- 1Molecular Oncology Research Center, Barretos Cancer Hospital, São Paulo, Brazil
- 2Chromatography Group, São Carlos Institute of Chemistry, University of São Paulo, São Paulo, Brazil
Introduction: Pollution has emerged as a significant threat to humanity, necessitating a thorough evaluation of its impacts. As a result, various methods for human biomonitoring have been proposed as vital tools for assessing, managing, and mitigating exposure risks. Among these methods, urine stands out as the most commonly analyzed biological sample and the primary matrix for biomonitoring studies.
Objectives: This review concentrates on exploring the literature concerning residual pesticide determination in urine, utilizing liquid and gas chromatography coupled with mass spectrometry, and its practical applications.
Method: The examination focused on methods developed since 2010. Additionally, applications reported between 2015 and 2022 were thoroughly reviewed, utilizing Web of Science as a primary resource.
Synthesis: Recent advancements in chromatography-mass spectrometry technology have significantly enhanced the development of multi-residue methods. These determinations are now capable of simultaneously detecting numerous pesticide residues from various chemical and use classes. Furthermore, these methods encompass analytes from a variety of environmental contaminants, offering a comprehensive approach to biomonitoring. These methodologies have been employed across diverse perspectives, including toxicological studies, assessing pesticide exposure in the general population, occupational exposure among farmers, pest control workers, horticulturists, and florists, as well as investigating consequences during pregnancy and childhood, neurodevelopmental impacts, and reproductive disorders.
Future directions: Such strategies were essential in examining the health risks associated with exposure to complex mixtures, including pesticides and other relevant compounds, thereby painting a broader and more accurate picture of human exposure. Moreover, the implementation of integrated strategies, involving international research initiatives and biomonitoring programs, is crucial to optimize resource utilization, enhancing efficiency in health risk assessment.
1 Introduction
Pollution currently poses a significant threat to humanity, necessitating the development of various biomonitoring techniques (1). Humans are exposed to a multitude of contaminants through air, dust, water, food, and personal care products, entering our bodies via ingestion, inhalation, or dermal absorption (2). Such exposures to complex combinations can lead to serious adverse effects, even when individual substances in mixtures are below safety limits, highlighting the need for a comprehensive understanding of combined human exposure for public health initiatives (3).
Several countries have established regulatory institutions to control and prevent pollution (4). However, only a few, including Germany, France, Israel, United States, and Canada, have successfully integrated human biomonitoring studies with health data (5). In this regard, health risk assessments play a crucial role in informing decision-makers on protecting human health and the environment (6, 7), with pesticide residues in food emerging as a particularly relevant concern (8).
While pesticides offer undeniable benefits to humanity, such as increased production and improved quality of life (9), these formulations also represent some of the oldest and most widely used environmental contaminants, posing severe toxicity issues necessitating decontamination strategies (10, 11). Risks associated with pesticide exposure range from short-term effects, like skin irritation and headaches, to chronic impacts such as cancer, Parkinson’s and Alzheimer’s diseases, asthma, and diabetes (12). Although the toxicological mechanisms are not fully elucidated, chronic pesticide exposure may involve different interactions than those targeting the pesticides’ primary intended use (13).
There are no population groups in the world unexposed to these compounds (14), but understanding these risks is challenging due to several factors, including the duration and level of exposure, type of contact, toxicity, persistence, and environmental characteristics (15). Therefore, studies employing direct and indirect determination methods for pesticide exposure have been conducted (16), with human biomonitoring enabling the measurement of personal exposure to specific chemicals, including both unmetabolized and metabolized compounds (17).
This is typically achieved through the analysis of biological samples like blood, plasma, serum, and urine, frequently complemented with oral fluid, hair, and nails, employing methods that must be fast, sensitive, and specific, emphasizing miniaturization and automation (18, 19). Technological advancements have led to increasingly sensitive detection methods capable of measuring pesticides accurately in parts per billion (ng·mL−1, or μg·kg−1), even with less expensive instrumentation and simplified approaches (20). Mass spectrometry, particularly in combination with chromatography, remains among the most selective, sensitive, and rapid analytical techniques for investigating organic contaminants in different sample matrices (21).
Urine stands out as the primary matrix in biomonitoring programs and cohort studies due to its non-invasive, painless, and easy collection process, making it ideal for studies requiring large participant numbers (22–24). However, these samples present challenges due to various interferents, prompting the development of numerous methods to address these issues (25). Taking pyrethroids as an illustration, reports of contamination in sediments, water, and crops have outnumbered those concerning humans (26).
Both endogenous and exogenous substances in biological samples contribute to matrix effects (MEs), a complex phenomenon with compound- and system-specific characteristics, requiring diverse management strategies (25). Depending on the sample matrix and analyte concentration, different sample preparation techniques may be necessary, such as dilution, protein precipitation, and extraction (27).
Understanding the environmental fate of pesticides, metabolites, and excreted forms is crucial for contamination assessment (28). In this regard, there are different groups of exposure biomarkers with general or specific associations with known contaminants (Figure 1). Different chemical classes, such glyphosate and 2,4-D frequently detected in urine, can be highlighted along with their respective metabolites AMPA and 2,4-dichlorophenol (Figures 1A,B). In contrast, paraquat is mainly excreted without modification (Figure 1C), while many active ingredients such as carbofuran, mancozeb, cypermethrin, chlorpyrifos, and acetamiprid (Figures 1D–H) are primarily monitored in humans through their biotransformation metabolites.
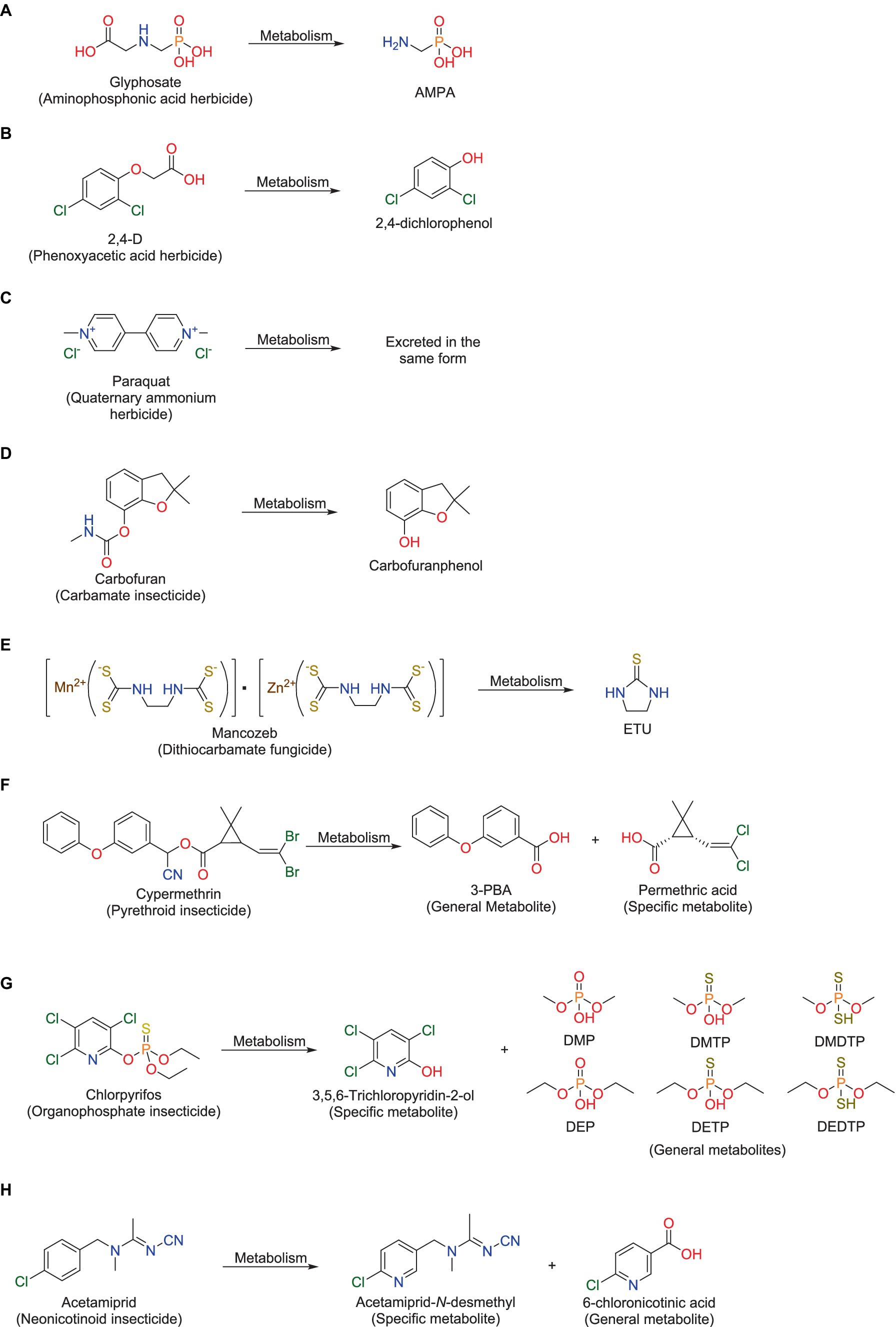
Figure 1. Few examples of active ingredients and their exposure biomarkers. (A) Glyphosate, (B) 2,4-D, (C) Paraquat, (D) Carbofuran, (E) Mancozeb, (F) Cypermethrin, (G) Chlorpyrifos, (H) Acetamiprid.
Some active ingredients, such as highly polar or ionic compounds like glyphosate, glufosinate, quaternary ammonium, and phenoxy acids, are often excluded from multi-residue methods and are instead addressed in more specialized methods (29). In this regard, it is crucial to highlight the widespread dispersion of these active ingredients in the environment, facilitated by their increased water solubility, emphasizing the need for new and broader analytical strategies (29).
New methodologies have emerged prioritizing microextraction techniques, which demand less solvent, labor, cost, and environmental impact (30). Consequently, traditional techniques like Liquid–Liquid Extraction (LLE) and Solid Phase Extraction (SPE), known for their time-consuming multistage operations and high solvent consumption, are no longer considered optimal (31). Recognizably, the analytical chemistry field now emphasizes eco-friendly alternatives and reduced use of harmful chemicals (32).
More recently, SPE cartridges utilizing few milligrams of stationary phase, have evolved into a well-established technique with new sorbents, experimental setups, and automation, facilitating widespread adoption and enabling various applications with reduced solvent use (33). Additionally, Solid-Phase Microextraction (SPME), involving thin fibers coated with a sorption material, and its derivatives like packed-in-tube solid-phase microextraction (IT-SPME), have gained attention (34, 35).
Another widely explored technique was the QuEChERS extraction method, known for its acronym representing Quick, Easy, Cheap, Effective, Rugged, and Safe, extensively utilized due to its efficient removal of matrix effects and high recovery rates of target analytes (36). Similarly, Dispersive Liquid–Liquid Microextraction (DLLME), a miniaturized LLE technique, found application in biological fluids (37), as Solidification of Floating Organic Drop Microextraction (SFODME), which involves solidifying a floating organic droplet (38). Nevertheless, exploring robotic and on-flow extraction methods was explored to mitigate labor-intensive and error-prone procedures (39).
Method validation is undeniably crucial in analytical chemistry. Therefore, several organizations have published guidance documents covering limits of detection and quantification, selectivity, specificity, confirmation of identity, robustness, linearity, precision, accuracy, trueness, matrix effect, quality control, and uncertainty, all vital for quality assurance (40, 41).
Thus, this review focuses on discussing literature concerning residual pesticide determination in urine, employing liquid and gas chromatography coupled with mass spectrometry from 2010 to 2022, emphasizing recent developments and trends. Furthermore, it delves into applications from 2015 to 2022, illustrating the identification of impacts and correlations of pesticide exposure with human health facilitated by these methodologies.
2 Scope of research
The literature search encompassed articles indexed in the Web of Science database from 2010 to 2022. Initial queries focused on key terms: “pesticide+urine+determination,” yielding 362 research articles; “pesticide+urine quantification,” yielding 251 research articles; and “pesticide+urine+chromatography,” yielding 621 research articles. Subsequently, these results underwent screening based on title and abstract criteria, targeting articles employing chromatography-mass spectrometry systems for determinations. The refined literature was then categorized by the authors into Methods and Techniques, delineating method development in the utilization of Gas Chromatography–Mass Spectrometry (GC–MS, 19 articles), Gas Chromatography–Mass Spectrometry Tandem (GC–MS/MS, 11 articles), and Liquid Chromatography-Mass Spectrometry Tandem (LC–MS/MS, 46 articles). Moreover, a separated category titled Applications was established aiming to derive meaningful and conclusive results for assessing exposure and health effects, comprising literature published between 2015 and 2022, and focusing on General Population Exposure (18 articles), Occupational Exposure (21 articles), and Reproductive Disorders and Early Stages of Life (38 articles).
3 Methods and techniques
The methods outlined were categorized based on the instrumentation employed for analysis: Gas Chromatography–Mass Spectrometry (GC–MS), Gas Chromatography–Mass Spectrometry Tandem (GC–MS/MS), and Liquid Chromatography-Mass Spectrometry Tandem (LC–MS/MS). Refer to Figure 2 for an overview of the typical workflow incorporating these prevalent techniques.
3.1 GC–MS
GC–MS has been employed for quantification of pesticide residues in urine, as outlined in Table 1. While this technique exhibits reduced selectivity compared to GC–MS/MS, it stands as a cost-effective alternative for determining residues from various active ingredients in multi-residue methods, including metabolites of organophosphates, pyrethroids, triazoles, and aminophosphonic acids (48, 55, 60).
Dialkylphosphates (DAPs), general metabolites of organophosphate insecticides, have been the subject of investigation in several studies utilizing GC–MS (43). Given their considerable solubility in water, extraction techniques utilizing solvents and solid phases with significant polarity have been extensively employed, followed by derivatization for analysis, as evidenced in different studies (43, 44).
A method specifically devised for the determination of four DAPs [dimethylphosphate (DMP), dimethylthiophosphate (DMTP), diethylphosphate (DEP), and diethylthiophosphate (DETP)] was developed for biomonitoring studies, employing LLE, derivatization with 2,3,4,5,6-pentafluorobenzyl bromide (PFB-Br), and clean-up with primary-secondary amine and florisil. In this study, 25 unexposed and 25 occupationally exposed volunteers were assessed, yielding a detection frequency ranging from 88 to 100% (42). Subsequent investigations expanded the scope to include the determination of six DAPs (43).
Various alternative extraction methods have been explored in analytical research. For instance, Molecularly Imprinted Solid Phase Extraction (MISPE) using 4-vinyl pyridine as the functional monomer and ethylene glycol dimethacrylate as cross-linker was developed for determining DETP and diethyldithiophosphate (DEDTP), indicating potential for further investigation (44).
Another assessed extraction method was Solidification of Floating Organic Drop Microextraction (SFODME) with 2-dodecanol for analyzing the organophosphate chlorpyrifos (45). In another method, LLE with subsequent N-(tert-butyldimethylsilyl)-N-methyltrifluoroacetamide (MTBSTFA) derivatization was developed for quantifying specific metabolites of organophosphate pesticides, 3-methyl-4-nitrophenol and 4-nitrophenol, revealing higher contamination levels in workers compared to the general population (46).
Addressing a diversity of environmental contaminants from the organophosphate class, a method was developed with SPE using Isolute ENV+ and derivatization with MTBSTFA for determining 15 organophosphorus metabolites found in insecticides, flame retardants, plasticizers, and moth repellents, with notable compounds being DMP, DEP, and 2,5-dichlorophenol (47).
In the use class of herbicides, the aminophosphonic acid glyphosate is a widely employed active ingredient being the focus of extensive research, including with monolithic spin-columns. This pesticide was targeted using a C–C18 column extraction method, along with its co-formulant glufosinate, and the organophosphates fenitrothion, malathion, and phenthoate (48), later, a TiO-C18 column was also explored for this purpose (49). In another approach, a method employing reduction with sodium borohydride and C18 monolithic spin column extraction was developed for determining the quaternary ammonium herbicides diquat and paraquat, alongside fenitrothion (50).
In the case of pyrethroids, such as bifenthrin, permethrin, β-cyfluthrin, and fenvalerate, these compounds were quantified using a salting-out assisted LLE method. This approach offered simplicity and environmental friendliness, eliminating the need for specialized equipment during sample preparation (51). Additionally, a combination of salting-out assisted LLE followed by Dispersive Liquid–Liquid Microextraction Based on Solidification of Floating Organic Drop (DLLME-SFO) with 1-undecanol was proposed for different matrices (52).
While previous methods focused on the parent active ingredients, there has been a shift towards determining the general metabolite 3-phenoxybenzoic acid (3-PBA) due to its high excretion rate. Consequently, two pyrethroid metabolites, trans-chrysanthemum dicarboxylic acid and 3-PBA, were identified in diaper samples using acetone, followed by a subsequent extraction with tert-butyl methyl ether and derivatization with 1,1,1,3,3,3-Hexafluoroisopropanol (HFIP) (53).
A more comprehensive approach to pyrethroid assessment involved analyzing 7 metabolites using SPE with C18 cartridges, followed by derivatization with HFIP and N,N′-Diisopropylcarbodiimide (DIC). Furthermore, permethric acid, 3-PBA, and 4-fluoro-3-phenoxybenzoic acid were detected in 100% of the samples from 10 children (54).
For triazole fungicides, an interesting Vortex-Assisted Liquid–Liquid Microextraction (VALLME) method was employed, and active ingredients were detected in all 21 exposed volunteers (55). Moreover, another innovative extraction method using membrane-protected stir-bar-supported micro-solid-phase extraction, characterized with double-layered hydroxide/graphene, was explored for 15 organochlorine pesticides, demonstrating its potential as a pioneering strategy (56).
Several multiresidue methods have been developed to assess exposure to mixtures of active ingredients across various chemical classes. One such approach utilized DLLME-SFO extraction to quantify nine active ingredients from diverse categories (57). In another strategy, the determination of five organophosphates, one carbamate, two pyrethroids, and one insect growth regulator was proposed using QuEChERS extraction followed by Dispersive Solid Phase Extraction (d-SPE) with Enhanced Matrix Removal-Lipid sorbent (58).
In addition, the use of Disposable Pipette Extraction with reverse phase styrene divinylbenzene (DPX-RP) was suggested, with a future aim at automation for high-throughput analyses. This method successfully determined 5 carbamates, 1 carbamate metabolite, and 4 organophosphates (59). This focus on multi-residue analyses was evident in different studies, for example, 13 metabolites from various chemical classes were assessed using LLE with hexane and derivatization with MTBSTFA. As a proof of concept, detection frequencies ranging from 3 to 81% were observed across 30 actual samples (60).
In summary, GC–MS methods were developed to assess pesticide exposure across different chemical and use classes, with emphasis on commonly employed active ingredients (57). Initially, methods were tailored for specific classes such as organophosphates, pyrethroids, organochlorines, or triazoles, before multiresidue strategies were adopted to provide a broader overview (42, 51, 56).
Given that some methods did not employ enzymatic deconjugation, either by enzymatic or acid hydrolysis, this procedure is typically recommended for pesticide metabolites, although most active ingredients excreted in unchanged form may not require this step (49, 53, 54, 60).
Pursuing lower limits of quantification (LOQs) with enhanced accuracy and precision, sample preparation methods initially targeted specific properties of analytes chemical classes before broader methods were explored. For instance, SPE with Isolute ENV+ was effective for hydrophilic compounds, while QuEChERS, DPX-RP, or LLE were suitable for hydrophobic analytes (47, 58–60). Furthermore, various derivatization agents were employed based on the nature of the exposure biomarkers, including PFB-Br, MTBSTFA, and HFIP with DIC (44, 46, 54).
In an advanced strategy, GC–MS/MS has captured growing interest following recent progress that have expanded its availability while reducing acquisition costs. This technology offered improved selectivity and lower limits of quantification compared to GC–MS, becoming widely employed (61).
3.2 GC–MS/MS
Approaches employing GC–MS/MS have been developed to target a wide number of analytes in multi-residue methods (Table 2). For example, a method was established for the determination of 8 pyrethroid metabolites using LLE with hexane, followed by derivatization with MTBSTFA. Subsequently, this method was applied to biomonitor 38 individuals from the general population, achieving quantification frequencies ranging from 5 to 100% (62).
Another strategy involved using two distinct analysis and derivatization methods, exemplified in the determination of 9 pyrethroid metabolites. LLE with tert-butyl methyl ether (MTBE) was employed, followed by derivatization with N,O-bis(trimethylsilyl)trifluoroacetamide (BSTFA) and trimethylchlorosilane (TMCS) for method 1, and HFIP/DIC for method 2. For 50 children, detection frequencies between 38 and 100% were observed (63).
Furthermore, the quantification of the neonicotinoid biomarker 6-chloronicotinic acid and the general metabolite of pyrethroids 3-PBA was performed in another method, also utilizing LLE with methyl tert-butyl ether (MTBE), followed by derivatization with BSTFA. Among 30 children, quantification frequencies were 23% for 6-chloronicotinic acid and 93% for 3-PBA (64).
Expanding the scope of multiresidue determinations, a method for determining 5 organophosphates and 3 pyrethroid metabolites employed LLE with hexane and isopropanol, followed by SPE with weak cation exchange cartridges and derivatization with MTBSTFA. Detection frequencies between 25 and 80% were determined through biomonitoring 20 adults and 20 children, showing that different studies presented high quantification frequency for these active ingredients (65).
Broader methodologies were employed to assess environmental contaminants with potential impacts on human health. For instance, the determination of 7 phenolic endocrine disruptors, including 3 pesticide metabolites, utilized SPE with Isolute-101 and derivatization with MTBSTFA (66). Similarly, a method for biomonitoring 19 chlorophenols, including pesticide metabolites, also employed Isolute-101 but derivatization with BSTFA and TMCS (67). Afterwards, a method for determining 19 phenolic compounds, such as specific metabolites of organophosphates, carbamates, and other pesticides, was developed utilizing LLE with MTBE and derivatization with BSTFA, with an emphasis on discerning exposure between active ingredients from the same chemical class (68).
In a comparable approach, a method for analyzing 20 phenolic compounds, including 7 pesticide biomarkers, used LLE extraction with hexane and MTBE, followed by SPE with K2CO3-treated silica gel and derivatization with BSTFA. In addition, large volume injection was employed for achieving low detection limits. The analysis of 29 participants revealed an 85% detection rate for most analytes, with mean concentrations ranging from 0.01 to 185 ng·mL−1 (69).
Urine and hair analysis, employing a similar analytical method, aimed to determine 2 carbamates, 3 phthalimides, 2 triazoles, and 1 carboxamide biomarker using two analysis methods: Supported Liquid Extraction (SLE) with Chem Elut cartridge and SPE with Strata-X cartridge, both followed by derivatization with N-methyl-N-(trimethylsilyl)trifluoroacetamide (MSTFA) (70). GC–MS/MS with negative chemical ionization was utilized for the determination of 24 organochlorines, 1 organophosphate, 8 organophosphate metabolites, 5 pyrethroids, 6 pyrethroid metabolites, 2 carbamate metabolites, 4 PCBs, and 6 other pesticides for urine and hair analysis. While urine remains the primary matrix for biomonitoring, hair analysis represents exciting alternative for biomonitoring (71).
An integrated approach was adopted to develop a comprehensive biomonitoring strategy, utilizing both GC–MS/MS and LC–MS/MS techniques for analyzing 205 active ingredients. This approach included a shared sample preparation method based on Solid Phase Extraction (SPE) employing C18 Sep-Pak cartridges. The GC–MS/MS method encompassed 118 pesticides from diverse classes, while the LC–MS/MS analysis determined another 87 analytes (72).
Despite fewer methods having been described for GC–MS/MS compared to GC–MS and LC–MS/MS during the reviewed period, it is noteworthy that this determination strategy offers increased selectivity and lower limits of quantification compared to GC–MS (73). Therefore, these strengths were leveraged for the development of multi-residue methods encompassing pesticides of different chemical classes and environmental contaminants originated from several consumer products, resulting in methods covering more than one hundred analytes (66, 70–72).
GC–MS/MS offers attractive cost-efficiency in contrast to LC–MS/MS, primarily because the latter’s equipment tends to be more expensive, often necessitating more recent and complex instrumentation than gas chromatography (74–76). However, despite this advantage, GC–MS/MS does have limitations that constrain its application range. This include the need for analyte derivatization, considerations regarding thermal stability, and issues with volatilization, all of which are not required for the use of LC as the inlet method (77).
3.3 LC–MS/MS
A diverse range of LC–MS/MS methods have been developed for analyzing various classes of active ingredients. While exposure biomarkers characterized by significant hydrophobic properties have been thoroughly investigated using multi-residue methods covering a wide range of analytes (77, 78), compounds exhibiting notable aqueous solubility required more restricted approaches, particularly regarding the number of analytes included (79, 80). Detailed information regarding these methodologies is provided in Table 3.
The quantification of the hydrophilic active ingredient glyphosate, its derivative glufosinate, and the metabolite AMPA was accomplished using Ultra-High-Performance Liquid Chromatography-Electrospray-Triple Quadrupole (UPLC-ESI-TQ). This analytical approach involved derivatization with acetate/acetic anhydride and trimethyl orthoacetate. By integrating methylation and acetylation reactions, this method circumvented extraction steps, resulting in a streamlined sample preparation process (81).
Furthermore, the viability of derivatization with FMOC-Cl for determining glyphosate and AMPA was investigated, with testing conducted on 20 farmers. Notably, one participant consistently recorded a glyphosate value of 200 ng·L−1 on a spraying day (82). In another method for glyphosate and AMPA determination, high-resolution mass spectrometry (UPLC-Orbitrap) coupled with cold-induced phase separation and hydrophilic DPX was employed with interesting results (83).
An investigation into the utilization of a microbore hypercarb column, comprised of porous graphitic carbon, was also conducted for determining glyphosate and three of its metabolites. While this method was specifically applied for analyses in pig urine to monitor contamination through feed, its potential extends to broader biomonitoring applications (84).
In another study, a method was developed employing protein precipitation for glyphosate, glufosinate, diquat, and paraquat, aiming at urine and blood samples for forensics analyses using a C18 reversed phase column, which was designed to allow the alkyl phase to remain accessible in highly aqueous mobile phases (85). Conversely, for enhanced chromatographic separations, a method targeting the quaternary ammonium chlormequat was developed employing HILIC and SPE with Isolute HCX-Q, yielding satisfactory results (86).
The fungicide mancozeb, another hydrophilic active ingredient pertinent to biomonitoring studies, is notable for its primary evaluated metabolite, ethylenethiourea (ETU), which was determined through supported liquid extraction using diatomaceous earth, followed by High-Performance Liquid Chromatography-Atmospheric Pressure Chemical Ionization-Single Quadrupole (HPLC-APCI-SQ). In a study encompassing 261 individuals from the UK general population, a detection frequency of 46% was observed (87).
In a separate study focusing on ETU biomonitoring, alkaline hydrolysis and subsequent on-column extraction was employed with determination by HPLC-APCI-TQ. During dermal exposure experiments, 10% of the administered dose was detected in urine (88). Moreover, in the quest for biomarkers of pesticide exposure, a method for determining 5-hydroxythiabendazole was elaborated, aiming to assess exposure to the benzimidazole fungicide thiabendazole (89).
Regarding phthalimides fungicides like folpet and captan, an HPLC-APCI-TQ method for determining metabolites was developed, utilizing Solid Phase Extraction (SPE) with Oasis HLB cartridges. Four workers were evaluated during 5 days of occupational exposure, with concentrations ranging between LOD and 8.5 ng·mL−1 (90). Additionally, an HPLC-APCI-TQ method, coupled with SPE using the cation exchange cartridge Strata X-C, was employed to determine 7 atrazine metabolites, utilized in the USA National Health and Nutrition Examination Study (91).
Recent advancements in LC–MS/MS have facilitated the development of multi-residue methods for detecting DAPs without requiring derivatization, thereby streamlining sample preparation efforts. A method for determining DEP, DETP, DMP, and DMTP by UPLC-ESI-TQ employed two sequential SPE procedures with Oasis WAX cartridge and a C18 column. This method was utilized for biomonitoring 225 three-year-old children, with detection frequencies ranging from 80 to 100% and medians between 0.6 and 14.4 ng·mL−1 (92).
Another approach was also devised to simplify sample preparation for the determination of 6 DAPs. This method involved lyophilization followed by dissolution in acetonitrile. In a study conducted with 30 children from Hyderabad, India, the average concentration of these metabolites ranged from 0.06 to 12 ng·mL−1, suggesting widespread exposure to pesticides (93).
In a different investigation, HILIC mode was selected to achieve effective chromatographic separation of DAPs, prompting exploration of various methodologies. The determination was performed using SPE with Strata X-AW for extraction, employing a Luna HILIC column (94). Alternatively, LLE with diethyl ether and ethyl acetate, alongside salting-out assisted extraction with MgSO4 and NaCl, also adopting HILIC chromatography but with a Hypersil Gold HILIC column, was assessed for the same 6 DAPs (95). In a separate investigation, five DAPs were determined using VALLME with ethyl acetate and (NH4)2SO4, however employing an Inspire C18 column for separation (96).
Ion-pair chromatography employing tripropylammonium formate emerged as a promising approach for the determination of DAPs. This method involved SPE utilizing Strata-X-AW coupled with UPLC-ESI-Quadrupole Time-of-Flight Mass Spectrometry (QTOF). When applied to biomonitoring the Norwegian mother–child cohort, consisting of approximately 100 participants, the method yielded detection rates of 40% for DMP, 95% for DEP, 96% for DMTP, 50% for DETP, 15% for DMDTP, and 1% for DEDTP (97).
A more comprehensive biomonitoring approach for organophosphorus compounds involved 9 DAPs and 5 monoalkylphosphates, utilizing ion pair chromatography with tributylamine. Accessing 19 volunteers, the concentration of these compounds totaled 20 ng·mL−1 (99). Ion chromatography was also employed for separation of six DAPs and glyphosate, and the analysis of individuals adhering to an organic diet demonstrated an 80% detection frequency, while 78% detection was observed in 40 subjects with suspected glyphosate exposure (98).
Several methods have been developed for biomonitoring organophosphorus compounds derived from additional sources. One such method identified 6 DAPs, 9 organophosphate flame retardants, and 1 brominated flame retardant. Concentrations were found to be higher in a cohort of 145 firefighters compared to 158 individuals from the general population, ranging between 2 and 37 times greater, encompassing the primary active ingredients along with specific exposure biomarkers (100). As also observed in another work that quantified disulfoton and five of its metabolites, employing QuEChERS and d-SPE techniques (101).
Expanding the scope, an HPLC-APCI-TQ approach was employed to ascertain 4 organophosphate pesticides (acephate, methamidophos, dimethoate, and omethoate) alongside 2 metabolites of bisdithiocarbamate fungicides (ETU and 1-phenyl 2-thiourea). This method encompassed lyophilization, dichloromethane suspension, filtration, and acetonitrile resuspension (102). Furthermore, a methodology was introduced for the determination of formamidine pesticides (amitraz, chlordimeform, formetanate) and five of their metabolites, underscoring the necessity for robust methodologies concerning emerging active ingredients in biomonitoring studies (103).
Given the widespread indoor use of neonicotinoid insecticides, it is imperative to assess their presence in the general population, especially among infants. Consequently, a method was developed for the simultaneous determination of 9 parent compounds. Then, 10 children were analyzed, revealing detection frequencies ranging from 0 to 80% (104).
Various studies have delved into different life stages, including early childhood, to understand pesticide exposure in an expossome approach. One such method involved the determination of 6 neonicotinoid insecticides and one metabolite through solvent extraction of urine from diapers, followed by SPE and SLE. This approach, applied to a cohort of 50 diapered children, revealed detection rates of 78% for N-desmethylacetamiprid and 84% for dinotefuran (105).
In another study focusing on neonicotinoids, researchers employed an enzymatic hydrolysis technique coupled with online SPE to detect 6 neonicotinoids and 2 metabolites. Analyzing samples from 60 individuals from the general population, it was found that 95% were contaminated with 3-diethyl-carbamoyl benzoic acid and 83% with 3-ethyl-carbamoyl benzoic acid (106).
Optimization of extraction methods was explored using different SPE cartridges to determine 7 neonicotinoid insecticides and 4 metabolites. Bond Elut Plexa emerged as the preferred choice, with at least two analytes detected in each sample from an analysis of 20 healthy volunteers (107). Employing a different extraction strategy, a method utilizing LLE with ethyl acetate was developed for the quantification of 6 neonicotinoids and 4 metabolites, highlighting the importance of exploring diverse extraction techniques (108).
Evaluation of UPLC-Orbitrap with online sample preparation using TurboFlow was conducted for the determination of 7 neonicotinoid insecticides and 1 metabolite, leading to the development of a semi-automated method. This approach showcased the potential of such strategies for biomonitoring studies (109). UPLC-Orbitrap with Turboflow was also employed to determine 6 organophosphates and 3 pyrethroid metabolites, which was applied to 30 individuals revealing the detection of 4-nitrophenol, 2-diethylamino-6-methylpyrimidin-4-ol, and 2-isopropyl-6-methyl-4-pyrimidinol in 6 samples, alongside the detection of 11 other pesticides through untargeted analyses (110).
In a broader scope for pesticide exposure assessment using HPLC-ESI-TQ setup, a method was proposed for the determination of 9 metabolites of organophosphates, pyrethroids, phenoxyacetic acids, and DEET. This method employed enzymatic hydrolysis and SPE using Oasis HLB. The analysis of 101 samples from a cohort study revealed detection frequencies of 98% for 3-PBA, 91% for IMPY, 89% for TCP, 66% for 2,4-D, 11% for F-3-PBA, and 0% for 2,4,5-T (111).
Moreover, a parallel investigation employing a similar strategy developed a method to determine 12 metabolites from organophosphates, pyrethroids, and phenoxyacetic acids, utilizing enzymatic hydrolysis and semi-automated SPE with Oasis HLB (112). Furthermore, another study, employing enzymatic hydrolysis with Oasis HLB, scrutinized 13 pesticides and 13 metabolites, encompassing organophosphates, pyrethroids, neonicotinoids, a phenylpyrazol, and a triazole (113).
A wider HPLC-ESI-TQ method targeting 28 compounds including neonicotinoids, organophosphates, carbamates, a pyrazole, and their metabolites, was developed. It incorporated enzymatic hydrolysis followed by a simplified extraction with acetone and MgSO4, revealing ubiquitous exposure to the evaluated pesticides in the form of metabolites among 20 children in South China (114).
UPLC-Orbitrap analysis was also explored for the determination of 29 urinary metabolites from various chemical and use classes, offering the advantage of post-target retrospective identification of biomarkers (115). In addition, another UPLC-Orbitrap method examined 10 nitrosable pesticides using different extraction methods; protein precipitation, SPE, and QuEChERS, showcasing promising potential for future biomonitoring studies (116).
An investigation about sample preparation evaluated protein precipitation, SPE with C18, QuEChERS, and d-SPE with PSA ahead of UPLC-ESI-TQ analysis. In conclusion, protein precipitation emerged as the most efficient strategy for determining 9 active ingredients in a low sample volume of 50 μL, ideal for postmortem analyses and biomonitoring studies with limited sample availability (117).
Furthermore, a comparison of extraction methods for 20 pesticides spanning 12 different chemical classes was conducted. This study revealed recovery rates of 79, 70, and 63% for SPE, DLLME with choline chloride and sesamol, and DLLME with chloroform and acetonitrile, respectively. Although DLLME showed promise in terms of time and solvent use, SPE demonstrated superior extraction efficiency (118).
In a more complete analytical strategy, a complementary approach employing both GC–MS/MS and LC–MS/MS was developed, utilizing unified sample preparation using SPE with C18 Sep-Pak cartridges. Combined, a total of 205 pesticides were assessed (72). Also focusing on robust and extensive multi-residue methods, a very interesting UPLC-ESI-TQ method targeting 260 pesticides was introduced, addressing biomonitoring, clinical, and forensic studies (119).
In an alternative strategy, a retrospective semi-quantitative analysis with 263 theoretical pesticide metabolites was performed using UPLC-Orbitrap. A total of 26 compounds were identified in actual samples. These compounds presented a 4–18% detection frequency with estimated concentrations of 1.2–14.7 ng·mL−1, except for propachlor oxanilic acid, which reached 141 ng·mL−1 (120).
The evaluation of various pesticide residues and other contaminants can be performed using TOF detection. For instance, two distinct UPLC-QTOF methods were employed to detect 38 pesticide exposure biomarkers, in addition to nicotine and cotinine. A subsequent analysis of samples from 15 pregnant women unveiled that most of the identified compounds were metabolites rather than the primary active ingredient (121).
In a study utilizing HPLC-QTOF, 38 compounds were identified in urine samples without the need for sample preparation and extraction. These compounds included 12 pesticides from different chemical classes, 1 pesticide metabolite, 7 veterinary drugs, 5 parabens, 1 UV filter, 1 plastic additive, 2 surfactants, and 9 additional substances, aiming to provide a rapid and straightforward method for high throughput screening (122).
Broad urine analyses aimed at unspecific toxicological emergencies were also developed via UPLC-ESI-TQ. This method facilitates the identification and quantification of 40 different compounds, including analgesics, benzodiazepines, antidepressants, anticonvulsants, drugs of abuse, and pesticides like aldicarb and carbofuran. Therefore, this approach enables rapid identification of toxic substances in cases of poisoning (123).
Multi-class analyses represent an exciting and cost-effective strategy. One of these methods successfully determined 50 analytes, including pesticides and compounds from different applications, using SPE with OASIS HLB followed by three injections on UPLC-ESI-TQ utilizing different columns (Betasil C18, Hypersil Gold AQ, and Synergi Polar RP), highlighting the potential of this reported approach (124).
Another biomonitoring method targeting various environmental pollutants was developed with a particular emphasis on pesticides. A total of 121 chemicals were addressed, including 45 plasticizers, 45 phenols, and 31 pesticides. The optimized method was validated using standard reference materials and proficiency test urine samples before analyzing 21 samples (125).
Methods employing LC–MS/MS as a determination technique were initially developed for singular or a few active ingredients and metabolites. Subsequently, multi-residue approaches for analytes from the same chemical group were introduced (119, 120). In recent years, strategies for determining pesticides from several classes have been assessed, and in some cases, contaminants from consumer products have been evaluated in the same analytical run, independently of employing Triple Quadrupole, Time of Flight, or Orbitrap spectrometers (120–125).
Unlocking the true potential of chromatography-mass spectrometry enables the analysis of numerous compounds of interest in high throughput (126). These advancements were made possible due to the expanded range of analytes at the LC inlet compared to the GC, which necessitates thermal stability (127). Furthermore, novel mass spectrometry ionization methods and direct sampling techniques have broadened the scope of applications, with the potential for further expansion (128).
4 Applications
Chromatography-mass spectrometry equipment has been utilized for the analysis of pesticide residues in urine samples, aimed at assessing potential health effects within a variety of population groups (Table 4 and Figure 3). These investigations are typically categorized into three main groups: assessments of exposure within the general population, evaluations of occupational exposure, and examinations focused on early stages of life.
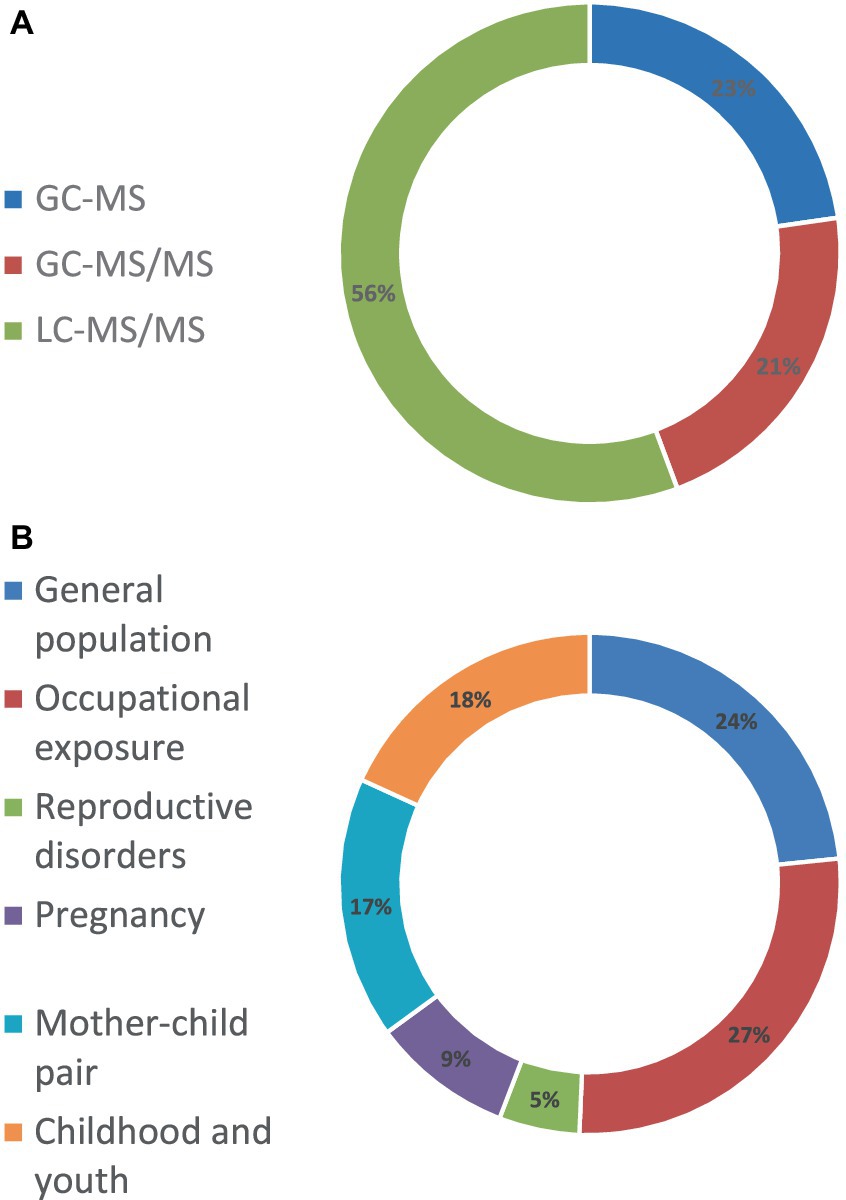
Figure 3. Distribution of literature analyzed based on the method of determination utilized (A) and the subject of the study (B).
4.1 General population exposure
Biomonitoring of the general population serves various purposes, such as assessing pharmacokinetics through residual pesticide determination in urine. For instance, a study evaluated dermal absorption from clothing treated with permethrin, commonly used as an insect repellent, revealing varying elimination rates among the six participants and establishing a half-life of 56 h (129).
Comparative analysis between urine and hair was another focal point in biomonitoring, especially concerning endocrine disruptors. A study employed repeated urine sampling to assess 16 phthalates, 4 bisphenols, and 8 pesticides. Findings suggested that while biomarkers were more frequently detected in urine, hair analysis yielded more consistent results (130).
The issue of public exposure to contaminants is increasingly pressing modern society, requiring a comprehensive global overview. In a small-scale study conducted in Kinshasa, Democratic Republic of Congo, involving 15 participants and pesticide determination. Urine analysis revealed detection frequencies exceeding 93% for glyphosate, 3-PBA, and TCP, with median concentrations of 0.2, 2.3, and 4.4 ng·mL−1, respectively (131).
Likewise, a pilot study conducted in Ireland delved into glyphosate exposure, unveiling a median concentration of 0.9 ng·mL−1 with a detection frequency of 20%, despite using a relatively elevated detection limit of 0.5 ng·mL−1 (133). In a separate exploratory investigation on glyphosate exposure in Portugal, encompassing 79 participants, a 73% detection frequency was noted, accompanied by a median concentration of 0.09 ng·mL−1. Furthermore, AMPA was detected in 97% of participants, with a median concentration of 0.10 ng·mL−1 (134).
Widespread exposure to low concentrations was observed in a study investigating neonicotinoid pesticides among 75 participants from Kumasi, Ghana. Approximately 92% of the participants were found to be exposed to multiple active ingredients (135).
Another study, conducted in North Carolina, United States, evaluated the exposure to pyrethroids in 50 residents over a six-week monitoring period. Analysis of urinary 3-PBA at weeks 1, 2, and 6 revealed a poor Intraclass Correlation Coefficient, indicating that a single measure of urinary 3-PBA was insufficient to characterize an individual’s average exposure effectively (136). Subsequently, examination using 24-h sampling demonstrated a positive association between exposure and factors such as outdoor activity, creatinine levels, and consumption of coffee and bread (137).
In a comparative study in North Carolina and New Jersey, United States, 30 individuals and their canine companions were enlisted to examine pesticide levels in both urine samples and silicone wristbands. The findings revealed that 7 out of 15 pesticide residues were detected in over 50% of the participants and their dogs, implying a parallel exposure to pesticides between humans and their animals (140).
A more comprehensive study involving 306 young men in Poland assessed four pyrethroid metabolites. Detection frequencies of 76% for permethric acid and 69% for 3-PBA were observed, with respective medians of 0.27 and 0.23 ng·mL−1. Higher exposure was associated with pyrethroid use in pet dogs and the consumption of seeds, nuts, and juice (138). In another investigation, 127 adult caregivers of children were assessed, revealing a detection frequency of 89% for various pesticides, with medians ranging from 0.3 to 3.4 ng·mL−1. Notably, exposure determinants varied for each active ingredient (139).
The exposure of Australian residents to organophosphates, pyrethroids, and phenoxyacetic acids was evaluated in a study involving 100 participants. Median concentrations for pesticides ranged from <0.1 to 36.8 ng·mL−1. Interestingly, for five organophosphate metabolites, higher exposure was observed in the youngest and oldest age strata (141).
Assessing urinary pesticide concentrations poses inherent challenges, particularly in studies involving large cohorts. However, this type of epidemiological study is widely recognized as the most appropriate method for obtaining reliable results and deriving meaningful conclusions. Consequently, methodologies for estimating exposure are pivotal in these investigations. In line with this, the Multi-Ethnic Study of Atherosclerosis conducted an assessment of organophosphate metabolite concentrations among its 4,466 participants. This endeavor produced consistent values, further validated within a subcohort comprising experimental data using GC–MS/MS for 240 individuals (142).
The effects of organophosphate pesticides on lung capacity were investigated in the Canadian Health Study cycle, a significant large cohort involving 4,446 participants. Residual urinary concentrations of DAPs were associated with reduced lung capacity, highlighting the adverse health effects associated with exposure to these pesticides (143). Subsequently, a global assessment was conducted with 322 participants from 8 different countries, targeting 10 analytes including 4 organophosphate metabolites, 2 phenoxyacetic acids, and 4 pyrethroid metabolites. Pesticide traces were detected in all urine samples with median ranging from 7.1 to 28.9 ng·mL−1. Regardless of regional exposure levels, pesticides represent a significant health issue that need prompt and effective intervention (144).
These large-scale studies afford the statistical power required to discern associations between pesticide exposure and health outcomes. In this vein, a study encompassing 2,116 participants from the general population of the United States was conducted to investigate pyrethroid exposure. Participants were stratified into tertiles based on 3-PBA medians of 0.1, 0.3, and 1 ng·mL−1. Exposure was correlated with overall mortality and cardiovascular disease mortality, although no discernible association with cancer was identified (145). Given the intricate nature of cancer as a broad and heterogeneous group of diseases, a detailed approach to its investigation is probably imperative.
The exposure of the United States population to neonicotinoid pesticides was examined in a cohort of 3,038 participants. Approximately half of the population had recently been exposed to at least one neonicotinoid pesticide. The 95th percentiles for N-desmethyl-acetamiprid and 5-hydroxy imidacloprid were determined to be 1.3 and 1.4 ng·mL−1, respectively, with young children and individuals of Asian descent exhibiting higher levels of exposure, although causal relationships remain unclear (146).
Socioeconomic characteristics have been extensively studied in relation to pesticide exposure associations. However, the impact of genetic variations, such as the polymorphism of the FLG gene encoding the filaggrin protein in the skin barrier, cannot be overlooked. For instance, null carriers of this gene were found to be more susceptible to skin exposure to pyrimethanil and two other environmental contaminants in dermal exposure experiments (147).
Research on the general population has predominantly centered on biomonitoring residual pesticide concentrations and identifying exposure determinants. These investigations aim to identify factors contributing to heightened exposure, encompassing objectives such as assessing dermal absorption, determining optimal sampling periods, utilizing urine and hair samples, and investigating the genetic dimensions interconnected with socioeconomic characteristics.
Notably, national and regional biomonitoring programs are the primary tools for assessing the general population and supporting chemical policies (208). These initiatives emphasize evaluating previously unidentified adverse effects of currently used pesticides and monitoring restricted or banned active ingredients (209). Additionally, they contribute to establishing human biomonitoring guidance values, which are crucial for identifying population groups at risk of negative health outcomes (210).
4.2 Occupational exposure
Occupational exposure to pesticides has been linked to a variety of adverse health effects, spanning noncommunicable diseases such as cancer, Parkinson’s disease, Alzheimer’s disease, as well as conditions like obesity and diabetes. In this context, extensive studies across various labor-related sectors are imperative to fully understand the scope and impact of these associations (211, 212).
4.2.1 Farmers and agricultural communities
Population groups have undergone scrutiny concerning pesticide exposure in connection with agricultural practices. For example, occupational exposure among 58 residents of an agricultural community engaged in tulip cultivation in Netherlands was examined for five pesticides. The detection frequencies of chlorpropham and tebuconazole were 94 and 86%, respectively, with median concentrations of 0.8 μg·g creatinine−1 and 0.5 μg·g creatinine−1. Moreover, heightened exposure was observed during the application season among both farmers and non-farming families, suggesting that proximity to spraying areas should be considered a risk factor (149).
Furthermore, exposure to miconazole, a triazole fungicide, was assessed by analyzing monohydroxyl-miconazole (PEN-OH) and carboxyl-miconazole levels in urine samples collected from 22 pesticide applicators before, during, and after spraying activities. PEN-OH was detected in all samples, with median concentrations ranging between 8.0 and 27.6 ng·mL−1, and significant occupational exposure associated with both current body burden and total exposure levels (150).
Mancozeb, another fungicide widely used in agriculture, was the focus of an occupational exposure assessment among tractor operators in Italian vineyards. Pre-exposure median concentration of ETU was measured at 0.6 ng·mL−1, escalating to 1.9 ng·mL−1 post-application. Exposure determinants included the type of tractor cabin (open or closed) and the usage of Personal Protective Devices such as coveralls and gloves (151).
Subsequently, a study aimed to compare estimation strategies for actual exposure by determining ETU levels in urine, clothes, skin pads, and hand wash. Findings indicated that estimations based on the actual exposure period in hours and the Fixed Fractional Approach correlated more closely with urine levels than hand washing. Notably, over 90% of the exposure was attributed to hand contact (152). Furthermore, the establishment of the Biological Exposure Limit of 10 μg ETU·kg of body weight−1 in the 24-h post-exposure urine was based on skin exposure data collected from field experiments, using patches and urine sampling from 16 participants (153).
A study addressing occupational exposure resulting from neonicotinoid spraying was also presented. Imidacloprid was detected in all 43 participants, with a pre-application median concentration of 2.79 μg·g creatinine−1, raising to 10.52 μg·g creatinine−1 post-application (154).
Some studies examined crucial historical moments, such as the assessment of glyphosate exposure among rural populations in Wisconsin, United States, in 1997 and 1998, following the introduction of glyphosate-resistant soybeans in 1996. Glyphosate and AMPA concentrations were determined, with medians of 4.0 ng·mL−1 for glyphosate and 4.1 ng·L−1 for AMPA. Remarkably, non-applicators showed no detectable glyphosate concentration, while applicators exhibited a detection frequency of 39%, underscoring the occupational nature of the exposure (155).
Furthermore, toxicokinetics of glyphosate were investigated in 59 Thai farmers before spraying, and up to 72 h post-application. Among one-time sprayers, the average urinary glyphosate concentration measured 27.4 ng·mL−1, markedly higher than values reported in other countries (156). Subsequently, the impact of glyphosate application on oxidative stress, inflammation, and lung function was scrutinized in other 180 Thai farmers. Serum analyses revealed elevated levels of malondialdehyde, indicative of oxidative stress, alongside reduced glutathione levels and compromised lung function parameters (157).
Amidst ongoing debates surrounding the health effects attributed to glyphosate, recent studies have shed light on the toxic impacts of its co-formulants polyoxyethyleneamine (POEA), leading to fatalities among rats in experimental setups, prompting significant concern (213, 214). Further investigations have revealed that both POEA and the isopropylamine (IPA) salt of glyphosate infusion can disrupt hemodynamics and result in fatalities in piglets, in contrast to glyphosate in NaOH base, which did not exhibit similar effects (215).
A diverse range of alternative surfactants different from the traditional POEA have emerged in glyphosate formulations. Within this context, a comparative cytotoxicity study conducted with human cell lines revealed that alkyl polyglucosides exhibited the least toxicity. Following closely were polyethoxylated alkyl phosphate ethers and quaternary ammonium surfactants, while polyethoxylated tallow amines showed relatively higher toxicity levels (216).
In addressing the specific instance of acute ocular toxicity reported among glyphosate applicators in the past, formulations have been introduced that combine POEA surfactants with compounds aimed at reducing eye irritation. However, opting for less aggressive surfactants, such as propoxylated quaternary ammonium surfactants, is considered the optimal choice (217). While co-formulants have traditionally been viewed as inert substances, multiple scientific studies have highlighted the combined toxicity of adjuvants, underscoring the imperative for further research into these supposedly inactive components (218).
Occupational exposure to organophosphates has garnered significant attention in recent studies. In one investigation conducted in agricultural communities in South Tyrol (Italy), 28 farmers and 43 non-farmers were assessed for exposure to chlorpyrifos. During the application season, both farmers and residents exhibited elevated levels of TCP, with levels of 6.80 μg·g creatinine−1 for farmers and 6.73 μg·g creatinine−1 for non-farmers, compared to the non-application season where levels dropped to 2.54 μg·g creatinine−1 and 3.22 μg·g creatinine−1, respectively. This observation suggests that indirect contact to spraying significantly contributes to the overall exposure (158).
In another study focusing on organophosphate pesticide sprayers, 80 applicators and a control group of 90 non-occupationally exposed participants from Greece were evaluated for 4 general metabolites. The median concentration of DETP was found to be 4.06 μg·g creatinine−1 in participants with allergic rhinitis compared to 1.97 μg·g creatinine−1 in those without, indicating a potential association between these compounds and increased case numbers (160).
Furthermore, an analysis of urinary concentrations of 6 specific organophosphate metabolites and two general pyrethroid metabolites was conducted on 48 farmers and 77 urban individuals from Spain. The findings revealed that farmers exhibited approximately twice the exposure to these pesticides compared to non-occupationally exposed populations, with concentrations for 2-(diethylamino)-6-methyl-4-pyrimidinol at 1.7 ng·mL−1 and 0.81 ng·mL−1, 4-nitrophenol at 2.3 ng·mL−1 and 1.3 ng·mL−1, TCP at 4.2 ng·mL−1 and 2.2 ng·mL−1, and 3-PBA at 2.4 ng·mL−1 and 1.1 ng·mL−1, respectively (161).
In a more complete approach combining urinary pesticide residue determination and epigenomic investigation, indigenous Huichol farmers in Mexico occupationally exposed to organophosphate pesticides were assessed by methylation profiling of CDKN2A and CDKN2B genes, alongside the determination of six general metabolites of organophosphates in urine samples. The study encompassed 160 farmers and 30 non-farmers with similar levels of DAPs in both, indicating that the primary source of contamination stemmed from their living environment or ingested food (164).
In a study conducted in Thailand, involving 79 pesticide sprayers, the pre and post-application seasons were evaluated for 6 organophosphate metabolites. Significantly elevated residue levels were noted during the spraying season, coinciding with markedly lower cognitive scores among the participants (162).
The determination of a variety of active ingredients was employed to tackle the epidemic of Mesoamerican nephropathy in northwest Nicaragua. This cohort encompassed 350 young adults from rural communities, identifying 3-PBA, 2,4-D, DCCA, ETU, hydroxy-tebuconazole, and 3,5,6-trichloro-2-pyridinyl at detection frequencies exceeding 90%, but data did not reveal any correlation between these exposure biomarkers and the incidence of this disease, showing that this exposure might be not related with this health condition (148).
In various studies, pesticide has been linked to the development of chronic diseases. Occupational exposure, characterized by prolonged contact with pesticides, has been notably associated with an elevated incidence of dementia, particularly Alzheimer’s disease (219). This correlation is underscored by the connection between pesticide-active ingredients and phenomena such as oxidative stress, neuronal impairment, and cognitive dysfunction (220). Notably, paraquat, a common active ingredient, has been prominently linked to an increased risk of Parkinson’s disease. At the same time, even rotenone, a plant-derived pesticide used by home gardeners, has been implicated in this neurological disorder (221).
It is crucial to recognize that oxidative stress, induced by many pesticides, is a common denominator in various health issues, including cancer and neurodegenerative diseases (222). Although the precise mechanisms connecting pesticides to cancer remain unclear, being likely influenced by complex factors, numerous epidemiological studies have observed associations between pesticide exposure and various human cancers (223).
Moreover, pesticide levels have been associated with congenital disabilities, reproductive abnormalities, and fatalities (224). However, establishing a definitive link between chronic diseases and pesticide exposure, like other environmental risk factors, often yields inconclusive findings. This inconsistency may be attributed to challenges in obtaining precise data regarding individuals’ exposure levels, which are frequently inferred rather than directly measured (225). However, employing a combination of both estimation and direct measurement in modeling would likely provide a more robust approach.
It is important to note that the presented literature highlights that farmers and agricultural communities face heightened exposure to pesticide concentrations, warranting special attention due to potential health ramifications stemming from this increased contamination.
4.2.2 Other occupations
Although much of the research documented in the literature has focused on agricultural communities, it’s important to recognize that other occupations face significant exposure to pesticides, including workers in flower-related industries and pest control.
The cultivation of flowers presents a high potential for pesticide contamination. For instance, a study conducted in Mexico assessed organophosphate pesticide exposure in 117 flower growers. The investigation found that over 90% of participants showed detectable levels. Notably, individuals working in greenhouses exhibited higher levels of exposure compared to those working outdoors, emphasizing the importance of personal protective equipment (165).
Similarly, occupational exposure among florists handling collected flowers was investigated in Belgium. Analysis of 56 workers revealed an average of 8 pesticide residues per urine sample, with an average total concentration of 4.3 mg.g creatinine−1. In contrast, the non-occupationally exposed group showed significantly lower levels at 2.0 mg.g creatinine−1. Therefore, florists exhibited higher concentrations and a greater variety of active ingredients in their exposure profiles (166).
Pest control workers were studied for their exposure to organophosphate insecticides. A study involving 230 Japanese employees explored gene polymorphism in relation to urinary concentrations of organophosphate metabolites. While no significant associations were found between metabolite concentrations and single nucleotide polymorphisms, there were noteworthy inverse associations observed between DAPs concentrations and the activities of fenitrothion oxidase and arylesterase (167).
Occupational exposure among amenity horticulturists was thoroughly examined, specifically targeting glyphosate. A cohort of 20 workers underwent monitoring via multiple spot urine samples. The resulting median glyphosate concentration was recorded at 1.9 ng·mL−1, with the peak excreted concentration observed after 3 h of exposure (168). Furthermore, associations were established between glyphosate and fluroxypyr urinary levels and various work-related factors, including breaks, extended tasks, and the utilization of controlled droplet applicators or boom sprayers (132).
Pyrimethanil and its metabolite 4-hydroxypyrimethanil were targeted for biomonitoring in both the general Swedish population and occupationally exposed horticulturists. Among 413 individuals, approximately 50% exhibited contamination, with a median hydroxypyrimethanil concentration of 0.1 ng·mL−1. In contrast, among 18 assessed horticulturists, a much higher detection frequency of 96% was observed, with a median concentration of 8 ng·mL−1 (169).
These findings suggest that various occupations can lead to increased contamination levels, as evidenced by the general population typically displaying lower concentrations of biomarkers for different active ingredients. In light of this, biomonitoring equivalents play a crucial role. These data are defined as the concentrations of chemicals in biological samples consistent with existing guidance values, such as reference doses, reference concentrations, minimal risk levels, or tolerable daily intakes, indicating the necessity for further assessment of exposure sources (210, 226, 227).
4.3 Reproductive disorders and early stages of human life
Pesticide exposure was also assessed with a focus on reproductive disorders and early stages of life, targeting various adverse health effects.
4.3.1 Reproductive disorders
The impact of pesticides on fertility prior to conception was investigated, particularly focusing on organochlorine pollutants and organophosphate pesticides in men and women. For example, the levels of op’-DDD (median: 0.018 ng·mL−1) and pp’-DDE (median: 0.044 ng·mL−1) in urine of 111 infertile men from Pakistan showed a negative correlation with sperm motility in men. While serum is typically used to assess organochlorine pesticide exposure, this alternative sampling can offer significant results (170).
In another investigation, involving 159 men from United States, exposure to organophosphate pesticides was assessed through the detection of 6 general metabolites, with DAPs being detected in all cases, averaging at 188 nmol·mL−1. Positive associations were found between DMTP, DMDTP, DEP, and DETP with sex chromosome disomy, whereas an inverse association was observed for DMP, suggesting the occurrence of complex relationships (171).
Both men’s and women’s infertility are equally significant and warrant attention. Thus, a cohort comprising 594 reproductive-age women from USA was analyzed to investigate the connections between endometriosis and 11 pesticides, known as endocrine disruptors. The odds ratios for endometriosis diagnosis notably escalated between the first and fourth quartiles of exposure to diazinon (OR = 1.89) and chlorpyrifos (OR = 1.65), underscoring a correlation between these pesticides and endometriosis (172).
Furthermore, exposure to organophosphate and pyrethroid pesticides prior to conception was evaluated in a cohort of 614 women in China. This study found that higher levels of DETP and 3-PBA were associated with prolonged time to pregnancy and increased risk of infertility (173). In the same context, an investigation also conducted in China examined the impact of organophosphates on in vitro fertilization outcomes. Women in the highest exposure quartile exhibited reduced odds of successful implantation, clinical pregnancy, and live birth (174).
These investigations highlight the significance of assessing pesticide exposure to reproductive health, revealing noteworthy adverse effects that require attention. These studies have shed light on various reproductive disorders discussed in the literature, implicating different active ingredients as endocrine-disrupting chemicals capable of prolonging the time to conceive, and increasing the risk of infertility and congenital disabilities in women (12). Furthermore, research suggests that pesticide exposure in males can adversely affect sperm and semen quality, leading to various reproductive disorders (14).
4.3.2 Pregnancy
Pregnancy represents a critical period of vulnerability to pesticide exposure, prompting focused investigation into its impact on gestation. Within this perspective, a study examined sampling periods to assess 6 general metabolites of organophosphates in a cohort of 62 pregnant women. Median concentrations ranging from 0.05 to 3.6 ng·L−1 were observed, with afternoon sampling showing stronger correlation with multiple sequential samples than first-morning void, suggesting its preferential consideration (175).
The variability of excretion for non-persistent chemicals and optimal urine sampling strategies were also assessed in a cohort comprising 154 pregnant women and 152 children (8 years old) from various European countries. Phthalates, phenols, DMTP, and DEP were detected in over 87% of samples, and the sampling scheme indicated that three daily pools of two urine samples each were sufficient for weekly exposure determination, resulting in reduced variability for biomonitoring (182).
Another pertinent area of inquiry was identifying exposure determinants, particularly during pregnancy among occupationally exposed farmers. This investigation involved 50 participants in Thailand and conducted a serum metabolome-wide association study on chlorpyrifos exposure. The median urinary concentration of TCP was found to be 2.39 ng·ml−1. Additionally, exposure correlated with indicators of oxidative stress, cellular damage and repair, as well as systemic inflammation, potentially posing adverse health effects on the fetus (176).
In a comprehensive investigation, a study evaluated the exposure of 573 Spanish pregnant women to 4 general and 4 specific metabolites of organophosphate pesticides. The detection frequency of these metabolites ranged from 6 to 59%, with a median concentration sum of 3 ng·mL−1. Subsequently, exposure was associated with factors such as fruit and vegetable intake, pre-pregnancy body mass index, and smoking habits during pregnancy (177).
Furthermore, the assessment of pyrethroid exposure was conducted on 480 pregnant women from non-rural areas of Yunnan, China. Detection frequencies for 4F-PBA, 3-PBA, and DBCA were 96, 90, and 72%, respectively, with a total pyrethroid median of 1.38 ng·mL−1. In addition, exposure positively correlated with the consumption of bananas, oranges, and the variety of fruits regularly consumed (178).
Exposure to both organophosphates and pyrethroids insecticides was investigated in a cohort of 30 pregnant Taiwanese women. The detection frequency of DMTP was 33%, with a median concentration of 1.19 ng·mL−1, while DCCA, a pyrethroid metabolite, showed a detection frequency of 73% with a median concentration of 11.31 ng·mL−1. Correlations between parent compounds in blood and their metabolites in urine indicated regular exposure, corroborated by the known use of these active ingredients in domestic insecticide products (179).
Further studies encompassing a wider array of contaminants were conducted. For example, 200 pregnant women from Denmark were assessed for exposure to parabens, phthalates, and phenols, including two metabolites of phenoxyacetic herbicides. Detectable levels were observed in nearly all participants, suggesting multiple combined exposures. Therefore, current risk assessments often overlook simultaneous exposure to several contaminants, potentially underestimating the health effects on fetuses (180).
Within the sphere of health effects, hypertensive disorders are among the leading causes of maternal and neonatal mortality and morbidity. Thus, a study involving 152 pregnant women from three European regions investigated blood pressure alterations and exposure to non-persistent chemicals. Although detection frequencies for organophosphate metabolites ranged from 66 to 98%, with medians of 1.4–3.9 μg·g creatinine−1, disorders were not associated with these exposures, showing that some hypothesis may be rejected by data (181).
The vulnerability of fetuses to environmental contaminants, including pesticides, has sparked concerns regarding exposure to various chemicals, making it an exceptionally pertinent research area that demands careful attention. Moreover, pregnancy represents a unique period characterized by biological changes that can heighten susceptibility to environmental chemicals and increase health risks for women (228).
Within this framework, pesticides have been associated to the promotion of inflammation and oxidative stress, resulting in membrane damage, protein dysfunction, and DNA impairment. In addition, specific active ingredients have been identified as endocrine disruptors with the potential to impact fetal growth (229). Therefore, an increasing necessity exists for studies to thoroughly investigate the health impacts of pesticide exposure on pregnant women. Currently, these investigations have primarily concentrated on growth, immunological and neurobehavioral development, respiratory function, and hormonal balance (230).
4.3.3 Childhood and youth
Children’s exposure to pesticides is a critical issue, especially during early childhood when even minimal exposure can have profound effects. Regarding this, a study encompassing 116 Japanese children investigated toddlers’ exposure to organophosphate pesticides through analysis of urine from diapers. Detection frequencies ranged from 49 to 100% for each DAP, with a median concentration of 137.6 nmol·L−1 (183). Subsequently, a larger study involving 1,037 toddlers reported a total median concentration of 120 nm·L−1. In conclusion, household products such as herbicides, insect repellent sprays, fragrances, and deodorants were identified as associated factors with DAPs levels (184).
The exploration of exposure determinants has been a focal point in certain studies focusing on children. In an investigation involving 222 children, both hair and urine analyses were employed to evaluate 4 DAPs. This study unveiled a detection frequency of 46% for at least one metabolite in urine and an impressive 99% in hair samples. Although distinct determinants were noted for hair analysis, none showed associations with urinary levels, except for age. Therefore, according to these data, the inclusion of hair analyses should be deemed essential in biomonitoring initiatives (185).
An assessment of 7 neonicotinoids focused on their use against pine wilt disease, which affects trees. Thus, 46 children in Nagano, Japan, were evaluated with 100% detection of neonicotinoid residues in urine. Air particulate and exposure association analysis suggested that the high detection frequency was primarily linked to dietary habits, rather than thiacloprid spraying in nearby areas (189). Regarding food intake, the impact of an organic diet was analyzed by assessing 23 compounds in 50 children from California, USA, over 16 consecutive days. While reduced concentrations of 2,4-D and organophosphate metabolites were observed due to ingestion of organic food, pesticide residues were still present, indicating other routes of exposure (190).
Glyphosate exposure was evaluated in 95 children and adolescents from agricultural communities in Chapala Lakeshore, Mexico. The detection frequency was 100% with a median of 0.37 ng·mL−1 for children from 6 to 16 years, and 0.03 ng·mL−1 for those between 3 and 6. In addition, season and age were significantly associated with determined contamination levels (192).
Furthermore, an investigation into the exposure determinants on agricultural communities involved the biomonitoring of glyphosate and its metabolite AMPA, in 149 children and 97 adolescents in Prekmurje, Slovenia. The detection frequencies were 27% for glyphosate and 50% for AMPA. The 95th percentile concentrations were ≤ 0.21 ng·mL−1 for glyphosate and ≤ 0.33 ng·mL−1 for AMPA. Increased exposure was correlated with the consumption of nuts and wholegrain rice, as well as elevated urinary levels of arsenic, lead, cobalt, zinc, and copper, indicating potential combined exposures (193).
The pyrethroid general metabolite 3-PBA was monitored in 80 children aged 2–3 years from Bangkok, Thailand, with a median concentration of 1.46 ng·mL−1 and a detection frequency of 92%. Exposure was linked to cypermethrin concentration in hand wipes, walking barefoot in the household, and gender (186). Subsequent research on the same cohort revealed a negative correlation between the median concentration of 3-PBA and gamma-aminobutyric acid, an inhibitory neurotransmitter, suggesting potential neurological consequences (187).
Also approaching health effects, a case–control study involving 40 children assessed the levels of exposure in participants with autism spectrum disorder (n = 21) compared to a control group (n = 19). The levels of 3-PBA were marginally higher in the case group, indicating a potential association, however a larger experimental design would be necessary for definitive conclusions (188).
Given that neonicotinoids bind to mammalian nicotinic acetylcholine receptors, researchers assessed the presence of 9 active ingredients and 4 metabolites in the cerebrospinal fluid (CSF) of 14 children and adolescents. Notably, 93% of CSF samples contained N-desmethyl-acetamiprid. Therefore, the broad exposure and detection of neonicotinoids even in CSF highlight the need for exposome studies to comprehend the risks of childhood cancers and other health consequences (191).
In a more comprehensive cross-sectional study encompassing 281 children under the age of 15 from two agricultural communities, Agua Caliente and Ahuacapán at Mexico, exposure to 16 pesticides was thoroughly investigated. Remarkably, every sample revealed detectable levels of at least two pesticides. Consequently, it is paramount to address potential long-term health effects stemming from concurrent exposure to diverse active ingredients (194).
An interesting temporal analysis was undertaken among Swedish adolescents, investigating 14 pesticides across the years 2000, 2004, 2009, 2013, and 2017. Despite participants displaying relatively low concentrations, with medians falling below 3.9 ng·mL−1, upward trends were discerned for 3-PBA, chlorpyrifos, pyrimethanil, and tebuconazole. Conversely, diminishing values were observed for chlormequat, thiabendazole, and ETU. These findings suggest a shift in pesticide usage habits and, consequently, exposure patterns (195).
Several studies have demonstrated that children and teenagers are exposed to various active ingredients. However, further research is needed to understand the associations with health outcomes and the impacts of observed contamination levels. Exposure biomarkers have significantly contributed to the study of pesticides and other environmental pollutants on childhood health, complementing traditional assessment methods such as questionnaires (231). Importantly, research suggests higher levels of exposure in children, possibly due to their smaller body mass, faster metabolism, increased ingestion of non-food items, and more outdoor activities on the ground, particularly in households where parents are occupationally exposed (232).
4.3.4 Mother–child pairs
Mother–child cohorts have emerged as a crucial strategy in numerous studies aimed at investigating the health impacts on offspring, particularly in assessing exposure to environmental contaminants. For example, a study conducted in Central Indiana, United States, examined glyphosate exposure in 71 pregnant women, uncovering a detection frequency of 93% and a median concentration of 3.3 ng·mL−1. Notably, this exposure was significantly associated with shortened gestational lengths, underscoring its potential impact on maternal and fetal health (196).
In another example highlighting associations with health effects. In-utero exposure to atrazine was examined in a case–control study involving 369 mother–child (girls) pairs at 12 weeks. The metabolite diaminochlorotriazine (DACT) was detected in 58% of cases and was linked with early menarche, providing further insight into the potential impacts of environmental contaminants on reproductive health (197).
Assessment of organophosphate exposure during pregnancy was carried out in 50 mother–child pairs from Thailand. The median DAPs concentration at week 28 of gestation was 85.5 nmol·L−1, with a detection frequency of 96%. It is important to note that this exposure was correlated with reduced neuromotor scores at five months of age (198).
Evaluating a larger cohort, prenatal and postnatal exposure to the organophosphate chlorpyrifos was investigated in 377 mother–child (3-year-old) pairs from agricultural communities in Jiangsu province, China. Median concentrations of TCP in the urine of mothers and children were 5.4 ng·mL−1 and 5.3 ng·mL−1, respectively. In this study, postnatal exposure was negatively associated with children’s motor and social development, particularly in boys (199).
Similarly, an examination of 310 mother–child pairs with 2-year-old children, also from China, uncovered heightened risks of adverse neurodevelopmental effects. These findings emphasize the pressing need for public policy intervention to mitigate the deleterious impacts of DAPs on children (200). In contrast, in an evaluation involving 231 French mother–child pairs, determination of 6 DAPs at 19 weeks’ gestation and one sample from the child at 6 years old did not reveal clear detrimental effects due to organophosphate exposure, indicating the need for additional research (201).
Furthermore, the association between exposure to organophosphate pesticides and traits of autism spectrum disorder was examined in 601 mother–child pairs from the agricultural Salinas Valley in California, United States. Prenatal concentrations of DAPs were associated with poorer reported social behavior, indicating a potential link between organophosphate exposure and neurodevelopmental disorders (202).
Contrastingly, a study involving 708 mother–child pairs from Generation R in Rotterdam (Netherlands) did not consistently observe an association between prenatal DAPs concentrations and the intelligence quotient of nonverbal children at 6 years old (203). Also from Rotterdam, 784 mother–child pairs were evaluated based on maternal urinary concentrations of 6 general metabolites of organophosphate pesticides during gestation. Despite a median DAPs metabolite concentration ranging from 308 to 316 nmol·g creatinine−1, no significant association was found between attention deficit hyperactivity disorder and autistic traits during a 10-year follow-up (204).
In the Columbia and Mount Sinai birth cohorts in the United States, prenatal exposure to organophosphates was assessed through DAPs determination in relation to fetal growth. The findings revealed that DAPs concentrations were linked to decreased birth length among non-Hispanic black women (206). Moreover, in the Generation R study in Rotterdam, prenatal exposure to organophosphates was significantly associated with decreased fetus in utero weight and length, although not at delivery, suggesting intricate correlations (205).
A study conducted in Scheyang County, China, involving 1,100 pregnant women investigated the exposure to the carbamate carbofuran and its associations with birth outcomes. The median carbofuranphenol concentration obtained was 0.8 ng·mL−1, with a detection frequency of 100%. Exposure to carbofuran was found to be negatively associated with neonatal head circumference in boys, while positively correlated with the neonatal ponderal index (207).
Research has demonstrated that pesticides can permeate both the placental barrier and the fetal blood–brain barrier, thereby impacting the developing fetus when the mother is exposed (233). Given the potential long-term health implications in both childhood and adulthood stemming from early exposure to environmental contaminants during pregnancy, it is imperative to conduct longitudinal studies to obtain meaningful and significant results (234). These findings add to the mounting evidence indicating that exposure to environmental contaminants during early life can hinder proper development (235).
Mother–child pairs form a cohort category wherein attaining a substantial participant count for enhanced statistical power can prove challenging, although specific cohorts already encompass more than 1,000 participants (236). Yet, this research strategy is not represented well in low-and middle-income countries (237, 238). Nevertheless, epidemiological studies have globally highlighted adverse health effects in children resulting from pesticide exposure, although further studies employing consistent methodologies are still necessary to mitigate inconclusive results (239, 240).
The literature highlights the necessity of cohorts with sufficient participant numbers and gold standard analyses to establish correlations, conduct health risk assessments, and perform meaningful statistical interpretations. However, this demand poses a significant obstacle to research, particularly in low-income countries with scarce resources. Nonetheless, collaborative efforts must prevail to facilitate a comprehensive global assessment of pesticide effects.
5 Synthesis and future directions
Chromatography coupled with mass spectrometry has emerged as a powerful tool for biomonitoring pesticides and organic contaminants across various sample matrices. Among these matrices, urine, blood, serum, and hair, have been consistently preferred choices (52). Urine sampling has particularly garnered attention in both specific assessments and large-scale biomonitoring programs (146). While other matrices such as blood, plasma, serum, and hair have also been explored for similar purposes, urine data have been extensively utilized in biomonitoring efforts, informing the development of public health policies (241). This preference may be attributed to the easiness of sample collection and processing, the wealth of available information from diverse geographical regions, and participants’ potential apprehension towards blood withdrawal.
Sample preparation has been a focal point in several studies, with an emphasis on deconjugation methods (242). Acid hydrolysis with HCl and enzymatic hydrolysis using Helix pomatia glucuronidase have been commonly employed, since the determination of free metabolites has been addressed in only a few studies (243). While acid hydrolysis is cost-effective, it’s important to note that it may induce reactions in parent compounds and byproducts, potentially altering biomarker profiles (244). Consequently, enzymatic deconjugation has gained traction in recent years due to increased product availability and the use of milder conditions (245).
Various extraction strategies have been explored as alternatives for pesticides analysis. Classical LLE and SPE were extensively utilized in the early 2010s (43, 46, 69). Subsequently, miniaturized SPE employing milligrams of sorbent, liquid-phase microextraction, and SPME gained attention (103, 125). QuEChERS (116) and d-SPE (58) emerged as trends in sample preparation, offering reduced solvent usage and simplified sample handling. Additionally, other strategies such as DPX (59), MISPE (44), SFODME (45), DLLME-SFO (57), and VALLME (55) have been investigated, each presenting distinct advantages in terms of efficiency and environmental sustainability. In summary, these diverse options reflect the abundance of options available in contemporary sample preparation methodologies.
The initial methods predominantly concentrated on analyzing individual or a restricted set of active ingredients, frequently belonging to the same chemical class. This strategy was preferred owing to the shared physicochemical properties, which favored sample preparation and chromatographic separation (13, 88). Conversely, developing comprehensive multi-residue methods posed distinct challenges, especially with outdated systems known for their limited separation efficiency and detection capabilities, ultimately undermining analytical performance.
Another suitable choice was the type of inlet system, GC or LC. Gas chromatography is frequently considered a more robust and cost-effective technique, but the requirement of high temperatures reduces the possibilities regarding the analyte range (246). Furthermore, derivatization was another issue that have impacted extensive studies for most of the biomarkers from non-persistent pesticides at GC–MS and GC–MS/MS analyses, since this step of sample preparation requires time, additional human resources, and/or sophisticated automation. Therefore, avoiding these disadvantages, LC–MS/MS methods have been reported in increasing numbers, notably in recent years (123).
In the pursuit of miniaturization, most analytical methods from the 2010s employed HPLC as the separation technique. In later years, there was a transition to core-shell technologies, replacing porous particles to enhance efficiency and speed (107, 119, 247). Subsequently, the development of UPLC methods utilizing columns with particles smaller than 2.1 μm, coupled with improved mass spectrometers, became extensively explored for evaluating a wide array of analytes, particularly in strategies aimed at critical exposure assessment (125).
Allied with these advancements and faster switching speed, the scope of analysis has broadened to encompass pesticides from various chemical classes. This expansion now includes environmental contaminants arising from diverse sources, such as plasticizers, nicotine biomarkers, polycyclic aromatic hydrocarbons, and personal care products (68, 122, 182).
However, a noticeable distinction emerges between biomarkers characterized by significant hydrophilic properties, such as ETU, quaternary amines, DAPs, and aminophosphonic acids (50, 98), and hydrophobic analytes like phenols, pyrethroids, neonicotinoids, organophosphates, pyrazoles, and triazines (72, 117, 118). This differentiation arises from the specificities inherent in sample preparation and chromatographic separation techniques, owing to the divergent physicochemical properties. Consequently, this leads to the development of distinct multi-residue methods for each group of analytes.
Literature highlights the vital significance of carefully choosing exposure biomarkers as analytes and crafting methodologies with LOQs below 1.0 ng·mL−1. This necessity arises from the observation that numerous studies revealed concentration medians hovering around this threshold (139, 141). Therefore, ensuring heightened detectability is imperative for achieving statistically significant findings capable of driving subsequent correlations. Furthermore, leveraging state-of-the-art equipment typically augments the frequency of quantification, even when employing the same overall methodology, as updated systems offer superior instrumental sensitivity.
Additionally, different urine sampling schemes have been explored to enhance data reproducibility. While most studies utilize the assessment of the first urine void, the afternoon void has also emerged as a compelling option (175). Furthermore, more extensive sampling strategies, including 24-h sampling and repetitive collections over different days and weeks, have been investigated (168). However, such procedures necessitate additional efforts from data collection teams, particularly in extensive cohort studies. Despite this, studies on contaminants suggest that single-spot urine sampling may adequately reflect average population exposure for large cohorts (248).
Alternative sampling methods were explored, such as wristbands and hair analyses. In spite of that, urine sampling is the most employed matrix for non-persistent pesticide residues at large biomonitoring programs (71, 130, 140). In this context, a crucial aspect of urine assessment is normalization, as various methods have been proposed (249). Notably, creatinine has emerged as the most used normalization option in studies aiming to identify exposure determinants and associations with health effects (149, 204). It is important to note that some studies adopt both approaches, considering the interpretation of pesticide residue concentration in urine and creatinine-adjusted values. Furthermore, other normalization strategies can be assessed, like specific gravity and combined modeling (250).
While LC-TQ prevail as the predominant tool owing to its heightened detectability, there has been exploration into utilizing high resolution mass spectrometry (HRMS), such as LC-TOF and LC-Orbitrap, for residual pesticide determination in urine, showing relevant detection limits (97, 109, 115). Their application in post-targeted and untargeted analyses warrants special attention, as they facilitate the identification and quantitative estimation of unknown or unpredicted biomarkers, offering relevant additional data (110).
Human biomonitoring programs play a pivotal role in acquiring data that can inform policymakers in responding to environmental and human health threats, as evidenced by the banning several toxic active ingredients like organochlorine pesticides under the Stockholm Convention (251). Additionally, other active ingredients, such as organophosphate insecticides parathion and methamidophos, carbamates like aldicarb and carbofuran, and herbicides as paraquat (quaternary biphenyl ammonium) and 2,4,5-T (chlorophenoxyacetic herbicide) have been widely banned or highly restricted due to their high toxicity, which has been linked to various health problems (252).
Herbicides like atrazine (triadimefon) and glyphosate (aminophosphonic acid) are also under intense scrutiny. However, it’s imperative to acknowledge that pesticides should be evaluated within a comprehensive picture of the agricultural system, as banned active ingredients may be replaced by even more harmful compounds (253, 254).
The outlook of banned and currently used pesticides can vary from one country to another, posing a challenge for biomonitoring methods and strategies. Notably, obsolete pesticides have been detected as legacy chemicals or due to inappropriate application in various studies (255–257). This diversity in active ingredients, physicochemical properties, and formulations creates a complexity barrier for elucidating exposure levels, further complicated by differences in sampling strategies, analytical methods, and data presentation, necessitating standardization for direct comparison (258, 259).
Significant progress has been achieved in international and national legal frameworks concerning pesticide management. However, many existing laws may not fully align with the requirements of agreements or regional initiatives aimed at harmonizing regulations. Additionally, these laws often need to adequately integrate with updated legislation about environmental protection, chemicals management, and related areas (260).
Broadly, developed nations tend to impose more stringent regulations than developing countries, leading to disparities in pesticide legislation that pose technical barriers to commercial relations (261). As the globalization of food systems advances, the sharing of risks associated with safety, including exposure to pesticide residues, becomes more pronounced (262).
Counterfeit pesticides exacerbate the issue by flooding the market with substandard products. These inferior items are created through the adulteration of raw materials, inadequate purification processes, and low-quality solvents and packaging. Consequently, they introduce harmful impurities such as ethylmethanesulfonate, isomalathion, or nonylphenol ethoxylates (263).
Additionally, the smuggling of pesticides contributes to the widespread dissemination of banned and restricted active ingredients within agricultural practices (264, 265). These illicit activities pose significant challenges to biomonitoring programs and lead to substantial crop losses and environmental hazards. This black market, estimated to be worth around $5.4 billion, perpetuates risks to human health and ecosystems (263, 266).
Moreover, developing new analytical methods is crucial for evaluating non-conventional pest control products that could be incorporated into future human biomonitoring initiatives, despite these substances generally being deemed low in toxicity. These include a range of biopesticide products, such as microbial, semiochemicals, and pheromones, as well as plant extracts and oils of vegetal and mineral origins, and other natural solutions like diatomaceous earth (267).
Another category to consider is Plant-Incorporated Protectants, such as Cry proteins and double-stranded ribonucleic acid expressed in genetically modified crops (268). Additionally, there is a growing interest in nanopesticides, where active ingredients are applied with nanocarriers such as polymers, clays, and zein particles, presenting a promising new approach that may be addressed in future biomonitoring (269).
The determination of residual pesticides in urine serves various purposes, encompassing toxicological studies, assessment of pesticide exposure among the general population, and occupational exposure among farmers, pest control workers, horticulturists, and florists (166, 167, 270). In addition, it sheds light on the consequences of pesticide exposure during pregnancy and childhood, impacts on neurodevelopment, and the occurrence of reproductive disorders (199, 271).
Furthermore, recent research has underscored the importance of evaluating adjuvants, counterions, and co-formulants among exposed populations (272). Since several studies have highlighted the toxic effects, particularly those of POEA, necessitating stricter regulations and accurate formulation descriptions (217, 218).
Pharmacokinetic and occupational exposure studies have revealed that dermal absorption significantly contributes to contamination, alongside ingestion and inhalation, resulting in elevated exposure levels (88, 129, 149, 150, 153). Moreover, data concerning the general population indicate widespread detection of pesticide exposure to various active ingredients, emphasizing the critical issue of mixture effects. This complexity increase the challenge of establishing human biomonitoring guidance values, which remain imperative (131, 141, 172, 210).
Genotyping holds immense potential for future studies, as specific polymorphisms can profoundly influence human pesticide exposure. For example, variations in dermal polymorphisms of the FLG gene, responsible for encoding the filaggrin protein, can have significant implications (147, 167). Thus, epigenomic assessments, such as studies on gene methylation, also offer promise as a valuable tool in human biomonitoring, offering reduced variability in assessments, as in the correlation between the CpG site of the CDKN2B gene and work time in farmers (164). Furthermore, occupational pesticide exposure has been extensively linked to genomic instability and other genetic and epigenetics aspects (273).
In the context of occupational exposure, pesticide spraying and residing in agricultural communities are particularly pertinent, especially given concerns about pesticide overuse (274). While historical data suggested limited glyphosate exposure primarily among those involved in spraying activities, recent studies indicate that residing in rural areas can significantly elevate exposure levels (38). Consequently, individuals in rural settings may exhibit double the pesticide residue concentrations compared to urban populations, potentially leading to associated health effects (149, 158, 160, 161).
Flower cultivation and gardening activities have been identified as occupations with significantly heightened exposure risks. For instance, working within greenhouses has been shown to pose a greater risk compared to working in open fields for flower cultivation (165). Additionally, florists have been found to have elevated pesticide residue levels compared to the general population, suggesting that flowers can serve as a potential source of contamination (166). In this context, a noteworthy strategy was determining Equivalent Biological Exposure Limit, which is an important data for biomonitoring at work environment (154).
Different studies have reported adverse health effects stemming from inadequate use of personal protective equipment (275), also requiring the determination of biomonitoring equivalents to identify risky incidents and scenarios (210). Prolonged exposure to pesticides, as seen in certain occupational settings, has been linked to various chronic diseases, as different forms of cancer, cardiovascular issues, pulmonary diseases, as well as Parkinson’s and Alzheimer’s disease, spark significant concerns regarding the health impacts on workers, particularly in advanced age (251, 276, 277). Thus, there is a pressing need for comprehensive investigations utilizing both direct and indirect methods of exposure level determination, as discussed herein.
The impact of pesticide exposure on fertility in both women and men deserves comprehensive evaluation, uncovering adverse effects. For instance, there is emerging evidence suggesting a potential correlation between exposure to diazinon and chlorpyrifos to the development of endometriosis (172). In men, heightened levels of pesticide residues were noted in individuals experiencing infertility, notably with HCB showing an association with reduced sperm motility (170). Furthermore, specific organophosphate metabolites have been positively linked to disomic rates, highlighting the significance of further research in this area (171).
The escalating global concern over the impact of pollution on children’s health and development prompted significant attention. In response, the Miami Declaration was formulated during the 1997 G8 Meeting, involving key nations such as the United States, Canada, France, the United Kingdom, Germany, Italy, Japan, and Russia. This landmark declaration was crafted with the intention of advancing research on children’s environmental health, emphasizing the necessity for biomonitoring studies and programs (278).
The susceptibility of fetuses to environmental contamination has been extensively assessed through the inclusion of pregnant women in research, with follow-up extending from birth into adolescence. Various studies have aimed to pinpoint exposure determinants to mitigate individual contamination. However, most findings have underscored associations with factors such as fruit and vegetable consumption, and body mass index. Thus, addressing these concerns ideally involves opting for organic foods and maintaining a balanced diet (177, 178).
Additionally, toddlers’ exposure has been evaluated through urine analysis from diapers. Regarding this, widespread exposure to organophosphates and other pesticides has been noted, alongside exposure determinants such as household products like pesticides, fragrances, and deodorants (183, 184). Regarding health effects, research has revealed associations between atrazine exposure during pregnancy and early menarche in offspring (196, 197). Similarly, glyphosate exposure has been significantly linked to shorter gestational periods, while prenatal exposure to carbofuran has shown adverse health effects in baby boys (207), emphasizing the relevance of such investigations.
Nevertheless, it’s crucial to recognize that certain hypotheses concerning adverse health effects have not been validated. For instance, exposure to organophosphate pesticides showed no correlation with lower blood pressure during pregnancy (181) or with ADHD and autistic traits in children (204). Conversely, prenatal exposure has been linked to adverse effects on infant cognitive and motor development (198, 199). Hence, it is imperative to implement diverse strategies and validation studies to confirm associations and draw comprehensive conclusions.
Recent advancements in chromatography-mass spectrometry for urine analyses have facilitated the development of multi-residue methods capable of simultaneously determining several pesticide residues from various chemical and use classes. These innovative approaches can be employed in studies investigating the health risks associated with exposure to mixtures, including pesticides and other relevant environmental contaminants, thereby providing a more holistic understanding of human biomonitoring.
The variability in urine sampling methods, coupled with the need for rigorous numerical treatment, highlights the critical importance of recruiting a substantial number of participants to ensure the attainment of statistically significant results. Moreover, it is imperative to validate these findings across diverse demographic groups to guarantee their robustness and applicability.
In this regard, integrated strategies that foster collaboration among international research groups and biomonitoring programs play an essential role. By leveraging such efforts, we can maximize the utilization of available resources and significantly enhance the efficiency of initiatives for health risk assessment.
Author contributions
WB: Writing – original draft, Writing – review & editing. FL: Writing – review & editing. AS: Writing – review & editing. HS: Writing – review & editing.
Funding
The author(s) declare financial support was received for the research, authorship, and/or publication of this article. This work was supported by São Paulo Research Foundation, FAPESP (grant numbers 2018/23105-6 and 2017/02147-0), and the Ministry of Health from Brazil (grant numbers 881187/2018, 879335/2018, 894586/2019 and 894588/2019). HS (grant 314247/2021-1), AS (grant 314731/2021-0), and FL (grant 308843/2019-3) are recipients of National Council for Scientific and Technological Development (CNPq) productivity fellowships. WB is a post-doc fellowship funded by the Ministry of Health from Brazil, grant number 879335/2018.
Conflict of interest
The authors declare that the research was conducted in the absence of any commercial or financial relationships that could be construed as a potential conflict of interest.
Publisher’s note
All claims expressed in this article are solely those of the authors and do not necessarily represent those of their affiliated organizations, or those of the publisher, the editors and the reviewers. Any product that may be evaluated in this article, or claim that may be made by its manufacturer, is not guaranteed or endorsed by the publisher.
References
1. Giráldez, P, Crujeiras, RM, Fernández, JÁ, and Aboal, JR. Establishment of background pollution levels and spatial analysis of moss data on a regional scale. Sci Total Environ. (2022) 839:156182. doi: 10.1016/J.SCITOTENV.2022.156182
2. Koch, HM, and Calafat, AM. Human body burdens of chemicals used in plastic manufacture. Philos Trans R Soc Lond B Biol Sci. (2009) 364:2063–78. doi: 10.1098/RSTB.2008.0208
3. Socianu, S, Bopp, SK, Govarts, E, Gilles, L, Buekers, J, Kolossa-Gehring, M, et al. Chemical mixtures in the EU population: composition and potential risks. Int J Environ Res Public Health. (2022) 19:6121. doi: 10.3390/IJERPH19106121
4. Kaur, H, Bala, M, and Bansal, G. Reproductive drugs and environmental contamination: quantum, impact assessment and control strategies. Environ Sci Pollut Res Int. (2018) 25:25822–39. doi: 10.1007/S11356-018-2754-Z
5. Tolonen, H, Moore, S, Lermen, D, Virgolino, A, Knudsen, LE, Andersson, AM, et al. What is required to combine human biomonitoring and health surveys? Int J Hyg Environ Health. (2022) 242:113964. doi: 10.1016/J.IJHEH.2022.113964
6. Bizjak, T, Capodiferro, M, Deepika, D, Dinçkol, Ö, Dzhedzheia, V, Lopez-Suarez, L, et al. Human biomonitoring data in health risk assessments published in peer-reviewed journals between 2016 and 2021: confronting reality after a preliminary review. Int J Environ Res Public Health. (2022) 19:3362. doi: 10.3390/IJERPH19063362/S1
7. Panis, C, Kawassaki, ACB, Crestani, APJ, Pascotto, CR, Bortoloti, DS, Vicentini, GE, et al. Evidence on human exposure to pesticides and the occurrence of health hazards in the Brazilian population: a systematic review. Front Public Health. (2022) 9:787438. doi: 10.3389/FPUBH.2021.787438
8. Merhi, A, Kordahi, R, and Hassan, HF. A review on the pesticides in coffee: usage, health effects, detection, and mitigation. Front Public Health. (2022) 10:1004570. doi: 10.3389/FPUBH.2022.1004570
9. Cooper, J, and Dobson, H. The benefits of pesticides to mankind and the environment. Crop Prot. (2007) 26:1337–48. doi: 10.1016/J.CROPRO.2007.03.022
10. Xiao, J, Dong, X, Zhang, X, Ye, F, Cheng, J, Dan, G, et al. Pesticides exposure and dopaminergic neurodegeneration. Expo Health. (2021) 13:295–306. doi: 10.1007/S12403-021-00384-X
11. Birolli, WG, da Silva, BF, and Rodrigues, FE. Biodegradation of the pyrethroid cypermethrin by bacterial consortia collected from orange crops. Environ Res. (2022) 215:114388. doi: 10.1016/J.ENVRES.2022.114388
12. Mostafalou, S, and Abdollahi, M. Pesticides: an update of human exposure and toxicity. Arch Toxicol. (2017) 91:549–99. doi: 10.1007/S00204-016-1849-X
13. Ueyama, J, Saito, I, and Kamijima, M. Analysis and evaluation of pyrethroid exposure in human population based on biological monitoring of urinary pyrethroid metabolites. J Pestic Sci. (2010) 35:87–98. doi: 10.1584/JPESTICS.R10-01
14. Rani, L, Thapa, K, Kanojia, N, Sharma, N, Singh, S, Grewal, AS, et al. An extensive review on the consequences of chemical pesticides on human health and environment. J Clean Prod. (2021) 283:124657. doi: 10.1016/J.JCLEPRO.2020.124657
15. Kim, KH, Kabir, E, and Jahan, SA. Exposure to pesticides and the associated human health effects. Sci Total Environ. (2017) 575:525–35. doi: 10.1016/J.SCITOTENV.2016.09.009
16. Zúñiga-Venegas, LA, Hyland, C, Muñoz-Quezada, MT, Quirós-Alcalá, L, Butinof, M, Buralli, R, et al. Health effects of pesticide exposure in Latin American and the Caribbean populations: a scoping review. Environ Health Perspect. (2022) 130:96002. doi: 10.1289/EHP9934
17. Longo, V, Forleo, A, Giampetruzzi, L, Siciliano, P, and Capone, S. Human biomonitoring of environmental and occupational exposures by GC-MS and gas sensor systems: a systematic review. Int J Environ Res Public Health. (2021) 18:10236. doi: 10.3390/IJERPH181910236
18. Soares, S, Rosado, T, Barroso, M, Vieira, DN, and Gallardo, E. Organophosphorus pesticide determination in biological specimens: bioanalytical and toxicological aspects. Int J Legal Med. (2019) 133:1763–84. doi: 10.1007/S00414-019-02119-9
19. Vargas Medina, DA, Sartore, DM, Maciel, EVS, Santos-Neto, ÁJ, and Lanças, FM. Microextraction columns for automated sample preparation. A review focusing on fully miniaturized column switching and bioanalytical applications. Adv Sample Prep. (2022) 3:100031. doi: 10.1016/J.SAMPRE.2022.100031
20. Vicini, JL, Jensen, PK, Young, BM, and Swarthout, JT. Residues of glyphosate in food and dietary exposure. Compr Rev Food Sci Food Saf. (2021) 20:5226–57. doi: 10.1111/1541-4337.12822
21. Nanita, SC, and Kaldon, LG. Emerging flow injection mass spectrometry methods for high-throughput quantitative analysis. Anal Bioanal Chem. (2016) 408:23–33. doi: 10.1007/S00216-015-9193-1
22. Escudero, LB, Castro Grijalba, A, Martinis, EM, and Wuilloud, RG. Bioanalytical separation and preconcentration using ionic liquids. Anal Bioanal Chem. (2013) 405:7597–613. doi: 10.1007/S00216-013-6950-X
23. Sabbioni, G . Hemoglobin adducts and urinary metabolites of Arylamines and Nitroarenes. Chem Res Toxicol. (2017) 30:1733–66. doi: 10.1021/ACS.CHEMRESTOX.7B00111
24. Huhn, S, Escher, BI, Krauss, M, Scholz, S, Hackermüller, J, and Altenburger, R. Unravelling the chemical exposome in cohort studies: routes explored and steps to become comprehensive. Environ Sci Eur. (2021) 33:1–20. doi: 10.1186/S12302-020-00444-0
25. Panuwet, P, Hunter, RE, D’Souza, PE, Chen, X, Radford, SA, Cohen, JR, et al. Biological matrix effects in quantitative tandem mass spectrometry-based analytical methods: advancing biomonitoring. Crit Rev Anal Chem. (2016) 46:93–105. doi: 10.1080/10408347.2014.980775
26. Tang, W, Wang, D, Wang, J, Wu, Z, Li, L, Huang, M, et al. Pyrethroid pesticide residues in the global environment: an overview. Chemosphere. (2018) 191:990–1007. doi: 10.1016/J.CHEMOSPHERE.2017.10.115
27. Schlittenbauer, L, Seiwert, B, and Reemtsma, T. Matrix effects in human urine analysis using multi-targeted liquid chromatography-tandem mass spectrometry. J Chromatogr A. (2015) 1415:91–9. doi: 10.1016/J.CHROMA.2015.08.069
28. Soares, PRS, Birolli, WG, Ferreira, IM, and Porto, ALM. Biodegradation pathway of the organophosphate pesticides chlorpyrifos, methyl parathion and profenofos by the marine-derived fungus aspergillus sydowii CBMAI 935 and its potential for methylation reactions of phenolic compounds. Mar Pollut Bull. (2021) 166:112185. doi: 10.1016/J.MARPOLBUL.2021.112185
29. Kumar, V, Upadhyay, N, Kumar, V, and Sharma, S. A review on sample preparation and chromatographic determination of acephate and methamidophos in different samples. Arab J Chem. (2015) 8:624–31. doi: 10.1016/J.ARABJC.2014.12.007
30. Jain, R, and Singh, R. Applications of dispersive liquid–liquid micro-extraction in forensic toxicology. TrAC Trends Anal Chem. (2016) 75:227–37. doi: 10.1016/J.TRAC.2015.07.007
31. Li, G, and Row, KH. Utilization of deep eutectic solvents in dispersive liquid-liquid micro-extraction. TrAC Trends Anal Chem. (2019) 120:115651. doi: 10.1016/J.TRAC.2019.115651
32. Lee, J, Kim, H, Kang, S, Baik, N, Hwang, I, and Chung, DS. Applications of deep eutectic solvents to quantitative analyses of pharmaceuticals and pesticides in various matrices: a brief review. Arch Pharm Res. (2020) 43:900–19. doi: 10.1007/S12272-020-01266-7
33. Płotka-Wasylka, J, Szczepańska, N, de la Guardia, M, and Namieśnik, J. Modern trends in solid phase extraction: new sorbent media. TrAC Trends Anal Chem. (2016) 77:23–43. doi: 10.1016/J.TRAC.2015.10.010
34. Płotka-Wasylka, J, Szczepańska, N, de la Guardia, M, and Namieśnik, J. Miniaturized solid-phase extraction techniques. TrAC Trends Anal Chem. (2015) 73:19–38. doi: 10.1016/J.TRAC.2015.04.026
35. Morisue Sartore, D, Costa, JL, Lanças, FM, and Santos-Neto, ÁJ. Packed in-tube SPME-LC-MS/MS for fast and straightforward analysis of cannabinoids and metabolites in human urine. Electrophoresis. (2022) 43:1555–66. doi: 10.1002/ELPS.202100389
36. Santana-Mayor, Á, Socas-Rodríguez, B, Herrera-Herrera, AV, and Rodríguez-Delgado, MÁ. Current trends in QuEChERS method. A versatile procedure for food, environmental and biological analysis. TrAC Trends Anal Chem. (2019) 116:214–35. doi: 10.1016/J.TRAC.2019.04.018
37. Rykowska, I, Ziemblińska, J, and Nowak, I. Modern approaches in dispersive liquid-liquid microextraction (DLLME) based on ionic liquids: a review. J Mol Liq. (2018) 259:319–39. doi: 10.1016/J.MOLLIQ.2018.03.043
38. Han, D, and Row, KH. Trends in liquid-phase microextraction, and its application to environmental and biological samples. Microchim Acta. (2012) 176:1–22. doi: 10.1007/S00604-011-0678-0/TABLES/5
39. Vargas Medina, DA, Maciel, EVS, and Lanças, FM. Modern automated sample preparation for the determination of organic compounds: a review on robotic and on-flow systems. TrAC Trends Anal Chem. (2023) 166:117171. doi: 10.1016/J.TRAC.2023.117171
40. Kruve, A, Rebane, R, Kipper, K, Oldekop, ML, Evard, H, Herodes, K, et al. Tutorial review on validation of liquid chromatography-mass spectrometry methods: part I. Anal Chim Acta. (2015) 870:29–44. doi: 10.1016/j.aca.2015.02.017
41. Kruve, A, Rebane, R, Kipper, K, Oldekop, ML, Evard, H, Herodes, K, et al. Tutorial review on validation of liquid chromatography-mass spectrometry methods: part II. Anal Chim Acta. (2015) 870:8–28. doi: 10.1016/J.ACA.2015.02.016
42. Ueyama, J, Kamijima, M, Kondo, T, Takagi, K, Shibata, E, Hasegawa, T, et al. Revised method for routine determination of urinary dialkyl phosphates using gas chromatography–mass spectrometry. J Chromatogr B. (2010) 878:1257–63. doi: 10.1016/J.JCHROMB.2010.02.005
43. Prapamontol, T, Sutan, K, Laoyang, S, Hongsibsong, S, Lee, G, Yano, Y, et al. Cross validation of gas chromatography-flame photometric detection and gas chromatography–mass spectrometry methods for measuring dialkylphosphate metabolites of organophosphate pesticides in human urine. Int J Hyg Environ Health. (2014) 217:554–66. doi: 10.1016/J.IJHEH.2013.10.005
44. Santos, MG, Vitor, RV, Andrade, FL, Martins, I, and Figueiredo, EC. Molecularly imprinted solid phase extraction of urinary diethyl thiophosphate and diethyl dithiophosphate and their analysis by gas chromatography–mass spectrometry. J Chromatogr B. (2012) 909:70–6. doi: 10.1016/J.JCHROMB.2012.10.015
45. Pelit, FO, and Yengin, Ç. Application of solidified floating organic drop microextraction method for biomonitoring of chlorpyrifos and its oxon metabolite in urine samples. J Chromatogr B. (2014) 949–950:109–14. doi: 10.1016/J.JCHROMB.2014.01.004
46. Okamura, A, Saito, I, Ueyama, J, Ito, Y, Nakajima, T, and Kamijima, M. New analytical method for sensitive quantification of urinary 3-methyl-4-nitrophenol to assess fenitrothion exposure in general population and occupational sprayers. Toxicol Lett. (2012) 210:220–4. doi: 10.1016/J.TOXLET.2011.10.008
47. Yoshida, T, and Yoshida, J. Simultaneous analytical method for urinary metabolites of organophosphorus compounds and moth repellents in general population. J Chromatogr B. (2012) 880:66–73. doi: 10.1016/J.JCHROMB.2011.11.018
48. Saito, T, Miura, N, Namera, A, Oikawa, H, Miyazaki, S, Nakamoto, A, et al. Mixed-mode C-C 18 monolithic spin-column extraction and GC-MS for simultaneous assay of organophosphorus compounds, glyphosate, and glufosinate in human serum and urine. Forensic Toxicol. (2012) 30:1–10. doi: 10.1007/S11419-011-0120-7/FIGURES/5
49. Saito, T, Aoki, H, Namera, A, Oikawa, H, Miyazaki, S, Nakamoto, A, et al. Mix-mode TiO-C18 monolith spin column extraction and GC-MS for the simultaneous assay of organophosphorus compounds and glufosinate, and glyphosate in human serum and urine. Anal Sci. (2011) 27:999–1005. doi: 10.2116/ANALSCI.27.999
50. Saito, T, Fukushima, T, Yui, Y, Miyazaki, S, Nakamoto, A, Namera, A, et al. Monolithic spin column extraction and GC-MS for the simultaneous assay of diquat, paraquat, and fenitrothion in human serum and urine. Anal Bioanal Chem. (2011) 400:25–31. doi: 10.1007/S00216-010-4633-4/TABLES/2
51. Niu, Z, Yu, C, He, X, Zhang, J, and Wen, Y. Salting-out assisted liquid–liquid extraction combined with gas chromatography-mass spectrometry for the determination of pyrethroid insecticides in high salinity and biological samples. J Pharm Biomed Anal. (2017) 143:222–7. doi: 10.1016/J.JPBA.2017.05.046
52. Zhu, M, Niu, Z, Zhang, W, Zhang, J, and Wen, Y. The combination of two microextraction methods coupled with gas chromatography for the determination of pyrethroid insecticides in multimedia environmental samples: air, water, soil, urine and blood. Water Environ J. (2020) 34:503–15. doi: 10.1111/WEJ.12553
53. Saito, S, Ueyama, J, Kondo, T, Saito, I, Shibata, E, Gotoh, M, et al. A non-invasive biomonitoring method for assessing levels of urinary pyrethroid metabolites in diapered children by gas chromatography-mass spectrometry. J Expo Sci Environ Epidemiol. (2014) 24:200–7. doi: 10.1038/JES.2013.31
54. Tao, L, Chen, M, Collins, E, and Lu, C. Simultaneous quantitation of seven pyrethroid metabolites in human urine by capillary gas chromatography–mass spectrometry. J Sep Sci. (2013) 36:773–80. doi: 10.1002/JSSC.201200655
55. Machado, SC, Souza, BM, de Aguiar Marciano, LP, Souza Pereira, AF, de Carvalho, DT, and Martins, I. A sensitive and accurate vortex-assisted liquid-liquid microextraction-gas chromatography-mass spectrometry method for urinary triazoles. J Chromatogr A. (2019) 1586:9–17. doi: 10.1016/J.CHROMA.2018.11.082
56. Sajid, M, Basheer, C, Daud, M, and Alsharaa, A. Evaluation of layered double hydroxide/graphene hybrid as a sorbent in membrane-protected stir-bar supported micro-solid-phase extraction for determination of organochlorine pesticides in urine samples. J Chromatogr A. (2017) 1489:1–8. doi: 10.1016/J.CHROMA.2017.01.089
57. Jouyban, A, Farajzadeh, MA, and Afshar Mogaddam, MR. Dispersive liquid–liquid microextraction based on solidification of deep eutectic solvent droplets for analysis of pesticides in farmer urine and plasma by gas chromatography–mass spectrometry. J Chromatogr B. (2019) 1124:114–21. doi: 10.1016/J.JCHROMB.2019.06.004
58. Iqbal, S, Iqbal, MM, Javed, M, Bahadur, A, Yasien, S, Najam-ud-din, HA, et al. Modified QuEChERS extraction method followed by simultaneous quantitation of nine multi-class pesticides in human blood and urine by using GC-MS. J Chromatogr B. (2020) 1152:122227. doi: 10.1016/J.JCHROMB.2020.122227
59. Oenning, AL, Merib, J, and Carasek, E. An effective and high-throughput analytical methodology for pesticide screening in human urine by disposable pipette extraction and gas chromatography – mass spectrometry. J Chromatogr B. (2018) 1092:459–65. doi: 10.1016/J.JCHROMB.2018.06.047
60. Hung, CC, Sari Simaremare, SR, Hsieh, CJ, and Yiin, LM. Simultaneous determination of Pyrethroid, organophosphate and carbamate metabolites in human urine by gas chromatography–mass spectrometry (GCMS). Appl Sci. (2019) 9:879. doi: 10.3390/APP9050879
61. Snow, NH . Flying high with sensitivity and selectivity: Gc-ms to gc-ms/ms. LCGC N Am. (2021) 39:61–7. doi: 10.56530/LCGC.NA.YN3065Q6
62. Schettgen, T, Dewes, P, and Kraus, T. A method for the simultaneous quantification of eight metabolites of synthetic pyrethroids in urine of the general population using gas chromatography-tandem mass spectrometry. Anal Bioanal Chem. (2016) 408:5467–78. doi: 10.1007/S00216-016-9645-2/TABLES/5
63. Ueda, Y, Oda, M, Saito, I, Hamada, R, Kondo, T, Kamijima, M, et al. A sensitive and efficient procedure for the high-throughput determination of nine urinary metabolites of pyrethroids by GC-MS/MS and its application in a sample of Japanese children. Anal Bioanal Chem. (2018) 410:6207–17. doi: 10.1007/S00216-018-1229-X
64. Charisiadis, P, Delplancke, T, and Makris, KC. Cohort-friendly protocol for the determination of two urinary biomarkers of exposure to pyrethroids and neonicotinoids using gas chromatography-triple quadrupole mass spectrometry. Anal Bioanal Chem. (2019) 411:5013–21. doi: 10.1007/S00216-019-01925-9/TABLES/4
65. Guo, XY, Sun, LS, Huang, MY, Xu, WL, Wang, Y, and Wang, N. Simultaneous determination of eight metabolites of organophosphate and pyrethroid pesticides in urine. J Environ Sci Health B. (2016) 52:1–9. doi: 10.1080/03601234.2016.1224695
66. Schmidt, L, Müller, J, and Göen, T. Simultaneous monitoring of seven phenolic metabolites of endocrine disrupting compounds (EDC) in human urine using gas chromatography with tandem mass spectrometry. Anal Bioanal Chem. (2013) 405:2019–29. doi: 10.1007/S00216-012-6618-Y
67. Schmidt, L, and Göen, T. Simultaneous determination of the full chlorophenol spectrum in human urine using gas chromatography with tandem mass spectrometry. Anal Chim Acta. (2017) 965:123–30. doi: 10.1016/J.ACA.2017.02.016
68. Denghel, H, and Göen, T. Simultaneous assessment of phenolic metabolites in human urine for a specific biomonitoring of exposure to organophosphate and carbamate pesticides. Toxicol Lett. (2018) 298:33–41. doi: 10.1016/J.TOXLET.2018.07.048
69. Lu, D, Feng, C, Wang, D, Lin, Y, Ip, HSS, She, J, et al. Analysis of twenty phenolic compounds in human urine: hydrochloric acid hydrolysis, solid-phase extraction based on K2CO3-treated silica, and gas chromatography tandem mass spectrometry. Anal Bioanal Chem. (2015) 407:4131–41. doi: 10.1007/S00216-015-8598-1/TABLES/3
70. Soulard, P, Dereumeaux, C, and Mercier, F. Determination of biomarkers of exposure to boscalid, captan, folpel, mancozeb and tebuconazole in urine and hair samples. MethodsX. (2022) 9:101671. doi: 10.1016/J.MEX.2022.101671
71. Hardy, EM, Duca, RC, Salquebre, G, and Appenzeller, BMR. Multi-residue analysis of organic pollutants in hair and urine for matrices comparison. Forensic Sci Int. (2015) 249:6–19. doi: 10.1016/J.FORSCIINT.2014.12.003
72. Cazorla-Reyes, R, Fernández-Moreno, JL, Romero-González, R, Frenich, AG, and Vidal, JLM. Single solid phase extraction method for the simultaneous analysis of polar and non-polar pesticides in urine samples by gas chromatography and ultra high pressure liquid chromatography coupled to tandem mass spectrometry. Talanta. (2011) 85:183–96. doi: 10.1016/J.TALANTA.2011.03.048
74. Marquet, P . LC-MS vs. GC-MS, online extraction systems, advantages of technology for drug screening assays. Methods Mol Biol. (2012) 902:15–27. doi: 10.1007/978-1-61779-934-1_2
75. Dorgerloh, U, Hofmann, A, Riedel, J, and Becker, R. Comparison of gas- and liquid chromatography-mass spectrometry for trace analysis of anilines in groundwater. Int J Environ Anal Chem. (2023) 103:8465–77. doi: 10.1080/03067319.2021.1987423
76. He, P, and Aga, DS. Comparison of GC-MS/MS and LC-MS/MS for the analysis of hormones and pesticides in surface waters: advantages and pitfalls. Anal Methods. (2019) 11:1436–48. doi: 10.1039/C8AY02774A
77. Brinco, J, Guedes, P, Gomes da Silva, M, Mateus, EP, and Ribeiro, AB. Analysis of pesticide residues in soil: a review and comparison of methodologies. Microchem J. (2023) 195:109465. doi: 10.1016/J.MICROC.2023.109465
78. Narenderan, ST, Meyyanathan, SN, and Babu, B. Review of pesticide residue analysis in fruits and vegetables. Pre-treatment, extraction and detection techniques. Food Res Int. (2020) 133:109141. doi: 10.1016/J.FOODRES.2020.109141
79. Guo, H, Li, L, and Gao, L. Paraquat and Diquat: recent updates on their pretreatment and analysis methods since 2010 in biological samples. Molecules. (2023) 28:684. doi: 10.3390/MOLECULES28020684
80. Kocadal, K, Alkas, FB, Battal, D, and Saygi, S. A review on advances and perspectives of glyphosate determination: challenges and opportunities. Arch Environ Prot. (2023) 48:89–98. doi: 10.24425/AEP.2022.142693
81. Ohara, T, Yoshimoto, T, Natori, Y, and Ishii, A. A simple method for the determination of glyphosate, glufosinate and their metabolites in biological specimen by liquid chromatography/tandem mass spectrometry: an application for forensic toxicology. Nagoya J Med Sci. (2021) 83:567. doi: 10.18999/NAGJMS.83.3.567
82. Martin-Reina, J, Dahiri, B, Carbonero-Aguilar, P, Soria-Dıaz, ME, González, AG, Bautista, J, et al. Validation of a simple method for the determination of glyphosate and aminomethylphosphonic acid in human urine by UPLC-MS/MS. Microchem J. (2021) 170:106760. doi: 10.1016/J.MICROC.2021.106760
83. Chen, D, Miao, H, Zhao, Y, and Wu, Y. A simple liquid chromatography-high resolution mass spectrometry method for the determination of glyphosate and aminomethylphosphonic acid in human urine using cold-induced phase separation and hydrophilic pipette tip solid-phase extraction. J Chromatogr A. (2019) 1587:73–8. doi: 10.1016/J.CHROMA.2018.11.030
84. Nørskov, NP, Jensen, SK, and Sørensen, MT. Robust and highly sensitive micro liquid chromatography–tandem mass spectrometry method for analyses of polar pesticides (glyphosate, aminomethylphosfonic acid, N-acetyl glyphosate and N-acetyl aminomethylphosfonic acid) in multiple biological matrices. J Chromatogr A. (2019) 1605:360343. doi: 10.1016/J.CHROMA.2019.06.064
85. Tsao, YC, Lai, YC, Liu, HC, Liu, RH, and Lin, DL. Simultaneous determination and quantitation of Paraquat, Diquat, Glufosinate and glyphosate in postmortem blood and urine by LC-MS-MS. J Anal Toxicol. (2016) 40:427–36. doi: 10.1093/JAT/BKW042
86. Lindh, CH, Littorin, M, Johannesson, G, and Jönsson, BAG. Analysis of chlormequat in human urine as a biomarker of exposure using liquid chromatography triple quadrupole mass spectrometry. J Chromatogr B. (2011) 879:1551–6. doi: 10.1016/J.JCHROMB.2011.03.046
87. Jones, K, Patel, K, Cocker, J, Bevan, R, and Levy, L. Determination of ethylenethiourea in urine by liquid chromatography–atmospheric pressure chemical ionisation–mass spectrometry for monitoring background levels in the general population. J Chromatogr B. (2010) 878:2563–6. doi: 10.1016/J.JCHROMB.2009.10.028
88. Ekman, E, Maxe, M, Littorin, M, Jönsson, BAG, and Lindh, CH. High-throughput method for the analysis of ethylenethiourea with direct injection of hydrolysed urine using online on-column extraction liquid chromatography and triple quadrupole mass spectrometry. J Chromatogr B. (2013) 934:53–9. doi: 10.1016/J.JCHROMB.2013.06.035
89. Ekman, E, Faniband, MH, Littorin, M, Maxe, M, Jönsson, BAG, and Lindh, CH. Determination of 5-hydroxythiabendazole in human urine as a biomarker of exposure to thiabendazole using LC/MS/MS. J Chromatogr B. (2014) 973:61–7. doi: 10.1016/J.JCHROMB.2014.10.003
90. Berthet, A, Bouchard, M, Schüpfer, P, Vernez, D, Danuser, B, and Huynh, CK. Liquid chromatography-tandem mass spectrometry (LC/APCI-MS/MS) methods for the quantification of captan and folpet phthalimide metabolites in human plasma and urine. Anal Bioanal Chem. (2011) 399:2243–55. doi: 10.1007/S00216-010-4601-Z/TABLES/4
91. Panuwet, P, Restrepo, PA, Magsumbol, M, Jung, KY, Montesano, MA, Needham, LL, et al. An improved high-performance liquid chromatography–tandem mass spectrometric method to measure atrazine and its metabolites in human urine. J Chromatogr B. (2010) 878:957–62. doi: 10.1016/J.JCHROMB.2010.02.025
92. Ueyama, J, Saito, I, Takaishi, A, Nomura, H, Inoue, M, Osaka, A, et al. A revised method for determination of dialkylphosphate levels in human urine by solid-phase extraction and liquid chromatography with tandem mass spectrometry: application to human urine samples from Japanese children. Environ Health Prev Med. (2014) 19:405. doi: 10.1007/S12199-014-0407-5
93. Sinha, SN, Venkat Reddy, B, Vasudev, K, Vishnu Vardhana Rao, M, Noor Ahmed, M, Ashu, S, et al. Analysis of dialkyl urine metabolites of organophosphate pesticides by a liquid chromatography mass spectrometry technique. Anal Methods. (2014) 6:1825–34. doi: 10.1039/C3AY41958D
94. Odetokun, MS, Montesano, MA, Weerasekera, G, Whitehead, RD, Needham, LL, and Barr, DB. Quantification of dialkylphosphate metabolites of organophosphorus insecticides in human urine using 96-well plate sample preparation and high-performance liquid chromatography–electrospray ionization-tandem mass spectrometry. J Chromatogr B. (2010) 878:2567–74. doi: 10.1016/J.JCHROMB.2010.04.027
95. Fernández, SF, Pastor, A, Yusà, V, Montesinos, L, and Pardo, O. Development of a novel methodology for determination of dialkyl phosphates in human urine using liquid chromatography-tandem mass spectrometry. J Chromatogr B. (2019) 1130–1131:121810. doi: 10.1016/J.JCHROMB.2019.121810
96. Pasupuleti, RR, Tsai, PC, Lin, PID, Wu, MT, and Ponnusamy, VK. Rapid and sensitive analytical procedure for biomonitoring of organophosphate pesticide metabolites in human urine samples using a vortex-assisted salt-induced liquid–liquid microextraction technique coupled with ultra-high-performance liquid chromatography/tandem mass spectrometry. Rapid Commun Mass Spectrom. (2020) 34:e8565. doi: 10.1002/RCM.8565
97. Cequier, E, Sakhi, AK, Haug, LS, and Thomsen, C. Development of an ion-pair liquid chromatography–high resolution mass spectrometry method for determination of organophosphate pesticide metabolites in large-scale biomonitoring studies. J Chromatogr A. (2016) 1454:32–41. doi: 10.1016/J.CHROMA.2016.05.067
98. Schütze, A, Morales-Agudelo, P, Vidal, M, Calafat, AM, and Ospina, M. Quantification of glyphosate and other organophosphorus compounds in human urine via ion chromatography isotope dilution tandem mass spectrometry. Chemosphere. (2021):274. doi: 10.1016/J.CHEMOSPHERE.2020.129427
99. Reemtsma, T, Lingott, J, and Roegler, S. Determination of 14 monoalkyl phosphates, dialkyl phosphates and dialkyl thiophosphates by LC-MS/MS in human urinary samples. Sci Total Environ. (2011) 409:1990–3. doi: 10.1016/J.SCITOTENV.2011.01.032
100. Jayatilaka, NK, Restrepo, P, Davis, Z, Vidal, M, Calafat, AM, and Ospina, M. Quantification of 16 urinary biomarkers of exposure to flame retardants, plasticizers, and organophosphate insecticides for biomonitoring studies. Chemosphere. (2019) 235:481–91. doi: 10.1016/J.CHEMOSPHERE.2019.06.181
101. Usui, K, Hayashizaki, Y, Minagawa, T, Hashiyada, M, Nakano, A, and Funayama, M. Rapid determination of disulfoton and its oxidative metabolites in human whole blood and urine using QuEChERS extraction and liquid chromatography–tandem mass spectrometry. Legal Med. (2012) 14:309–16. doi: 10.1016/J.LEGALMED.2012.06.005
102. Jayatilaka, NK, Angela Montesano, M, Whitehead, RD, Schloth, SJ, Needham, LL, and Barr, DB. High-throughput sample preparation for the quantitation of acephate, methamidophos, omethoate, dimethoate, ethylenethiourea, and propylenethiourea in human urine using 96-well-plate automated extraction and high-performance liquid chromatography-tandem mass spectrometry. Arch Environ Contam Toxicol. (2011) 61:59–67. doi: 10.1007/S00244-010-9593-3/TABLES/3
103. Gao, X, Tan, Y, and Guo, H. Simultaneous determination of amitraz, chlordimeform, formetanate and their main metabolites in human urine by high performance liquid chromatography–tandem mass spectrometry. J Chromatogr B. (2017) 1052:27–33. doi: 10.1016/J.JCHROMB.2017.03.004
104. Zhang, Q, Wang, X, Li, Z, Jin, H, Lu, Z, Yu, C, et al. Simultaneous determination of nine neonicotinoids in human urine using isotope-dilution ultra-performance liquid chromatography–tandem mass spectrometry. Environ Pollut. (2018) 240:647–52. doi: 10.1016/J.ENVPOL.2018.04.144
105. Ueyama, J, Aoi, A, Ueda, Y, Oya, N, Sugiura, Y, Ito, Y, et al. Biomonitoring method for neonicotinoid insecticides in urine of non-toilet-trained children using LC-MS/MS. Food Addit Contam Part A Chem Anal Control Expo Risk Assess. (2019) 37:304–15. doi: 10.1080/19440049.2019.1696020
106. Baker, SE, Serafim, AB, Morales-Agudelo, P, Vidal, M, Calafat, AM, and Ospina, M. Quantification of DEET and neonicotinoid pesticide biomarkers in human urine by online solid-phase extraction high-performance liquid chromatography-tandem mass spectrometry. Anal Bioanal Chem. (2019) 411:669–78. doi: 10.1007/S00216-018-1481-0
107. Honda, M, Robinson, M, and Kannan, K. A simple method for the analysis of neonicotinoids and their metabolites in human urine. Environ Chem. (2019) 16:171–8. doi: 10.1071/EN18240
108. Song, S, He, Y, Zhang, B, Gui, M, Ouyang, J, and Zhang, T. A novel extraction method for simultaneous determination of neonicotinoid insecticides and their metabolites in human urine. Anal Methods. (2019) 11:2571–8. doi: 10.1039/C9AY00288J
109. López-García, M, Romero-González, R, Lacasaña, M, and Garrido, FA. Semiautomated determination of neonicotinoids and characteristic metabolite in urine samples using TurboFlow™ coupled to ultra high performance liquid chromatography coupled to Orbitrap analyzer. J Pharm Biomed Anal. (2017) 146:378–86. doi: 10.1016/J.JPBA.2017.08.026
110. López-García, M, Romero-González, R, and Garrido, FA. Monitoring of organophosphate and pyrethroid metabolites in human urine samples by an automated method (TurboFlow™) coupled to ultra-high performance liquid chromatography-Orbitrap mass spectrometry. J Pharm Biomed Anal. (2019) 173:31–9. doi: 10.1016/J.JPBA.2019.05.018
111. Behniwal, PK, and She, J. Development of HPLC-MS/MS method for the simultaneous determination of metabolites of organophosphate pesticides, synthetic pyrethroids, herbicides and DEET in human urine. Int J Environ Anal Chem. (2017) 97:548–62. doi: 10.1080/03067319.2017.1325881
112. Davis, MD, Wade, EL, Restrepo, PR, Roman-Esteva, W, Bravo, R, Kuklenyik, P, et al. Semi-automated solid phase extraction method for the mass spectrometric quantification of 12 specific metabolites of organophosphorus pesticides, synthetic pyrethroids, and select herbicides in human urine. J Chromatogr B. (2013) 929:18–26. doi: 10.1016/J.JCHROMB.2013.04.005
113. Gao, B, Poma, G, Malarvannan, G, Dumitrascu, C, Bastiaensen, M, Wang, M, et al. Development of an analytical method based on solid-phase extraction and LC-MS/MS for the monitoring of current-use pesticides and their metabolites in human urine. J Environ Sci. (2022) 111:153–63. doi: 10.1016/J.JES.2021.03.029
114. Huang, Z, Li, H, Xiong, J, and You, J. Target and suspect screening of urinary biomarkers for current-use pesticides: application of a simple extraction method. Environ Toxicol Chem. (2022) 41:73–80. doi: 10.1002/ETC.5234
115. Roca, M, Leon, N, Pastor, A, and Yusà, V. Comprehensive analytical strategy for biomonitoring of pesticides in urine by liquid chromatography–orbitrap high resolution mass spectrometry. J Chromatogr A. (2014) 1374:66–76. doi: 10.1016/J.CHROMA.2014.11.010
116. Sweeney, C, Park, Y, and Kim, JS. Comparison of sample preparation approaches and validation of an extraction method for nitrosatable pesticides and metabolites in human serum and urine analyzed by liquid chromatography – orbital ion trap mass spectrometry. J Chromatogr A. (2019) 1603:83–91. doi: 10.1016/J.CHROMA.2019.06.065
117. Mouskeftara, T, Virgiliou, C, Iakovakis, A, Raikos, N, and Gika, HG. Liquid chromatography tandem mass spectrometry for the determination of nine insecticides and fungicides in human postmortem blood and urine. J Chromatogr B. (2021) 1179:122824. doi: 10.1016/J.JCHROMB.2021.122824
118. Gallo, V, Tomai, P, Gherardi, M, Fanali, C, De Gara, L, D’Orazio, G, et al. Dispersive liquid-liquid microextraction using a low transition temperature mixture and liquid chromatography-mass spectrometry analysis of pesticides in urine samples. J Chromatogr A. (2021) 1642:462036. doi: 10.1016/J.CHROMA.2021.462036
119. Shin, Y, Lee, JJ, Park, E, Lee, JJ, Lee, HS, and Kim, J-HH. A quantitative tandem mass spectrometry and scaled-down QuEChERS approach for simultaneous analysis of pesticide multiresidues in human urine. Molecules. (2019) 24:1330. doi: 10.3390/MOLECULES24071330
120. López, A, Dualde, P, Yusà, V, and Coscollà, C. Retrospective analysis of pesticide metabolites in urine using liquid chromatography coupled to high-resolution mass spectrometry. Talanta. (2016) 160:547–55. doi: 10.1016/J.TALANTA.2016.07.065
121. Mercier, F, Rouillon-Bartoletti, C, Dimeglio, A, Gilles, E, Lecorgne, A, Bonvallot, N, et al. Simultaneous determination of selected pesticides and/or their metabolites in urine by off-line solid phase extraction and ultra high performance liquid chromatography/hybrid quadrupole time-of-flight mass spectrometry. Microchem J. (2022) 180:107539. doi: 10.1016/J.MICROC.2022.107539
122. Cortéjade, A, Kiss, A, Cren, C, Vulliet, E, and Buleté, A. Development of an analytical method for the targeted screening and multi-residue quantification of environmental contaminants in urine by liquid chromatography coupled to high resolution mass spectrometry for evaluation of human exposures. Talanta. (2016) 146:694–706. doi: 10.1016/J.TALANTA.2015.06.038
123. Franco de Oliveira, SCWSE, Zucoloto, AD, de Oliveira, CDR, Hernandez, EMM, Fruchtengarten, LVG, de Oliveira, TF, et al. A fast and simple approach for the quantification of 40 illicit drugs, medicines, and pesticides in blood and urine samples by UHPLC-MS/MS. J Mass Spectrom. (2019) 54:600–11. doi: 10.1002/JMS.4369
124. Jagani, R, Pulivarthi, D, Patel, D, Wright, RJ, Wright, RO, Arora, M, et al. Validated single urinary assay designed for exposomic multi-class biomarkers of common environmental exposures. Anal Bioanal Chem. (2022) 414:5943–66. doi: 10.1007/S00216-022-04159-4
125. Zhu, H, Chinthakindi, S, and Kannan, K. A method for the analysis of 121 multi-class environmental chemicals in urine by high-performance liquid chromatography-tandem mass spectrometry. J Chromatogr A. (2021) 1646:462146. doi: 10.1016/J.CHROMA.2021.462146
126. Plumb, RS, Gethings, LA, Rainville, PD, Isaac, G, Trengove, R, King, AM, et al. Advances in high throughput LC/MS based metabolomics: a review. TrAC Trends Anal Chem. (2023) 160:116954. doi: 10.1016/j.trac.2023.116954
127. Koželj, G, and Prosen, H. Thermal (in)stability of atropine and scopolamine in the GC-MS inlet. Toxics. (2021) 9:156. doi: 10.3390/toxics9070156
128. Thomas, SN, French, D, Jannetto, PJ, Rappold, BA, and Clarke, WA. Liquid chromatography–tandem mass spectrometry for clinical diagnostics. Nat Rev Methods Primers. (2022) 2:1–14. doi: 10.1038/s43586-022-00175-x
129. Buchholz, BA, Ahn, KC, Huang, H, Gee, SJ, Stewart, BJ, Ognibene, TJ, et al. Pharmacokinetics, metabolite measurement, and biomarker identification of dermal exposure to permethrin using accelerator mass spectrometry. Toxicol Sci. (2021) 183:49–59. doi: 10.1093/TOXSCI/KFAB082
130. Fäys, F, Hardy, EM, Palazzi, P, Haan, S, Beausoleil, C, and Appenzeller, BMR. Biomonitoring of fast-elimination endocrine disruptors – results from a 6-month follow up on human volunteers with repeated urine and hair collection. Sci Total Environ. (2021) 778:146330. doi: 10.1016/J.SCITOTENV.2021.146330
131. Bayebila Menanzambi, T, Dufour, P, Pirard, C, Nsangu, J, Mufusama, JP, Mbinze Kindenge, J, et al. Bio-surveillance of environmental pollutants in the population of Kinshasa, Democratic Republic of Congo (DRC): a small pilot study. Arch Public Health. (2021) 79:197. doi: 10.1186/S13690-021-00717-X
132. Connolly, A, Jones, K, Galea, KS, Basinas, I, Kenny, L, McGowan, P, et al. Exposure assessment using human biomonitoring for glyphosate and fluroxypyr users in amenity horticulture. Int J Hyg Environ Health. (2017) 220:1064–73. doi: 10.1016/J.IJHEH.2017.06.008
133. Connolly, A, Leahy, M, Jones, K, Kenny, L, and Coggins, MA. Glyphosate in Irish adults – a pilot study in 2017. Environ Res. (2018) 165:235–6. doi: 10.1016/J.ENVRES.2018.04.025
134. Nova, P, Calheiros, CSC, and Silva, M. Glyphosate in Portuguese adults – a pilot study. Environ Toxicol Pharmacol. (2020) 80:103462. doi: 10.1016/J.ETAP.2020.103462
135. Nimako, C, Ikenaka, Y, Akoto, O, Bortey-Sam, N, Ichise, T, Nakayama, SMM, et al. Human exposures to neonicotinoids in Kumasi, Ghana. Environ Toxicol Chem. (2021) 40:2306–18. doi: 10.1002/ETC.5065
136. Morgan, MK, Sobus, JR, Barr, DB, Croghan, CW, Chen, FL, Walker, R, et al. Temporal variability of pyrethroid metabolite levels in bedtime, morning, and 24-h urine samples for 50 adults in North Carolina. Environ Res. (2016) 144:81–91. doi: 10.1016/J.ENVRES.2015.11.003
137. Morgan, M, Jones, P, Sobus, J, and Barr, DB. Predictors of urinary 3-Phenoxybenzoic acid levels in 50 North Carolina adults. Int J Environ Res Public Health. (2016) 13:1172. doi: 10.3390/IJERPH13111172
138. Rodzaj, W, Wileńska, M, Klimowska, A, Dziewirska, E, Jurewicz, J, Walczak-Jędrzejowska, R, et al. Concentrations of urinary biomarkers and predictors of exposure to pyrethroid insecticides in young, polish, urban-dwelling men. Sci Total Environ. (2021) 773:145666. doi: 10.1016/J.SCITOTENV.2021.145666
139. Morgan, MK . Predictors of urinary levels of 2,4-dichlorophenoxyacetic acid, 3,5,6-trichloro-2-pyridinol, 3-phenoxybenzoic acid, and pentachlorophenol in 121 adults in Ohio. Int J Hyg Environ Health. (2015) 218:479–88. doi: 10.1016/J.IJHEH.2015.03.015
140. Wise, CF, Hammel, SC, Herkert, NJ, Ospina, M, Calafat, AM, Breen, M, et al. Comparative assessment of pesticide exposures in domestic dogs and their owners using silicone passive samplers and biomonitoring. Environ Sci Technol. (2022) 56:1149–61. doi: 10.1021/ACS.EST.1C06819/ASSET/IMAGES/LARGE/ES1C06819_0007.JPEG
141. Heffernan, A, English, K, Toms, L, Calafat, A, Valentin-Blasini, L, Hobson, P, et al. Cross-sectional biomonitoring study of pesticide exposures in Queensland, Australia, using pooled urine samples. Environ Sci Pollut Res. (2016) 23:23436–48. doi: 10.1007/S11356-016-7571-7/FIGURES/1
142. Curl, CL, Beresford, SAA, Fenske, RA, Fitzpatrick, AL, Lu, C, Nettleton, JA, et al. Estimating pesticide exposure from dietary intake and organic food choices: the multi-ethnic study of atherosclerosis (MESA). Environ Health Perspect. (2015) 123:475–83. doi: 10.1289/EHP.1408197
143. Ye, M, Beach, J, Martin, JW, and Senthilselvan, A. Urinary Dialkyl phosphate concentrations and lung function parameters in adolescents and adults: results from the Canadian health measures survey. Environ Health Perspect. (2015) 124:491–7. doi: 10.1289/EHP.1509745
144. Li, AJ, and Kannan, K. Urinary concentrations and profiles of organophosphate and pyrethroid pesticide metabolites and phenoxyacid herbicides in populations in eight countries. Environ Int. (2018) 121:1148–54. doi: 10.1016/J.ENVINT.2018.10.033
145. Bao, W, Liu, B, Simonsen, DW, and Lehmler, HJ. Association between exposure to Pyrethroid insecticides and risk of all-cause and cause-specific mortality in the general US adult population. JAMA Intern Med. (2020) 180:367–74. doi: 10.1001/JAMAINTERNMED.2019.6019
146. Ospina, M, Wong, LY, Baker, SE, Serafim, AB, Morales-Agudelo, P, and Calafat, AM. Exposure to neonicotinoid insecticides in the U.S. general population: data from the 2015-2016 national health and nutrition examination survey. Environ Res. (2019) 176:108555. doi: 10.1016/J.ENVRES.2019.108555
147. Liljedahl, ER, Johanson, G, de Paula, HK, Faniband, M, Assarsson, E, Littorin, M, et al. Filaggrin polymorphisms and the uptake of chemicals through the skin-a human experimental study. Environ Health Perspect. (2021) 129:1–10. doi: 10.1289/EHP7310
148. Smpokou, ET, González-Quiroz, M, Martins, C, Alvito, P, Le Blond, J, Glaser, J, et al. Environmental exposures in young adults with declining kidney function in a population at risk of Mesoamerican nephropathy. Occup Environ Med. (2019) 76:920–6. doi: 10.1136/OEMED-2019-105772
149. Oerlemans, A, Figueiredo, DM, Mol, JGJ, Nijssen, R, Anzion, RBM, van Dael, MFP, et al. Personal exposure assessment of pesticides in residents: the association between hand wipes and urinary biomarkers. Environ Res. (2021) 199:111282. doi: 10.1016/J.ENVRES.2021.111282
150. Mercadante, R, Polledri, E, Rubino, FM, Mandic-Rajcevic, S, Vaiani, A, Colosio, C, et al. Assessment of penconazole exposure in winegrowers using urinary biomarkers. Environ Res. (2019) 168:54–61. doi: 10.1016/J.ENVRES.2018.09.013
151. Mandic-Rajcevic, S, Rubino, FM, Ariano, E, Cottica, D, Neri, S, and Colosio, C. Environmental and biological monitoring for the identification of main exposure determinants in vineyard mancozeb applicators. J Expo Sci Environ Epidemiol. (2018) 28:289–96. doi: 10.1038/jes.2017.14
152. Mandic-Rajcevic, S, Rubino, FM, Ariano, E, Cottica, D, Negri, S, and Colosio, C. Exposure duration and absorbed dose assessment in pesticide-exposed agricultural workers: implications for risk assessment and modeling. Int J Hyg Environ Health. (2019) 222:494–502. doi: 10.1016/J.IJHEH.2019.01.006
153. Mandić-Rajčević, S, Rubino, FM, and Colosio, C. Establishing health-based biological exposure limits for pesticides: a proof of principle study using mancozeb. Regul Toxicol Pharmacol. (2020) 115:104689. doi: 10.1016/J.YRTPH.2020.104689
154. Tao, Y, Phung, D, Dong, F, Xu, J, Liu, X, Wu, X, et al. Urinary monitoring of neonicotinoid imidacloprid exposure to pesticide applicators. Sci Total Environ. (2019) 669:721–8. doi: 10.1016/J.SCITOTENV.2019.03.040
155. Perry, MJ, Mandrioli, D, Belpoggi, F, Manservisi, F, Panzacchi, S, and Irwin, C. Historical evidence of glyphosate exposure from a US agricultural cohort. Environ Health. (2019) 18:42. doi: 10.1186/S12940-019-0474-6
156. Kohsuwan, K, Intayoung, U, Khacha-ananda, S, Sapbamrer, R, Koonrungsesomboon, N, Techatoei, S, et al. Urinary glyphosate kinetics after occupational exposure. Int J Hyg Environ Health. (2022) 245:114021. doi: 10.1016/J.IJHEH.2022.114021
157. Sidthilaw, S, Sapbamrer, R, Pothirat, C, Wunnapuk, K, and Khacha-ananda, S. Effects of exposure to glyphosate on oxidative stress, inflammation, and lung function in maize farmers, northern Thailand. BMC Public Health. (2022) 22:1343. doi: 10.1186/S12889-022-13696-7
158. Paglia, G, Del Greco, FM, Carli, C, Sigurdsson, BB, Smarason, S, Wegher, M, et al. Longitudinal assessment of Chlorpyrifos exposure in farmers and residents of an Italian alpine region. Expo Health. (2021) 13:651–9. doi: 10.1007/S12403-021-00409-5/FIGURES/1
159. Koureas, M, Tsakalof, A, Tzatzarakis, M, Vakonaki, E, Tsatsakis, A, and Hadjichristodoulou, C. Biomonitoring of organophosphate exposure of pesticide sprayers and comparison of exposure levels with other population groups in Thessaly (Greece). Occup Environ Med. (2014) 71:126–33. doi: 10.1136/OEMED-2013-101490
160. Koureas, M, Rachiotis, G, Tsakalof, A, and Hadjichristodoulou, C. Increased frequency of rheumatoid arthritis and allergic rhinitis among pesticide sprayers and associations with pesticide use. Int J Environ Res Public Health. (2017) 14:865. doi: 10.3390/IJERPH14080865
161. Garí, M, González-Quinteiro, Y, Bravo, N, and Grimalt, JO. Analysis of metabolites of organophosphate and pyrethroid pesticides in human urine from urban and agricultural populations (Catalonia and Galicia). Sci Total Environ. (2018) 622–623:526–33. doi: 10.1016/J.SCITOTENV.2017.11.355
162. Chittrakul, J, Sapbamrer, R, and Hongsibsong, S. Exposure to organophosphate insecticides, inappropriate personal protective equipment use, and cognitive performance among pesticide applicators. Front Public Health. (2022) 10:1060284. doi: 10.3389/FPUBH.2022.1060284/FULL
163. Valcke, M, Samuel, O, Bouchard, M, Dumas, P, Belleville, D, and Tremblay, C. Biological monitoring of exposure to organophosphate pesticides in children living in peri-urban areas of the province of Quebec, Canada. Int Arch Occup Environ Health. (2006) 79:568–77. doi: 10.1007/S00420-006-0085-8/FIGURES/1
164. Paredes-Céspedes, DM, Bernal-Hernández, YY, Herrera-Moreno, JF, Rojas-García, AE, Medina-Díaz, IM, González-Arias, CA, et al. Methylation patterns of the CDKN2B and CDKN2A genes in an indigenous population exposed to pesticides. Hum Exp Toxicol. (2022) 41:9603271211063161. doi: 10.1177/09603271211063161
165. Aguilar-Garduño, C, Blanco-Muñoz, J, Roxana Antonio, K, Escamilla-Nuñez, C, Juárez-Pérez, CA, Schilmann, A, et al. Occupational predictors of urinary dialkyl phosphate concentrations in Mexican flower growers. Int J Occup Environ Health. (2017) 23:151–9. doi: 10.1080/10773525.2018.1441676
166. Toumi, K, Joly, L, Vleminckx, C, and Schiffers, B. Biological monitoring of exposure to pesticide residues among Belgian florists. Hum Ecol Risk Assess. (2020) 26:636–53. doi: 10.1080/10807039.2018.1528860
167. Sato, H, Ito, Y, Ueyama, J, Kano, Y, Arakawa, T, Gotoh, M, et al. Effects of Paraoxonase 1 gene polymorphisms on organophosphate insecticide metabolism in Japanese pest control workers. J Occup Health. (2016) 58:56–65. doi: 10.1539/JOH.15-0175-OA
168. Connolly, A, Basinas, I, Jones, K, Galea, KS, Kenny, L, McGowan, P, et al. Characterising glyphosate exposures among amenity horticulturists using multiple spot urine samples. Int J Hyg Environ Health. (2018) 221:1012–22. doi: 10.1016/J.IJHEH.2018.06.007
169. Faniband, M, Ekman, E, Littorin, M, Maxe, M, Larsson, E, and Lindh, CH. Biomarkers of exposure to Pyrimethanil after controlled human experiments. J Anal Toxicol. (2019) 43:277–83. doi: 10.1093/JAT/BKY091
170. Amir, S, Tzatzarakis, M, Mamoulakis, C, Bello, JH, Eqani, SAMAS, Vakonaki, E, et al. Impact of organochlorine pollutants on semen parameters of infertile men in Pakistan. Environ Res. (2021) 195:110832. doi: 10.1016/J.ENVRES.2021.110832
171. Figueroa, ZI, Young, HA, Meeker, JD, Martenies, SE, Barr, DB, Gray, G, et al. Dialkyl phosphate urinary metabolites and chromosomal abnormalities in human sperm. Environ Res. (2015) 143:256–65. doi: 10.1016/J.ENVRES.2015.10.021
172. Li, AJ, Chen, Z, Lin, TC, Buck Louis, GM, and Kannan, K. Association of urinary metabolites of organophosphate and pyrethroid insecticides, and phenoxy herbicides with endometriosis. Environ Int. (2020) 136:105456. doi: 10.1016/J.ENVINT.2019.105456
173. Hu, Y, Ji, L, Zhang, Y, Shi, R, Han, W, Tse, LA, et al. Organophosphate and Pyrethroid pesticide exposures measured before conception and associations with time to pregnancy in Chinese couples enrolled in the Shanghai birth cohort. Environ Health Perspect. (2018) 126:077001. doi: 10.1289/EHP2987
174. Hu, P, Vinturache, A, Li, H, Tian, Y, Yuan, L, Cai, C, et al. Urinary organophosphate metabolite concentrations and pregnancy outcomes among women conceiving through in vitro fertilization in Shanghai, China. Environ Health Perspect. (2020) 128:97007. doi: 10.1289/EHP7076
175. Hioki, K, Ito, Y, Oya, N, Nakayama, SF, Isobe, T, Ebara, T, et al. Intra-individual variations of organophosphate pesticide metabolite concentrations in repeatedly collected urine samples from pregnant women in Japan. Environ Health Prev Med. (2019) 24:7. doi: 10.1186/S12199-019-0761-4
176. Liang, D, Batross, J, Fiedler, N, Prapamontol, T, Suttiwan, P, Panuwet, P, et al. Metabolome-wide association study of the relationship between chlorpyrifos exposure and first trimester serum metabolite levels in pregnant Thai farmworkers. Environ Res. (2022) 215:114319. doi: 10.1016/J.ENVRES.2022.114319
177. Llop, S, Murcia, M, Iñiguez, C, Roca, M, González, L, Yusà, V, et al. Distributions and determinants of urinary biomarkers of organophosphate pesticide exposure in a prospective Spanish birth cohort study. Environ Health. (2017) 16:46. doi: 10.1186/S12940-017-0255-Z
178. Xu, Q, Song, X, Li, Y, Jian, X, Chen, S, Chen, Y, et al. Urinary concentrations and determinants of pyrethroid metabolites in pregnant women from non-rural areas of Yunnan, China. Ann Agric Environ Med. (2021) 28:627–32. doi: 10.26444/AAEM/140619
179. Simaremare, SRS, Hung, CC, Hsieh, CJ, and Yiin, LM. Relationship between organophosphate and Pyrethroid insecticides in blood and their metabolites in urine: a pilot study. Int J Environ Res Public Health. (2019) 17:34. doi: 10.3390/IJERPH17010034
180. De Renzy-Martin, KT, Frederiksen, H, Christensen, JS, Kyhl, HB, Andersson, AM, Husby, S, et al. Current exposure of 200 pregnant Danish women to phthalates, parabens and phenols. Reproduction. (2014) 147:443–53. doi: 10.1530/REP-13-0461
181. Warembourg, C, Basagaña, X, Seminati, C, de Bont, J, Granum, B, Lyon-Caen, S, et al. Exposure to phthalate metabolites, phenols and organophosphate pesticide metabolites and blood pressure during pregnancy. Int J Hyg Environ Health. (2019) 222:446–54. doi: 10.1016/J.IJHEH.2018.12.011
182. Casas, M, Basagaña, X, Sakhi, AK, Haug, LS, Philippat, C, Granum, B, et al. Variability of urinary concentrations of non-persistent chemicals in pregnant women and school-aged children. Environ Int. (2018) 121:561–73. doi: 10.1016/J.ENVINT.2018.09.046
183. Oya, N, Ito, Y, Hioki, K, Asai, Y, Aoi, A, Sugiura, Y, et al. Quantitative analysis of organophosphate insecticide metabolites in urine extracted from disposable diapers of toddlers in Japan. Int J Hyg Environ Health. (2017) 220:209–16. doi: 10.1016/J.IJHEH.2016.10.009
184. Oya, N, Ito, Y, Ebara, T, Kato, S, Hioki, K, Aoi, A, et al. Exposure levels of organophosphate pesticides in Japanese diapered children: contributions of exposure-related behaviors and mothers’ considerations of food selection and preparation. Environ Int. (2020) 134:105294. doi: 10.1016/J.ENVINT.2019.105294
185. Hernández, AF, Lozano-Paniagua, D, González-Alzaga, B, Kavvalakis, MP, Tzatzarakis, MN, López-Flores, I, et al. Biomonitoring of common organophosphate metabolites in hair and urine of children from an agricultural community. Environ Int. (2019) 131:104997. doi: 10.1016/J.ENVINT.2019.104997
186. Kunno, J, Ong-Artborirak, P, Panicharoen, P, Robson, MG, and Siriwong, W. Pyrethroid insecticides in households from urban areas: an association of the 3-PBA metabolite and hand wipes. Ann Glob Health. (2020) 86:1–7. doi: 10.5334/AOGH.2746
187. Kunno, J, Ong-Artborirak, P, Taneepanichskul, N, Robson, MG, and Siriwong, W. Effect of pyrethroid insecticides exposure in relation to pyrethroid metabolite and GABA concentration of young children, Bangkok Thailand. Hum Ecol Risk Assess. (2021) 27:1–14. doi: 10.1080/10807039.2019.1689098
188. Domingues, VF, Nasuti, C, Piangerelli, M, Correia-Sá, L, Ghezzo, A, Marini, M, et al. Pyrethroid pesticide metabolite in urine and microelements in hair of children affected by autism spectrum disorders: a preliminary investigation. Int J Environ Res Public Health. (2016) 13:388. doi: 10.3390/IJERPH13040388
189. Ikenaka, Y, Miyabara, Y, Ichise, T, Nakayama, S, Nimako, C, Ishizuka, M, et al. Exposures of children to neonicotinoids in pine wilt disease control areas. Environ Toxicol Chem. (2019) 38:71–9. doi: 10.1002/ETC.4316
190. Bradman, A, Quirós-Alcalá, L, Castorina, R, Schall, RA, Camacho, J, Holland, NT, et al. Effect of organic diet intervention on pesticide exposures in Young children living in low-income urban and agricultural communities. Environ Health Perspect. (2015) 123:1086–93. doi: 10.1289/EHP.1408660
191. Laubscher, B, Diezi, M, Renella, R, Mitchell, EAD, Aebi, A, Mulot, M, et al. Multiple neonicotinoids in children’s cerebro-spinal fluid, plasma, and urine. Environ Health. (2022) 21:10. doi: 10.1186/S12940-021-00821-Z
192. Lozano-Kasten, F, Sierra-Diaz, E, Chavez, HG, Peregrina Lucano, AA, Cremades, R, and Pinto, ES. Seasonal urinary levels of glyphosate in children from agricultural communities. Dose Response. (2021) 19:15593258211053184. doi: 10.1177/15593258211053184
193. Stajnko, A, Snoj Tratnik, J, Kosjek, T, Mazej, D, Jagodic, M, Eržen, I, et al. Seasonal glyphosate and AMPA levels in urine of children and adolescents living in rural regions of northeastern Slovenia. Environ Int. (2020) 143:105985. doi: 10.1016/J.ENVINT.2020.105985
194. Sierra-Diaz, E, Celis-de la Rosa, A, Lozano-Kasten, F, Trasande, L, Peregrina-Lucano, A, Sandoval-Pinto, E, et al. Urinary pesticide levels in children and adolescents residing in two agricultural communities in Mexico. Int J Environ Res Public Health. (2019) 16:562. doi: 10.3390/IJERPH16040562
195. Norén, E, Lindh, C, Rylander, L, Glynn, A, Axelsson, J, Littorin, M, et al. Concentrations and temporal trends in pesticide biomarkers in urine of Swedish adolescents, 2000–2017. J Expo Sci Environ Epidemiol. (2020) 30:756–67. doi: 10.1038/s41370-020-0212-8
196. Parvez, S, Gerona, RR, Proctor, C, Friesen, M, Ashby, JL, Reiter, JL, et al. Glyphosate exposure in pregnancy and shortened gestational length: a prospective Indiana birth cohort study. Environ Health. (2018) 17:23. doi: 10.1186/S12940-018-0367-0
197. Namulanda, G, Taylor, E, Maisonet, M, Boyd Barr, D, Flanders, WD, Olson, D, et al. In utero exposure to atrazine analytes and early menarche in the Avon longitudinal study of parents and children cohort. Environ Res. (2017) 156:420–5. doi: 10.1016/J.ENVRES.2017.04.004
198. Kongtip, P, Techasaensiri, B, Nankongnab, N, Adams, J, Phamonphon, A, Surach, A, et al. The impact of prenatal organophosphate pesticide exposures on Thai infant neurodevelopment. Int J Environ Res Public Health. (2017) 14:570. doi: 10.3390/IJERPH14060570
199. Guo, J, Zhang, J, Wu, C, Lv, S, Lu, D, Qi, X, et al. Associations of prenatal and childhood chlorpyrifos exposure with neurodevelopment of 3-year-old children. Environ Pollut. (2019) 251:538–46. doi: 10.1016/J.ENVPOL.2019.05.040
200. Liu, P, Wu, C, Chang, X, Qi, X, Zheng, M, and Zhou, Z. Adverse associations of both prenatal and postnatal exposure to Organophosphorous pesticides with infant neurodevelopment in an agricultural area of Jiangsu Province, China. Environ Health Perspect. (2016) 124:1637–43. doi: 10.1289/EHP196
201. Cartier, C, Warembourg, C, Le Maner-Idrissi, G, Lacroix, A, Rouget, F, Monfort, C, et al. Organophosphate insecticide metabolites in prenatal and childhood urine samples and intelligence scores at 6 years of age: results from the mother–child PELAGIE cohort (France). Environ Health Perspect. (2015) 124:674–80. doi: 10.1289/EHP.1409472
202. Sagiv, SK, Harris, MH, Gunier, RB, Kogut, KR, Harley, KG, Deardorff, J, et al. Prenatal organophosphate pesticide exposure and traits related to autism spectrum disorders in a population living in proximity to agriculture. Environ Health Perspect. (2018) 126:047012. doi: 10.1289/EHP2580
203. Jusko, TA, Van Den Dries, MA, Pronk, A, Shaw, PA, Guxens, M, Spaan, S, et al. Organophosphate pesticide metabolite concentrations in urine during pregnancy and offspring nonverbal IQ at age 6 years. Environ Health Perspect. (2019) 127:17007. doi: 10.1289/EHP3024
204. van den Dries, MA, Guxens, M, Pronk, A, Spaan, S, El Marroun, H, Jusko, TA, et al. Organophosphate pesticide metabolite concentrations in urine during pregnancy and offspring attention-deficit hyperactivity disorder and autistic traits. Environ Int. (2019) 131:105002. doi: 10.1016/J.ENVINT.2019.105002
205. Ferguson, KK, Van Den Drie, MA, Gaillar, R, Pron, A, Spaa, S, Tiemeie, H, et al. Organophosphate pesticide exposure in pregnancy in association with ultrasound and delivery measures of fetal growth. Environ Health Perspect. (2019) 127:87005. doi: 10.1289/EHP4858
206. Harley, KG, Engel, SM, Vedar, MG, Eskenazi, B, Whyatt, RM, Lanphear, BP, et al. Prenatal exposure to Organophosphorous pesticides and fetal growth: pooled results from four longitudinal birth cohort studies. Environ Health Perspect. (2015) 124:1084–92. doi: 10.1289/EHP.1409362
207. Zhang, J, Guo, J, Lu, D, Qi, X, Chang, X, Wu, C, et al. Maternal urinary carbofuranphenol levels before delivery and birth outcomes in Sheyang birth cohort. Sci Total Environ. (2018) 625:1667–72. doi: 10.1016/J.SCITOTENV.2017.10.062
208. Gilles, L, Govarts, E, Martin, LR, Andersson, AM, Appenzeller, BMR, Barbone, F, et al. Harmonization of human biomonitoring studies in Europe: characteristics of the HBM4EU-aligned studies participants. Int J Environ Res Public Health. (2022) 19:6787. doi: 10.3390/IJERPH19116787
209. Scorza, FA, Beltramim, L, and Bombardi, LM. Pesticide exposure and human health: toxic legacy. Clinics. (2023) 78:100249. doi: 10.1016/J.CLINSP.2023.100249
210. Nakayama, SF, St-Amand, A, Pollock, T, Apel, P, Bamai, YA, Barr, DB, et al. Interpreting biomonitoring data: introducing the international human biomonitoring (i-HBM) working group’s health-based guidance value (HB2GV) dashboard. Int J Hyg Environ Health. (2023) 247:114046. doi: 10.1016/J.IJHEH.2022.114046
211. Francisco, LFV, da Silva, RN, Oliveira, MA, dos Santos Neto, MF, Gonçalves, IZ, Marques, MMC, et al. Occupational exposures and risks of non-Hodgkin lymphoma: a meta-analysis. Cancers (Basel). (2023) 15:2600. doi: 10.3390/CANCERS15092600
212. Kumar, D, Sinha, SN, Rajendra, S, and Sharma, K. Assessing farmer’s exposure to pesticides and the risk for non-communicable diseases: a biomonitoring study. Sci Total Environ. (2023) 891:164429. doi: 10.1016/J.SCITOTENV.2023.164429
213. Adam, A, Marzuki, A, AbdulRahman, H, and AbdulAziz, M. The oral and intratracheal toxicities of ROUNDUP and its components to rats. Vet Hum Toxicol. (1997) 39:147–51.
214. Tauhata, SBF, Araújo, GB, Alves, SDFO, Martins, DNV, Lopes, LS, and Casaletti, L. The glyphosate controversy: an update. Arq Inst Biol (São Paulo). (2020) 87:e1002018. doi: 10.1590/1808-1657001002018
215. Lee, H-L, Kan, C-D, Tsai, C-L, Liou, M-J, and Guo, H-R. Comparative effects of the formulation of glyphosate-surfactant herbicides on hemodynamics in swine. Clin Toxicol. (2009) 47:651–8. doi: 10.1080/15563650903158862
216. Defarge, N, Takács, E, Lozano, V, Mesnage, R, Spiroux de Vendômois, J, Séralini, G-E, et al. Co-Formulants in glyphosate-based herbicides disrupt aromatase activity in human cells below toxic levels. Int J Environ Res Public Health. (2016) 13:264. doi: 10.3390/ijerph13030264
217. Mesnage, R, Benbrook, C, and Antoniou, MN. Insight into the confusion over surfactant co-formulants in glyphosate-based herbicides. Food Chem Toxicol. (2019) 128:137–45. doi: 10.1016/j.fct.2019.03.053
218. Klátyik, S, Simon, G, Oláh, M, Takács, E, Mesnage, R, Antoniou, MN, et al. Aquatic ecotoxicity of glyphosate, its formulations, and co-formulants: evidence from 2010 to 2023. Environ Sci Eur. (2024) 36:22. doi: 10.1186/s12302-024-00849-1
219. Tang, BL . Neuropathological mechanisms associated with pesticides in Alzheimer’s disease. Toxics. (2020) 8:21. doi: 10.3390/toxics8020021
220. Rahman, MA, Rahman, MS, Uddin, MJ, Mamum-Or-Rashid, ANM, Pang, M-G, and Rhim, H. Emerging risk of environmental factors: insight mechanisms of Alzheimer’s diseases. Environ Sci Pollut Res. (2020) 27:44659–72. doi: 10.1007/s11356-020-08243-z
221. Tansey, MG, Wallings, RL, Houser, MC, Herrick, MK, Keating, CE, and Joers, V. Inflammation and immune dysfunction in Parkinson disease. Nat Rev Immunol. (2022) 22:657–73. doi: 10.1038/s41577-022-00684-6
222. Sule, RO, Condon, L, and Gomes, AV. A common feature of pesticides: oxidative stress—the role of oxidative stress in pesticide-induced toxicity. Oxidative Med Cell Longev. (2022) 2022:1–31. doi: 10.1155/2022/5563759
223. Stanganelli, I, De Felici, MB, Mandel, VD, Caini, S, Raimondi, S, Corso, F, et al. The association between pesticide use and cutaneous melanoma: a systematic review and meta-analysis. J Eur Acad Dermatol Venereol. (2020) 34:691–708. doi: 10.1111/jdv.15964
224. Sharma, A, Shukla, A, Attri, K, Kumar, M, Kumar, P, Suttee, A, et al. Global trends in pesticides: a looming threat and viable alternatives. Ecotoxicol Environ Saf. (2020) 201:110812. doi: 10.1016/j.ecoenv.2020.110812
225. Chin-Chan, M, Navarro-Yepes, J, and Quintanilla-Vega, B. Environmental pollutants as risk factors for neurodegenerative disorders: Alzheimer and Parkinson diseases. Front Cell Neurosci. (2015) 9:124. doi: 10.3389/fncel.2015.00124
226. Tranfo, G . The growing importance of the human biomonitoring of exposure. Int J Environ Res Public Health. (2020) 17:3934. doi: 10.3390/IJERPH17113934
227. Hays, SM, Aylward, LL, LaKind, JS, Bartels, MJ, Barton, HA, Boogaard, PJ, et al. Guidelines for the derivation of biomonitoring equivalents: report from the biomonitoring equivalents expert workshop. Regul Toxicol Pharmacol. (2008) 51:S4–S15. doi: 10.1016/J.YRTPH.2008.05.004
228. Varshavsky, J, Smith, A, Wang, A, Hom, E, Izano, M, Huang, H, et al. Heightened susceptibility: a review of how pregnancy and chemical exposures influence maternal health. Reprod Toxicol. (2020) 92:14–56. doi: 10.1016/J.REPROTOX.2019.04.004
229. Lin, S, Li, J, Yan, X, Pei, L, and Shang, X. Maternal pesticide exposure and risk of preterm birth: a systematic review and meta-analysis. Environ Int. (2023) 178:108043. doi: 10.1016/J.ENVINT.2023.108043
230. Saillenfait, AM, Ndiaye, D, and Sabaté, JP. Pyrethroids: exposure and health effects – an update. Int J Hyg Environ Health. (2015) 218:281–92. doi: 10.1016/j.ijheh.2015.01.002
231. Vrijheid, M, Casas, M, Gascon, M, Valvi, D, and Nieuwenhuijsen, M. Environmental pollutants and child health—a review of recent concerns. Int J Hyg Environ Health. (2016) 219:331–42. doi: 10.1016/J.IJHEH.2016.05.001
232. Gillezeau, C, Lieberman-Cribbin, W, and Taioli, E. Update on human exposure to glyphosate, with a complete review of exposure in children. Environ Health. (2020) 19:115. doi: 10.1186/S12940-020-00673-Z
233. van den Dries, MA, Ferguson, KK, Keil, AP, Pronk, A, Spaan, S, Ghassabian, A, et al. Prenatal exposure to nonpersistent chemical mixtures and offspring IQ and emotional and behavioral problems. Environ Sci Technol. (2021) 55:16502–14. doi: 10.1021/ACS.EST.1C04455/SUPPL_FILE/ES1C04455_SI_001.PDF
234. Svensson, K, Gennings, C, Lindh, C, Kiviranta, H, Rantakokko, P, Wikström, S, et al. Prenatal exposures to mixtures of endocrine disrupting chemicals and sex-specific associations with children’s BMI and overweight at 5.5 years of age in the SELMA study. Environ Int. (2023) 179:108176. doi: 10.1016/J.ENVINT.2023.108176
235. Lenters, V, Iszatt, N, Forns, J, Čechová, E, Kočan, A, Legler, J, et al. Early-life exposure to persistent organic pollutants (OCPs, PBDEs, PCBs, PFASs) and attention-deficit/hyperactivity disorder: a multi-pollutant analysis of a Norwegian birth cohort. Environ Int. (2019) 125:33–42. doi: 10.1016/J.ENVINT.2019.01.020
236. Kahn, LG, Philippat, C, Nakayama, SF, Slama, R, and Trasande, L. Endocrine-disrupting chemicals: implications for human health. Lancet Diabetes Endocrinol. (2020) 8:703–18. doi: 10.1016/S2213-8587(20)30129-7
237. Bliznashka, L, Roy, A, Christiani, DC, Calafat, AM, Ospina, M, Diao, N, et al. Pregnancy pesticide exposure and child development in low- and middle-income countries: a prospective analysis of a birth cohort in rural Bangladesh and meta-analysis. PLoS One. (2023) 18:e0287089. doi: 10.1371/JOURNAL.PONE.0287089
238. Calderón-Garcidueñas, AL, Martínez-Valenzuela, M d C, and Waliszewski-Kubiak, SM. Pesticide exposure and its effects on intrauterine and postnatal development. Perinatol Reprod Hum. (2023) 37:23–30. doi: 10.24875/PER.22000014
239. Buralli, RJ, Marques, RC, and Dórea, JG. Pesticide effects on children’s growth and neurodevelopment. Curr Opin Environ Sci Health. (2023) 31:100417. doi: 10.1016/J.COESH.2022.100417
240. Bliznashka, L, Roy, A, and Jaacks, LM. Pesticide exposure and child growth in low- and middle-income countries: a systematic review. Environ Res. (2022) 215:114230. doi: 10.1016/J.ENVRES.2022.114230
241. Willenbockel, CT, Prinz, J, Dietrich, S, Marx-Stoelting, P, Weikert, C, Tralau, T, et al. A critical scoping review of pesticide exposure biomonitoring studies in overhead cultures. Toxics. (2022) 10:170. doi: 10.3390/TOXICS10040170
242. Rodríguez-Morató, J, Pozo, ÓJ, and Marcos, J. Targeting human urinary metabolome by LC-MS/MS: a review. Bioanalysis. (2018) 10:489–516. doi: 10.4155/BIO-2017-0285
243. Romberg, RW, and Lee, L. Comparison of the hydrolysis rates of Morphine-3-glucuronide and Morphine-6-glucuronide with acid and β-Glucuronidase*. J Anal Toxicol. (1995) 19:157–62. doi: 10.1093/jat/19.3.157
244. Sitasuwan, P, Melendez, C, Marinova, M, Mastrianni, KR, Darragh, A, Ryan, E, et al. Degradation of opioids and opiates during acid hydrolysis leads to reduced recovery compared to enzymatic hydrolysis. J Anal Toxicol. (2016) 40:601–7. doi: 10.1093/jat/bkw085
245. Dwivedi, P, Zhou, X, Powell, TG, Calafat, AM, and Ye, X. Impact of enzymatic hydrolysis on the quantification of total urinary concentrations of chemical biomarkers. Chemosphere. (2018) 199:256–62. doi: 10.1016/j.chemosphere.2018.01.177
246. Simonetti, G, Castellani, F, Di Filippo, P, Riccardi, C, Pomata, D, Risoluti, R, et al. Determination of Mancozeb, a pesticide used worldwide in agriculture: comparison among GC, LC, and CE. Curr Anal Chem. (2020) 16:1041–53. doi: 10.2174/1389203721666200426234827
247. Wu, Y, Guo, H, Bu, J, Tan, Y, Zhong, J, and Zhao, Q. Rapid and sensitive determination of formamidines and metabolites with HPLC-MS/MS using core-shell columns. J Chromatogr B Analyt Technol Biomed Life Sci. (2018) 1076:22–8. doi: 10.1016/J.JCHROMB.2018.01.012
248. Ye, X, Wong, LY, Bishop, AM, and Calafat, AM. Variability of urinary concentrations of bisphenol A in spot samples, first morning voids, and 24-hour collections. Environ Health Perspect. (2011) 119:983–8. doi: 10.1289/EHP.1002701
249. Khodadadi, M, and Pourfarzam, M. A review of strategies for untargeted urinary metabolomic analysis using gas chromatography-mass spectrometry. Metabolomics. (2020) 16:66. doi: 10.1007/S11306-020-01687-X
250. MacPherson, S, Arbuckle, TE, and Fisher, M. Adjusting urinary chemical biomarkers for hydration status during pregnancy. J Expo Sci Environ Epidemiol. (2018) 28:481–93. doi: 10.1038/s41370-018-0043-z
251. Sanders, CL, Rattinger, GB, Deberard, MS, Hammond, AG, Wengreen, H, Kauwe, JSK, et al. Interaction between physical activity and genes related to Neurotrophin signaling in late-life cognitive performance: the Cache County study. J Gerontol A Biol Sci Med Sci. (2020) 75:1633–42. doi: 10.1093/gerona/glz200
252. Pesticide Action Network . PesticideInfo – Global Ban Map; (2022). Available at: https://www.pesticideinfo.org/pesticide-maps/global-ban (Accessed March 12, 2024)
253. Hvězdová, M, Kosubová, P, Košíková, M, Scherr, KE, Šimek, Z, Brodský, L, et al. Currently and recently used pesticides in central European arable soils. Sci Total Environ. (2018) 613–614:361–70. doi: 10.1016/j.scitotenv.2017.09.049
254. Finger, R . Take a holistic view when making pesticide policies stricter. Nature. (2018) 556:174–4. doi: 10.1038/d41586-018-04166-5
255. Keswani, C, Dilnashin, H, Birla, H, Roy, P, Tyagi, RK, Singh, D, et al. Global footprints of organochlorine pesticides: a pan-global survey. Environ Geochem Health. (2022) 44:149–77. doi: 10.1007/s10653-021-00946-7
256. Wang, L, Zhang, Z-F, Liu, L-Y, Zhu, F-J, and Ma, W-L. National-scale monitoring of historic used organochlorine pesticides (OCPs) and current used pesticides (CUPs) in Chinese surface soil: old topic and new story. J Hazard Mater. (2023) 443:130285. doi: 10.1016/j.jhazmat.2022.130285
257. Affum, AO, Acquaah, SO, Osae, SD, and Kwaansa-Ansah, EE. Distribution and risk assessment of banned and other current-use pesticides in surface and groundwaters consumed in an agricultural catchment dominated by cocoa crops in the Ankobra Basin, Ghana. Sci Total Environ. (2018) 633:630–40. doi: 10.1016/j.scitotenv.2018.03.129
258. Connolly, A, Coggins, MA, and Koch, HM. Human biomonitoring of glyphosate exposures: state-of-the-art and future research challenges. Toxics. (2020) 8:60. doi: 10.3390/toxics8030060
259. Samsidar, A, Siddiquee, S, and Shaarani, SM. A review of extraction, analytical and advanced methods for determination of pesticides in environment and foodstuffs. Trends Food Sci Technol. (2018) 71:188–201. doi: 10.1016/j.tifs.2017.11.011
260. Food and Agriculture Organization of the United Nations . International code of conduct on pesticide management guidelines on pesticide legislation; (2015). Available at: http://www.who.int/about/licensing/copyright_form/en/index.html (Accessed March 12, 2024)
261. Handford, CE, Elliott, CT, and Campbell, K. A review of the global pesticide legislation and the scale of challenge in reaching the global harmonization of food safety standards. Integr Environ Assess Manag. (2015) 11:525–36. doi: 10.1002/ieam.1635
262. Kubiak-Hardiman, P, Haughey, SA, Meneely, J, Miller, S, Banerjee, K, and Elliott, CT. Identifying gaps and challenges in global pesticide legislation that impact the protection of consumer health: Rice as a case study. Expo Health. (2023) 15:597–618. doi: 10.1007/s12403-022-00508-x
263. Bayoumi, AE . Counterfeit pesticides. ACS Chem Health Saf. (2021) 28:232–7. doi: 10.1021/acs.chas.1c00010
264. de Moraes, RF . Constructing a transnational crime: pesticide smuggling in Brazil. Crime Law Soc Change. (2022) 78:379–404. doi: 10.1007/s10611-022-10026-1
265. Alcántara-de la Cruz, R, Cruz-Hipolito, HE, Domínguez-Valenzuela, JA, and De Prado, R. Glyphosate ban in Mexico: potential impacts on agriculture and weed management. Pest Manag Sci. (2021) 77:3820–31. doi: 10.1002/ps.6362
266. Płonka, M, Walorczyk, S, and Miszczyk, M. Chromatographic methods for the determination of active substances and characterization of their impurities in pesticide formulations. TrAC Trends Anal Chem. (2016) 85:67–80. doi: 10.1016/j.trac.2016.03.011
267. Health Canada . Guidance for the registration of non-conventional pest control products. (2023). Available at: https://www.canada.ca/en/health-canada/services/consumer-product-safety/reports-publications/pesticides-pest-management/policies-guidelines/registration-non-conventional-pest-control-products.html (Accessed March 12, 2024).
268. Abdollahdokht, D, Gao, Y, Faramarz, S, Poustforoosh, A, Abbasi, M, Asadikaram, G, et al. Conventional agrochemicals towards nano-biopesticides: an overview on recent advances. Chem Biol Technol Agric. (2022) 9:13. doi: 10.1186/s40538-021-00281-0
269. Wang, D, Saleh, NB, Byro, A, Zepp, R, Sahle-Demessie, E, Luxton, TP, et al. Nano-enabled pesticides for sustainable agriculture and global food security. Nat Nanotechnol. (2022) 17:347–60. doi: 10.1038/s41565-022-01082-8
270. Díaz-Criollo, S, Palma, M, Monroy-García, AA, Idrovo, AJ, Combariza, D, and Varona-Uribe, ME. Chronic pesticide mixture exposure including paraquat and respiratory outcomes among Colombian farmers. Ind Health. (2020) 58:15–21. doi: 10.2486/INDHEALTH.2018-0111
271. Konthonbut, P, Kongtip, P, Nankongnab, N, Tipayamongkholgul, M, Yoosook, W, and Woskie, S. Paraquat exposure of pregnant women and neonates in agricultural areas in Thailand. Int J Environ Res Public Health. (2018) 15:1163. doi: 10.3390/IJERPH15061163
272. Cox, C, and Zeiss, M. Health, pesticide adjuvants, and inert ingredients: California case study illustrates need for data access. Environ Health Perspect. (2022) 130:85001. doi: 10.1289/EHP10634
273. dos Santos, IC, da Silva, JT, Rohr, P, Lengert, AH, de Lima, MA, Kahl, VFS, et al. Genomic instability evaluation by BMCyt and telomere length in Brazilian family farmers exposed to pesticides. Mutat Res Genet Toxicol Environ Mutagen. (2022) 878:503479. doi: 10.1016/J.MRGENTOX.2022.503479
274. Ren, Z, and Jiang, H. Risk cognition, agricultural cooperatives training, and farmers’ pesticide overuse: Evidence from Shandong Province, China. Front Public Health. (2022) 10:1032862. doi: 10.3389/FPUBH.2022.1032862
275. Tessema, RA, Nagy, K, and Ádám, B. Occupational and environmental pesticide exposure and associated health risks among pesticide applicators and non-applicator residents in rural Ethiopia. Front Public Health. (2022) 10:1017189. doi: 10.3389/FPUBH.2022.1017189
276. Hayden, KM, Norton, MC, Darcey, D, Østbye, T, Zandi, PP, Breitner, JCS, et al. Occupational exposure to pesticides increases the risk of incident AD. Neurology. (2010) 74:1524–30. doi: 10.1212/WNL.0b013e3181dd4423
277. Chen, W-L, Lin, G-L, Lin, Y-J, Su, T-Y, Wang, C-C, and Wu, W-T. Cancer risks in a population-based study of agricultural workers: results from the Taiwan’s farmers and health cohort study. Scand J Work Environ Health. (2023) 49:419–27. doi: 10.5271/sjweh.4106
Keywords: biomonitoring, agrochemical, occupational exposure, risk assessment, GC–MS/MS, LC–MS/MS, toxicity, health outcome
Citation: Birolli WG, Lanças FM, dos Santos Neto &J and Silveira HCS (2024) Determination of pesticide residues in urine by chromatography-mass spectrometry: methods and applications. Front. Public Health. 12:1336014. doi: 10.3389/fpubh.2024.1336014
Edited by:
Mo Salman, Colorado State University, United StatesReviewed by:
Rafael Valencia-Quintana, Autonomous University of Tlaxcala, MexicoConcettina Fenga, University of Messina, Italy
Copyright © 2024 Birolli, Lanças, dos Santos Neto and Silveira. This is an open-access article distributed under the terms of the Creative Commons Attribution License (CC BY). The use, distribution or reproduction in other forums is permitted, provided the original author(s) and the copyright owner(s) are credited and that the original publication in this journal is cited, in accordance with accepted academic practice. No use, distribution or reproduction is permitted which does not comply with these terms.
*Correspondence: Willian Garcia Birolli, d2lsbGlhbmJpcm9sbGlAZ21haWwuY29t