzymes or SUMO proteases, including SENP1-3, SENP5-7, DESI-1, DESI-2, and USPL1, catalyze the removal of SUMO from substrates (Hickey et al., 2012; Kunz et al., 2018). Therefore, SUMOylation is highly dynamic in cells.
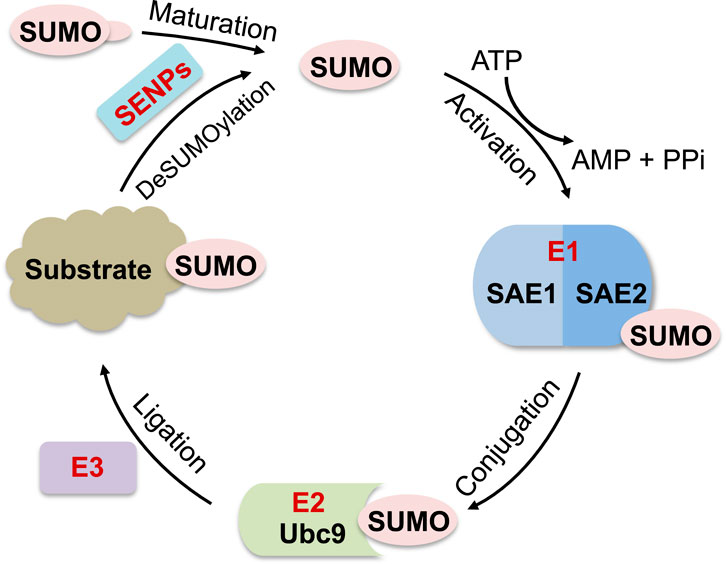
Figure 1. Diagram of SUMOylation pathway. SUMO precursors are cleaved by SUMO proteases (SENPs) to generate mature SUMOs with di-glycine at their C-terminus, which forms a thioester bond with the heterodimeric SUMO E1 (SAE1/SAE2) in an ATP-dependent manner. Activated SUMO is then transferred to SUMO E2 (Ubc9) also via forming a thioester bond with Ubc9. Finally, Ubc9 conjugates SUMO to substrates, which is facilitated by a SUMO E3. SUMOylated protein substrates can be deSUMOylated by SUMO proteases.
The SUMO modification facilitates and/or stabilizes protein-protein interaction through SUMO binding to the SUMO-interacting motif (SIM) in other proteins (Gareau and Lima, 2010; Jentsch and Psakhye, 2013). SIMs are characterized by a short stretch of core hydrophobic amino acids (V/I)x(V/I)(V/I) flanked by acidic residues (Song et al., 2004; Hannich et al., 2005; Song et al., 2005; Kerscher, 2007). SUMOylation can also interfere with protein-protein interactions through steric hindrance (Moldovan et al., 2006) or compete with other lysine-directed modifications like acetylation or ubiquitination (Desterro et al., 1998). Consequently, SUMOylation can regulate protein localization, trafficking, stability and activity (Gareau and Lima, 2010; Jentsch and Psakhye, 2013). Interestingly, emerging evidence suggests that SUMOylation tends to target an entire group of functionally and physically connected proteins, called protein group SUMOylation (Psakhye and Jentsch, 2012; Jentsch and Psakhye, 2013), such as DNA damage repair proteins (Psakhye and Jentsch, 2012), yeast septins (Johnson and Blobel, 1999; Johnson and Gupta, 2001; Takahashi et al., 2001), telomeres (Potts and Yu, 2007; Ferreira et al., 2011; Hang et al., 2011) and ribosome biogenesis-related proteins (Panse et al., 2006; Finkbeiner et al., 2011a; Finkbeiner et al., 2011b; Castle et al., 2012). Multiple SUMO-SIM interactions contribute to the formation and stabilization of the multi-protein complexes (Psakhye and Jentsch, 2012; Jentsch and Psakhye, 2013).
SUMOylation has been shown to play a vital role in regulating ribosome biogenesis (Han et al., 2018). It was initially found that many ribosome biogenesis factors and pre-ribosomal particles are modified by SUMO and the SUMOylation of pre-ribosomal particles in the nucleus and subsequent deSUMOylation at the nuclear pore complex (NPC) is necessary for efficient ribosome biogenesis and export in yeast (Panse et al., 2006). Recent proteomic studies found that ribosome biogenesis-related proteins are one of the major groups of SUMOylated proteins (Vertegaal et al., 2004; Matafora et al., 2009; Amente et al., 2012; Hendriks et al., 2014). In human, several nucleolar proteins critical for rRNA synthesis, processing and ribosome maturation, such as nucleophosmin (NPM) (Tago et al., 2005; Liu et al., 2007), nucleolin (Zhang et al., 2015) and Las1L (Finkbeiner et al., 2011b; Castle et al., 2012), are modified by SUMOylation. Interestingly, proteins (e.g., Nop58, Nhp2 and DKC1) in small nucleolar ribonucleoprotein (snoRNP) complexes that are responsible for rRNA 2′-O-ribose methylation and pseudouridylation critical for rRNA processing (Mannoor et al., 2012; Watkins and Bohnsack, 2012; Lui and Lowe, 2013; Dupuis-Sandoval et al., 2015) are modified by SUMO (Matic et al., 2010; Westman et al., 2010). SUMOylation of Nop58 has been shown to be essential for its efficient binding to snoRNAs (Westman et al., 2010). Similarly, components of the RNA degradation rixosome or Rix1 complex (PELP1, TEX10, WDR18, LAS1L, NOL9, MDN1, and SENP3) critical for ribosome biogenesis (Raman et al., 2016; Chen et al., 2018; Vanden Broeck and Klinge, 2023) and gene silencing (Shipkovenska et al., 2020; Zhou et al., 2022; Zhou et al., 2023), are also modified by SUMOylation. PELP1 SUMOylation is critical for ribosome maturation by promoting the recruitment of MDN1, an AAA ATPase that removes assembly factors from the pre-60S particles (Chen et al., 2018). On the other hand, the removal of SUMO via deSUMOylation is also important for ribosome biogenesis. DeSUMOylation of NPM, a nucleolar protein required for rRNA processing (Savkur and Olson, 1998; Itahana et al., 2003), by SENP3, is critical for 28S rRNA maturation and the subsequent nucleolar export of the 60S pre-ribosomal subunit (Haindl et al., 2008). Depletion of SENP3 inhibits rRNA processing reminiscent of the NPM knockdown (Yun et al., 2008). Likewise, deSUMOylation of LAS1L and PELP1 by SENP3 is essential for these proteins partitioning from the nucleoplasm to nucleolus (Finkbeiner et al., 2011b; Castle et al., 2012), and deSUMOylation of PELP1 is needed to release both MDN1 and PELP1 from pre-ribosomes (Raman et al., 2016). Thus, balanced levels of SUMOylation and deSUMOylation of ribosome biogenesis factors are critical for ribosome biogenesis and maturation in the nucleus.
SUMOylation also regulates rDNA transcription. Genome-wide binding of SUMO pathway proteins in both human fibroblast cells and yeast showed that SUMO is widely distributed in pro-growth genes, including ribosomal protein genes (RPGs), tRNA genes and rDNA (Neyret-Kahn et al., 2013; Chymkowitch et al., 2015b). However, there are conflicting results regarding how SUMOylation regulates the transcription of these ribosome biogenesis-related genes. SUMOylation of Rap1 has been shown to stimulate the recruitment of basal transcription factors to promote RPG transcription in yeast (Chymkowitch et al., 2015b). Consistently, the recruitment of SUMO isopeptidase Ulp2 to rDNA promotes rDNA silencing by opposing the degradation of silencing protein Tof2 and Net1 mediated by the SUMO-targeted ubiquitin ligase (STUBL) Slx5:Slx8 (Gillies et al., 2016; Liang et al., 2017). Deletion of Ulp2 leads to the increased expression of rRNA, snoRNAs, RP genes, and ribosome biogenesis factors (Ryu et al., 2018). Yet, other studies have suggested that SUMOylation negatively regulates rRNA transcription by mediating rDNA silencing. Both SUMO and Ubc9 are required for efficient repression of interrupted rDNA units and variable expression of intact rDNA. Disruption of the SUMO pathway leads to uniformly high expression of individual rDNA in single cells (Luo et al., 2020). Similarly, inhibiting SUMOylation by knockdown of Ubc9 has been shown to promote gene transcription resulting in senescence in human fibroblast cells (Neyret-Kahn et al., 2013). SUMOylation-induced inhibition of upstream-binding factor (UBF) and c-Myc expression was shown to be primarily responsible for the repression of rDNA transcription (Peng et al., 2019). Interestingly, accumulation of Tof2 due to the loss of the SUMO ligase Siz1 can also result in increased rDNA recombination (Abraham et al., 2019). rDNA is highly dynamic and vulnerable to excess recombination events. Also, rDNA damage repair via homologous recombination requires transient release of rDNA from the nucleolus that is controlled by SUMOylation of RAD52 (Torres-Rosell et al., 2007) and the CLIP-cohibin complex (Capella et al., 2021) in yeast. Thus, SUMOylation can prevent overactivation of rDNA recombination. Further studies are needed to clarify the cell context-dependent and dynamic role of SUMOylation in regulating rDNA transcription, recombination and damage repair.
USP36, a nucleolar deubiquitinating enzyme
USP36 was initially identified by sequence alignment and differential display in ovarian cancer cell lines as a ubiquitously expressed deubiquitinating enzyme (DUB) (Li et al., 2008). USP36 contains a ubiquitin-specific protease (USP) domain at its N-terminus and a nucleolar localization signal (NoLS) at its C-terminus (Endo et al., 2009a; Endo et al., 2009b), the rest of the protein are largely unstructured (Reed et al., 2015). Early work by Komada group has revealed that USP36 is a nucleolar DUB implicated in nucleolar structure and function in ribosomal biogenesis by deubiquitinating NPM and fibrillarin (Endo et al., 2009a; Endo et al., 2009b). Subsequently, USP36 has been shown to deubiquitinate and stabilize the RNA helicase DHX33 (Fraile et al., 2018). Also, human USP36 can restore the stability and levels of Rpa190, the largest subunit of RNA Pol I, pre-rRNA processing, and cell growth of yeast strain with the deletion of the yeast ortholog Ubp10 (Thevenon et al., 2009), suggesting that USP36 may regulate the ubiquitination and stability of human RNA Pol I. Knockdown of USP36 results in the morphological changes in the nucleolus and defects in rRNA synthesis and processing (Endo et al., 2009b; Fraile et al., 2018; Ryu et al., 2021; Chen et al., 2023). Thus, USP36 plays a critical role in the regulatory network of ribosome biogenesis and it is not surprising that homozygous deletion of the USP36 gene in mice is embryonic lethal (Fraile et al., 2018; Ryu et al., 2021). Furthermore, we identified that USP36 deubiquitinates c-Myc and stabilizes c-Myc in the nucleolus (Sun et al., 2015a; Sun et al., 2015b). c-Myc is considered as a master regulator of ribosome biogenesis as it promotes transcription by all three classes of RNA Polymerases (van Riggelen et al., 2010). The role of USP36 as a c-Myc DUB in promoting cell growth has been further demonstrated in a number of model systems including Drosophila, in which a nucleolar isoform of Drosohpila USP36 (dUSP36) stabilizes dMYC and is essential for cell growth and drosophila development (Liu et al., 2019; Zhang et al., 2019; Thevenon et al., 2020; Deng et al., 2022). In addition, USP36 has been shown to deubiquitinate and stabilize N-Myc (MYCN) in neuroblastoma cells (Juvvuna et al., 2021). Thus, by regulating c-Myc, USP36 also coordinates ribosome biogenesis with cell cycle progression. Together, it appears that the major cellular function of USP36 is to contribute to ribosome biogenesis in the nucleolus. Supporting this notion is that our recently purified USP36-associated protein complex was enriched ribosome biogenesis-related nucleolar proteins (Chen et al., 2023).
In addition to its role in ribosome biogenesis, USP36 also exerts notable effects in other cellular processes by deubiquitinating various substrates (Table 1), including cell survival, gene expression, and apoptosis, underscoring its multifunctionality in cells (Zhu et al., 2021). Like its yeast homolog Ubp10 (Gardner et al., 2005) and Drosophila homolog dUSP36/Scny (Buszczak et al., 2009), USP36 is a histone H2B deubiquitinating enzyme and regulates histone H2B modifications and thus gene transcription (DeVine et al., 2018). Drosophila USP36 interacts with immune deficiency (IMD) and prevents K63-polyubiquitinated IMD accumulation while promoting IMD degradation, thereby preventing constitutive immune activation (Thevenon et al., 2009). Deletion of dUSP36 also suppresses cell growth by inducing autophagy (Taillebourg et al., 2012). USP36 interacts with chromodomain helicase DNA binding protein 7 (CHD7) and deubiquitinates and stabilizes CHD7, resulting in increased expression of SOX9 to promote neuroblastoma growth (Mondal et al., 2018). USP36 also stabilizes mitochondria SOD2 (Kim et al., 2011) to protect cells from ischemia-induced apoptosis (Liu et al., 2019). Recently, it has also been shown that USP36 deubiquitinates and stabilizes the DNA damage-tolerant polymerase PrimPol, thus regulating chemotherapy response (Yan et al., 2020). USP36 has been shown to deubiquitinate and stabilize SNAIL2, thus promoting epithelial-mesenchymal transition (EMT) (Qiu et al., 2021). Likewise, USP36 deubiquitinates and stabilizes the nucleolar SNAIL1 to promote ribosome biogenesis and tumor cell survival under ribotoxic stress and contributes to chemoresistance (Qin et al., 2023). USP36 stabilizes YAP and regulates the Hippo signaling in esophageal squamous carcinoma cells (Zhang et al., 2022) and TAZ activation (Wang et al., 2022). USP36 regulates the levels of PKM2 to promote aerobic glycolysis (Wu et al., 2023). By deubiquitinating and stabilizing the m6A demethylase ALKBH5, USP36 regulates its function in gene expression and glioblastoma progression (Chang et al., 2023). USP36 also deubiquitinates ALR (augmenter of liver regeneration) and may play a role in the transition of the steatotic hepatocytes into malignant hepatocellular carcinoma (HCC) (Zheng et al., 2023). Thus, USP36 may play a crucial role in diverse cellular processes beyond ribosome biogenesis.
USP36 acts as a novel SUMO ligase
Intriguingly, we recently found that USP36 acts as a novel SUMO ligase to promote nucleolar protein SUMOylation (Ryu et al., 2021). USP36 has all SUMO E3 characteristics: 1) It binds to Ubc9; 2) It binds to SUMO; 3) It promotes SUMOylation of multiple proteins including components of snoRNPs in cells; 4) It SUMOylates multiple substrates, including Nop58, Nhp2 and PARP1, in vitro using recombinant proteins; 5) The full USP36’s SUMO E3 activity requires the SUMO interaction with the backside of Ubc9 (Ryu et al., 2021), as in the case of PIAS1 and ZNF451 family SUMO ligases (Cappadocia et al., 2015; Eisenhardt et al., 2015; Koidl et al., 2016; Eisenhardt et al., 2019). Mutating either Phe 22 of Ubc9 or Asp 63 of SUMO2, which disrupts the SUMO-Ubc9 backside interaction, markedly inhibited USP36’s activity to promote SUMOylation in vitro (Ryu et al., 2021). These data suggest that USP36 possesses a SUMO ligase activity, arguing that it acts as an adaptor protein to increase Ubc9 function. The SUMO ligase activity is located at the N-terminal domain which overlaps with the USP domain. Interestingly, mutating the DUB catalytic Cys 131 (C131) that inactivates USP36’s DUB activity also abolished its SUMO ligase activity (Ryu et al., 2021). We did find that mutating the DUB catalytic triad residue His 382 to Ala (H382A) abolishes its DUB, but not SUMO E3, activity (Ryu et al., 2021). However, mutant(s) specifically inactivating SUMO E3, but not DUB activity, are yet to be identified. Thus, further structural analyses are warranted to delineate the mechanism of the SUMO ligase activity and the crosstalk between its DUB activity and SUMO E3 activity. Nevertheless, to the best of our knowledge, USP36 is the only nucleolar SUMO ligase and the only protein with DUB and SUMO ligase dual functions identified so far.
Roles of USP36 in nucleolar SUMOylation
We showed that USP36 mainly promotes the nucleolar protein SUMOylation (Ryu et al., 2021), suggesting that its SUMO ligase function is linked to its role in regulating nucleolar dynamics and ribosome biogenesis (Table 1). Our initial proteomic analysis identified Nop58, a component of Box C/D snoRNP complex, as one of the proteins whose SUMOylation is increased by overexpression of USP36. We subsequently revealed that Nop56 from Box C/D snoRNP and Nhp2 and DKC1 from Box H/ACA snoRNP complex are all SUMOylated by USP36 (Ryu et al., 2021), supporting the notion that SUMOylation occurs in multiple proteins in protein complexes. Consistent with early studies showing that Nop58 SUMOylation regulates its binding to snoRNAs, we also showed that USP36-mediated SUMOylation promotes Nop58 binding to snoRNAs, implying that SUMO modification of the snoRNP complex may stabilize the complex and facilitate its conformation favoring snoRNA binding. We then purified USP36-associated protein complex that enriches ribosome biogenesis-related proteins, including all the ten components of the nucleolar RNA exosome complex. We found that USP36 interacts with the RNA exosome by binding to the exoribonuclease protein EXOSC10 (Chen et al., 2023) and SUMOylates EXOSC10 at Lys 583. We showed that USP36 SUMOylation of EXOSC10 promotes its binding to rRNA and the activity of the RNA exosome in rRNA processing, underscoring its role in maintaining rRNA biogenesis and cellular growth. Additionally, the perturbation of ribosome biogenesis (ribosomal stress) reduces USP36 levels and results in a noticeable reduction in EXOSC10 SUMOylation. Therefore, USP36 acts as a nucleolar SUMO E3 ligase to SUMOylate EXOSC10 and promotes the functionality of RNA exosomes within the realm of ribosome biogenesis. It would be interesting to test whether USP36 also promotes group SUMOylation of core exosome subunits and its adaptor proteins such as TRAMP complexes (Lubas et al., 2011; Kilchert et al., 2016; Zinder and Lima, 2017).
Notably, it appears that the levels and stability of both snoRNP and RNA exosome complex proteins are not significantly regulated by USP36, suggesting that at least under normal cell growth conditions, USP36 SUMO ligase activity may play a major role in regulating the targeting of these complexes to rRNA and thereby facilitating rRNA processing compared to its DUB activity. Further supporting the role of these regulations, we showed that USP36 is critical for multiple steps of rRNA processing, including RNA exosome-mediated 5.8S rRNA maturation as well as the maturation of both 18S and 28S rRNA. Knockdown of USP36 results in the accumulation of pre-rRNAs and reduction of 21S, 12S and 7S rRNA intermediates. Interestingly, we observed that USP36 strongly binds to pre-rRNA (Chen et al., 2023). The RNA-binding property of USP36 has also been shown by others wherein it binds to long noncoding RNAs (lncRNAs) (Mondal et al., 2018) and microRNAs (Treiber et al., 2017; Li et al., 2023). Indeed, it plays a role in microRNA biogenesis by binding to the microprocessor complex and mediating DGCR8 SUMOylation in the nucleolus (Li et al., 2023). These studies suggest that USP36 itself might be an RNA-binding protein. Further studies are warranted to examine whether USP36 binds to these RNAs directly or indirectly through its interacting partner proteins.
Our proteomic analysis revealed that USP36 associates with a large group of nucleolar proteins involved in ribosome biogenesis (Chen et al., 2023), suggesting that USP36 may mediate group SUMOylation of additional protein complexes in the nucleolus and possibly regulates SUMOylation-ubiquitination crosstalk. By doing so, USP36 may act as a nucleolar regulation hub controlling nucleolar dynamics and protein-RNA complex formation, stability, and activity in ribosome biogenesis.
Together, it is evident that USP36, an established DUB, is not solely confined to this role, as it also plays a pivotal role in orchestrating the SUMOylation of the nucleolar proteins involved in ribosome biogenesis, thereby extending the regulatory landscape of USP36 and shedding light on its multifaceted involvement in cellular processes.
USP36 is upregulated in cancers and could be a therapeutic target
An increasing body of research highlights the aberrant expression of USP36 in various cancers and its crucial role in tumor development and growth. USP36 has been shown to be overexpressed in various human cancers, including breast cancer (Sun et al., 2015a; Wu et al., 2023), ovarian cancer (Yan et al., 2020), esophageal squamous cell carcinoma (Zhang et al., 2022), gastric cancer (Wang et al., 2022), hepatocellular carcinoma (HCC) (Sun et al., 2022), lung cancer (Deng et al., 2022), neuroblastoma (Juvvuna et al., 2021), and glioblastoma (Chang et al., 2023). High expression of USP36 correlates with poor patient prognosis in these tumors. Consistently, the knockdown of USP36 suppresses cancer cell proliferation and xenograft tumor growth in various tumor types (Sun et al., 2015a; Juvvuna et al., 2021; Wang et al., 2022; Chang et al., 2023). These studies also established the regulation of an array of target proteins by USP36 via deubiquitination, whose expression is correlatively upregulated in these cancers, including MYC, EMT factor SNAILs (Qiu et al., 2021; Qin et al., 2023), YAP (Zhang et al., 2022) and TAZ (Wang et al., 2022) in Hippo signaling, PrimPol in DNA replication and replication stress (Chang et al., 2023), and PKM2 in glycolysis (Wu et al., 2023). It will be interesting to test whether these substrates are also regulated by USP36’s SUMO ligase activity. Intriguingly, it is observed that high USP36 expression positively correlated with both macrophage infiltration levels and multiple immune checkpoint molecules in HCC tissues (Sun et al., 2022). These studies, together with the established role of USP36 in ribosome biogenesis shed light on a potential role for USP36 in tumor growth and progression and thus as a promising therapeutic target in cancer.
Selective USP36 DUB inhibitor is currently not available. Future efforts are thus warranted to develop USP36 DUB-specific inhibitors. Particularly, USP36 possesses DUB/SUMO ligase dual activities and both activities positively regulate cell growth and proliferation. Thus, targeting both DUB and SUMO ligase activities would have extra benefit and this is likely, as we have observed that inactivating USP36’s DUB activity by mutating the DUB catalytic Cys 131 also abolishes its SUMO ligase activity. Developing inhibitors specifically targeting USP36’s SUMO ligase activity would be even more promising as this could avoid the non-specific effects of DUB inhibitors. Cancer cell proliferation and survival often require sustained oncogenic signaling called oncogene addiction, as well as elevated ribosome biogenesis and protein translation. Therefore, inhibiting ribosome biogenesis would be synthetic lethal to these cancer cells addictive to oncogenic signals, providing a therapeutic window to overcome USP36’s essential role in normal cell growth. Supporting the therapeutic role of USP36, ribosome biogenesis has emerged as a key therapeutic target. Early chemotherapeutic compounds such as actinomycin D, etoposide, and 5-fluorouracil inhibit ribosome biogenesis by blocking rRNA synthesis and/or inhibiting rRNA processing (Ferreira et al., 2020). The small molecule CX-5461 is a potent selective inhibitor of RNA Pol I transcription by targeting the interaction between SL-1 transcription factor complex and RNA Pol I (Drygin et al., 2011; Bywater et al., 2012; Haddach et al., 2012). Recent phase I clinical trial showed that CX-5461 has anti-tumor activity against advanced hematologic cancers (Khot et al., 2019). In addition, inhibiting USP36 would also have therapeutic effects via targeting cellular pathways depicted above beyond ribosome biogenesis. A recent study reported that a natural product extracted from Baccharis coridifolia, OST-01, has anti-leukemic activity by targeting c-MYC via down-regulating USP36, although it is not clear whether USP36 is the direct target of OST-01 (Kang et al., 2024). Future studies will delve deeper into the feasibility of USP36 as a potential therapeutic target, offering novel insights for the advancement of cancer treatment strategies.
Perspective
The exploration of USP36’s multifaceted roles in ribosome biogenesis has yielded valuable insights into its intricate functions on cell growth and proliferation. As we consider the implications of these findings, several exciting perspectives and potential avenues for future research emerge. Identifying additional substrates in the nucleolus and deciphering their influence on various cellular processes beyond ribosome biogenesis would delve into the broader landscape of USP36-mediated SUMOylation. Elucidating the mechanistic details of how USP36 promotes malignancy and its interactions with specific oncogenic pathways could pave the way for the development of targeted therapies that disrupt these processes and hinder tumor growth. Structural and mechanistic characterization of USP36 to differentiate its DUB activity from SUMO ligase activity would help develop DUB-specific, SUMO ligase-specific, or DUB/SUMO ligase dual inhibitors. Investigating potential crosstalk between USP36 and other cellular pathways could integrate into broader cellular networks, such as DNA repair, cell cycle control, and autophagy, providing new perspectives on its role in maintaining cellular homeostasis. In conclusion, the multifaceted roles of USP36 in ribosome biogenesis, SUMOylation, and cancer have set the stage for exciting future research endeavors.
Author contributions
YY: Writing–original draft. YL: Writing–review and editing. RS: Writing–review and editing. X-XS: Writing–original draft, Writing–review and editing. M-SD: Writing–original draft, Writing–review and editing.
Funding
The author(s) declare that financial support was received for the research, authorship, and/or publication of this article. NIH grants R01 GM130604 and R01 CA262104 to M-SD.
Conflict of interest
The authors declare that the research was conducted in the absence of any commercial or financial relationships that could be construed as a potential conflict of interest.
Publisher’s note
All claims expressed in this article are solely those of the authors and do not necessarily represent those of their affiliated organizations, or those of the publisher, the editors and the reviewers. Any product that may be evaluated in this article, or claim that may be made by its manufacturer, is not guaranteed or endorsed by the publisher.
References
Abraham, N. M., Ramalingam, K., Murthy, S., and Mishra, K. (2019). Siz2 prevents ribosomal DNA recombination by modulating levels of Tof2 in Saccharomyces cerevisiae. mSphere 4, e00713-19. doi:10.1128/msphere.00713-19
Amente, S., Lavadera, M. L., Palo, G. D., and Majello, B. (2012). SUMO-activating SAE1 transcription is positively regulated by Myc. Am. J. Cancer Res. 2, 330–334.
Andrews, E. A., Palecek, J., Sergeant, J., Taylor, E., Lehmann, A. R., and Watts, F. Z. (2005). Nse2, a component of the Smc5-6 complex, is a SUMO ligase required for the response to DNA damage. Mol. Cell. Biol. 25, 185–196. doi:10.1128/mcb.25.1.185-196.2005
Ashcroft, M., Taya, Y., and Vousden, K. H. (2000). Stress signals utilize multiple pathways to stabilize p53. Mol. Cell. Biol. 20, 3224–3233. doi:10.1128/mcb.20.9.3224-3233.2000
Baßler, J., and Hurt, E. (2019). Eukaryotic ribosome assembly. Annu. Rev. Biochem. 88, 281–306. doi:10.1146/annurev-biochem-013118-110817
Bergink, S., and Jentsch, S. (2009). Principles of ubiquitin and SUMO modifications in DNA repair. Nature 458, 461–467. doi:10.1038/nature07963
Berkholz, J., Michalick, L., and Munz, B. (2014). The E3 SUMO ligase Nse2 regulates sumoylation and nuclear-to-cytoplasmic translocation of skNAC-Smyd1 in myogenesis. J. Cell Sci. 127, 3794–3804. doi:10.1242/jcs.150334
Bohnsack, K. E., and Bohnsack, M. T. (2019). Uncovering the assembly pathway of human ribosomes and its emerging links to disease. EMBO J. 38, e100278. doi:10.15252/embj.2018100278
Brighenti, E., Trere, D., and Derenzini, M. (2015). Targeted cancer therapy with ribosome biogenesis inhibitors: a real possibility? Oncotarget 6, 38617–38627. doi:10.18632/oncotarget.5775
Buszczak, M., Paterno, S., and Spradling, A. C. (2009). Drosophila stem cells share a common requirement for the histone H2B ubiquitin protease scrawny. Science 323, 248–251. doi:10.1126/science.1165678
Bywater, M. J., Poortinga, G., Sanij, E., Hein, N., Peck, A., Cullinane, C., et al. (2012). Inhibition of RNA polymerase I as a therapeutic strategy to promote cancer-specific activation of p53. Cancer Cell 22, 51–65. doi:10.1016/j.ccr.2012.05.019
Capella, M., Mandemaker, I. K., Martín Caballero, L., den Brave, F., Pfander, B., Ladurner, A. G., et al. (2021). Nucleolar release of rDNA repeats for repair involves SUMO-mediated untethering by the Cdc48/p97 segregase. Nat. Commun. 12, 4918. doi:10.1038/s41467-021-25205-2
Cappadocia, L., Pichler, A., and Lima, C. D. (2015). Structural basis for catalytic activation by the human ZNF451 SUMO E3 ligase. Nat. Struct. Mol. Biol. 22, 968–975. doi:10.1038/nsmb.3116
Carbia-Nagashima, A., Gerez, J., Perez-Castro, C., Paez-Pereda, M., Silberstein, S., Stalla, G. K., et al. (2007). RSUME, a small RWD-containing protein, enhances SUMO conjugation and stabilizes HIF-1α during hypoxia. Cell 131, 309–323. doi:10.1016/j.cell.2007.07.044
Castle, C. D., Cassimere, E. K., and Denicourt, C. (2012). LAS1L interacts with the mammalian Rix1 complex to regulate ribosome biogenesis. Mol. Biol. Cell 23, 716–728. doi:10.1091/mbc.e11-06-0530
Chang, G., Xie, G. S., Ma, L., Li, L., and Richard, H. T. (2023). USP36 promotes tumorigenesis and drug sensitivity of glioblastoma by deubiquitinating and stabilizing ALKBH5. Neuro-Oncology 25, 841–853. doi:10.1093/neuonc/noac238
Chedin, S., Laferté, A., Hoang, T., Lafontaine, D. L., Riva, M., and Carles, C. (2007). Is ribosome synthesis controlled by pol I transcription? Cell Cycle 6, 11–15. doi:10.4161/cc.6.1.3649
Chen, Y., Li, Y., Dai, R. S., Savage, J. C., Shinde, U., Klimek, J., et al. (2023). The ubiquitin-specific protease USP36 SUMOylates EXOSC10 and promotes the nucleolar RNA exosome function in rRNA processing. Nucleic Acids Res. 51, 3934–3949. doi:10.1093/nar/gkad140
Chen, Z., Suzuki, H., Kobayashi, Y., Wang, A. C., DiMaio, F., Kawashima, S. A., et al. (2018). Structural insights into Mdn1, an essential AAA protein required for ribosome biogenesis. Cell 175, 822–834. e18. doi:10.1016/j.cell.2018.09.015
Chu, Y., and Yang, X. (2011). SUMO E3 ligase activity of TRIM proteins. Oncogene 30, 1108–1116. doi:10.1038/onc.2010.462
Chymkowitch, P., Nguéa P, A., Aanes, H., Koehler, C. J., Thiede, B., Lorenz, S., et al. (2015b). Sumoylation of Rap1 mediates the recruitment of TFIID to promote transcription of ribosomal protein genes. Genome Res. 25, 897–906. doi:10.1101/gr.185793.114
Chymkowitch, P., Nguéa P, A., and Enserink, J. M. (2015a). SUMO-regulated transcription: challenging the dogma. Bioessays 37, 1095–1105. doi:10.1002/bies.201500065
Cubenas-Potts, C., and Matunis, M. J. (2013). SUMO: a multifaceted modifier of chromatin structure and function. Dev. Cell 24, 1–12. doi:10.1016/j.devcel.2012.11.020
Dai, M. S., and Lu, H. (2008). Crosstalk between c-Myc and ribosome in ribosomal biogenesis and cancer. J. Cell. Biochem. 105, 670–677. doi:10.1002/jcb.21895
Dai, M. S., Sears, R., and Lu, H. (2007). Feedback regulation of c-Myc by ribosomal protein L11. Cell Cycle 6, 2735–2741. doi:10.4161/cc.6.22.4895
Danilova, N., and Gazda, H. T. (2015). Ribosomopathies: how a common root can cause a tree of pathologies. Dis. Models Mech. 8, 1013–1026. doi:10.1242/dmm.020529
Deng, Q., Wu, M., and Deng, J. (2022). USP36 promotes tumor growth of non-small cell lung cancer via increasing KHK-A expression by regulating c-MYC-hnRNPH1/H2 axis. Hum. Cell 35, 694–704. doi:10.1007/s13577-022-00677-6
Derenzini, M., Montanaro, L., and Trere, D. (2017). Ribosome biogenesis and cancer. Acta Histochem. 119, 190–197. doi:10.1016/j.acthis.2017.01.009
Deshaies, R. J., and Joazeiro, C. A. (2009). RING domain E3 ubiquitin ligases. Annu. Rev. Biochem. 78, 399–434. doi:10.1146/annurev.biochem.78.101807.093809
Desterro, J. M., Rodriguez, M. S., and Hay, R. T. (1998). SUMO-1 modification of IκBα inhibits NF-κB activation. Mol. Cell 2, 233–239. doi:10.1016/s1097-2765(00)80133-1
DeVine, T., Sears, R. C., and Dai, M. S. (2018). The ubiquitin-specific protease USP36 is a conserved histone H2B deubiquitinase. Biochem. Biophysical Res. Commun. 495, 2363–2368. doi:10.1016/j.bbrc.2017.12.107
Dorner, K., Ruggeri, C., Zemp, I., and Kutay, U. (2023). Ribosome biogenesis factors-from names to functions. EMBO J. 42, e112699. doi:10.15252/embj.2022112699
Drygin, D., Lin, A., Bliesath, J., Ho, C. B., O’Brien, S. E., Proffitt, C., et al. (2011). Targeting RNA polymerase I with an oral small molecule CX-5461 inhibits ribosomal RNA synthesis and solid tumor growth. Cancer Res. 71, 1418–1430. doi:10.1158/0008-5472.can-10-1728
Dupuis-Sandoval, F., Poirier, M., and Scott, M. S. (2015). The emerging landscape of small nucleolar RNAs in cell biology. WIREs RNA 6, 381–397. doi:10.1002/wrna.1284
Eifler, K., and Vertegaal, A. C. (2015). SUMOylation-mediated regulation of cell cycle progression and cancer. Trends Biochem. Sci. 40, 779–793. doi:10.1016/j.tibs.2015.09.006
Eisenhardt, N., Chaugule, V. K., Koidl, S., Droescher, M., Dogan, E., Rettich, J., et al. (2015). A new vertebrate SUMO enzyme family reveals insights into SUMO-chain assembly. Nat. Struct. Mol. Biol. 22, 959–967. doi:10.1038/nsmb.3114
Eisenhardt, N., Ilic, D., Nagamalleswari, E., and Pichler, A. (2019). Biochemical characterization of SUMO-conjugating enzymes by in vitro sumoylation assays. Methods Enzymol. 618, 167–185. doi:10.1016/bs.mie.2018.12.025
Endo, A., Kitamura, N., and Komada, M. (2009a). Nucleophosmin/B23 regulates ubiquitin dynamics in nucleoli by recruiting deubiquitylating enzyme USP36. J. Biol. Chem. 284, 27918–27923. doi:10.1074/jbc.m109.037218
Endo, A., Matsumoto, M., Inada, T., Yamamoto, A., Nakayama, K. I., Kitamura, N., et al. (2009b). Nucleolar structure and function are regulated by the deubiquitylating enzyme USP36. J. Cell Sci. 122, 678–686. doi:10.1242/jcs.044461
Enserink, J. M. (2015). Sumo and the cellular stress response. Cell Div. 10, 4. doi:10.1186/s13008-015-0010-1
Ferreira, H. C., Luke, B., Schober, H., Kalck, V., Lingner, J., and Gasser, S. M. (2011). The PIAS homologue Siz2 regulates perinuclear telomere position and telomerase activity in budding yeast. Nat. Cell Biol. 13, 867–874. doi:10.1038/ncb2263
Ferreira, R., Schneekloth, J. S., Panov, K. I., Hannan, K. M., and Hannan, R. D. (2020). Targeting the RNA polymerase I transcription for cancer therapy comes of age. Cells 9, 266. doi:10.3390/cells9020266
Finkbeiner, E., Haindl, M., and Muller, S. (2011b). The SUMO system controls nucleolar partitioning of a novel mammalian ribosome biogenesis complex. EMBO J. 30, 1067–1078. doi:10.1038/emboj.2011.33
Finkbeiner, E., Haindl, M., Raman, N., and Muller, S. (2011a). SUMO routes ribosome maturation. Nucleus 2, 527–532. doi:10.4161/nucl.2.6.17604
Fraile, J. M., Campos-Iglesias, D., Rodríguez, F., Astudillo, A., Vilarrasa-Blasi, R., Verdaguer-Dot, N., et al. (2018). Loss of the deubiquitinase USP36 destabilizes the RNA helicase DHX33 and causes preimplantation lethality in mice. J. Biol. Chem. 293, 2183–2194. doi:10.1074/jbc.m117.788430
Gardner, R. G., Nelson, Z. W., and Gottschling, D. E. (2005). Ubp10/Dot4p regulates the persistence of ubiquitinated histone H2B: distinct roles in telomeric silencing and general chromatin. Mol. Cell. Biol. 25, 6123–6139. doi:10.1128/mcb.25.14.6123-6139.2005
Gareau, J. R., and Lima, C. D. (2010). The SUMO pathway: emerging mechanisms that shape specificity, conjugation and recognition. Nat. Rev. Mol. Cell Biol. 11, 861–871. doi:10.1038/nrm3011
Geiss-Friedlander, R., and Melchior, F. (2007). Concepts in sumoylation: a decade on. Nat. Rev. Mol. Cell Biol. 8, 947–956. doi:10.1038/nrm2293
Gentilella, A., Kozma, S. C., and Thomas, G. (2015). A liaison between mTOR signaling, ribosome biogenesis and cancer. Biochimica Biophysica Acta (BBA) - Gene Regul. Mech. 1849, 812–820. doi:10.1016/j.bbagrm.2015.02.005
Gillies, J., Hickey, C. M., Su, D., Wu, Z., Peng, J., and Hochstrasser, M. (2016). SUMO pathway modulation of regulatory protein binding at the ribosomal DNA locus in Saccharomyces cerevisiae. Genetics 202, 1377–1394. doi:10.1534/genetics.116.187252
Greber, B. J. (2016). Mechanistic insight into eukaryotic 60S ribosomal subunit biogenesis by cryo-electron microscopy. Rna 22, 1643–1662. doi:10.1261/rna.057927.116
Grummt, I. (2003). Life on a planet of its own: regulation of RNA polymerase I transcription in the nucleolus. Genes Dev. 17, 1691–1702. doi:10.1101/gad.1098503R
Guervilly, J. H., Takedachi, A., Naim, V., Scaglione, S., Chawhan, C., Lovera, Y., et al. (2015). The SLX4 complex is a SUMO E3 ligase that impacts on replication stress outcome and genome stability. Mol. Cell 57, 123–137. doi:10.1016/j.molcel.2014.11.014
Haddach, M., Schwaebe, M. K., Michaux, J., Nagasawa, J., O’Brien, S. E., Whitten, J. P., et al. (2012). Discovery of CX-5461, the first direct and selective inhibitor of RNA polymerase I, for cancer therapeutics. ACS Med. Chem. Lett. 3, 602–606. doi:10.1021/ml300110s
Haindl, M., Harasim, T., Eick, D., and Muller, S. (2008). The nucleolar SUMO-specific protease SENP3 reverses SUMO modification of nucleophosmin and is required for rRNA processing. EMBO Rep. 9, 273–279. doi:10.1038/embor.2008.3
Han, Z. J., Feng, Y. H., Gu, B. H., Li, Y. M., and Chen, H. (2018). The post-translational modification, SUMOylation, and cancer (Review). Int. J. Oncol. 52, 1081–1094. doi:10.3892/ijo.2018.4280
Hang, L. E., Liu, X., Cheung, I., Yang, Y., and Zhao, X. (2011). SUMOylation regulates telomere length homeostasis by targeting Cdc13. Nat. Struct. Mol. Biol. 18, 920–926. doi:10.1038/nsmb.2100
Hannich, J. T., Lewis, A., Kroetz, M. B., Li, S. J., Heide, H., Emili, A., et al. (2005). Defining the SUMO-modified proteome by multiple approaches in Saccharomyces cerevisiae. J. Biol. Chem. 280, 4102–4110. doi:10.1074/jbc.m413209200
Hay, R. T. (2013). Decoding the SUMO signal. Biochem. Soc. Trans. 41, 463–473. doi:10.1042/bst20130015
Hendriks, I. A., D’Souza, R. C. J., Yang, B., Verlaan-de Vries, M., Mann, M., and Vertegaal, A. C. O. (2014). Uncovering global SUMOylation signaling networks in a site-specific manner. Nat. Struct. Mol. Biol. 21, 927–936. doi:10.1038/nsmb.2890
Hickey, C. M., Wilson, N. R., and Hochstrasser, M. (2012). Function and regulation of SUMO proteases. Nat. Rev. Mol. Cell Biol. 13, 755–766. doi:10.1038/nrm3478
Itahana, K., Bhat, K. P., Jin, A., Itahana, Y., Hawke, D., Kobayashi, R., et al. (2003). Tumor suppressor ARF degrades B23, a nucleolar protein involved in ribosome biogenesis and cell proliferation. Mol. Cell 12, 1151–1164. doi:10.1016/s1097-2765(03)00431-3
Jentsch, S., and Psakhye, I. (2013). Control of nuclear activities by substrate-selective and protein-group SUMOylation. Annu. Rev. Genet. 47, 167–186. doi:10.1146/annurev-genet-111212-133453
Johnson, E. S., and Blobel, G. (1999). Cell cycle-regulated attachment of the ubiquitin-related protein SUMO to the yeast septins. J. Cell Biol. 147, 981–994. doi:10.1083/jcb.147.5.981
Johnson, E. S., and Gupta, A. A. (2001). An E3-like factor that promotes SUMO conjugation to the yeast septins. Cell 106, 735–744. doi:10.1016/s0092-8674(01)00491-3
Juvvuna, P. K., Mondal, T., Di Marco, M., Kosalai, S. T., Kanduri, M., and Kanduri, C. (2021). NBAT1/CASC15-003/USP36 control MYCN expression and its downstream pathway genes in neuroblastoma. Neuro-Oncology Adv. 3, vdab056. doi:10.1093/noajnl/vdab056
Kagey, M. H., Melhuish, T. A., and Wotton, D. (2003). The polycomb protein Pc2 is a SUMO E3. Cell 113, 127–137. doi:10.1016/s0092-8674(03)00159-4
Kahyo, T., Nishida, T., and Yasuda, H. (2001). Involvement of PIAS1 in the sumoylation of tumor suppressor p53. Mol. Cell 8, 713–718. doi:10.1016/s1097-2765(01)00349-5
Kamitani, T., Kito, K., Nguyen, H. P., Fukuda-Kamitani, T., and Yeh, E. T. (1998a). Characterization of a second member of the sentrin family of ubiquitin-like proteins. J. Biol. Chem. 273, 11349–11353. doi:10.1074/jbc.273.18.11349
Kamitani, T., Nguyen, H. P., Kito, K., Fukuda-Kamitani, T., and Yeh, E. T. (1998b). Covalent modification of PML by the sentrin family of ubiquitin-like proteins. J. Biol. Chem. 273, 3117–3120. doi:10.1074/jbc.273.6.3117
Kamitani, T., Nguyen, H. P., and Yeh, E. T. (1997). Preferential modification of nuclear proteins by a novel ubiquitin-like molecule. J. Biol. Chem. 272, 14001–14004. doi:10.1074/jbc.272.22.14001
Kang, H., Hoang, D. H., Valerio, M., Pathak, K., Zhang, L., Buettner, R., et al. (2024). OST-01, a natural product from Baccharis coridifolia, targets c-Myc-dependent ribogenesis in acute myeloid leukemia. Leukemia 38, 657–662. doi:10.1038/s41375-024-02146-5
Kerscher, O. (2007). SUMO junction-what’s your function? New insights through SUMO-interacting motifs. EMBO Rep. 8, 550–555. doi:10.1038/sj.embor.7400980
Khot, A., Brajanovski, N., Cameron, D. P., Hein, N., Maclachlan, K. H., Sanij, E., et al. (2019). First-in-Human RNA polymerase I transcription inhibitor CX-5461 in patients with advanced hematologic cancers: results of a phase I dose-escalation study. Cancer Discov. 9, 1036–1049. doi:10.1158/2159-8290.cd-18-1455
Kilchert, C., Wittmann, S., and Vasiljeva, L. (2016). The regulation and functions of the nuclear RNA exosome complex. Nat. Rev. Mol. Cell Biol. 17, 227–239. doi:10.1038/nrm.2015.15
Kim, M. S., Ramakrishna, S., Lim, K. H., Kim, J. H., and Baek, K. H. (2011). Protein stability of mitochondrial superoxide dismutase SOD2 is regulated by USP36. J. Cell. Biochem. 112, 498–508. doi:10.1002/jcb.22940
Koidl, S., Eisenhardt, N., Fatouros, C., Droescher, M., Chaugule, V. K., and Pichler, A. (2016). The SUMO2/3 specific E3 ligase ZNF451-1 regulates PML stability. Int. J. Biochem. Cell Biol. 79, 478–487. doi:10.1016/j.biocel.2016.06.011
Kunz, K., Piller, T., and Muller, S. (2018). SUMO-specific proteases and isopeptidases of the SENP family at a glance. J. Cell Sci. 131, jcs211904. doi:10.1242/jcs.211904
Lett, K. E. M., McLaurin, D. M., Tucker, S. K., and Hebert, M. D. (2023). The Cajal body marker protein coilin is SUMOylated and possesses SUMO E3 ligase-like activity. Front. RNA Res. 1, 1197990. doi:10.3389/frnar.2023.1197990
Li, J., Olson, L. M., Zhang, Z., Li, L., Bidder, M., Nguyen, L., et al. (2008). Differential display identifies overexpression of the USP36 gene, encoding a deubiquitinating enzyme, in ovarian cancer. Int. J. Med. Sci. 5, 133–142. doi:10.7150/ijms.5.133
Li, Y., Carey, T. S., Feng, C. H., Zhu, H. M., Sun, X. X., and Dai, M. S. (2023). The ubiquitin-specific protease USP36 associates with the microprocessor complex and regulates miRNA biogenesis by SUMOylating DGCR8. Cancer Res. Commun. 3, 459–470. doi:10.1158/2767-9764.crc-22-0344
Liang, J., Singh, N., Carlson, C. R., Albuquerque, C. P., Corbett, K. D., and Zhou, H. (2017). Recruitment of a SUMO isopeptidase to rDNA stabilizes silencing complexes by opposing SUMO targeted ubiquitin ligase activity. Genes Dev. 31, 802–815. doi:10.1101/gad.296145.117
Liu, Q., Sheng, W., Ma, Y., Zhen, J., Roy, S., Alvira Jafar, C., et al. (2019). USP36 protects proximal tubule cells from ischemic injury by stabilizing c-Myc and SOD2. Biochem. Biophysical Res. Commun. 513, 502–508. doi:10.1016/j.bbrc.2019.03.043
Liu, X., Liu, Z., Jang, S. W., Ma, Z., Shinmura, K., Kang, S., et al. (2007). Sumoylation of nucleophosmin/B23 regulates its subcellular localization, mediating cell proliferation and survival. Proc. Natl. Acad. Sci. U. S. A. 104, 9679–9684. doi:10.1073/pnas.0701806104
Lubas, M., Christensen, M., Kristiansen, M., Domanski, M., Falkenby, L., Lykke-Andersen, S., et al. (2011). Interaction profiling identifies the human nuclear exosome targeting complex. Mol. Cell 43, 624–637. doi:10.1016/j.molcel.2011.06.028
Lui, L., and Lowe, T. (2013). Small nucleolar RNAs and RNA-guided post-transcriptional modification. Essays Biochem. 54, 53–77. doi:10.1042/bse0540053
Luo, Y., Fefelova, E., Ninova, M., Chen, Y. C. A., and Aravin, A. A. (2020). Repression of interrupted and intact rDNA by the SUMO pathway in Drosophila melanogaster. Elife 9, e52416. doi:10.7554/elife.52416
Mannoor, K., Liao, J., and Jiang, F. (2012). Small nucleolar RNAs in cancer. Biochimica Biophysica Acta (BBA) - Rev. Cancer 1826, 121–128. doi:10.1016/j.bbcan.2012.03.005
Matafora, V., D’Amato, A., Mori, S., Blasi, F., and Bachi, A. (2009). Proteomics analysis of nucleolar SUMO-1 target proteins upon proteasome inhibition. Mol. Cell. Proteomics 8, 2243–2255. doi:10.1074/mcp.m900079-mcp200
Matic, I., Schimmel, J., Hendriks, I. A., van Santen, M. A., van de Rijke, F., van Dam, H., et al. (2010). Site-specific identification of SUMO-2 targets in cells reveals an inverted SUMOylation motif and a hydrophobic cluster SUMOylation motif. Mol. Cell 39, 641–652. doi:10.1016/j.molcel.2010.07.026
Metzger, M. B., Hristova, V. A., and Weissman, A. M. (2012). HECT and RING finger families of E3 ubiquitin ligases at a glance. J. Cell Sci. 125, 531–537. doi:10.1242/jcs.091777
Mills, E. W., and Green, R. (2017). Ribosomopathies: there’s strength in numbers. Science 358, eaan2755. doi:10.1126/science.aan2755
Moldovan, G. L., Pfander, B., and Jentsch, S. (2006). PCNA controls establishment of sister chromatid cohesion during S phase. Mol. Cell 23, 723–732. doi:10.1016/j.molcel.2006.07.007
Mondal, T., Juvvuna, P. K., Kirkeby, A., Mitra, S., Kosalai, S. T., Traxler, L., et al. (2018). Sense-antisense lncRNA pair encoded by locus 6p22.3 determines neuroblastoma susceptibility via the USP36-CHD7-SOX9 regulatory Axis. Cancer Cell 33, 417–434.e7. doi:10.1016/j.ccell.2018.01.020
Morita, Y., Kanei-Ishii, C., Nomura, T., and Ishii, S. (2005). TRAF7 sequesters c-Myb to the cytoplasm by stimulating its sumoylation. Mol. Biol. Cell 16, 5433–5444. doi:10.1091/mbc.e05-08-0731
Moss, T., and Stefanovsky, V. Y. (2002). At the center of eukaryotic life. Cell 109, 545–548. doi:10.1016/s0092-8674(02)00761-4
Muller, S., Hoege, C., Pyrowolakis, G., and Jentsch, S. (2001). SUMO, ubiquitin’s mysterious cousin. Nat. Rev. Mol. Cell Biol. 2, 202–210. doi:10.1038/35056591
Nakagawa, K., and Yokosawa, H. (2002). PIAS3 induces SUMO-1 modification and transcriptional repression of IRF-1. FEBS Lett. 530, 204–208. doi:10.1016/s0014-5793(02)03486-5
Neo, S. H., Itahana, Y., Alagu, J., Kitagawa, M., Guo, A. K., Lee, S. H., et al. (2015). TRIM28 is an E3 ligase for ARF-mediated NPM1/B23 SUMOylation that represses centrosome amplification. Mol. Cell. Biol. 35, 2851–2863. doi:10.1128/mcb.01064-14
Neyret-Kahn, H., Benhamed, M., Ye, T., Le Gras, S., Cossec, J. C., Lapaquette, P., et al. (2013). Sumoylation at chromatin governs coordinated repression of a transcriptional program essential for cell growth and proliferation. Genome Res. 23, 1563–1579. doi:10.1101/gr.154872.113
Nishida, T., and Yasuda, H. (2002). PIAS1 and PIASxα function as SUMO-E3 ligases toward androgen receptor and repress androgen receptor-dependent transcription. J. Biol. Chem. 277, 41311–41317. doi:10.1074/jbc.m206741200
Nuro-Gyina, P. K., and Parvin, J. D. (2015). Roles for SUMO in pre-mRNA processing. WIREs RNA 7, 105–112. doi:10.1002/wrna.1318
Oh, S. M., Liu, Z., Okada, M., Jang, S. W., Liu, X., Chan, C. B., et al. (2010). Ebp1 sumoylation, regulated by TLS/FUS E3 ligase, is required for its anti-proliferative activity. Oncogene 29, 1017–1030. doi:10.1038/onc.2009.411
Oh, Y., and Chung, K. C. (2013). UHRF2, a ubiquitin E3 ligase, acts as a small ubiquitin-like modifier E3 ligase for zinc finger protein 131. J. Biol. Chem. 288, 9102–9111. doi:10.1074/jbc.m112.438234
Ouyang, J., Garner, E., Hallet, A., Nguyen, H., Rickman, K., Gill, G., et al. (2015). Noncovalent interactions with SUMO and ubiquitin orchestrate distinct functions of the SLX4 complex in genome maintenance. Mol. Cell 57, 108–122. doi:10.1016/j.molcel.2014.11.015
Panse, V. G., Kressler, D., Pauli, A., Petfalski, E., Gnädig, M., Tollervey, D., et al. (2006). Formation and nuclear export of preribosomes are functionally linked to the small-ubiquitin-related modifier pathway. Traffic 7, 1311–1321. doi:10.1111/j.1600-0854.2006.00471.x
Pelisch, F., Gerez, J., Druker, J., Schor, I. E., Muñoz, M. J., Risso, G., et al. (2010). The serine/arginine-rich protein SF2/ASF regulates protein sumoylation. Proc. Natl. Acad. Sci. U. S. A. 107, 16119–16124. doi:10.1073/pnas.1004653107
Pelletier, J., Thomas, G., and Volarevic, S. (2018). Ribosome biogenesis in cancer: new players and therapeutic avenues. Nat. Rev. Cancer 18, 51–63. doi:10.1038/nrc.2017.104
Peng, Y., Wang, Z., Wang, Z., Yu, F., Li, J., and Wong, J. (2019). SUMOylation down-regulates rDNA transcription by repressing expression of upstream-binding factor and proto-oncogene c-Myc. J. Biol. Chem. 294, 19155–19166. doi:10.1074/jbc.ra119.010624
Pichler, A., Gast, A., Seeler, J. S., Dejean, A., and Melchior, F. (2002). The nucleoporin RanBP2 has SUMO1 E3 ligase activity. Cell 108, 109–120. doi:10.1016/s0092-8674(01)00633-x
Potts, P. R., and Yu, H. (2005). Human MMS21/NSE2 is a SUMO ligase required for DNA repair. Mol. Cell. Biol. 25, 7021–7032. doi:10.1128/mcb.25.16.7021-7032.2005
Potts, P. R., and Yu, H. (2007). The SMC5/6 complex maintains telomere length in ALT cancer cells through SUMOylation of telomere-binding proteins. Nat. Struct. Mol. Biol. 14, 581–590. doi:10.1038/nsmb1259
Psakhye, I., and Jentsch, S. (2012). Protein group modification and synergy in the SUMO pathway as exemplified in DNA repair. Cell 151, 807–820. doi:10.1016/j.cell.2012.10.021
Pungaliya, P., Kulkarni, D., Park, H. J., Marshall, H., Zheng, H., Lackland, H., et al. (2007). TOPORS functions as a SUMO-1 E3 ligase for chromatin-modifying proteins. J. Proteome Res. 6, 3918–3923. doi:10.1021/pr0703674
Qin, K., Yu, S., Liu, Y., Guo, R., Guo, S., Fei, J., et al. (2023). USP36 stabilizes nucleolar Snail1 to promote ribosome biogenesis and cancer cell survival upon ribotoxic stress. Nat. Commun. 14, 6473. doi:10.1038/s41467-023-42257-8
Qiu, W., Cai, X., Xu, K., Song, S., Xiao, Z., Hou, Y., et al. (2021). PRL1 promotes glioblastoma invasion and tumorigenesis via activating USP36-mediated Snail2 deubiquitination. Front. Oncol. 11, 795633. doi:10.3389/fonc.2021.795633
Quin, J. E., Devlin, J. R., Cameron, D., Hannan, K. M., Pearson, R. B., and Hannan, R. D. (2014). Targeting the nucleolus for cancer intervention. Biochimica Biophysica Acta (BBA) - Mol. Basis Dis. 1842, 802–816. doi:10.1016/j.bbadis.2013.12.009
Raiser, D. M., Narla, A., and Ebert, B. L. (2014). The emerging importance of ribosomal dysfunction in the pathogenesis of hematologic disorders. Leukemia Lymphoma 55, 491–500. doi:10.3109/10428194.2013.812786
Raman, N., Nayak, A., and Muller, S. (2013). The SUMO system: a master organizer of nuclear protein assemblies. Chromosoma 122, 475–485. doi:10.1007/s00412-013-0429-6
Raman, N., Weir, E., and Muller, S. (2016). The AAA ATPase MDN1 acts as a SUMO-targeted regulator in mammalian pre-ribosome remodeling. Mol. Cell 64, 607–615. doi:10.1016/j.molcel.2016.09.039
Reed, B. J., Locke, M. N., and Gardner, R. G. (2015). A conserved deubiquitinating enzyme uses intrinsically disordered regions to scaffold multiple protein interaction sites. J. Biol. Chem. 290, 20601–20612. doi:10.1074/jbc.m115.650952
Reverter, D., and Lima, C. D. (2005). Insights into E3 ligase activity revealed by a SUMO-RanGAP1-Ubc9-Nup358 complex. Nature 435, 687–692. doi:10.1038/nature03588
Rodnina, M. V., and Wintermeyer, W. (2009). Recent mechanistic insights into eukaryotic ribosomes. Curr. Opin. Cell Biol. 21, 435–443. doi:10.1016/j.ceb.2009.01.023
Rodriguez, M. S., Dargemont, C., and Hay, R. T. (2001). SUMO-1 conjugation in vivo requires both a consensus modification motif and nuclear targeting. J. Biol. Chem. 276, 12654–12659. doi:10.1074/jbc.m009476200
Ryu, H., Sun, X., Chen, Y., Li, Y., Wang, X., Dai, R. S., et al. (2021). The deubiquitinase USP36 promotes snoRNP group SUMOylation and is essential for ribosome biogenesis. EMBO Rep. 22, e50684. doi:10.15252/embr.202050684
Ryu, H. Y., López-Giráldez, F., Knight, J., Hwang, S. S., Renner, C., Kreft, S. G., et al. (2018). Distinct adaptive mechanisms drive recovery from aneuploidy caused by loss of the Ulp2 SUMO protease. Nat. Commun. 9, 5417. doi:10.1038/s41467-018-07836-0
Sachdev, S., Bruhn, L., Sieber, H., Pichler, A., Melchior, F., and Grosschedl, R. (2001). PIASy, a nuclear matrix-associated SUMO E3 ligase, represses LEF1 activity by sequestration into nuclear bodies. Genes Dev. 15, 3088–3103. doi:10.1101/gad.944801
Sampson, D. A., Wang, M., and Matunis, M. J. (2001). The small ubiquitin-like modifier-1 (SUMO-1) consensus sequence mediates Ubc9 binding and is essential for SUMO-1 modification. J. Biol. Chem. 276, 21664–21669. doi:10.1074/jbc.m100006200
Sarangi, P., and Zhao, X. (2015). SUMO-mediated regulation of DNA damage repair and responses. Trends Biochem. Sci. 40, 233–242. doi:10.1016/j.tibs.2015.02.006
Savkur, R. S., and Olson, M. O. (1998). Preferential cleavage in pre-ribosomal RNA byprotein B23 endoribonuclease. Nucleic Acids Res. 26, 4508–4515. doi:10.1093/nar/26.19.4508
Schmidt, D., and Muller, S. (2002). Members of the PIAS family act as SUMO ligases for c-Jun and p53 and repress p53 activity. Proc. Natl. Acad. Sci. U. S. A. 99, 2872–2877. doi:10.1073/pnas.052559499
Shipkovenska, G., Durango, A., Kalocsay, M., Gygi, S. P., and Moazed, D. (2020). A conserved RNA degradation complex required for spreading and epigenetic inheritance of heterochromatin. Elife 9, e54341. doi:10.7554/elife.54341
Singh, S., Vanden Broeck, A., Miller, L., Chaker-Margot, M., and Klinge, S. (2021). Nucleolar maturation of the human small subunit processome. Science 373, eabj5338. doi:10.1126/science.abj5338
Song, J., Durrin, L. K., Wilkinson, T. A., Krontiris, T. G., and Chen, Y. (2004). Identification of a SUMO-binding motif that recognizes SUMO-modified proteins. Proc. Natl. Acad. Sci. U. S. A. 101, 14373–14378. doi:10.1073/pnas.0403498101
Song, J., Zhang, Z., Hu, W., and Chen, Y. (2005). Small ubiquitin-like modifier (SUMO) recognition of a SUMO binding motif: a reversal of the bound orientation. J. Biol. Chem. 280, 40122–40129. doi:10.1074/jbc.m507059200
Sulima, S. O., Hofman, I. J. F., De Keersmaecker, K., and Dinman, J. D. (2017). How ribosomes translate cancer. Cancer Discov. 7, 1069–1087. doi:10.1158/2159-8290.cd-17-0550
Sun, W., Shen, J., Liu, J., Han, K., Liang, L., and Gao, Y. (2022). Gene signature and prognostic value of ubiquitin-specific proteases members in hepatocellular carcinoma and explored the immunological role of USP36. Front. Biosci. Landmark Ed. 27, 190. doi:10.31083/j.fbl2706190
Sun, X. X., He, X., Yin, L., Komada, M., Sears, R. C., and Dai, M. S. (2015a). The nucleolar ubiquitin-specific protease USP36 deubiquitinates and stabilizes c-Myc. Proc. Natl. Acad. Sci. U. S. A. 112, 3734–3739. doi:10.1073/pnas.1411713112
Sun, X. X., Sears, R. C., and Dai, M. S. (2015b). Deubiquitinating c-Myc: USP36 steps up in the nucleolus. Cell Cycle 14, 3786–3793. doi:10.1080/15384101.2015.1093713
Tago, K., Chiocca, S., and Sherr, C. J. (2005). Sumoylation induced by the Arf tumor suppressor: a p53-independent function. Proc. Natl. Acad. Sci. U. S. A. 102, 7689–7694. doi:10.1073/pnas.0502978102
Taillebourg, E., Gregoire, I., Viargues, P., Jacomin, A. C., Thevenon, D., Faure, M., et al. (2012). The deubiquitinating enzyme USP36 controls selective autophagy activation by ubiquitinated proteins. Autophagy 8, 767–779. doi:10.4161/auto.19381
Takahashi, Y., Kahyo, T., Toh-e, A., Yasuda, H., and Kikuchi, Y. (2001). Yeast Ull1/Siz1 is a novel SUMO1/Smt3 ligase for septin components and functions as an adaptor between conjugating enzyme and substrates. J. Biol. Chem. 276, 48973–48977. doi:10.1074/jbc.m109295200
Teng, T., Thomas, G., and Mercer, C. A. (2013). Growth control and ribosomopathies. Curr. Opin. Genet. Dev. 23, 63–71. doi:10.1016/j.gde.2013.02.001
Thevenon, D., Engel, E., Avet-Rochex, A., Gottar, M., Bergeret, E., Tricoire, H., et al. (2009). The Drosophila ubiquitin-specific protease dUSP36/Scny targets IMD to prevent constitutive immune signaling. Cell host microbe 6, 309–320. doi:10.1016/j.chom.2009.09.007
Thevenon, D., Seffouh, I., Pillet, C., Crespo-Yanez, X., Fauvarque, M. O., and Taillebourg, E. (2020). A nucleolar isoform of the Drosophila ubiquitin specific protease dUSP36 regulates MYC-dependent cell growth. Front. Cell Dev. Biol. 8, 506. doi:10.3389/fcell.2020.00506
Torres-Rosell, J., Sunjevaric, I., De Piccoli, G., Sacher, M., Eckert-Boulet, N., Reid, R., et al. (2007). The Smc5-Smc6 complex and SUMO modification of Rad52 regulates recombinational repair at the ribosomal gene locus. Nat. Cell Biol. 9, 923–931. doi:10.1038/ncb1619
Treiber, T., Treiber, N., Plessmann, U., Harlander, S., Daiß, J. L., Eichner, N., et al. (2017). A compendium of RNA-binding proteins that regulate MicroRNA biogenesis. Mol. Cell 66, 270–284. e213. doi:10.1016/j.molcel.2017.03.014
Vanden Broeck, A., and Klinge, S. (2023). Principles of human pre-60S biogenesis. Science 381, eadh3892. doi:10.1126/science.adh3892
van Riggelen, J., Yetil, A., and Felsher, D. W. (2010). MYC as a regulator of ribosome biogenesis and protein synthesis. Nat. Rev. Cancer 10, 301–309. doi:10.1038/nrc2819
Varejao, N., Lascorz, J., Li, Y., and Reverter, D. (2020). Molecular mechanisms in SUMO conjugation. Biochem. Soc. Trans. 48, 123–135. doi:10.1042/bst20190357
Vertegaal, A. C., Ogg, S. C., Jaffray, E., Rodriguez, M. S., Hay, R. T., Andersen, J. S., et al. (2004). A proteomic study of SUMO-2 target proteins. J. Biol. Chem. 279, 33791–33798. doi:10.1074/jbc.m404201200
Wang, D., Li, Z., Li, X., Yan, C., Yang, H., Zhuang, T., et al. (2022). DUB1 suppresses Hippo signaling by modulating TAZ protein expression in gastric cancer. J. Exp. Clin. Cancer Res. 41, 219. doi:10.1186/s13046-022-02410-5
Watkins, N. J., and Bohnsack, M. T. (2012). The box C/D and H/ACA snoRNPs: key players in the modification, processing and the dynamic folding of ribosomal RNA. WIREs RNA 3, 397–414. doi:10.1002/wrna.117
Weger, S., Hammer, E., and Heilbronn, R. (2005). Topors acts as a SUMO-1 E3 ligase for p53 in vitro and in vivo. FEBS Lett. 579, 5007–5012. doi:10.1016/j.febslet.2005.07.088
Westman, B. J., Verheggen, C., Hutten, S., Lam, Y. W., Bertrand, E., and Lamond, A. I. (2010). A proteomic screen for nucleolar SUMO targets shows SUMOylation modulates the function of Nop5/Nop58. Mol. Cell 39, 618–631. doi:10.1016/j.molcel.2010.07.025
Wu, H., Jiao, Y., Zhou, C., Guo, X., Wu, Z., and Lv, Q. (2023). miR-140-3p/usp36 axis mediates ubiquitination to regulate PKM2 and suppressed the malignant biological behavior of breast cancer through Warburg effect. Cell Cycle 22, 680–692. doi:10.1080/15384101.2022.2139554
Yamashita, D., Moriuchi, T., Osumi, T., and Hirose, F. (2016). Transcription factor hDREF is a novel SUMO E3 ligase of Mi2α. J. Biol. Chem. 291, 11619–11634. doi:10.1074/jbc.m115.713370
Yan, Y., Xu, Z., Huang, J., Guo, G., Gao, M., Kim, W., et al. (2020). The deubiquitinase USP36 Regulates DNA replication stress and confers therapeutic resistance through PrimPol stabilization. Nucleic Acids Res. 48, 12711–12726. doi:10.1093/nar/gkaa1090
Yang, Y., Tse, A. K. W., Li, P., Ma, Q., Xiang, S., Nicosia, S. V., et al. (2011). Inhibition of androgen receptor activity by histone deacetylase 4 through receptor SUMOylation. Oncogene 30, 2207–2218. doi:10.1038/onc.2010.600
Yun, C., Wang, Y., Mukhopadhyay, D., Backlund, P., Kolli, N., Yergey, A., et al. (2008). Nucleolar protein B23/nucleophosmin regulates the vertebrate SUMO pathway through SENP3 and SENP5 proteases. J. Cell Biol. 183, 589–595. doi:10.1083/jcb.200807185
Zhang, D., Liang, Y., Xie, Q., Gao, G., Wei, J., Huang, H., et al. (2015). A novel post-translational modification of nucleolin, SUMOylation at lys-294, mediates arsenite-induced cell death by regulating gadd45α mRNA stability. J. Biol. Chem. 290, 4784–4800. doi:10.1074/jbc.m114.598219
Zhang, J., Ren, P., Xu, D., Liu, X., Liu, Z., Zhang, C., et al. (2019). Human UTP14a promotes colorectal cancer progression by forming a positive regulation loop with c-Myc. Cancer Lett. 440-441, 106–115. doi:10.1016/j.canlet.2018.10.010
Zhang, W., Luo, J., Xiao, Z., Zang, Y., Li, X., Zhou, Y., et al. (2022). USP36 facilitates esophageal squamous carcinoma progression via stabilizing YAP. Cell Death Dis. 13, 1021. doi:10.1038/s41419-022-05474-5
Zheng, M., Ai, Z., Guo, Y., Chen, Y., Xie, P., and An, W. (2023). Imbalance in ALR ubiquitination accelerates the progression of nonalcoholic steatohepatitis to hepatocellular carcinoma. Oncogene 42, 308–321. doi:10.1038/s41388-022-02549-7
Zhou, H., Feng, W., Yu, J., Shafiq, T. A., Paulo, J. A., Zhang, J., et al. (2023). SENP3 and USP7 regulate Polycomb-rixosome interactions and silencing functions. Cell Rep. 42, 112339. doi:10.1016/j.celrep.2023.112339
Zhou, H., Stein, C. B., Shafiq, T. A., Shipkovenska, G., Kalocsay, M., Paulo, J. A., et al. (2022). Rixosomal RNA degradation contributes to silencing of Polycomb target genes. Nature 604, 167–174. doi:10.1038/s41586-022-04598-0
Zhu, S., Hou, S., Lu, Y., Sheng, W., Cui, Z., Dong, T., et al. (2021). USP36-Mediated deubiquitination of DOCK4 contributes to the diabetic renal tubular epithelial cell injury via wnt/β-catenin signaling pathway. Front. Cell Dev. Biol. 9, 638477. doi:10.3389/fcell.2021.638477
Keywords: USP36, ribosome biogenesis, SUMOylation, ubiquitin, deubiquitination
Citation: Yang Y, Li Y, Sears RC, Sun X-X and Dai M-S (2024) SUMOylation regulation of ribosome biogenesis: Emerging roles for USP36. Front. RNA Res. 2:1389104. doi: 10.3389/frnar.2024.1389104
Received: 20 February 2024; Accepted: 15 March 2024;
Published: 03 April 2024.
Edited by:
A. Gregory Matera, University of North Carolina at Chapel Hill, United StatesReviewed by:
Sander Granneman, University of Edinburgh, United KingdomCopyright © 2024 Yang, Li, Sears, Sun and Dai. This is an open-access article distributed under the terms of the Creative Commons Attribution License (CC BY). The use, distribution or reproduction in other forums is permitted, provided the original author(s) and the copyright owner(s) are credited and that the original publication in this journal is cited, in accordance with accepted academic practice. No use, distribution or reproduction is permitted which does not comply with these terms.
*Correspondence: Mu-Shui Dai, ZGFpbUBvaHN1LmVkdQ==; Xiao-Xin Sun, c3VueEBvaHN1LmVkdQ==