- 1College of Earth, Ocean and Atmospheric Sciences, Oregon State University, Corvallis, OR, United States
- 2School of Marine Sciences, University of Maine, Orono, ME, United States
- 3Sea-Bird Scientific, Bellevue, WA, United States
- 4Scripps Institution of Oceanography, University of California San Diego, La Jolla, CA, United States
Ocean color satellites require a procedure known as System Vicarious Calibration (SVC) after launch as the pre-launch and on-orbit calibration accuracy is insufficient. The current approach for determination of post-launch SVC uses a single fixed measurement location and may be susceptible to unexpected biases in satellite processing algorithms. Here we describe a novel SVC program which is based on a high resolution and high accuracy radiometric system integrated with an autonomous profiling float (providing a buoyancy engine, physical observations, and communication). This float + radiometer (HyperNav) system can be shipped via air, land, ocean and is deployable from small boats. This SVC program relies on multiple deployment sites with associated facilities to collect a significant amount of SVC quality data in a relatively short time. It has centralized logistics and command-and-control centers ensuring easy access to information regarding the status of each asset and to ensure floats stay within a certain ocean area. The development of the program has been associated with the launch of NASA’s PACE satellite and has been executed by academic institutions in collaboration with an industrial partner. Other approaches for a future float-based operational SVC program are discussed.
1 Introduction
Ocean color sensors on satellites require a System Vicarious Calibration (SVC) procedure after launch as the pre-launch ground and on-board calibration uncertainties are insufficient to meet ocean color product requirements (Gordon, 1987; IOCCG, 2012). The SVC process has been used post-launch to evaluate and adjust the calibration factor of visible bands of the ocean color satellite sensor. The SVC method utilizes the in-situ water leaving radiance measurements propagated to the top of the atmosphere (TOA) using the same atmospheric correction functions as in operational processing, to provide estimated TOA radiance (Franz et al., 2007). The ratio between the estimated and measured TOA radiance is the vicarious gain (if no correction is necessary this value would be 1). Currently, the SVC process relies on obtaining a sufficient number of matchups (i.e., satellite TOA measurements and in-situ water leaving radiance measurements) to determine the average value of the calibration gain factors and their uncertainty. This process results in a lookup table of band-specific gains that are applied to post-launch ocean color observations. Thus, inherently, the SVC process relies on both the quality of in-situ water leaving radiance data, as well as the quality of atmospheric correction models used in ocean color processing. Since the launch of NASA’s SeaWiFS in 1997, the strategy for SVC of satellite ocean color radiometers has relied primarily on the deployment of an in-situ buoy instrumented with precision radiometers near the island of Lana’i in Hawaii (named Marine Optical BuoY, or MOBY). Historically, it has taken approximately 2–3 years following a satellite launch to determine required SVC gains by obtaining sufficient high-quality match-ups at the MOBY site (i.e., high accuracy in-situ radiometric measurements in ocean/atmosphere conditions that meet the SVC criteria, Franz et al. (2007)). Forty high-quality match-ups (concurrent satellite observation and in water observations) that meet acceptable atmosphere and ocean conditions were deemed sufficient to obtain sufficiently small uncertainties in the calibration gain factors of visible bands (Franz et al., 2007). This is because the uncertainty of a gain value scales inversely with the square root of the number of independent match-ups. This process is not designed to correct for drift in the sensor over its lifetime for which other procedures, such as lunar calibrations or on-board solar diffusers, are used. Suitable SVC infrastructure must ensure the lowest uncertainties on the measured radiometry that allow meeting the ocean color mission requirements. The coverage of the ocean color spectral domain and a hyperspectral capability must support SVC needs of the applicable missions.
Suitable SVC sites must satisfy specific and rather strict criteria (Gordon, 1987; IOCCG, 2012; Zibordi and Mélin, 2017). Firstly, the aerosols should be mostly of maritime origin (i.e., non-absorbing) with aerosol optical thickness (AOT) below 0.1 in the visible (i.e., very clear atmosphere), and the surface should be free of whitecaps (i.e., local surface wind speeds
Multiple sites meeting the SVC specifications can be preferable, providing the highest standard and equivalence in radiometric performance IOCCG (2012). Multiple sites may allow to understand and reduce residual uncertainties in atmospheric correction modelling, if any. A possibility of a seasonal bias in the visible band radiometry and particulate backscattering retrievals in the ocean color sensors processed using the same atmospheric correction code was found in comparisons with MOBY, lidar and profiling floats Bisson et al. (2021b,a). Performing SVC measurements at multiple sites across the globe may thus be a prudent approach.
From the above it follows that to improve on the current approach for SVC, future ocean color SVC programs should include: 1) an increase the number of high quality in-situ measurements available for SVC after satellite launch, 2) an increase the number of sites for SVC, and 3) such sites should have a high probability for ocean color satellite match-ups (i.e., meet the stringent criteria for SVC purposes). These improvements will reduce the time to derive stable and accurate SVC gain adjustments for a new ocean color satellite, better constrain potential uncertainties in the atmospheric correction, and reduce the time for scientific use of satellite imagery after launch.
The number of current and future satellite ocean color missions has significantly increased in recent years1, with each of these missions having different visible spectral bands. In particular, various organizations, such as NASA and European Commission Copernicus Programme, are planning new ocean color satellite missions to observe the Earth’s oceans at unprecedented spectral resolution with the goal to improve understanding of the surface biology and biogeochemistry (e.g., phytoplankton functional groups; Werdell et al. (2019)). Thus, future generation of hyperspectral ocean color satellite will require a concordant advancement in in-situ hyperspectral radiometric measurements for SVC purposes.
In this paper we describe a novel SVC system, HyperNav, that provides a pathway to meet the current and future needs of ocean color satellite sensors. It is based on high-precision high-accuracy hyperspectral upwelling radiance radiometers (two per system) and utilizes an autonomous profiling float which is portable and can be deployed and retrieved by relatively small coastal vessels. The system includes a command and control platform, termed the HyperNav Portal, to aid in navigation of the float to remain in a bounded region near the deployment site, provide time series of in-situ high-accuracy radiometric measurements, and provide open access to collected data from deployed HyperNav float systems. Furthermore, the program is scalable, whereby several HyperNav float systems can be deployed to collect measurements at multiple sites simultaneously, thereby increasing the number of high-quality, low-uncertainty in-situ SVC-usable observations. Together with in-situ mooring-based SVC measurement approaches such as MOBY (Brown et al., 2007), these systems can provide complementary information, support retrieval and uncertainty analysis of SVC gain factors using independent approaches (i.e., different in hardware and location), and rapid delivery of the gains with multiple floats operating at different sites.
2 Components of a float-based SVC system
The idea of using radiometers on profiling floats is not new. In the early 2000s floats equipped with radiometers were used to study the onset of the spring bloom in the sea of Japan (Mitchell, 2003) and have since become an integral part of BGC-Argo (Johnson and Claustre, 2016). The idea of using autonomous profiling floats as a component of an SVC program for in-situ radiometric measurements has also been previously explored (Gerbi et al., 2016; Leymarie et al., 2018), including the advantages of putting two upwelling radiometers per float on extended arms (Leymarie et al., 2018) for redundancy and to ensure that there is always a radiometer that is not shaded by the float itself. Redundancy and minimizing shading both contribute to reducing the uncertainty in the in-situ radiometric measurements for SVC. Below we expand on the HyperNav end-to-end system that has been designed and tested which we suggest should be part of an ocean color satellite SVC system.
2.1 The float and HyperNav system
The HyperNav float-based profiling system consists of a buoyancy-driven autonomous profiling float with standard Argo sensors (e.g., an Argo-program-like profiling float, Roemmich et al. (2019)) integrated with a high spectral resolution and accuracy radiometric system (HyperNav radiometric sensor, Sea-Bird Scientific (SBS)) equipped with additional sensors to measure pressure and platform orientation and other optical properties. The profiling float we currently use is a SBS Navis float, modified to power the HyperNav system, receive and transmit HyperNav data via Iridium satellite communication to shore, and change mission configurations of the HyperNAV system (Figure 1). The Navis float is equipped with a standard Argo CTD and the Navis firmware has been modified to enable power, sampling, and data transmission for the HyperNav radiometric system.
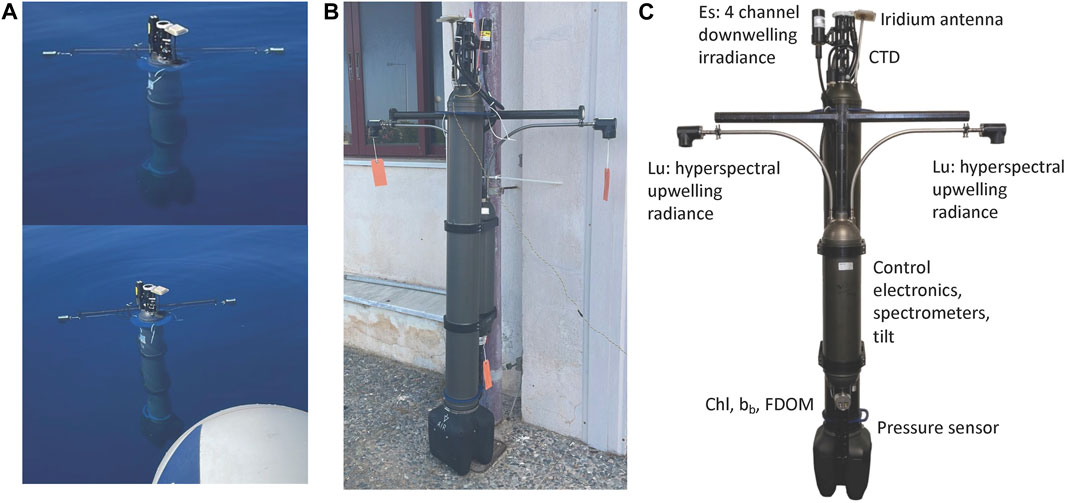
Figure 1. The HyperNav float-based system. (A) Photos of the system from a test deployment North of Crete. (B) Photo of the system taken onshore before deployment during system testing. The Navis float is shown in the forefront. (C) Photo of HyperNav showing the locations of the sensors.
The integrated HyperNav radiometric system includes dual independent upwelling radiance sensors, integrated pressure and tilt sensor. The sensors, the system, and how they meet the SVC measurment requirements are described in a parallel paper Barnard et al. (2024). The HyperNav sensor system that is integrated with the Navis float includes an SBS OCR-504 four-wavelength downwelling radiometer (providing above water downwelling irradiance) which is mounted to the Navis float CTD stalk. The purpose of the above-water downwelling radiometer is to help ensure conditions for SVC are optimal in terms of atmospheric transmission and account for varying sky conditions during the HyperNav data acquisition period. The HyperNav sensor system also includes integrated pressure and tilt sensors, both of which are used to evaluate the upwelling radiance measurements for SVC observations. The development of the HyperNav sensor system includes a SBS MCOMS triplet sensor (measuring backscattering at 700 nm, chlorophyll and CDOM fluoresence). The purpose of the MCOMS is to provide verification that the upper part of the ocean is not optically stratified, a key requirement of SVC qualified in-situ measurements. While MCOMS are included on all HyperNav sensor systems, full data integration and sample sequencing is still under developed, and is intended to be enabled in the near future.
2.2 Float command and control center
As part of the currently funded HyperNav project, an online command and control operations and data delivery site, termed the HyperNav Portal, was created (http://misclab.umeoce.maine.edu/HyperNav/) where key information is available (Figure 2):
Such information is available for current as well historical deployments.
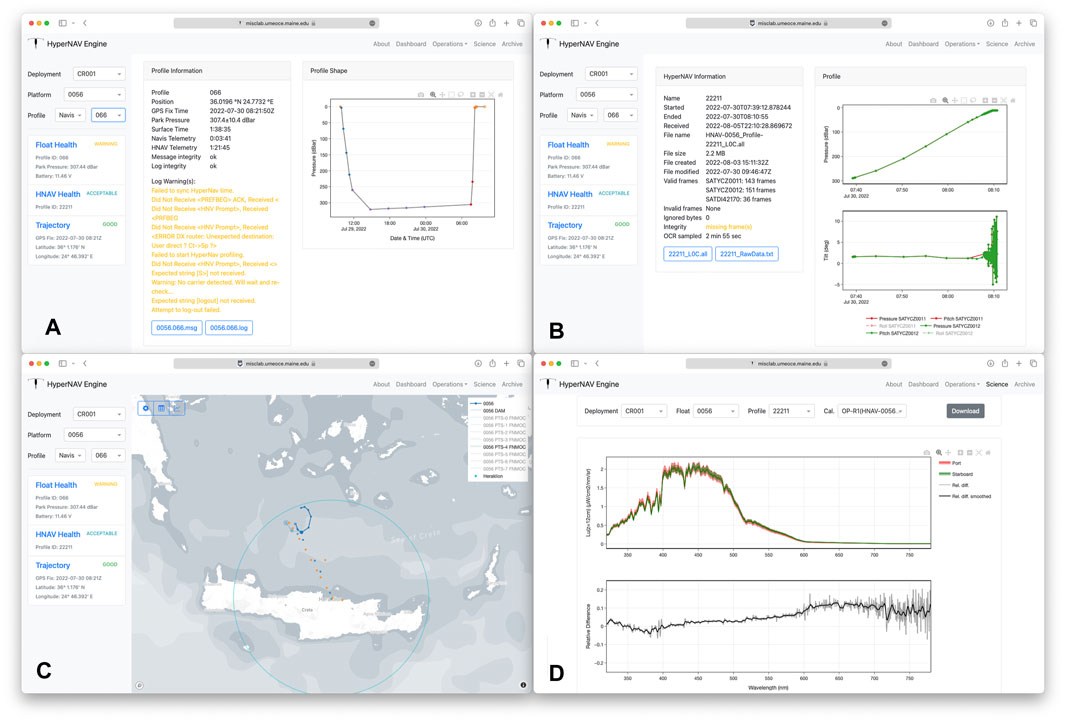
Figure 2. Four different screens from the HyperNav Portal site. (A) Dive pattern of a given profile. (B) HyperNav data acquisition profile. (C) Float trajectory for 5 day period North of Crete. (D) Water leaving radiance spectra and its uncertainty computed for a given profile. All can be accessed from the HyperNav Portal https://misclab.umeoce.maine.edu/HyperNav/.
This information allows the operations team to assess the health of each float, the quality of the radiometric data being collected (e.g., to decide whether it needs to be picked up), consult with weather patterns for decision-making of future float surfacing, and consult with circulation models output to navigate and steer the float to remain within the predefined sampling and recovery area. The command and control center also includes hardware such as a Router-Based Unrestricted Digital Internet-working Connectivity Solutions (RUDICS) server, a web server (and backup servers) for two way communication with the float and the on-shore server to enable public distribution/access of the data. All servers are virtual machine hosted in the cloud of the University of Maine and could be transferred to other cloud providers if need be.
2.3 Logistics center
The logistics center of the HyperNav Portal consists of a series of Google worksheets that aggregate all the information regarding the past, present and future status of floats and HyperNav systems and is accessible to all relevant partners. This information is used by the operational team to aid in deployment/recovery and future asset planning. Key attributes includes HyperNav calibration status (e.g., which sensors need to be post-calibrated after a mission), float and HyperNav asset tracking for deployments (i.e., asset availability to support next deployment locations), which is used to plan for future deployments and integration of future builds of float and HyperNav systems (e.g., new systems deliveries and scheduling). The logistics center facilitates coordination with local deployment groups as well as planning and coordination with shipping agents to deliver the systems to the next location in a timely manner.
2.4 Deployment sites
One key characteristic of the float-based SVC program is its ability to obtain data from multiple sites. Each deployment site is evaluated based on:
We expand on some of these below and much more in Chamberlain et al. (2024).
We developed partnerships with local scientists and boat operators to facilitate operations in each of the selected regions. All project team members and local personnel are trained in the necessary procedures to assemble a HyperNav system, perform operational system checks before deployment, enable deployment mission configuration, and ensure effective operations at time of deployment and through post deployment. This strategy of utilizing project teams and/or local partners (if available) for deployments, adds flexibility to the HyperNav program to maintain presence in longer-term deployment sites as well as facilitating “seasonal” and sites of opportunity for deployments. In the later case, deployment/logistics actions are performed by a project team that travels to the deployment site for deployment and/or recovery. As part of the deployment planning process, the logistic center includes working with local teams to ensure local knowledge and availability of boat operators in the deployment region that can support deployment/recovery operations on short notice in case a system recovery is needed. Since it will be very useful to have capabilities to replace a float within a system or batteries of a float locally (to avoid shipping the whole float back to the manufacturer), training local personnel in those tasks is performed. Issues of customs are also taken into account in site selection as those add delays as well as costs to the operation.
The selection of deployment sites is based on a comprehensive analysis of various factors to ensure suitability for SVC measurements (e.g., Figure 3). This analysis includes evaluating ocean and atmosphere properties, as discussed in studies by Franz et al. (2007) and Zibordi and Mélin (2017), assessing ocean current fields to guarantee that floats can remain within a predefined region for a 3-month deployment, and considering logistics and associated costs for each site. The process of the HyperNav system site selection, which includes flow analysis, is described in more detail in Chamberlain et al. (2024).
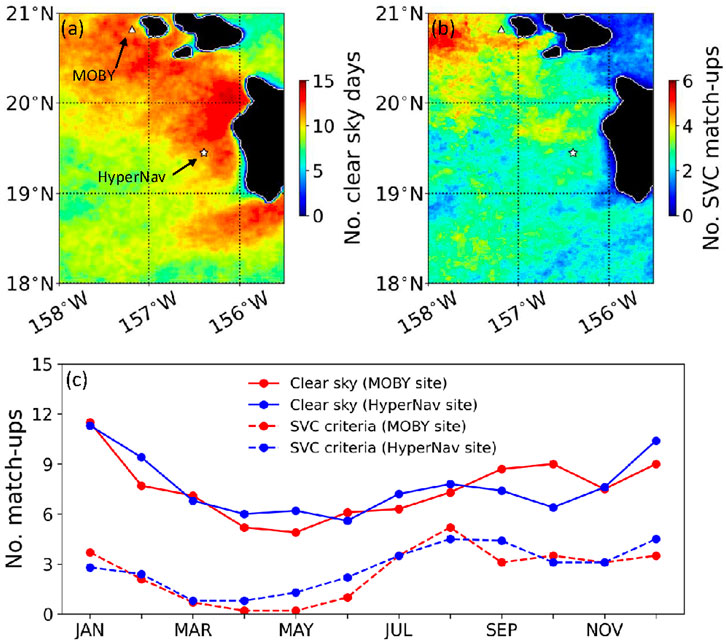
Figure 3. (A) Map of the average number of clear sky conditions in January in the seas around Hawaiian islands. White lines delineate the land/water boundaries. (B) Same as (A) but for the more stringent conditions where the sky is clear, aerosol optical thickness at 869 nm
Figure 3 depicts maps illustrating the number of clear sky and SVC days, indicating the potential number of match-ups in January in the Hawaiian region during MODIS-A overpass. The SVC criteria, although similar, differ somewhat from those outlined in previous studies, specifically in their stringency regarding aerosol type and optical thickness thresholds. In particular the influence of absorbing aerosols is minimized. At identified locations such as MOBY and the HyperNav site west of Kona, Hawaii, there are approximately 3 potential SVC match-ups per month from July to January, totaling about 25–30 SVC match-ups annually. In comparison, Franz et al. (2007) obtained roughly 15 SVC match-ups per year at the MOBY site, reflecting the challenges of maintaining continuous measurements throughout the year. Relaxing the SVC criteria would lead to a larger number of potential SVC match-ups, as indicated by the SVC and clear sky curves in Figure 3.
For the PACE mission post-launch support, four sites have been identified for initial operation: West of Kona, Hawaii, United States; North of the island of Crete, Greece; South of Puerto Rico, United States; and South of Moorea, French Polynesia. Over the past 2 years, multiple deployments have been conducted at each location to assess HyperNav performance, evaluate upwelled radiance measurement quality against SVC criteria, and determine the ability of HyperNav float trajectories to remain in the desired location. Additionally, recent deployments off Southern California have contributed to the assessment and refinement of site selection for seasonal and opportunistic future deployments, as well as testing HyperNav system performance with newly delivered systems.
2.5 Data analysis
As data arrives from the in-situ HyperNav system to the command and control center via Iridium communication, it is automatically processed in near-real time and subsequently posted to the HyperNav Portal (Haëntjens et al., this issue). This processing includes:
Following the recovery and post-calibration of the HyperNav is performed, the data is reprocessed, and a delayed-mode data set is generated for the spectrum of upwelled radiance and reflectance. Note that as with ocean color remote sensing, the reflectance computation is based on the measured spectral in-situ upwelled radiance and a model-based downwelling irradiance spectrum Tan et al. (2024). In addition, all data and associated metadata (e.g., sensors calibrations) is archived and shared on the HyperNav Portal. Computed products are archived in compliance with the NetCDF Climate and Forecast Metadata Conventions v1.10.
2.6 Liaison to space agency
NASA, the funding agency of HyperNav program, has been interested in both near-real-time and delayed-mode data. A person in the agency and at the SVC program are in regular contact to ensure the SVC system is fit for purpose, and that data flows automatically to the agency as soon as it is available in raw format as well as processed to SI units.
3 Models for operational float-based SVC systems
3.1 Governance structures
The float-based SVC program described here is currently funded as a research and development program. The long-term prospects depend not only on evolving such system to a sustainable operational state, but also on ensuring sustainable funding for such a program in the future. Below we discuss different models that may be chosen for this purpose:
Each of these models has advantages and disadvantages. Academic institutions are generally less expensive and nimble (e.g., if there is a need to swap sites) but lack the robustness of services that may be provided via a private company. There may be issues of conflict of interest when a funding agency also operates services, though it is a model that best ensures fitness for purpose.
Whichever governance system is chosen, it is critical to maximize its utility to the oceanographic community, that it follows the FAIR (findable, accessible, inter-operable, and reusable) data management principles Tanhua et al. (2019); Davidson et al. (2019). In addition, it is expected that data will be made available to users in near-real time for short-term decision making as well as in a delayed mode, following recovery and radiance sensors post-calibration.
3.2 External advisory committee
It is critical that the latest science and technological know-how informs the program operations while providing the funding agency with unbiased information regarding the return on investment. This goal can be achieved by having an external advisory committee which includes outside experts in the subject of ocean optics, radiometry, SVC and metrology, that meets annually to review the program and provide recommendation to the funder as well as the entity responsible for the operation of the SVC program.
4 Summary
In this paper we summarized the features associated with a float-based SVC program building on our experience with the program we have designed and tested to date. More details about the different elements of this program are detailed in accompanying papers Barnard et al. (2024); Chamberlain et al. (2024); Tan et al. (2024). We believe the program to have been very successful and it has been improving continuously. However, such an SVC program is not sustainable in its current form and in order to be operational over ocean color satellite lifetimes, funding agencies interested in its data will need to decide on its future funding and governance structure. We hope this paper has provided sufficient information on the needs, importance, and potential pathways forward to consider for maintaining and sustaining such a critical SVC program in the future.
Data availability statement
The raw data supporting the conclusions of this article will be made available by the authors, without undue reservation.
Author contributions
AB: Conceptualization, Funding acquisition, Investigation, Methodology, Project administration, Supervision, Validation, Writing–original draft, Writing–review and editing. EB: Conceptualization, Investigation, Methodology, Supervision, Validation, Writing–original draft, Writing–review and editing. NH: Formal Analysis, Methodology, Software, Validation, Writing–review and editing. CO: Project administration, Supervision, Writing–review and editing. PC: Conceptualization, Formal Analysis, Methodology, Writing–review and editing. RF: Formal Analysis, Investigation, Methodology, Writing–original draft, Writing–review and editing. MM: Conceptualization, Methodology, Supervision, Writing–review and editing. JT: Formal Analysis, Investigation, Methodology, Writing–review and editing.
Funding
The author(s) declare that financial support was received for the research, authorship, and/or publication of this article. Funding for the HyperNav system has been provided by NASA Goddard Space Flight Center, through the Ocean Biology and Biogeochemistry program, from contract numbers 80GSFC20C0101 and 180GSFC22CA050.
Acknowledgments
We thank Andrew Banks and Nektarios Spiyridakis for extraordinary support for operations in Crete. We thank Eric Rehm for useful comments on an early version of this manuscript.
Conflict of interest
Author CO was employed by company Se-Bird Scientific.
The remaining authors declare that the research was conducted in the absence of any commercial or financial relationships that could be construed as a potential conflict of interest.
Publisher’s note
All claims expressed in this article are solely those of the authors and do not necessarily represent those of their affiliated organizations, or those of the publisher, the editors and the reviewers. Any product that may be evaluated in this article, or claim that may be made by its manufacturer, is not guaranteed or endorsed by the publisher.
Footnotes
1https://ioccg.org/resources/missions-instruments/current-ocean-colour-sensors/
References
Barnard, A., Boss, E., Häentjens, N., Orrico, C., Frouin, R., Tan, J., et al. (2024). Design and verification of a highly accurate in-situ hyperspectral radiometric measurement system (hypernav). Front. Remote Sens. 5. doi:10.3389/frsen.2024.1369769
Bisson, K. M., Boss, E., Werdell, P. J., Ibrahim, A., and Behrenfeld, M. J. (2021a). Particulate backscattering in the global ocean: a comparison of independent assessments. Geophys. Resa Lett. 48, e2020GL090909. doi:10.1029/2020gl090909
Bisson, K. M., Boss, E., Werdell, P. J., Ibrahim, A., Frouin, R., and Behrenfeld, M. J. (2021b). Seasonal bias in global ocean color observations. Appl. Opt. 60, 6978–6988. doi:10.1364/AO.426137
Brown, S. W., Flora, S. J., Feinholz, M. E., Yarbrough, M. A., Houlihan, T., Peters, D., et al. (2007). “The marine optical buoy (MOBY) radiometric calibration and uncertainty budget for ocean color satellite sensor vicarious calibration,”. Sensors, systems, and next-generation satellites XI. Editors S. Habib, R. Meynart, S. P. Neeck, and H. Shimoda (SPIE: International Society for Optics and Photonics), 6744. doi:10.1117/12.73740067441M
Chamberlain, P., Frouin, R., Tan, J., Mazloff, M., Barnard, A., Boss, E., et al. (2024). Selecting hypernav deployment sites for calibrating and validating pace ocean color observations. Front. Remote Sens doi:10.3389/frsen.2024.1333851
Davidson, F., Alvera-Azcárate, A., Barth, A., Brassington, G. B., Chassignet, E. P., Clementi, E., et al. (2019). Synergies in operational oceanography: the intrinsic need for sustained ocean observations. Front. Mar. Sci. 6. doi:10.3389/fmars.2019.00450
Franz, B. A., Bailey, S. W., Werdell, P. J., and McClain, C. R. (2007). Sensor-independent approach to the vicarious calibration of satellite ocean color radiometry. Appl. Opt. 46, 5068–5082. doi:10.1364/AO.46.005068
Gerbi, G. P., Boss, E., Werdell, P. J., Proctor, C. W., Haëntjens, N., Lewis, M. R., et al. (2016). Validation of ocean color remote sensing reflectance using autonomous floats. J. Atmos. Ocean. Technol. 33, 2331–2352. doi:10.1175/JTECH-D-16-0067.1
Gordon, H. R. (1987). Calibration requirements and methodology for remote sensors viewing the ocean in the visible. Remote Sens. Environ. 22, 103–126. doi:10.1016/0034-4257(87)90029-0
Johnson, K. S., and Claustre, H. (2016). Bringing biogeochemistry into the argo age. EOS 97. doi:10.1029/2016EO062427
Leymarie, E., Penkerc’h, C., Vellucci, V., Lerebourg, C., Antoine, D., Boss, E., et al. (2018). Proval: a new autonomous profiling float for high quality radiometric measurements. Front. Mar. Sci. 5. doi:10.3389/fmars.2018.00437
Mitchell, B. G. (2003). “Resolving spring bloom dynamics in the sea of Japan,” in ALPS: Autonomous and Lagrangian Platforms and Sensors, Workshop Report. Editors D. L. Rudnick, and M. J. Perry (La Jolla, CA: ALPS), 26 –27.
Roemmich, D., Alford, M. H., Claustre, H., Johnson, K., King, B., Moum, J., et al. (2019). On the future of argo: a global, full-depth, multi-disciplinary array. Front. Mar. Sci. 6. doi:10.3389/fmars.2019.00439
Tan, J., Frouin, R., Häentjens, N., Barnard, A., Boss, E., Chamberlain, P., et al. (2024). Reconstructing hyper-spectral downwelling irradiance from multi-spectral measurements. Front. Remote Sens. 5. doi:10.3389/frsen.2024.1335627
Tanhua, T., Pouliquen, S., Hausman, J., O’Brien, K., Bricher, P., de Bruin, T., et al. (2019). Ocean fair data services. Front. Mar. Sci. 6. doi:10.3389/fmars.2019.00440
Werdell, P. J., Behrenfeld, M. J., Bontempi, P. S., Boss, E., Cairns, B., Davis, G. T., et al. (2019). The plankton, aerosol, cloud, ocean ecosystem mission: status, science, advances. Bull. Am. Meteorological Soc. 100, 1775–1794. doi:10.1175/BAMS-D-18-0056.1
Keywords: ocean color remote sensing, system vicarious calibration, ocean optics, radiometry, governance
Citation: Barnard A, Boss E, Haëntjens N, Orrico C, Chamberlain P, Frouin R, Mazloff M and Tan J (2024) A float-based Ocean color vicarious calibration program. Front. Remote Sens. 5:1373540. doi: 10.3389/frsen.2024.1373540
Received: 19 January 2024; Accepted: 15 July 2024;
Published: 05 August 2024.
Edited by:
Kevin Ruddick, Royal Belgian Institute of Natural Sciences, BelgiumReviewed by:
Xiuqing Hu, China Meteorological Administration, ChinaEwa Kwiatkowska, European Organisation for the Exploitation of Meteorological Satellites, Germany
Violeta Sanjuan Calzado, National Aeronautics and Space Administration (NASA), United States
Copyright © 2024 Barnard, Boss, Haëntjens, Orrico, Chamberlain, Frouin, Mazloff and Tan. This is an open-access article distributed under the terms of the Creative Commons Attribution License (CC BY). The use, distribution or reproduction in other forums is permitted, provided the original author(s) and the copyright owner(s) are credited and that the original publication in this journal is cited, in accordance with accepted academic practice. No use, distribution or reproduction is permitted which does not comply with these terms.
*Correspondence: Andrew Barnard, YmFybmFyYW5Ab3JlZ29uc3RhdGUuZWR1