- Cavendish Laboratory, Department of Physics, University of Cambridge, Cambridge, United Kingdom
Nitrogen-vacancy centres in diamond nanocrystals are among the leading candidates for realising nanoscale quantum sensing under ambient conditions. Due to their exceptional electronic spin coherence at room temperature and optical addressability, these solid state spin-based sensors can achieve a wide selection of sensing modalities, including probing temperature, external magnetic and electric field, as well as the detection of nearby electronic and nuclear spins. In this article, we discuss recent progress made utilizing nanodiamond quantum sensors in living systems and explore both opportunities for future advances and challenges that lie ahead.
1 Introduction to nanodiamond bio-sensing
Nitrogen vacancy (NV) centres in diamond nanocrystals provide an exciting platform for realising high sensitivity and high spatial resolution quantum sensing in living systems (Yu et al. (2005); McGuinness et al. (2011); Perona Martinez et al. (2020); Qureshi et al. (2022)). Their robust optical signature, along with coherent control and readout of the ground state spin levels through microwave and optical excitation have opened the door to realising quantum sensing in a range of different modalities with the same sensor device (Degen et al. (2017)). Several characteristics of this quantum probe have made it an attractive candidate for sensing biological phenomena. These include the small probing volume (on the order of 10s of zeptolitres (corresponding to the volume of a 50 nm diameter sphere) (Holzgrafe et al., 2020), low nanodiamond cytotoxicity even in high concentrations (Woodhams et al., 2018), their straightforward uptake into living cells through endocytosis (Figure 1A) (Liu et al., 2009; Chu et al., 2014), the possibility to decouple the signal of interest from background fluorescence fluctuations (Kuo et al., 2013), and practical in-situ calibration protocols for quantitative metrology (Yukawa et al., 2020).
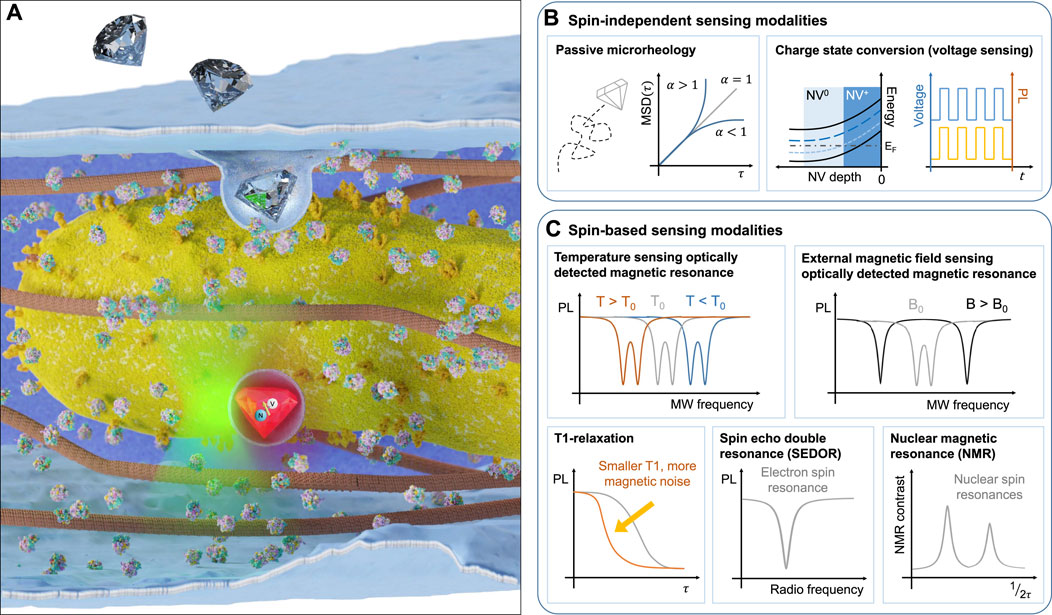
FIGURE 1. Utilising NV centres inside nanoscale diamond crystals for sensing under ambient conditions. (A) Illustration of nanodiamond uptake by a cell and subsequent proximity of the sensor to organelles (such as mitochondria). Examples of the typical readout for different nanodiamond sensor modalities including photoluminescence-based modalities (B) (passive microrheology, charge state conversion for voltage sensing) and spin-based modalities (C) (optically detected magnetic resonance for temperature and external magnetic field sensing, T1-relaxation, spin-echo double resonance and nuclear magnetic resonance).
Different sensing modalities can be realised using purely the photoluminescence signal of the NV centre (Figure 1B), while others rely on a combination of optical and microwave modulation (Figure 1C). For thermometry, magnetometry and nearby spin detection, the relevant sensor signal originates from detecting a shift of the ground state spin energy levels as a result of a change in the immediate nanoscale environment of the NV (Acosta et al., 2010). Readout of this change in energy can be achieved either by pulsed or continuous wave approaches. In the simplest protocol, the fluorescence signal from the NV is monitored when an external microwave resonantly excites the NV centre ground state from the ms = 0 state into the ms = ±1 state, which promotes relaxation through a transition with reduced fluorescence in the visible detection range. This method is termed optically detected magnetic resonance (ODMR) (Gruber et al., 1997). For achieving nuclear and electronic spin detection, more sophisticated schemes are required, that lock to the precession frequency of the target spins and provide selective detection of a chosen spin species (Mamin et al., 2013).
2 Recent advances
A number of sensing protocols have been developed that do not require coherent spin control, but instead rely on the NV centre’s optical and charge state properties. Due to their stable photoluminescence (Reineck et al., 2016), NV-containing nanodiamonds have been used as long-lived tracer probes in a variety of biological systems ranging from neurons (Haziza et al., 2017) to mice (Hui et al., 2014). Information about local rheological properties or active trafficking mechanisms can be extracted from the motion of nanodiamonds in cells using the mean squared displacement (MSD). Different regimes of motion can be identified by the power-law exponent of the MSD, α. When α < 1, the motion is known as sub-diffusive, indicating local trapping of the probe or movement in a viscoelastic medium. In the case where α > 1, the motion is super-diffusive, suggesting active directed motion, such as flow or intracellular trafficking (Waigh, 2016). Due to the absence of bleaching and the exceptional photostability of NV centres compared with commonly used fluorescent dyes (which typically have a photobleaching half-life on the order of 100s of seconds (Hirano et al., 2022), nanodiamond-based sensors can be tracked over the entire life cycle of a cell or organism, facilitating long term passive microrheology analysis using recently developed neural networks (Han et al., 2020). Typically, NV centre-based quantum sensing uses the negatively charged spin state (i.e., NV−), however, the transition to the neutral (NV0) and positively charged states (NV+) can occur and can be exploited for electric field sensing (Figure 1B, right). A strong local electrostatic field (which can be achieved through a negatively charged surface termination or adsorbate layer) can change the energy levels the NV centre relative to the diamond Fermi energy in such as way as to facilitate voltage-dependent charge state conversion (McCloskey et al., 2022). Implementation of this sensing modality has been demonstrated in nanodiamonds (Karaveli et al., 2016), but has yet to be applied in a biological setting where it could be used to sense physiological changes to the environment, such as local changes in pH (Sow et al., 2020).
The behaviour of intracellular temperature, long assumed to be homogeneous throughout the cell interior, has recently come under question following the observation of large local gradients within the mitochondrial intermembrane space (Chretien et al., 2018). This experimental result, along with others (Zhou et al., 2020; Chung et al., 2022), has come under scrutiny due to the conflict with theoretical models that suggest that such large temperature gradients should not persist over micron-scale distances in living systems due to the diffusion of heat (Baffou et al., 2014). Nanodiamonds, by virtue of their nanoscale size, are well suited for sensing temperature gradients on the nanoscale and addressing the open debate. The NV− centre temperature sensitivity originates from the expansion and contraction of the diamond lattice with changing temperature, which alters the energy separation between the ground state spin levels (Figure 1C top left). In addition, optical and charge state properties are also altered, allowing for a purely optical, ratiometric detection of temperature (Plakhotnik et al. (2015), although this has not been applied in vitro. A readout technique which provides robustness to background optical fluctuations is enabled by probing the ground state energy level splitting through combined optical and microwave excitation in a scheme called optically detected magnetic resonance (ODMR) McGuinness et al. (2011). The application of nanodiamonds for intracellular thermal sensing was first demonstrated by measuring the temperature changes induced by laser-heated gold nanoparticles (Kucsko et al., 2013). In the decade since, several experiments have expanded on the single-cell temperature sensing regime (Simpson et al., 2017), investigating how heat affects embryogenesis (Choi et al., 2020) and the thermogenic effect due to mitochondrial-uncoupling (Fujiwara et al., 2020) in the multicellular nematode Caenorhabditis elegans. Opportunities to measure thermogenic effects arising from mitochndrial uncoupling extend to a range of organisms, including plants (Vlot et al., 2009).
An external magnetic field will shift the spin energy levels of the NV centre ground state, resulting in a splitting between the two ODMR resonances that corresponds directly to the projection of the magnetic field to the quantisation axis of the NV spin (see illustration in Figure 1C top right). As the magnetic field changes induced in biological systems are often small and transient, magnetic field sensing of living systems has remained limited to NVs implanted close to the diamond surface in bulk diamond samples, where the orientation of the NV axis is known and fixed and higher sensitivities can be achieved. For example, the magnetic field change induced by the action potential of a single neuron in a Myxicola infundibulum worm was detected (Barry et al., 2016) using shallow NV ensemble magnetometry. For intracellular measurements, typical signals of interest can originate from magnetosomes generated by magnetotactic bacteria, imaged at the sub-cellular level with shallow NV quantum sensing (Le Sage et al., 2013).
An alternative and popular sensing modality that does not require microwave excitation is to measure the spin relaxation time (T1), the time taken for the polarised NV state to return to a thermal equilibrium polarisation. This timescale is affected by the local electromagnetic noise at the NV location and provides an indication of charge and spin concentration changes in the immediate environment of the NV centre (Figure 1C bottom left). The readout is performed optically by acquiring the change in fluorescence between spin initialisation and a finite time, typically a microsecond scale relaxation time (Mzyk et al., 2022). There are many reports of intracellular T1 sensing to detect reactive oxygen species and nitrogen oxide radicals (Nie et al., 2021; Sigaeva et al., 2022). Whilst minimally invasive, T1 measurements alone lack specificity, as the individual spin species influencing the polarisation relaxation can not be resolved. This can make the interpretation of results challenging. If the target spin species can be externally driven by a radio-frequency pulse, it becomes possible to isolate the noise effect through the coupling between the NV centre and the spin target (in a pulse delivery technique called spin echo double resonance, SEDOR, illustrated in Figure 1C bottom middle). Whilst this technique has been experimentally demonstrated in nanodiamonds to detect local nitrogen impurities within the diamond lattice (Knowles et al. (2014; 2016)), no biological application has yet been realised.
Nuclear magnetic resonance (NMR) spectroscopy detects the precession of nuclear spins in an external magnetic field. Realised with chemical shift resolution, where changes in spin-active nuclear isotope resonances due to the local electronic environment surrounding the nucleus are resolved, the technique can reveal structural information about a molecule or protein of interest. Conventional NMR however requires macroscopic samples (around 1 mm3) and an external magnetic field of several Tesla. NV centres can realise NMR spectroscopy by exploiting the coupling between the electronic NV spin and nearby nuclear spins (Figure 1C bottom right). Shallow ensemble experiments are even able to resolve chemical shifts (Aslam et al., 2017), and nanodiamond sensors containing single NV centres have demonstrated the capability to detect spin-active nuclei in ∼1500 oil molecules within a sensing volume of 7 zeptolitres (Holzgrafe et al., 2020).
3 Challenges and future opportunities
Nanodiamond quantum sensors offer a powerful platform for nanoscale sensing in vivo, conveniently combining a number of different modalities into one single nanoparticle device. While these have been able to give us many new insights into soft systems at the nanoscale, there remain a number of outstanding challenges. Heat damage caused by continuous microwave and optical excitation can be a significant source of perturbation for a living system. As nanodiamond tracking is often required for in vitro experiments, many modalities rely on continuous excitation. Microwave radiation can cause heating in cellular environments due to the prevalence of water, which has a broad absorption spectrum in the microwave range. Laser illumination for continuous spin initialisation and readout (commonly at 532 nm) can result in localised heat spots within the cell and can produce cytotoxic compounds such as reactive oxygen species (Lavi et al., 2010). Heating damage can be mitigated in a number of ways. Targeted, lower power microwave delivery can be achieved through the design of waveguide structures that are evaporated onto cell substrates and enable efficient excitation of the NV centre without heating the biological sample (Oshimi et al., 2022). In order to reduce the overall microwave power delivered to the surrounding medium, pulse sequences that allow time for the heat conduction away from the sample can be used. Pulsed illumination can be used to reduce the local photothermal load in a particular region of the cell, and could even increase sensitivity (Barry et al., 2020).
A further challenge is the mechanically dynamic nature of the samples of interest, which typically leads to nanodiamond motion. Nanodiamonds are actively transported around living cells by the collective motion of motor proteins and cytoskeleton remodelling, exhibiting velocities up to 200 nm/s (Simpson et al., 2014). This can make targeted sensing in specific locations difficult, e.g., in the outer membranes of mitochondria. As well as translational motion, nanodiamonds also undergo rotational motion, which can make alignment of an external magnetic field with a crystal axis, required for magnetic field sensing, SEDOR and NMR modalities, a significant challenge. Translational movement of the nanodiamond can be compensated for with reactive tracking techniques, allowing continued probing of the quantum sensor even at high velocities (McGuinness et al., 2011). Rotational diffusion rates can be inferred from the change in angle between the NV quantisation axis and a fixed external magnetic field, which is reflected in the relative change of the ODMR resonances over time. Simultaneous translational and rotational tracking of nanodiamonds on cell membranes has been demonstrated, with a maximum nanodiamond rotation tracking speed of 5°C per second (Feng et al., 2021), much faster than previously reported intracellular rotational speeds (McGuinness et al., 2011).
A different approach, based on reducing nanodiamond movement and rotation in cells can be achieved through chemical treatment of the living system. Nocodazole, a binding agent to beta-tubulin, effectively disrupts the microtubule network by preventing tubulin polymerization. This has been shown to reduce intracellular trafficking and cell motility, whilst being reversible and, if used transiently, has minimal effect on overall cell viability (Pelling et al., 2007). Depending on the parameter of interest, ATP depletion has also been shown to reduce intracellular movement (Guo et al., 2014). Nanodiamond movement may also be reduced by surface functionalization, which has been shown to facilitate organelle targeting (Mkandawire et al., 2009).
Alternatively, physically moving the nanodiamond to a desired location or holding it in position is possible with optical trapping. This is enabled by the high refractive index difference between the nanodiamond and the surrounding medium. By switching between trapping and pulsed MW excitation, sequential trapping and pulsed ODMR readout of a nanodiamond in an aqueous environment has been shown, including rotational control of the nanodiamond by changing the polarisation of the trapping beam (Russell et al., 2021). Modulation of the infrared beam also minimizes the localised heating effects (which are particularly prescient due to the strong absorption of infrared radiation by water (Peterman et al., 2003). Successful non-disruptive optical trapping would additionally present the opportunity to use nanodiamonds for active microrheology, both inside and outside of a cellular environment. Active microrheology, where the response of the nano particle to a driven force induced by the trap is probed, and can reveal viscoelastic properties of the environment the particle is experiencing. Several studies have used polystyrene beads to probe the dynamic material properties using this technique during changing physiological conditions (such as ATP depletion or disruption of the cytoskeletal structure) (Guo et al., 2014) or different stages of cellular mitosis (Hurst et al., 2021). Implemented for nanodiamond quantum sensing in vitro, these solutions for sensor tracking, active localisation and perturbation minimisation present an essential toolkit for realising nanodiamond quantum sensors as a widely applicable biosensing platform.
Nanodiamond quantum sensors offer a plethora of opportunities for sensing both in vitro and in vivo. They are the basis of ultrasentisive diagnostics with orders of magnitude enhanced sensitivity in biomarker detection (Miller et al., 2020) and could enable nanoparticle-based drug delivery with integrated nanosensor in model systems (Chauhan et al., 2020). The ability of NV centres to operate concurrently in different modalities opens the door to implementing interleaved protocols, where multiple parameters are measured simultaneously or in fast succession, without the need to correlate results from different sensors and different experimental runs (Gu et al., 2023). This unique feature of NV centres facilitates experiments to probe the interdependence of multiple properties at the same time. Nearby nuclear spins may be able to assist in providing higher sensitivity and spectral resolution by acting as a quantum memory for the NV quantum sensor (Aslam et al., 2017; Knowles et al., 2017; Holzgrafe et al., 2020). Optimal control techniques offer many benefits for enhanced sensitivity in nanodiamond-based sensing, where coherent control of NV defects in a complex and dynamic environment is required (Konzelmann et al., 2018). While many sensing applications for the NV centre have been developed over the last decades, other emerging materials present promising avenues for in vitro quantum sensing. Silicon carbide hosts a number of defects that can be used as quantum sensors (Castelletto and Boretti, 2020). Other defects in diamond, such as silicon-vacancy centres and germanium-vacancy centres, can act as thermometers through a temperature-dependent zero-phonon line, that can be used to read out local temperature in cells without the need for microwave excitation (Fan et al., 2018; Liu et al., 2022). Alternatively, emerging opportunities for quantum sensing applications can be found in newly discovered defects in two-dimensional materials, such as hexagonal boron-nitride, where optical thermometry (Chen et al., 2020) and ODMR (Stern et al., 2022) have been demonstrated recently. In the near future, next-generation quantum sensors can provide robustness and quantitative sensing, crucial for understanding complexity and interdependencies in living systems at the nanoscale.
Data availability statement
The original contributions presented in the study are included in the article/Supplementary Material, further inquiries can be directed to the corresponding author.
Author contributions
All authors listed have made a substantial, direct, and intellectual contribution to the work and approved it for publication.
Funding
This work was supported by the Gordon and Betty Moore Foundation (Grant No. GBMF7872), by UKRI (New Investigator Grant EP/V049704/1) and by the Royal Society through a University Research Fellowship held by HK.
Acknowledgments
We would like to thank Qiushi Gu, Louise Shanahan, Sophia Belser, Mete Atatüre, Ljiljana Fruk, Alexander Evtushenko, Ross Waller, Eric Miska and David Jordan for their helpful insights.
Conflict of interest
The authors declare that the research was conducted in the absence of any commercial or financial relationships that could be construed as a potential conflict of interest.
Publisher’s note
All claims expressed in this article are solely those of the authors and do not necessarily represent those of their affiliated organizations, or those of the publisher, the editors and the reviewers. Any product that may be evaluated in this article, or claim that may be made by its manufacturer, is not guaranteed or endorsed by the publisher.
References
Acosta, V. M., Bauch, E., Ledbetter, M. P., Waxman, A., Bouchard, L.-S., and Budker, D. (2010). Temperature dependence of the nitrogen-vacancy magnetic resonance in diamond. Phys. Rev. Lett. 104, 070801. doi:10.1103/physrevlett.104.070801
Aslam, N., Pfender, M., Neumann, P., Reuter, R., Zappe, A., Fávaro de Oliveira, F., et al. (2017). Nanoscale nuclear magnetic resonance with chemical resolution. Science 357, 67–71. doi:10.1126/science.aam8697
Baffou, G., Rigneault, H., Marguet, D., and Jullien, L. (2014). A critique of methods for temperature imaging in single cells. Nat. methods 11, 899–901. doi:10.1038/nmeth.3073
Barry, J. F., Schloss, J. M., Bauch, E., Turner, M. J., Hart, C. A., Pham, L. M., et al. (2020). Sensitivity optimization for nv-diamond magnetometry. Rev. Mod. Phys. 92, 015004. doi:10.1103/revmodphys.92.015004
Barry, J. F., Turner, M. J., Schloss, J. M., Glenn, D. R., Song, Y., Lukin, M. D., et al. (2016). Optical magnetic detection of single-neuron action potentials using quantum defects in diamond. Proc. Natl. Acad. Sci. 113, 14133–14138. doi:10.1073/pnas.1601513113
Castelletto, S., and Boretti, A. (2020). Silicon carbide color centers for quantum applications. J. Phys. Photonics 2, 022001. doi:10.1088/2515-7647/ab77a2
Chauhan, S., Jain, N., and Nagaich, U. (2020). Nanodiamonds with powerful ability for drug delivery and biomedical applications: Recent updates on in vivo study and patents. J. Pharm. analysis 10, 1–12. doi:10.1016/j.jpha.2019.09.003
Chen, Y., Tran, T. N., Duong, N. M. H., Li, C., Toth, M., Bradac, C., et al. (2020). Optical thermometry with quantum emitters in hexagonal boron nitride. ACS Appl. Mater. interfaces 12, 25464–25470. doi:10.1021/acsami.0c05735
Choi, J., Zhou, H., Landig, R., Wu, H.-Y., Yu, X., Von Stetina, S. E., et al. (2020). Probing and manipulating embryogenesis via nanoscale thermometry and temperature control. Proc. Natl. Acad. Sci. 117, 14636–14641. doi:10.1073/pnas.1922730117
Chretien, D., Bénit, P., Ha, H.-H., Keipert, S., El-Khoury, R., Chang, Y.-T., et al. (2018). Mitochondria are physiologically maintained at close to 50 c. PLoS Biol. 16, e2003992. doi:10.1371/journal.pbio.2003992
Chu, Z., Zhang, S., Zhang, B., Zhang, C., Fang, C.-Y., Rehor, I., et al. (2014). Unambiguous observation of shape effects on cellular fate of nanoparticles. Sci. Rep. 4, 4495. doi:10.1038/srep04495
Chung, C. W., Stephens, A. D., Konno, T., Ward, E., Avezov, E., Kaminski, C. F., et al. (2022). Intracellular aβ42 aggregation leads to cellular thermogenesis. J. Am. Chem. Soc. 144, 10034–10041. doi:10.1021/jacs.2c03599
Degen, C. L., Reinhard, F., and Cappellaro, P. (2017). Quantum sensing. Rev. Mod. Phys. 89, 035002. doi:10.1103/revmodphys.89.035002
Fan, J.-W., Cojocaru, I., Becker, J., Fedotov, I. V., Alkahtani, M. H. A., Alajlan, A., et al. (2018). Germanium-vacancy color center in diamond as a temperature sensor. ACS Photonics 5, 765–770. doi:10.1021/acsphotonics.7b01465
Feng, X., Leong, W.-H., Xia, K., Liu, C.-F., Liu, G.-Q., Rendler, T., et al. (2021). Association of nanodiamond rotation dynamics with cell activities by translation-rotation tracking. Nano Lett. 21, 3393–3400. doi:10.1021/acs.nanolett.0c04864
Fujiwara, M., Sun, S., Dohms, A., Nishimura, Y., Suto, K., Takezawa, Y., et al. (2020). Real-time nanodiamond thermometry probing in vivo thermogenic responses. Sci. Adv. 6, eaba9636. doi:10.1126/sciadv.aba9636
Gruber, A., Drabenstedt, A., Tietz, C., Fleury, L., Wrachtrup, J., and Borczyskowski, C. v. (1997). Scanning confocal optical microscopy and magnetic resonance on single defect centers. Science 276, 2012–2014. doi:10.1126/science.276.5321.2012
Gu, Q., Shanahan, L., Hart, J. W., Belser, S., Shofer, N., Atature, M., et al. (2023). Simultaneous nanorheometry and nanothermometry using intracellular diamond quantum sensors. arXiv:2306.17306.
Guo, M., Ehrlicher, A. J., Jensen, M. H., Renz, M., Moore, J. R., Goldman, R. D., et al. (2014). Probing the stochastic, motor-driven properties of the cytoplasm using force spectrum microscopy. Cell 158, 822–832. doi:10.1016/j.cell.2014.06.051
Han, D., Korabel, N., Chen, R., Johnston, M., Gavrilova, A., Allan, V. J., et al. (2020). Deciphering anomalous heterogeneous intracellular transport with neural networks. Elife 9, e52224. doi:10.7554/elife.52224
Haziza, S., Mohan, N., Loe-Mie, Y., Lepagnol-Bestel, A.-M., Massou, S., Adam, M.-P., et al. (2017). Fluorescent nanodiamond tracking reveals intraneuronal transport abnormalities induced by brain-disease-related genetic risk factors. Nat. Nanotechnol. 12, 322–328. doi:10.1038/nnano.2016.260
Hirano, M., Ando, R., Shimozono, S., Sugiyama, M., Takeda, N., Kurokawa, H., et al. (2022). A highly photostable and bright green fluorescent protein. Nat. Biotechnol. 40, 1132–1142. doi:10.1038/s41587-022-01278-2
Holzgrafe, J., Gu, Q., Beitner, J., Kara, D. M., Knowles, H. S., and Atatüre, M. (2020). Nanoscale nmr spectroscopy using nanodiamond quantum sensors. Phys. Rev. Appl. 13, 044004. doi:10.1103/physrevapplied.13.044004
Hui, Y. Y., Su, L.-J., Chen, O. Y., Chen, Y.-T., Liu, T.-M., and Chang, H.-C. (2014). Wide-field imaging and flow cytometric analysis of cancer cells in blood by fluorescent nanodiamond labeling and time gating. Sci. Rep. 4, 5574–5577. doi:10.1038/srep05574
Hurst, S., Vos, B. E., Brandt, M., and Betz, T. (2021). Intracellular softening and increased viscoelastic fluidity during division. Nat. Phys. 17, 1270–1276. doi:10.1038/s41567-021-01368-z
Karaveli, S., Gaathon, O., Wolcott, A., Sakakibara, R., Shemesh, O. A., Peterka, D. S., et al. (2016). Modulation of nitrogen vacancy charge state and fluorescence in nanodiamonds using electrochemical potential. Proc. Natl. Acad. Sci. 113, 3938–3943. doi:10.1073/pnas.1504451113
Knowles, H. S., Kara, D. M., and Atatüre, M. (2017). Controlling a nuclear spin in a nanodiamond. Phys. Rev. B 96, 115206. doi:10.1103/physrevb.96.115206
Knowles, H. S., Kara, D. M., and Atatüre, M. (2016). Demonstration of a coherent electronic spin cluster in diamond. Phys. Rev. Lett. 117, 100802. doi:10.1103/physrevlett.117.100802
Knowles, H. S., Kara, D. M., and Atatüre, M. (2014). Observing bulk diamond spin coherence in high-purity nanodiamonds. Nat. Mater. 13, 21–25. doi:10.1038/nmat3805
Konzelmann, P., Rendler, T., Bergholm, V., Zappe, A., Pfannenstill, V., Garsi, M., et al. (2018). Robust and efficient quantum optimal control of spin probes in a complex (biological) environment. towards sensing of fast temperature fluctuations. New J. Phys. 20, 123013. doi:10.1088/1367-2630/aaf315
Kucsko, G., Maurer, P. C., Yao, N. Y., Kubo, M., Noh, H. J., Lo, P. K., et al. (2013). Nanometre-scale thermometry in a living cell. Nature 500, 54–58. doi:10.1038/nature12373
Kuo, Y., Hsu, T.-Y., Wu, Y.-C., and Chang, H.-C. (2013). Fluorescent nanodiamond as a probe for the intercellular transport of proteins in vivo. Biomaterials 34, 8352–8360. doi:10.1016/j.biomaterials.2013.07.043
Lavi, R., Shainberg, A., Shneyvays, V., Hochauser, E., Isaac, A., Zinman, T., et al. (2010). Detailed analysis of reactive oxygen species induced by visible light in various cell types. Lasers Surg. Med. 42, 473–480. doi:10.1002/lsm.20919
Le Sage, D., Arai, K., Glenn, D. R., DeVience, S. J., Pham, L. M., Rahn-Lee, L., et al. (2013). Optical magnetic imaging of living cells. Nature 496, 486–489. doi:10.1038/nature12072
Liu, K.-K., Wang, C.-C., Cheng, C.-L., and Chao, J.-I. (2009). Endocytic carboxylated nanodiamond for the labeling and tracking of cell division and differentiation in cancer and stem cells. Biomaterials 30, 4249–4259. doi:10.1016/j.biomaterials.2009.04.056
Liu, W., Alam, M. N. A., Liu, Y., Agafonov, V. N., Qi, H., Koynov, K., et al. (2022). Silicon-vacancy nanodiamonds as high performance near-infrared emitters for live-cell dual-color imaging and thermometry. Nano Lett. 22, 2881–2888. doi:10.1021/acs.nanolett.2c00040
Mamin, H., Kim, M., Sherwood, M., Rettner, C., Ohno, K., Awschalom, D., et al. (2013). Nanoscale nuclear magnetic resonance with a nitrogen-vacancy spin sensor. Science 339, 557–560. doi:10.1126/science.1231540
McCloskey, D., Dontschuk, N., Stacey, A., Pattinson, C., Nadarajah, A., Hall, L., et al. (2022). A diamond voltage imaging microscope. Nat. Photonics 16, 730–736. doi:10.1038/s41566-022-01064-1
McGuinness, L. P., Yan, Y., Stacey, A., Simpson, D. A., Hall, L. T., Maclaurin, D., et al. (2011). Quantum measurement and orientation tracking of fluorescent nanodiamonds inside living cells. Nat. Nanotechnol. 6, 358–363. doi:10.1038/nnano.2011.64
Miller, B. S., Bezinge, L., Gliddon, H. D., Huang, D., Dold, G., Gray, E. R., et al. (2020). Spin-enhanced nanodiamond biosensing for ultrasensitive diagnostics. Nature 587, 588–593. doi:10.1038/s41586-020-2917-1
Mkandawire, M., Pohl, A., Gubarevich, T., Lapina, V., Appelhans, D., Roedel, G., et al. (2009). Selective targeting of green fluorescent nanodiamond conjugates to mitochondria in hela cells. J. Biophot. 2, 596–606. doi:10.1002/jbio.200910002
Mzyk, A., Sigaeva, A., and Schirhagl, R. (2022). Relaxometry with nitrogen vacancy (nv) centers in diamond. Accounts Chem. Res. 55, 3572–3580. doi:10.1021/acs.accounts.2c00520
Nie, L., Nusantara, A. C., Damle, V. G., Sharmin, R., Evans, E. P., Hemelaar, S. R., et al. (2021). Quantum monitoring of cellular metabolic activities in single mitochondria. Sci. Adv. 7, eabf0573–9. doi:10.1126/sciadv.abf0573
Oshimi, K., Nishimura, Y., Matsubara, T., Tanaka, M., Shikoh, E., Zhao, L., et al. (2022). Glass-patternable notch-shaped microwave architecture for on-chip spin detection in biological samples. Lab a Chip 22, 2519–2530. doi:10.1039/d2lc00112h
Pelling, A. E., Dawson, D. W., Carreon, D. M., Christiansen, J. J., Shen, R. R., Teitell, M. A., et al. (2007). Distinct contributions of microtubule subtypes to cell membrane shape and stability. Nanomedicine Nanotechnol. Biol. Med. 3, 43–52. doi:10.1016/j.nano.2006.11.006
Perona Martinez, F., Nusantara, A. C., Chipaux, M., Padamati, S. K., and Schirhagl, R. (2020). Nanodiamond relaxometry-based detection of free-radical species when produced in chemical reactions in biologically relevant conditions. ACS sensors 5, 3862–3869. doi:10.1021/acssensors.0c01037
Peterman, E. J., Gittes, F., and Schmidt, C. F. (2003). Laser-induced heating in optical traps. Biophysical J. 84, 1308–1316. doi:10.1016/s0006-3495(03)74946-7
Plakhotnik, T., Aman, H., and Chang, H.-C. (2015). All-optical single-nanoparticle ratiometric thermometry with a noise floor of 0.3 k hz- 1/2. Nanotechnology 26, 245501. doi:10.1088/0957-4484/26/24/245501
Qureshi, S. A., Hsiao, W. W.-W., Hussain, L., Aman, H., Le, T.-N., and Rafique, M. (2022). Recent development of fluorescent nanodiamonds for optical biosensing and disease diagnosis. Biosensors 12, 1181. doi:10.3390/bios12121181
Reineck, P., Francis, A., Orth, A., Lau, D. W. M., Nixon-Luke, R. D. V., Rastogi, I. D., et al. (2016). Brightness and photostability of emerging red and near-ir fluorescent nanomaterials for bioimaging. Adv. Opt. Mater. 4, 1549–1557. doi:10.1002/adom.201600212
Russell, L. W., Dossetor, E. C., Wood, A. A., Simpson, D. A., and Reece, P. J. (2021). Optimizing optical tweezers experiments for magnetic resonance sensing with nanodiamonds. ACS Photonics 8, 1214–1221. doi:10.1021/acsphotonics.1c00137
Sigaeva, A., Shirzad, H., Martinez, F. P., Nusantara, A. C., Mougios, N., Chipaux, M., et al. (2022). Diamond-based nanoscale quantum relaxometry for sensing free radical production in cells. Small 18, 2105750. doi:10.1002/smll.202105750
Simpson, D. A., Morrisroe, E., McCoey, J. M., Lombard, A. H., Mendis, D. C., Treussart, F., et al. (2017). Non-neurotoxic nanodiamond probes for intraneuronal temperature mapping. ACS Nano 11, 12077–12086. doi:10.1021/acsnano.7b04850
Simpson, D. A., Thompson, A. J., Kowarsky, M., Zeeshan, N. F., Barson, M. S., Hall, L. T., et al. (2014). In vivo imaging and tracking of individual nanodiamonds in drosophila melanogaster embryos. Biomed. Opt. express 5, 1250–1261. doi:10.1364/boe.5.001250
Sow, M., Steuer, H., Adekanye, S., Ginés, L., Mandal, S., Gilboa, B., et al. (2020). High-throughput nitrogen-vacancy center imaging for nanodiamond photophysical characterization and ph nanosensing. Nanoscale 12, 21821–21831. doi:10.1039/d0nr05931e
Stern, H. L., Gu, Q., Jarman, J., Eizagirre Barker, S., Mendelson, N., Chugh, D., et al. (2022). Room-temperature optically detected magnetic resonance of single defects in hexagonal boron nitride. Nat. Commun. 13, 618. doi:10.1038/s41467-022-28169-z
Vlot, A. C., Dempsey, D. A., and Klessig, D. F. (2009). Salicylic acid, a multifaceted hormone to combat disease. Annu. Rev. phytopathology 47, 177–206. doi:10.1146/annurev.phyto.050908.135202
Waigh, T. A. (2016). Advances in the microrheology of complex fluids. Rep. Prog. Phys. 79, 074601. doi:10.1088/0034-4885/79/7/074601
Woodhams, B., Ansel-Bollepalli, L., Surmacki, J., Knowles, H., Maggini, L., De Volder, M., et al. (2018). Graphitic and oxidised high pressure high temperature (hpht) nanodiamonds induce differential biological responses in breast cancer cell lines. Nanoscale 10, 12169–12179. doi:10.1039/c8nr02177e
Yu, S.-J., Kang, M.-W., Chang, H.-C., Chen, K.-M., and Yu, Y.-C. (2005). Bright fluorescent nanodiamonds: No photobleaching and low cytotoxicity. J. Am. Chem. Soc. 127, 17604–17605. doi:10.1021/ja0567081
Yukawa, H., Fujiwara, M., Kobayashi, K., Kumon, Y., Miyaji, K., Nishimura, Y., et al. (2020). A quantum thermometric sensing and analysis system using fluorescent nanodiamonds for the evaluation of living stem cell functions according to intracellular temperature. Nanoscale Adv. 2, 1859–1868. doi:10.1039/d0na00146e
Keywords: nanodiamond, quantum sensing, biosensing, nitrogen-vacancy center, nanoscale probes
Citation: Hart JW and Knowles HS (2023) Multimodal quantum metrology in living systems using nitrogen-vacancy centres in diamond nanocrystals. Front. Quantum Sci. Technol. 2:1220015. doi: 10.3389/frqst.2023.1220015
Received: 09 May 2023; Accepted: 20 July 2023;
Published: 01 August 2023.
Edited by:
Philipp Reineck, RMIT University, AustraliaReviewed by:
Taras Plakhotnik, The University of Queensland, AustraliaCopyright © 2023 Hart and Knowles. This is an open-access article distributed under the terms of the Creative Commons Attribution License (CC BY). The use, distribution or reproduction in other forums is permitted, provided the original author(s) and the copyright owner(s) are credited and that the original publication in this journal is cited, in accordance with accepted academic practice. No use, distribution or reproduction is permitted which does not comply with these terms.
*Correspondence: Helena S. Knowles, aHNrMzVAY2FtLmFjLnVr