- 1Department of Genetics and Molecular Biology, School of Medicine, Isfahan University of Medical Sciences, Isfahan, Iran
- 2Pediatric Inherited Diseases Research Center, Isfahan University of Medical Sciences, Isfahan, Iran
- 3Department of Parasitology and Mycology, School of Medicine, Isfahan University of Medical Sciences, Isfahan, Iran
Despite the development of a vaccine against cutaneous leishmaniasis in preclinical and clinical studies, we still do not have a safe and effective vaccine for human use. Given this situation, the search for a new prophylactic alternative to control leishmaniasis should be a global priority. A first-generation vaccine strategy—leishmanization, in which live Leishmania major parasites are inoculated into the skin to protect against reinfection, is taking advantage of this situation. Live attenuated Leishmania vaccine candidates are promising alternatives due to their robust protective immune responses. Importantly, they do not cause disease and could provide long-term protection following challenges with a virulent strain. In addition to physical and chemical methods, genetic tools, including the Cre-loxP system, have enabled the selection of safer null mutant live attenuated Leishmania parasites obtained by gene disruption. This was followed by the discovery and introduction of CRISPR/Cas-based gene editing tools, which can be easily and precisely used to modify genes. Here, we briefly review the immunopathology of L. major parasites and then present the classical methods and their limitations for the production of live attenuated vaccines. We then discuss the potential of current genetic engineering tools to generate live attenuated vaccine strains by targeting key genes involved in L. major pathogenesis and then discuss their discovery and implications for immune responses to control leishmaniasis.
Introduction
Leishmaniasis is a vector-borne infection caused by Leishmania—an obligate intracellular protozoan parasite. The two morphologically distinct forms of this are the promastigote, which is passed on by female Phlebotomine sandflies, and the amastigote, which occurs in mammalian hosts. The several clinical forms of the disease can be grouped into three main clinical forms: visceral (VL), mucocutaneous (MCL), and cutaneous leishmaniasis (CL) (1). CL is a painless and chronic ulcer at the site of sandfly bites and is the most common clinical syndrome in many affected regions, especially in the Middle East, where it has been reported in two main forms: zoonotic CL (ZCL) caused by Leishmania major and anthroponotic CL (ACL) caused by Leishmania tropica and mixed infection with them, which is high there (2, 3). In 2022, WHO reported that 85% of the global CL incidence occurred in eight countries, Afghanistan, Iran, Iraq, Syria, Algeria, Brazil, Colombia, and Peru (4). New outbreaks in the Middle East in recent years have been linked to wars in Syria, Yemen, Turkey, and Iraq. Refugee migration from endemic to non-endemic areas and vice versa, poor hygiene, malnutrition, weak immune systems, poor housing, lack of resources, environmental conditions, climate change, poor urbanization management, use of agricultural lands for residential purposes, and changes in vector populations link to a substantial rise in CL prevalence, which are present circumstances in most of the Middle East (3, 5, 6) (Figure 1). Although the first line of treatment of leishmaniasis with pentavalent antimonials is affordable and generally available in many endemic countries in the Middle East, economic sanctions, war, and counterfeit drug markets make access to the standard treatment difficult. In addition, the efficacy of this type of treatment is variable due to drug resistance and induction of organ toxicity (2, 3).
Fortunately, the development of immunity to the parasite in infected individuals following rehabilitation has highlighted the role of vaccination in disease management (5). In addition, the partial understanding of the immunopathogenesis of leishmaniasis has motivated immunologists and researchers in the leishmaniasis field to investigate and develop the different types of vaccines required. In the early 20th century, controlled inoculation of live virulent L. major promastigotes was used to immunize people in hyperendemic regions, preventing parasite infection in up to 80% of people. However, leishmanization as effectively powerful to control CL had several disadvantages that led to its abandonment (except in Uzbekistan, where this method is still used), including permanent skin lesions, safety concerns about HIV transmission, limitations in immunosuppressed people, and issues with Good Manufacturing Practice (GMP) standards (7, 8). Given these challenges, vaccine development shifted to inactivated vaccines. Due to the simplicity and cost-effectiveness of the production process, inactivated vaccines have been developed in various formulations. They are considered safe human vaccines and have been used as an alternative medication for drug-resistant type CL (9). Inactivation of the parasite while preserving the antigenic structures has been achieved by physical methods such as heat, chemicals, sonication, or UV radiation. This category has been studied in many clinical trials, but none of them have been approved by the World Health Organization (WHO) due to the lack of remarkable efficacy and the need for multiple vaccine doses (7, 8).
In addition to extended vaccines based on whole organism components, purified immunogenic fragments of the parasite have been developed as vaccine candidates, reducing the possibility of adverse reactions. Leishmune®–a commercial canine vaccine consisting of fucose–mannose ligand (FML) from Leishmania donovani adjuvanted with QuilA saponin shows moderate clinical signs and lesions in vaccinated/infected dogs (8, 10). Remarkable advances in molecular biology have led to a new generation emergence of subunit or synthetic leishmaniasis vaccines based on membrane or soluble parasite proteins, replacing the previous native form vaccines. Cost-effectiveness and a straightforward manufacturing process allow their large-scale production. There is no live pathogen and no risk of infection in immunosuppressed individuals. With all these advantages, there are also some disadvantages, including an attempt to escape immune system deactivation and increased immunogenicity. Variations in the final conformation and structure of peptides occur due to heterologous expression systems, which could almost be related to post-translational modifications. Also, the epitopes could be selected to induce the desired immune response, and a particular antigenic arrangement could be chosen to induce a milder immune response (8, 11).
The Leish-111f vaccine is a tandem combination of three highly conserved Leishmania antigens, thiol-specific antioxidant (TSA), L. major stress-inducible protein 1 (LmSTI1), and Leishmania elongation initiation factor (LeIF), resulting in a 111 kDa polyprotein. In addition, studies indicated that Leish-111f formulated in IL-12 induces antibody response and IFN-γ production as well as soluble Leishmania antigen (SLA), but MPL-SE is considered a suitable alternative due to problems related to the manufacturing process and uncertainty of safety (12). Leish-111f is the first leishmaniasis vaccine to demonstrate immunogenicity in human clinical trials (12). In human clinical trials, Leish-111f is the first leishmaniasis vaccine that has demonstrated immunogenicity. A total of 77 healthy Indian subjects, with or without previous exposure to Leishmania, were administered three doses of Leish-F1+MPL-SE and followed for 168 days. Results showed safe and mild reactions associated with an increase in Th1-type cytokines (13). Purified peptides from different hosts administered with CpG adjuvant in BALB/c mice and eukaryotically expressed vaccine resulted in greater immune protection than the prokaryotic vaccine due to critical modifications that occur during protein construction in L. tarentolae, such as glycosylation, which involves the attachment of carbohydrate molecules to the N- or C-terminus of proteins, responsible for efficient peptide folding and interaction. Moreover, many studies have shown that glycosylation improves the immunogenicity and duration of conjugated vaccines compared to non-glycosylated vaccines (14, 15). Recently, significant advances in gene editing tools and Leishmania genome manipulation and generation of mutant weakened parasites have been explored as a desirable means of disease management. In this paper, we have reviewed the development of genetically live attenuated Leishmania vaccines.
Leishmania immunology
Following the entry of Leishmania promastigotes into the host's dermal layer via the sandfly bite, the parasites reside in phagocytic cells such as tissue macrophages and dendritic cells or neutrophils. Leishmania GP63 directly uses complement C3 cleavage to prevent complement-mediated lysis, allowing C3bi to interact with the phagocytic cell receptor CR3 for facilitating attachment and uptake. Activated dendritic cells migrate from sites of antigen acquisition to draining lymph nodes and present Leishmania antigens to naive T cells, accompanied by the production of cytokines leading to CD4+ and CD8+ activation. The future fate of the parasite depends on the polarization and the final phenotype of the macrophages. The differentiation of macrophages into pro-inflammatory (M1) or anti-inflammatory (M2) phenotypes, known as macrophage polarization, plays a critical role in the immune response to leishmaniasis. Resistance to Leishmania infection is associated with the M1 phenotype, whereas the M2 phenotype dominates in susceptible environments. The balance between M1 and M2 macrophage polarization can be regulated by cytokines produced by CD4+ Th1 and Th2 lymphocyte subpopulations. The M1 macrophage polarization is mainly due to LPS, IFN, TNF, and GM-CSF, which also activates the complement system and recruits the immune cells. The polarization of macrophages into the M2 subset by the Leishmania parasites under secretion of Th2 cytokines and reduction of dendritic cells results in a decrease in antigen presentation and an immunosuppressive environment that supports their survival (16–18). Toll-like receptors (TLRs) play a key role in enhancing the immune response in the context of cutaneous vaccination by identifying pathogens. Some TLR ligands, such as prokaryotic CpG oligodeoxynucleotide (ODN) motifs, are considered effective adjuvants identified by TLR9. CpG ODNs induce the production of pro-inflammatory cytokines, including IL-12 and IFN-γ, which promote the development of a Th1 immune response (19). In a case-control study, gene expression measurement of IL-12 P40, IFN-γ, IL-1β, IL-4, and IL-10 from peripheral blood mononuclear cells (PBMC) of patients with anthroponotic cutaneous leishmaniasis (ACL) who responded and those who did not respond to meglumine antimoniate treatment showed a significant increase in Th1 cytokines (IL-12 P40, IFN-γ, and IL-1β) in the responsive group and Th2 cytokines (IL-4 and IL-10) in the non-responsive group (20). It has also been reported that the CD4+ T-cell response weakens in people with symptomatic visceral leishmaniasis but could return along with central memory T-cells that induce immunity after medication (21).
Strategies to produce attenuated vaccines
Attenuated vaccines could be produced by limiting the pathogenicity of the parasite through some techniques (Table 1). Weakened pathogens as whole-organism vaccines could present a set of antigens to the immune system, limiting the effect of antigenic polymorphism and genetic variation (22). It could also simulate actual infection and potentially activate the Th1 immune response. But sometimes, depending on the attenuation method, important immunogenic epitopes cannot be generated. This is a major drawback that limits the use of attenuated vaccines in immunosuppressive conditions such as HIV infection, organ transplantation, chemotherapy, or pregnancy. Strategies used to attenuate parasites based on defined and undefined genetic alterations include chemical, physical, and genetic attenuation (Table 1).
Physical methods include techniques such as prolonged subculture, use of radiation (gamma rays or UV), and temperature sensitivity. Treatment with mutagenic agents or promastigote culture under antibiotic pressure is considered chemical attenuation. The gentamicin-attenuated L. major vaccine is now in clinical trials and has shown promising results in mice and humans.
On the other hand, it also defined modifications that lead to the knocking out of genes responsible for pathogenicity. Today, this approach could be a suitable alternative that reduces the potential for reversibility (23–25). In addition, unlike the old method of leishmanization, mutant parasites altered using precise gene manipulation tools led to the appearance of an improved leishmanization in terms of non-pathogenicity and protection against all divergent Leishmania species (26).
Genetically attenuated parasites
Good candidate gene for attenuated vaccines
Live attenuated Leishmania vaccines as non-pathogenic parasites that provide the immune system with whole antigens that are almost identical to the wild type stimulate immunologic memory cells and are considered potent vaccine candidates (35). Disruption of the activity of Leishmania genes could be achieved by knocking out one or two alleles. Parasites with one mutated allele, although showing a different phenotype from wild-type parasites, are considered dangerous vaccines due to the possibility of reversion. Knocking out two alleles results in loss of function (homozygous inactivation), thus maintaining survival in the host and culture environment and eliminating the risk of reactivation and pathogenesis, which could enhance immunity (25). The identification of Leishmania growth factors and virulence biomarkers, which play an important role in the immunomodulatory mechanisms and host interactions, was considered essential. The expansion of genetically live attenuated Leishmania vaccines could be improved through the attenuation of these biomarkers. Furthermore, the complete representation of the genetically live attenuated parasites prepares the analysis of the characteristics such as virulence and growth potential or the strength of immunogenicity (36).
There is strong evidence for the efficacy of genetically attenuated vaccines against malaria and leishmaniasis. Currently, mutant forms of Plasmodium falciparum have been produced that are reproducible parasites with the ability to be attenuated at the appropriate time of liver stage development, so-called early liver stage-arresting, replication-deficient (EARD) genetically attenuated parasites (GAP). These attenuated parasites were able to infect hepatocytes and transform into trophozoites (37). Next-generation GAPs, in addition to critical gene deletions, have acquired a specific gene sequence (gain of function) or additional function that results in the ability of the parasite to self-destruct at a desired time (38). Genetic knockout of the sporozoite liver-stage asparagine-rich protein (SLARP or SAP1) disrupts parasite growth in the primary liver stage before nuclear division. There is a broad consensus that the existence of the parasite in the hepatocyte, with its dynamic metabolism and restricted cell division, is necessary for long-term protection and immunity (39). The first in-human clinical trial and evaluation of the non-replicating, live, genetically attenuated Plasmodium falciparum sporozoite vaccine (PfSPZ-GA1), a double knockout parasite lacking the b9 and slarp genes important for liver development (PfΔb9Δslarp), demonstrated safety, immunogenicity, and efficacy in malaria-naive Dutch volunteers (40).
In the case of genetically attenuated Leishmania, there is no limit to the selection of different target genes, provided that the disruption results in parasites that can infect cells and induce strong immunity without clinical observations. Various protein gene deletions such as metabolic enzymes, signaling pathway proteins, cell surface, and cytoskeleton-related proteins could be considered as suitable interventions (26) (Table 2). Namely, mutated L. major parasites with deletion of gene encoding the p27 protein (41), DHFR-TS (42, 43), GP63 (44), LPG (45), Centrin1, and many other genes have shown a significant reduction in parasite burden and symptoms as well as high immunity to challenge (46). Characterization of some live attenuated L. donovani vaccine candidates with deletion of the Centrin1 and p27 genes has shown that the expression pattern of immunomodulatory proteins, such as HSP70 and tryparedoxin tubulins, DEAD-box RNA helicases, and host-protective proteins, including cytochrome c, calreticulin, and glyceraldehyde-3-phosphate dehydrogenase (GAPDH) are regulated in these parasites (47). Thus, these proteins could be studied as biomarkers for their role in attenuating the reproductive effect.
L. major mutant strains generated using advanced gene editing techniques, in which the targeted modification of the Centrin gene is accompanied by the insertion of an antibiotic resistance marker into the genome, are superior for development in Phase I human clinical trials. L. major Centrin gene-deleted parasites (L.mCen–/–) have also been shown to be safe and protective in immunodeficient mouse models. In addition, LmCen–/– parasites demonstrated immunity to sandfly challenge (48).
Cre-loxP system
The Cre-loxP system has been used as a genetic engineering tool to enhance recombination between two loxP sequences for in vivo/vitro studies. The Cre recombinase gene is located near an inducible promoter to perform controllable or stage-specific gene deletion during the recombination process, which is advantageous for the phenotypic analysis of different genes. Genome editing by excision action of the Cre recombinase enzyme on the sequences flanked by the locus of crossover of the bacteriophage P1 (loxP) sites has been used in mammalian systems, given the absence of a regulated induction system, not long ago had not been administered to Leishmania. The advent of diCre technology overcame some of the system's drawbacks, such as sensitivity to leakage and promoter type. In this system, the Cre protein is cleaved into two functional inactive domains and lined to FKBP12 (FK506 binding protein) and FRB (binding domain of the FKBP12-rapamycin-associated protein). The addition of rapamycin or its analogs leads to fusion and activation of the separate domains, resulting in a recombination process between loxP sequences. This technique is an effective way to reduce the side effects of overexpression of active, potentially cytotoxic Cre recombinase. The diCre approach is unlikely to apply to some important genes that are organized in multi-copy arrays. Also, diCre will not avoid compensatory genetic reorganization in long-term null mutant studies (72, 73). For example, the inducible diCre system was used to knock out the CRK3 gene in Leishmania, demonstrating the requirement for CRK3 function in the regulation of mitosis and clearly showing growth failure in the cells 48 h after targeted deletion of CRK3 (71).
CRISPR
Clustered regulatory interspaced short palindromic repeats—the CRISPR/Cas system is a defense mechanism in bacterial microorganisms against foreign genetic material. CRISPR-Cas interference occurs when an infection occurs, and viruses or foreign plasmids enter the bacterial cell. After infection, unknown genetic sequences integrate into the bacterial CRISPR locus as spacer arrays, conferring immunity to subsequent infections associated with these viruses. RNA polymerase then transcribes pre-CRISPR RNAs (pre-crRNAs) from the spacer sequence of the CRISPR region, which eventually bind to Cas nucleases and form hydrogen bonds specifically with the DNA sequence target. This is accompanied by a transcription of the trans-activating crRNA (tracrRNA) from the CRISPR locus, leading to the maturation of the pre-crRNA by the enzyme RNase III and crRNA-directed DNA cleavage. The tracrRNA: crRNA complex is packaged with CRISPR-associated nuclease (Cas) to form a ribonucleoprotein (RNP) complex. This active complex releases Cas nuclease to create a double-strand break (DSB) in the DNA at the target sequence correlative to the crRNA sequence (72, 73). The Cas9 endonuclease, the class 2 type II CRISPR system, is the most widely used and precise genome editing tool. The first Cas9 endonuclease used in mammalian systems for gene editing belongs to Streptococcus pyogenes. The Cas9 enzyme has two endonuclease domains, RuvC and HNH, which cleaves the DNA strand non-complementary to the spacer sequence and the complementary strand, respectively (74, 75). Adhesion of the Cas-RNA complex to the target DNA spacer sequence (~20 nucleotides) near the protospacer adjacent motif (PAM 5′-NGG) induces the two Cas9 domains to cooperate, resulting in blunt double-strand breaks in DNA (76). Most of the DSBs could be repaired by DNA repair systems, including microhomology-mediated end joining (MMEJ) or homology direct repair (HDR) (77).
CRISPR technology has several advantages, such as its availability and simplicity for consumers, high efficiency, and suitability for genetic screening, which have allowed the application of this technique in all major fields (78). However, despite the efforts that have been made, there are some major concerns and limitations for the adoption of CRISPR/Cas9. The high incidence of off-target genome editing, probably more than 50%, has been observed and is mostly related to DNA modifications in non-specific regions or by misguidance of single guide RNA (sgRNA). An efficient approach to reduce off-target effects is to use Cas variants such as Cas9 nickase, which produces single-stranded breaks, whereas a double sgRNA targets both DNA strands at the target site and produces the DSB. Another limitation of CRISPR/Cas9 is the need for a PAM sequence adjacent to the target region.
CRISPR could cause DNA damage and apoptosis as a result of DSBs rather than the targeted gene editing (75). CRISPR has great superiority in indel efficiency in various cells compared to some gene-editing nucleases, but insufficient indels and high HDR could be increased depending on the variation of the target region (78). Designing an efficient gRNA for post-transcriptional modification of mRNA is a challenge for CRISPR technology. In 2014, Gao et al. designed an artificial gene RGR (ribozyme-gRNA-ribozyme) that promotes guide RNA production feasible (79). In addition, targeted delivery of CRISPR/Cas9 effectors is critical. Delivery methods vary depending on the cell type and include physical methods and viral methods (adenovirus or lentivirus vectors).
To date, major improvements in gene editing tools such as CRISPR technology have enabled the creation of genetically modified parasites with reduced virulence, persistent survival, and growth rate (35). Recent studies have shown that Leishmania strains, as polyploid organisms, have more than one set of chromosomes, and that genome evolution and repair mutations lead to the breakdown of the gene editing process. Leishmania could adapt to unstable situations through evolutionary mechanisms; furthermore, this parasite makes use of heterogeneous genome and regulatory procedures at different levels such as genomic, transcriptomic, and translational steps, which contribute to the ultimate survival and reversion of the pathogen so that genetic manipulation of crucial genes of trypanosomatids is considered more challenging than it seems (26, 80). Before the CRISPR-Cas9 era, gene deletion in Leishmania was more challenging due to low recombination capacity and the presence of an extra chromosome. Since the initial approval of CRISPR/Cas9 technology in Trypanosoma cruzi, Leishmania, and Trypanosoma brucei, gene replacement in trypanosomatids has become convenient and time-saving. It has also contributed to the study of basic biological mechanisms and functions in parasites (81).
Second-generation leishmanization was presented by introducing an attenuated L. major strain mutated in the Centrin1 gene (a cytoskeletal protein involved in mitosis) (LmCen–/–) using the CRISPR/Cas system (Figure 2). This attenuated parasite was found to be free of antibiotic resistance markers and there were no detectable off-target mutations, allowing it to be developed into a Phase 1 clinical trial. Animal models immunized with this attenuated vaccine showed a strong immune response but no visible lesions after the challenge with the infected sandfly, while non-immunized mice showed visible lesions and higher parasite loads. LmCen–/– is considered safe and effective compared to conventional leishmanization. It does not induce leishmaniasis in immunocompromised animals but does induce host immunity against sandfly infection (48). Of note, to fully exploit the editing potential of CRISPR/Cas9, they must be successfully delivered into target cells or tissues using appropriate viral and non-viral vectors, as reviewed in Goyal et al. (82) and Ayari-Riabi et al. (83).
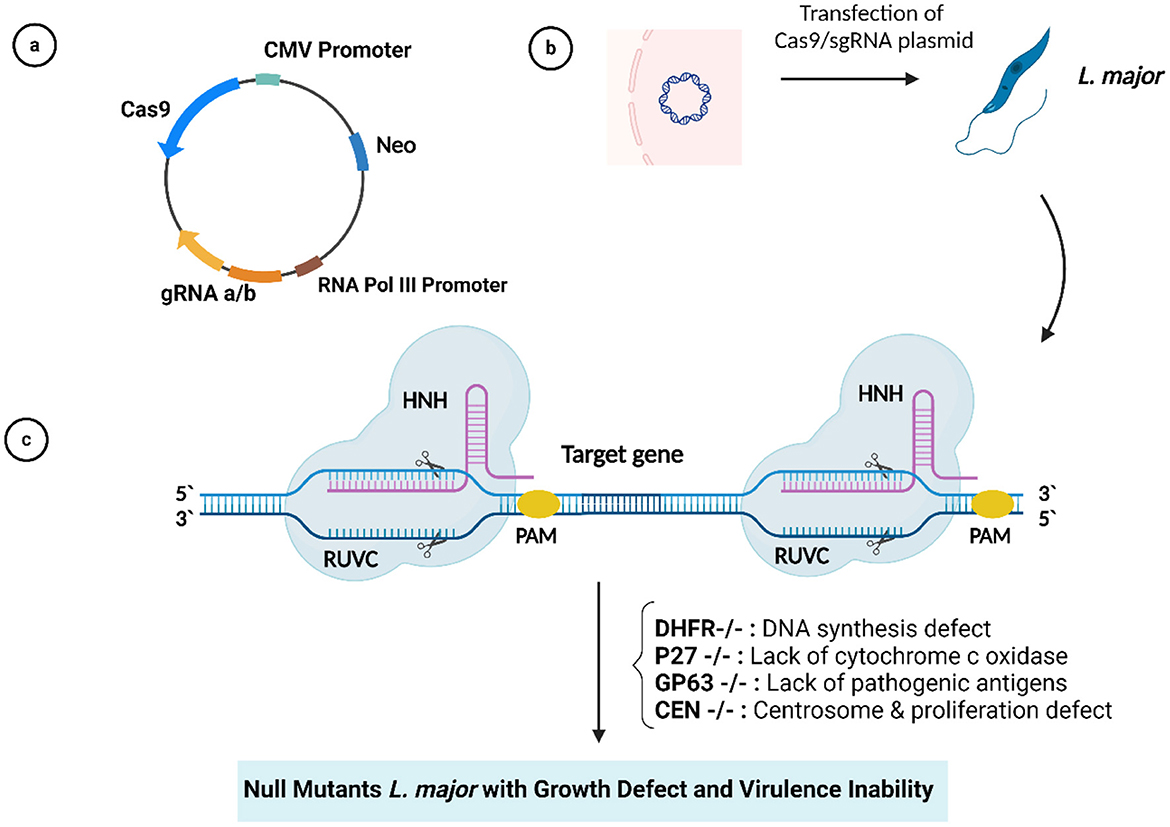
Figure 2. (A) Plasmid with Cas9/gRNA sequences. (B) Plasmid transfection into Leishmania major. (C) CRISPR/Cas9-mediated target gene deletion.
Overall, new live vaccine platforms are also being explored but are still in the early stages of development for use against infectious pathogens. However, similar to classical whole-organism vaccine platforms, these novel vaccines also require the cultivation of the pathogen. Moreover, one of the disadvantages of this platform is that it must be delivered directly into cells, which requires a special injection device or a carrier molecule and carries the risk of low transfection rates and limited immunogenicity. However, next-generation live vaccines can be constructed using only the genetic sequence of the pathogen, significantly increasing the speed of development and manufacturing processes.
Immunization of genetically live attenuated vaccines
The development of genetically modified live attenuated L. major Centrin-deleted parasites as a second method of leishmanization could induce protection via the action of IFN-γ-secreting Ly6+CD4+ T effector cells and multifunctional T cells that secrete cytokines such as IFN-γ, which is necessary for their production and survival. The LmCen–/– vaccine could also generate CD4+ skin tissue-resident memory (TRM) T cells that proliferate at the site of infection and secrete more IFN-γ and granzyme B in immunized animal models (46, 48). Central memory T cells (TCM) and skin TRM have been characterized as Leishmania-independent memory T cells (Figure 3). TRM cells are particularly suitable for protection, probably due to their localization and recruitment following vaccination or Leishmania infection. Following the parasite challenge, TRM cells immediately begin to reduce parasite loads, and it has been suggested that development strategies involving these cells will be helpful in pursuit of a leishmaniasis vaccine (84).
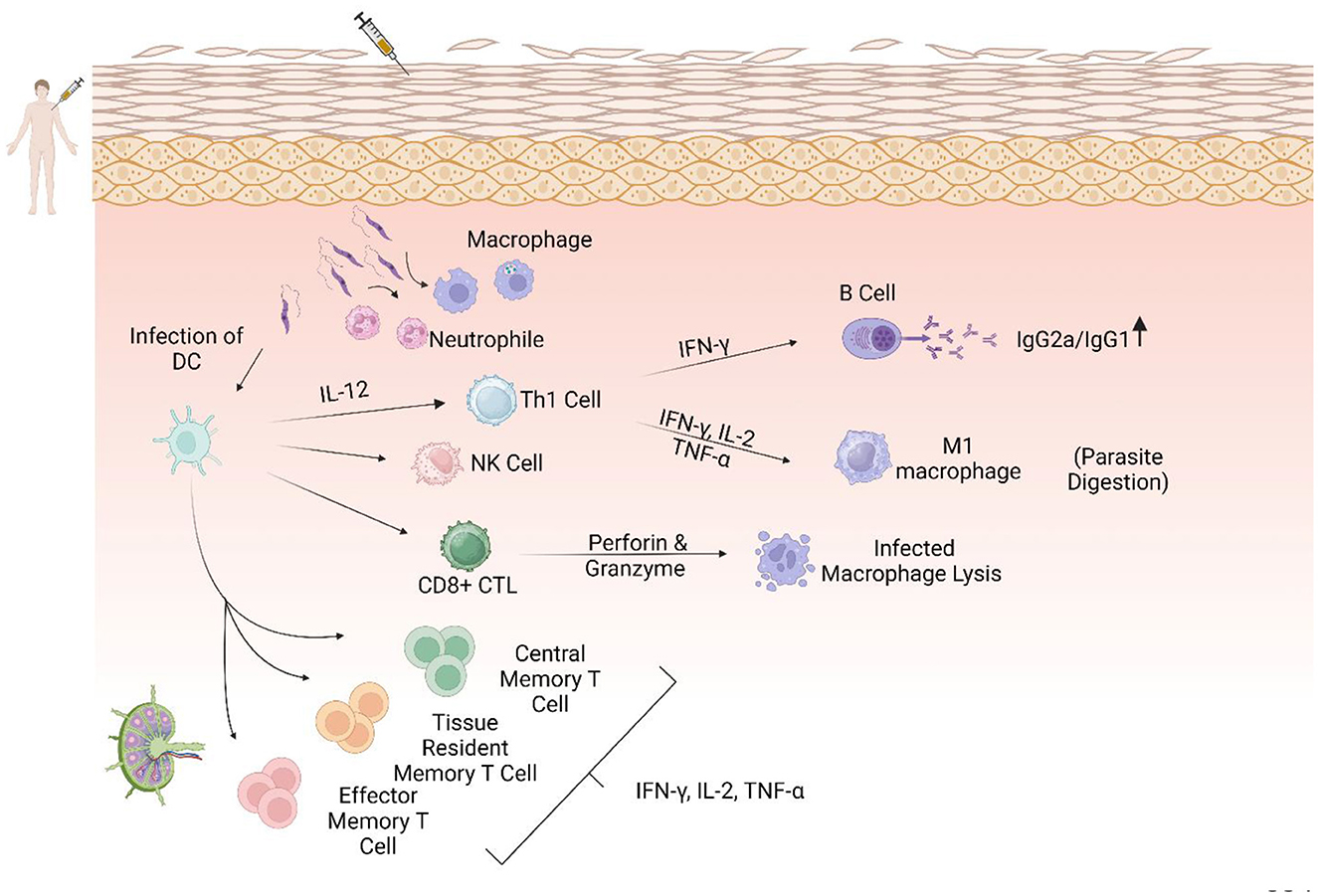
Figure 3. The central role of the Th1 response in immunization against leishmaniasis. Dendritic cells as antigen-presenting cells (APCs) after some interactions, including TLR4-L. LPG and phagocytic process, migrate to the lymphatic drains, activate CD4+ and CD8+ T cells, and secrete IL-12, which could promote a Th1 immune response. Th1 cells secrete pro-inflammatory cytokines such as IL-2, IFN-γ, and TNF-α, which activate M1 macrophages and increase NO and ROS production, leading to parasite clearance. In addition, CD8+ cells become cytotoxic T cells that produce perforin and granzymes that lyse infected macrophages. Increasing the ratio of IgG2a/IgG1 could lead to a protective immune response.
In addition, Greta Volpedo et al. reported that immunization with Centrin-deficient L. mexicana also results in higher levels of IL-12 and generation of central memory T cells (CD4+CD44+CD62L+) and significantly higher Th1 immune responses in the skin and lymph nodes of BALB/c mice compared to non-immunized mice. Overall, the ratio of IFN-γ/IL-10 to IFN-γ/IL-4 represents the physiological balance between Th1 and Th2 responses that determines disease outcome and can make the difference between resistance and susceptibility. However, when compared to the New World Leishmania strains that cause cutaneous disease, L. major exhibits different immunological characteristics and pathologies. Analysis of metabolic responses in immune cells following immunization with LmexCen–/– revealed increased aspartate metabolism and pentose phosphate pathway (PPP), which induce M1 polarization in macrophages, and PPP also promotes nitric oxide production. In addition, increased taurine/hypotaurine metabolism at the site of infection and linoleic acid in lymph nodes could motivate macrophage and T-cell activation against the parasite. In addition, arachidonic acid (AA)—an endocannabinoid metabolite with significant anti-inflammatory properties—showed an escalation in the course of infection in vivo. In general, the discovery of metabolic and immunological interactions following Leishmania vaccination could improve the development of innovative strategies in vaccine formulation (67). Given the endemicity of CL, a vaccine that prevents severe disease could have a significant impact on public opinion. However, a live attenuated vaccine that could also block parasite infection and thus prevent both cutaneous manifestations would have a much greater impact by reducing community transmission and potentially establishing herd immunity. Advances in molecular parasitology, creating deleterious gene mutations, altering replication fidelity, optimizing codons, and exerting control through genetic engineering tools, particularly the CRISPR/Cas9 system, which offers new ways to control L. major infection and replication, are renewing interest in a new generation of live attenuated vaccines, although potentially safer and more broadly applicable live vaccines require further testing before further advancing to human trials.
Conclusion
The spectrum of leishmaniasis varies due to host genetics and situation, parasite strain, and climate change. However, enough studies have shown that different forms of leishmaniasis can be prevented by vaccination. Unfortunately, there is currently no vaccine approved for human immunization on the global market. The development of an effective vaccine depends on its profitability for key stakeholders, vaccine developers, and manufacturers. Vaccine production requires a high level of trust in the public interest. Of course, government support attention to public health problems and international reflection are considered effective. Great advances have been made in the field of biological technologies to expand the range of vaccines. Recombinant multi-peptide adjuvanted vaccines such as Leish-F1 + MPL-SE and adenovirus-based DNA vaccines such as ChAd63-KH are now available. The priority of live attenuated Leishmania vaccines is considered to be a strong technique for the control of leishmaniasis, which has gained great attention due to the improvement of genetic engineering technologies such as the CRISPR/Cas system. The evaluation of gene candidates in terms of efficacy and immune response against the wild parasite has shown that Centrin1 is the most encouraging and is recognized as a good option for genetically live attenuated Leishmania vaccines. As we know, all the in vivo studies have been performed in animal models, which represent the early stages of the development of genetically attenuated vaccines and have not yet reached human clinical trials. In general, confirmation of logical guidelines related to live attenuated Leishmania development could administer a fine direction to major studies before handling human clinical trials and seriously reorganize the timeline of vaccine candidates.
Author contributions
ZR: Investigation, Visualization, Writing – original draft. HD-M: Conceptualization, Investigation, Project administration, Supervision, Validation, Visualization, Writing – review & editing. ME: Conceptualization, Investigation, Writing – review & editing. HK: Funding acquisition, Project administration, Resources, Supervision, Writing – review & editing.
Funding
The author(s) declare financial support was received for the research, authorship, and/or publication of this article. This work was supported by the Department of Genetics and Molecular Biology, School of Medicine, Isfahan University of Medical Sciences, Isfahan, Iran (Grant Nos.: 3401732 and 1401277).
Conflict of interest
The authors declare that the research was conducted in the absence of any commercial or financial relationships that could be construed as a potential conflict of interest.
Publisher's note
All claims expressed in this article are solely those of the authors and do not necessarily represent those of their affiliated organizations, or those of the publisher, the editors and the reviewers. Any product that may be evaluated in this article, or claim that may be made by its manufacturer, is not guaranteed or endorsed by the publisher.
References
1. Kaye PM, Cruz I, Picado A, Van Bocxlaer K, Croft SL. Leishmaniasis immunopathology-impact on design and use of vaccines, diagnostics and drugs. Semin Immunopathol. (2020) 42:247–64. doi: 10.1007/s00281-020-00788-y
2. Santos GA, Sousa JM, Aguiar AHBM, Torres KCS, Coelho AJS, Ferreira AL, et al. Systematic review of treatment failure and clinical relapses in leishmaniasis from a multifactorial perspective: clinical aspects, factors associated with the parasite and host. Trop Med Infect Dis. (2023) 8:430. doi: 10.3390/tropicalmed8090430
3. Karami M, Gorgani-Firouzjaee T, Chehrazi M. Prevalence of cutaneous leishmaniasis in the Middle East: a systematic review and meta-analysis. Pathog Glob Health. (2022) 117:1–10. doi: 10.1080/20477724.2022.2133452
4. Kaye PM, Matlashewski G, Mohan S, Le Rutte E, Mondal D, Khamesipour A, et al. Vaccine value profile for leishmaniasis. Vaccine. (2023) 41:S153–75. doi: 10.1016/j.vaccine.2023.01.057
5. Alawieh A, Musharrafieh U, Jaber A, Berry A, Ghosn N, Bizri AR. Revisiting leishmaniasis in the time of war: the Syrian conflict and the Lebanese outbreak. Int J Infect Dis. (2014) 29:115–9. doi: 10.1016/j.ijid.2014.04.023
6. Trájer AJ, Grmasha RA. The potential effects of climate change on the climatic suitability patterns of the Western Asian vectors and parasites of cutaneous leishmaniasis in the mid- and late twenty-first century. Theor Appl Climatol. (2024) 155:1897–914. doi: 10.1007/s00704-023-04726-4
7. Mutiso JM, Macharia JC, Kiio MN, Ichagichua JM, Rikoi H, Gicherub MM. Development of leishmania vaccines: predicting the future from past and present experience. J Biomed Res. (2013) 27:85–102. doi: 10.7555/JBR.27.20120064
8. Cecílio P, Oliveira F, Silva AC. Vaccines for human leishmaniasis: where do we stand and what is still missing? In:Afrin F, Hemeg H, , editors. Leishmaniases as Re-emerging Diseases. Croatia: IntechOpen (2018). doi: 10.5772/intechopen.75000
9. Volpedo G, Huston RH, Holcomb EA, Pacheco-Fernandez T, Gannavaram S, Bhattacharya P, et al. From infection to vaccination: reviewing the global burden, history of vaccine development, and recurring challenges in global leishmaniasis protection. Expert Rev Vaccines. (2021) 20:1431–46. doi: 10.1080/14760584.2021.1969231
10. Almeida GG, Coura FM, De Melo Barbieri J, Moura ACJ, De Oliveira Paes-Leme F, Da Costa-Val AP. FML/QuilA-vaccinated dogs naturally infected with Leishmania infantum: serum cytokines, clinicopathological profile, and parasitological parameters. Biomed Res Int. (2021) 2021:3192960. doi: 10.1155/2021/3192960
11. De Brito RCF, Cardoso JMO, Reis LES, Vieira JF, Mathias FAS, Roatt BM, et al. Peptide vaccines for leishmaniasis. Front Immunol. (2018) 9:1043. doi: 10.3389/fimmu.2018.01043
12. Skeiky YAW, Coler RN, Brannon M, Stromberg E, Greeson K, Thomas Crane R, et al. Protective efficacy of a tandemly linked, multi-subunit recombinant leishmanial vaccine (Leish-111f) formulated in MPL® adjuvant. Vaccine. (2002) 20:3292–303. doi: 10.1016/S0264-410X(02)00302-X
13. Chakravarty J, Kumar S, Trivedi S, Rai VK, Singh A, Ashman JA, et al. A clinical trial to evaluate the safety and immunogenicity of the LEISH-F1+MPL-SE vaccine for use in the prevention of visceral leishmaniasis. Vaccine. (2011) 29:3531–7. doi: 10.1016/j.vaccine.2011.02.096
14. Salari S, Sharifi I, Bamorovat M, Ghasemi Nejad Almani P. The immunity of the recombinant prokaryotic and eukaryotic subunit vaccines against cutaneous leishmaniasis. Microb Pathog. (2021) 153:104807. doi: 10.1016/j.micpath.2021.104807
15. Ojha R, Prajapati VK. Cognizance of posttranslational modifications in vaccines: a way to enhanced immunogenicity. J Cell Physiol. (2021) 236:8020–34. doi: 10.1002/jcp.30483
16. Almeida FS, Vanderley SER, Comberlang FC, Andrade AG, Cavalcante-Silva LHA, Silva E dos S, et al. Leishmaniasis: immune cells crosstalk in macrophage polarization. Trop Med Infect Dis. (2023) 8:276. doi: 10.20944/preprints202304.0903.v1
17. Sandoval Pacheco CM, Araujo Flores GV, Gonzalez K, De Castro Gomes CM, Passero LFD, Tomokane TY, et al. Macrophage polarization in the skin lesion caused by neotropical species of Leishmania sp. J Immunol Res. 2021:5596876. doi: 10.1155/2021/5596876
18. de Freitas e Silva R, von Stebut E. Unraveling the role of immune checkpoints in leishmaniasis. Front Immunol. (2021) 12:620144. doi: 10.3389/fimmu.2021.620144
19. Engelke L, Winter G, Hook S, Engert J. Recent insights into cutaneous immunization: How to vaccinate via the skin. Vaccine. (2015) 33:4663–74. doi: 10.1016/j.vaccine.2015.05.012
20. Bamorovat M, Sharifi I, Aflatoonian MR, Sadeghi B, Shafiian A, Oliaee RT, et al. Host's immune response in unresponsive and responsive patients with anthroponotic cutaneous leishmaniasis treated by meglumine antimoniate: a case-control study of Th1 and Th2 pathways. Int Immunopharmacol. (2019) 69:321–7. doi: 10.1016/j.intimp.2019.02.008
21. Rodrigues LS, Barreto AS, Bomfim LGS, Gomes MC, Ferreira NLC, da Cruz GS, et al. Multifunctional, TNF-α and IFN-γ-secreting CD4 and CD8 T Cells and CD8High T cells are associated with the cure of human visceral leishmaniasis. Front Immunol. (2021) 12:773983. doi: 10.3389/fimmu.2021.773983
22. Stanisic DI, Ho MF, Nevagi R, Cooper E, Walton M, Islam MT, et al. Development and evaluation of a cryopreserved whole-parasite vaccine in a rodent model of blood-stage malaria. MBio. (2021) 12:e0265721. doi: 10.1128/mBio.02657-21
23. Yeganeh F, Haji M, Hoseini M. Current approaches to develop a live vaccine against leishmania. Nov Biomed. (2017) 5:133–7. doi: 10.22037/nbm.v5i3.14942
24. Saljoughian N, Taheri T, Rafati S. Live vaccination tactics: possible approaches for controlling visceral leishmaniasis. Front Immunol. (2014) 5:134. doi: 10.3389/fimmu.2014.00134
25. Zabala-Peñafiel A, Todd D, Daneshvar H, Burchmore R. The potential of live attenuated vaccines against cutaneous leishmaniasis. Exp Parasitol. (2020) 210:107849. doi: 10.1016/j.exppara.2020.107849
26. Moreira POL, Nogueira PM, Monte-Neto RL. Next-generation leishmanization: revisiting molecular targets for selecting genetically engineered live-attenuated Leishmania. Microorganisms. (2023) 11:1043. doi: 10.3390/microorganisms11041043
27. Mitchell GF, Handman E, Spithill TW. Vaccination against cutaneous Leishmaniasis in mice using nonpathogenic cloned promastigotes of Leishmania major and importance of route of injection. Aust J Exp Biol Med Sci. (1984) 62:145–53. doi: 10.1038/icb.1984.14
28. Streit JA, Recker TJ, Filho FG, Beverley SM, Wilson ME. Protective immunity against the protozoan Leishmania chagasi is induced by subclinical cutaneous infection with virulent but not avirulent organisms. J Immunol. (2001) 166:1921–9. doi: 10.4049/jimmunol.166.3.1921
29. de Souza MC, de Assis EA, Gomes RS, Marques da Silva EA, Melo MN, Fietto JLR, et al. The influence of ecto-nucleotidases on Leishmania amazonensis infection and immune response in C57B/6 mice. Acta Trop. (2010) 115:262–9. doi: 10.1016/j.actatropica.2010.04.007
30. Gorczynski RM. Immunization of susceptible BALB/c mice against Leishmania braziliensis. Cell Immunol. (1985) 94:11–20. doi: 10.1016/0008-8749(85)90081-4
31. Rivier D, Shah R, Bovay P, Mauel J. Vaccine development against cutaneous leishmaniasis. Subcutaneous administration of radioattenuated parasites protects CBA mice against virulent Leishmania major challenge. Parasite Immunol. (1993) 15:75–84. doi: 10.1111/j.1365-3024.1993.tb00587.x
32. Kimsey PB, Theodos CM, Mitchen TK, Turco SJ, Titus RG. An avirulent lipophosphoglycan-deficient Leishmania major clone induces CD4+ T cells which protect susceptible BALB/c mice against infection with virulent L. major. Infect Immun. (1993) 61:5205–13. doi: 10.1128/iai.61.12.5205-5213.1993
33. Daneshvar H, Coombs GH, Hagan P, Phillips RS. Leishmania mexicana and Leishmania major: attenuation of wild-type parasites and vaccination with the attenuated lines. J Infect Dis. (2003) 187:8–14. doi: 10.1086/374783
34. Daneshvar H, Namazi MJ, Kamiabi H, Burchmore R, Cleaveland S, Phillips S. Gentamicin-attenuated Leishmania infantum vaccine: protection of dogs against canine visceral leishmaniosis in endemic area of Southeast of Iran. PLoS Negl Trop Dis. (2014) 8:2–8. doi: 10.1371/journal.pntd.0002757
35. Silvestre R, Cordeiro-Da-Silva A, Ouaissi A. Live attenuated Leishmania vaccines: a potential strategic alternative. Arch Immunol Ther Exp. (2008) 56:123–6. doi: 10.1007/s00005-008-0010-9
36. Gannavaram S, Torcivia J, Gasparyan L, Kaul A, Ismail N, Simonyan V, et al. Whole genome sequencing of live attenuated Leishmania donovani parasites reveals novel biomarkers of attenuation and enables product characterization. Sci Rep. (2017) 7:1–10. doi: 10.1038/s41598-017-05088-4
37. Goswami D, Minkah NK, Kappe SHI. Designer parasites: genetically engineered Plasmodium as vaccines to prevent malaria infection. J Immunol. (2019) 202:20–8. doi: 10.4049/jimmunol.1800727
38. Singer M, Frischknecht F. Time for genome editing: next-generation attenuated malaria parasites. Trends Parasitol. (2017) 33:202–13. doi: 10.1016/j.pt.2016.09.012
39. Kreutzfeld O, Müller K, Matuschewski K. Engineering of genetically arrested parasites (GAPs) for a precision malaria vaccine. Front Cell Infect Microbiol. (2017) 7:198. doi: 10.3389/fcimb.2017.00198
40. Roestenberg M, Walk J, Van Der Boor SC, Langenberg MCC, Hoogerwerf MA, Janse JJ, et al. A double-blind, placebo-controlled phase 1/2a trial of the genetically attenuated malaria vaccine PfSPZ-GA1. Sci Transl Med. (2020) 12:1–10. doi: 10.1126/scitranslmed.aaz5629
41. Elikaee S, Zarei Z, Khamesipour A, Akhoundi B, Borjian AR, Afshar MJA, et al. Live attenuated Leishmania major p27 gene knockout as a novel vaccine candidate: a study on safety, protective immunity and efficacy against canine leishmaniasis caused by Leishmania infantum. Acta Trop. (2022) 225:106153. doi: 10.1016/j.actatropica.2021.106153
42. Titus RG, Gueiros-Filho FJ, De Freitas LAR, Beverley SM. Development of a safe live Leishmania vaccine line by gene replacement. Proc Natl Acad Sci U S A. (1995) 92:10267–71. doi: 10.1073/pnas.92.22.10267
43. Amaral VF, Teva A, Oliveira-Neto MP, Silva AJ, Pereira MS, Cupolillo E, et al. Study of the safety, immunogenicity and efficacy of attenuated and killed Leishmania (Leishmania) major vaccines in a rhesus monkey (Macaca mulatta) model of the human disease. Mem Inst Oswaldo Cruz. (2002) 97:1041–8. doi: 10.1590/S0074-02762002000700019
44. Joshi PB, Kelly BL, Kamhawi S, Sacks DL, McMaster WR. Targeted gene deletion in Leishmania major identifies leishmanolysin (GP63) as a virulence factor. Mol Biochem Parasitol. (2002) 120:33–40. doi: 10.1016/S0166-6851(01)00432-7
45. Späth GF, Epstein L, Leader B, Singer SM, Avila HA, Turco SJ, et al. Lipophosphoglycan is a virulence factor distinct from related glycoconjugates in the protozoan parasite Leishmania major. Proc Natl Acad Sci U S A. (2000) 97:9258–63. doi: 10.1073/pnas.160257897
46. Ismail N, Karmakar S, Bhattacharya P. Sepahpour T. Leishmania major centrin gene- deleted parasites generate skin resident memory T-cell immune response analogous to leishmanization. (2022) 13:864031. doi: 10.3389/fimmu.2022.864031
47. Yoshii SR, Mizushima N. Monitoring and measuring autophagy. Int J Mol Sci. (2017) 18:1–13. doi: 10.3390/ijms18091865
48. Zhang WW, Karmakar S, Gannavaram S, Dey R, Lypaczewski P, Ismail N, et al. A second generation leishmanization vaccine with a markerless attenuated Leishmania major strain using CRISPR gene editing. Nat Commun. (2020) 11:3416. doi: 10.1038/s41467-020-17154-z
49. Mottram JC, Souza AE, Hutchison JE, Carter R, Frame MJ, Coombs GH. Evidence from disruption of the Imcpb gene array of Leishmania mexicana that cysteine proteinases are virulence factors (protease/parasite/trypanosomatid/transfection/null mutant). Cell Biol. (1996) 93:6008–13. doi: 10.1073/pnas.93.12.6008
50. Alexander J, Coombs GH, Mottram JC. Leishmania mexicana cystein proteinase-deficient mutants have attenuated virulence for mice and potentiate a Th1 response. J Immunol. (1998) 1810:6794–801. doi: 10.4049/jimmunol.161.12.6794
51. Saravia NG, Escorcia B, Osorio Y, Valderrama L, Brooks D, Arteaga L, et al. Pathogenicity and protective immunogenicity of cysteine proteinase-deficient mutants of Leishmania mexicana in non-murine models. Vaccine. (2006) 24:4247–59. doi: 10.1016/j.vaccine.2005.05.045
52. Mukherjee S, Xu W, Hsu FF, Patel J, Huang J, Zhang K. Sterol methyltransferase is required for optimal mitochondrial function and virulence in Leishmania major. Mol Microbiol. (2019) 111:65–81. doi: 10.1111/mmi.14139
53. Stewart J, Curtis J, Spurck TP, Ilg T, Garami A, Baldwin T, et al. Characterisation of a Leishmania mexicana knockout lacking guanosine diphosphate-mannose pyrophosphorylase. Int J Parasitol. (2005) 35:861–73. doi: 10.1016/j.ijpara.2005.03.008
54. Semini G, Paape D, Blume M, Fleur Sernee M, Peres-Alonso D, Calvignac-Spencer S, et al. Leishmania encodes a bacterium-like 2,4-dienoyl-coenzyme a reductase that is required for fatty acid β-oxidation and intracellular parasite survival. MBio. (2020) 11:1–19. doi: 10.1128/mBio.01057-20
55. Zufferey R, Allen S, Barron T, Sullivan DR, Denny PW, Almeida IC, et al. Ether phospholipids and glycosylinositolphospholipids are not required for amastigote virulence or for inhibition of macrophage activation by Leishmania major. J Biol Chem. (2003) 278:44708–18. doi: 10.1074/jbc.M308063200
56. Naderer T, Ellis MA, Sernee MF, De Souza DP, Curtis J, Handman E, et al. Virulence of Leishmania major in macrophages and mice requires the gluconeogenic enzyme fructose-1,6-bisphosphatase. Proc Natl Acad Sci U S A. (2006) 103:5502–7. doi: 10.1073/pnas.0509196103
57. Ortiz D, Sanchez MA, Pierce S, Herrmann T, Kimblin N, Archie Bouwer HG, et al. Molecular genetic analysis of purine nucleobase transport in Leishmania major. Mol Microbiol. (2007) 64:1228–43. doi: 10.1111/j.1365-2958.2007.05730.x
58. Manzano JI, Perea A, León-Guerrero D, Campos-Salinas J, Piacenza L, Castanys S, et al. Leishmania LABCG1 and LABCG2 transporters are involved in virulence and oxidative stress: functional linkage with autophagy. Parasit Vectors. (2017) 10:1–12. doi: 10.1186/s13071-017-2198-1
59. Mittra B, Laranjeira-Silva MF, Perrone Bezerra de Menezes J, Jensen J, Michailowsky V, Andrews NW. A trypanosomatid iron transporter that regulates mitochondrial function is required for Leishmania amazonensis virulence. PLoS Pathog. (2016) 12:1–28. doi: 10.1371/journal.ppat.1005340
60. Feng X, Tran KD, Sanchez MA, Al Mezewghi H, Landfear SM. Glucose transporters and virulence in Leishmania mexicana. mSphere. (2018) 3:e00349-18. doi: 10.1128/mSphere.00349-18
61. Tran KD, Vieira DP, Sanchez MA, Valli J, Gluenz E, Landfear SM. Kharon1 null mutants of leishmania mexicana are avirulent in mice and exhibit a cytokinesis defect within macrophages. PLoS ONE. (2015) 10:1–19. doi: 10.1371/journal.pone.0134432
62. Al Kufi SGJH, Emmerson J, Rosenqvist H, Garcia CMM, Rios-Szwed DO, Wiese M. Absence of DEATH kinesin is fatal for Leishmania mexicana amastigotes. Sci Rep. (2022) 12:1–12. doi: 10.1038/s41598-022-07412-z
63. Price HP, Paape D, Hodgkinson MR, Farrant K, Doehl J, Stark M, et al. The Leishmania major BB some subunit BBS1 is essential for parasite virulence in the mammalian host. Mol Microbiol. (2013) 90:597–611. doi: 10.1111/mmi.12383
64. Madeira Da Silva L, Beverley SM. Expansion of the target of rapamycin (TOR) kinase family and function in Leishmania shows that TOR3 is required for acidocalcisome biogenesis and animal infectivity. Proc Natl Acad Sci U S A. (2010) 107:11965–70. doi: 10.1073/pnas.1004599107
65. Padmanabhan PK, Dumas C, Samant M, Rochette A, Simard MJ, Papadopoulou B. Novel features of a PIWI-like protein homolog in the parasitic protozoan Leishmania. PLoS ONE. (2012) 7:e0052612. doi: 10.1371/journal.pone.0052612
66. Taheri T, Salmanian AH, Gholami E, Doustdari F, Zahedifard F, Rafati S. Leishmania major: disruption of signal peptidase type I and its consequences on survival, growth and infectivity. Exp Parasitol. (2010) 126:135–45. doi: 10.1016/j.exppara.2010.04.009
67. Volpedo G, Pacheco-Fernandez T, Holcomb EA, Zhang WW, Lypaczewski P, Cox B, et al. Centrin-deficient Leishmania mexicana confers protection against new world cutaneous leishmaniasis. NPJ Vaccines. (2022) 7:1–14. doi: 10.1038/s41541-022-00449-1
68. Tupperwar N, Shrivastava R, Shapira M. LeishIF4E1 deletion affects the promastigote proteome, morphology, and infectivity. mSphere. (2019) 4:e00625-19. doi: 10.1128/mSphere.00625-19
69. Corrales RM, Vaselek S, Neish R, Berry L, Brunet CD, Crobu L, et al. The kinesin of the flagellum attachment zone in Leishmania is required for cell morphogenesis, cell division and virulence in the mammalian host. PLoS Pathog. (2021) 17:1–29. doi: 10.1371/journal.ppat.1009666
70. Ishemgulova A, Hlaváčová J, Majerová K, Butenko A, Lukeš J, Votýpka J, et al. CRISPR/Cas9 in Leishmania mexicana: a case study of LmxBTN1. PLoS One. (2018) 13:1–17. doi: 10.1371/journal.pone.0192723
71. Duncan SM, Myburgh E, Philipon C, Brown E, Meissner M, Brewer J, et al. Conditional gene deletion with DiCre demonstrates an essential role for CRK3 in Leishmania mexicana cell cycle regulation. Mol Microbiol. (2016) 100:931–44. doi: 10.1111/mmi.13375
72. Duncan SM, Jones NG, Mottram JC. Recent advances in Leishmania reverse genetics: manipulating a manipulative parasite. Mol Biochem Parasitol. (2017) 216:30–8. doi: 10.1016/j.molbiopara.2017.06.005
73. Späth GF, Clos J. Joining forces: first application of a rapamycin-induced dimerizable Cre system for conditional null mutant analysis in Leishmania. Mol Microbiol. (2016) 100:923–7. doi: 10.1111/mmi.13374
74. Pickar-Oliver A, Gersbach CA. The next generation of CRISPR–Cas technologies and applications. Nat Rev Mol Cell Biol. (2019) 20:490–507. doi: 10.1038/s41580-019-0131-5
75. Uddin F, Rudin CM, Sen T. CRISPR gene therapy: applications, limitations, and implications for the future. Front Oncol. (2020) 10:1387. doi: 10.3389/fonc.2020.01387
76. Knott GJ. Doudna JA. CRISPR-Cas guides the futurepdf. Science. (2018) 361:866–9. doi: 10.1126/science.aat5011
77. Zhang WW, Matlashewski G. CRISPR-Cas9-mediated genome editing in Leishmania donovani. MBio. (2015) 6:e00861. doi: 10.1128/mBio.00861-15
78. Moon S Bin, Kim DY, Ko JH, Kim YS. Recent advances in the CRISPR genome editing tool set. Exp Mol Med. (2019) 51:1–11. doi: 10.1038/s12276-019-0339-7
79. Gao Y, Zhao Y. Self-processing of ribozyme-flanked RNAs into guide RNAs in vitro and in vivo for CRISPR-mediated genome editing. J Integr Plant Biol. (2014) 56:343–9. doi: 10.1111/jipb.12152
80. Piel L, Rajan KS, Bussotti G, Varet H, Legendre R, Proux C, et al. Experimental evolution links posttranscriptional regulation to Leishmania fitness gain. PLoS Pathog. (2022) 18:e1010375. doi: 10.1371/journal.ppat.1010375
81. Yagoubat A, Corrales RM, Bastien P, Lévêque MF, Sterkers Y. Gene editing in trypanosomatids: tips and tricks in the CRISPR-Cas9 era. Trends Parasitol. (2020) 36:745–60. doi: 10.1016/j.pt.2020.06.005
82. Goyal DK, Keshav P, Kaur S. Adjuvanted vaccines driven protection against visceral infection in BALB/c mice by Leishmania donovani. Microb Pathog. (2021) 151:104733. doi: 10.1016/j.micpath.2021.104733
83. Ayari-Riabi S, Ben khalaf N, Bouhaouala-Zahar B, Verrier B, Trimaille T, Benlasfar Z, et al. Polylactide nanoparticles as a biodegradable vaccine adjuvant: a study on safety, protective immunity and efficacy against human leishmaniasis caused by Leishmania major. Molecules. (2022) 27:8677. doi: 10.3390/molecules27248677
Keywords: attenuated vaccines, CRISPR, cutaneous leishmaniasis, drug resistance, leishmanization, immunization
Citation: Rooholamini Z, Dianat-Moghadam H, Esmaeilifallah M and Khanahmad H (2024) From classical approaches to new developments in genetic engineering of live attenuated vaccine against cutaneous leishmaniasis: potential and immunization. Front. Public Health 12:1382996. doi: 10.3389/fpubh.2024.1382996
Received: 06 February 2024; Accepted: 07 June 2024;
Published: 05 July 2024.
Edited by:
Chiara de Waure, University of Perugia, ItalyReviewed by:
Greta Volpedo, University of Genoa, ItalySarfaraz Ahmad Ejazi, University of Maryland, College Park, United States
Copyright © 2024 Rooholamini, Dianat-Moghadam, Esmaeilifallah and Khanahmad. This is an open-access article distributed under the terms of the Creative Commons Attribution License (CC BY). The use, distribution or reproduction in other forums is permitted, provided the original author(s) and the copyright owner(s) are credited and that the original publication in this journal is cited, in accordance with accepted academic practice. No use, distribution or reproduction is permitted which does not comply with these terms.
*Correspondence: Hossein Khanahmad, aG9zc2Vpbl9raGFuYWhtYWRAeWFob28uY29t; Hassan Dianat-Moghadam, ZGlhbmF0LmhAbWVkLm11aS5hYy5pcg==