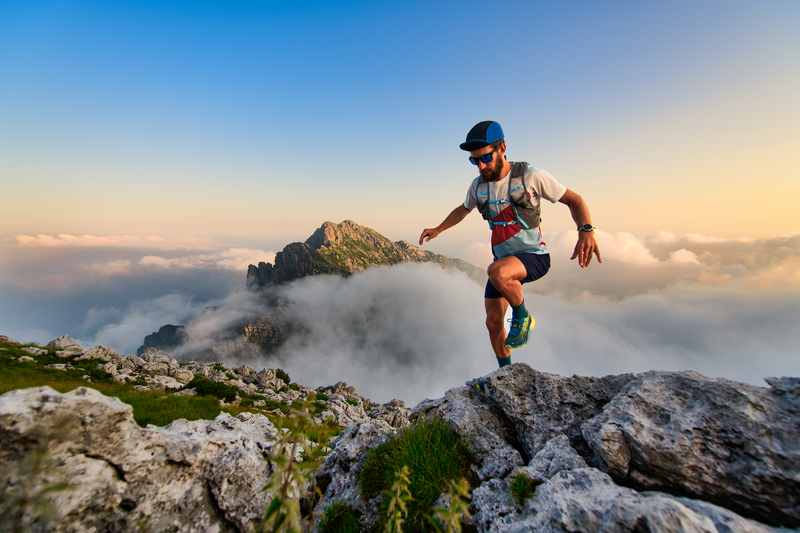
95% of researchers rate our articles as excellent or good
Learn more about the work of our research integrity team to safeguard the quality of each article we publish.
Find out more
OPINION article
Front. Public Health , 10 January 2024
Sec. Aging and Public Health
Volume 11 - 2023 | https://doi.org/10.3389/fpubh.2023.1330131
This article is part of the Research Topic Trends, Trajectories and Predictors of Healthy Aging View all 21 articles
Healthy lifestyles, such as those that include regular physical activity and a balanced diet, are a powerful means to prevent chronic disease and age-related functional decline. A common denominator of health improvements resulting from good exercise and diet habits is the optimization of metabolic processes. These processes include energy metabolism and, thus, the activity of mitochondria. Mitochondria represent hubs not only of cellular metabolism but also of the regulation of redox states, inflammatory response, and immunity, as well as many other cellular features (1). Mitochondria have emerged as highly flexible organelles that, quickly—and sometimes persistently—adapt to changing conditions in response to systemic or cellular challenges. Next to exercise and diets that promote mitochondrial health, transient exposures to environmental stressors, such as to altitude/hypoxia or extreme temperatures, also induce mitochondrial adaptations.
In this paper, we discuss how different systemic and cellular challenges trigger specific and overlapping mitochondrial responses that—under the right conditions—may translate into protective mitochondrial adaptations (2). We specifically focus on adaptations in skeletal muscle and sarcopenia, the age-related loss of skeletal muscle mass, strength, and function (3). Such responses rely on mechanisms such as mitochondrial stress responses and quality control; therefore, these mechanisms are believed to be required to maintain mitochondrial health (4). The resulting adaptations increase the capacity of mitochondria to respond to future stressors (e.g., altered oxygen or substrate availability), which otherwise might trigger pathological processes. Considering potential synergistic/anti-synergistic and complementary/competitive effects among lifestyle factors and environmental challenges on mitochondria, we argue that recommendations can be developed to increase performance, prevent sarcopenia, and improve healthy aging.
Exercise represents a potent measure to foster healthy aging and to prevent and/or treat a large number of chronic diseases, including cardiovascular, pulmonary, neurological, metabolic, musculoskeletal diseases, and cancer (5). Those benefits, and in particular those promoted by endurance type training, are closely related to improved mitochondrial quality control (MQC, including mitophagy, the clearance of dysfunctional mitochondria), mitochondrial content, respiration, and dynamics in striated muscles (i.e., skeletal and heart muscle) (6–8). Regular exercise is thought to benefit mitochondria depending on the exercise type and intensity, although the specific determinants for mitochondrial improvements are still under debate, also due to the high diversity of exercise interventions and study populations (8, 9).
A recent systematic review reinforced the favorable effects of exercise training in older adults on mitochondrial quality, density, dynamics, oxidative, and antioxidant capacity, which varied according to the exercise type (9): While improvements of the mitochondrial antioxidant capacity appear to be important consequences of endurance exercise, resistance training seems to be particularly beneficial for mitochondrial density and dynamics.
Life-long high-volume exercise training specifically improved mitochondrial volume and network connectivity in skeletal muscle and associated oxidative capacity in older adults (10). Moreover, it preserved mitochondrial morphology, Ca2+ handling, and ATP production, contributing to the maintenance of skeletal muscle function in older individuals (11).
In subjects suffering from sarcopenia, more intense aerobic exercise protocols may more efficiently improve mitochondrial biogenesis (12); for example, exercise increased the mRNA levels of the mitochondrial biogenesis-related transcription factor peroxisome proliferator-activated receptor γ coactivator 1 α (PGC-1α) 10.2-fold at 80% of VO2max (maximum rate of oxygen consumption), but only 3.8-fold at 40% of VO2max (13). Low-volume high-intensity interval training (HIIT) represents a time-efficient alternative to improving skeletal muscle mass and cardiorespiratory fitness (CRF) in individuals, and even in octogenarians with co-morbidities, probably by increasing the mitochondrial oxidative phosphorylation capacity in skeletal muscle (14). Comparisons between HIIT, resistance training (RT), and the combination of HIIT and RT revealed that 12 weeks of HIIT enhanced mitochondrial content and resulted in protein changes in skeletal muscle indicative of increased mitochondrial fusion, while smaller effects were seen after combined training and (surprisingly) no effects after RT (15). These changes were associated with improved mitochondrial respiration, CRF, and insulin sensitivity in populations of untrained but lean young (18–30 years) and older (65–80 years) adults (15). Conversely, long-term RT (over 6 months) was found to considerably increase mitochondrial volume density in older individuals (16). In one recent study, 12 weeks of HIIT combined with L-citrulline supplementation increased markers of mitochondrial biogenesis, mitochondrial fusion and mitophagy in obese older adults and acted synergistically for improving muscle strength and muscle quality when compared with HIIT alone (17).
Taken together, these study findings indicate that exercise has the potential to improve or maintain mitochondrial content and health in skeletal muscle. This has been associated with healthy aging in older subjects provided that the training stimulus is appropriate, and higher intensities seeming to be more effective. Thus, it is crucial to individually tailor exercise interventions, considering individual conditions like existing diseases, exercise preferences and tolerability, training targets, as well as nutritional and supplementation strategies to support exercise-induced adaptations.
The role of nutritional supplementation on sarcopenia risk and related outcomes (i.e., muscle strength, muscle mass, and performance) has been extensively summarized in previous reviews (18–21) highlighting the anti-aging potential of practicing a Mediterranean-style diet and demonstrating some evidence for the benefits of protein supplementation, especially in sarcopenic/frail older adults, when combined with RT. Frailty is a multidimensional condition that is closely related to sarcopenia (22) and mainly characterized by decreased functional reserves and stress resistance, and increased vulnerability (23). The widely used Fried frailty phenotype assesses physical frailty through five criteria: unintentional weight loss; weakness or poor handgrip strength; self-reported exhaustion; slow walking speed; and low physical activity (24).
Recently, the ProMuscle in Practice study demonstrated that increasing the amount of protein ingested per meal (≥25 g) along with twice-weekly progressive RT over a 12-week intensive support intervention was effective for counteracting sarcopenia in community-dwelling older adults who were frail or pre-frail based on Fried frailty criteria or who experienced strength loss (25). The recommended daily protein intakes are 1.0–1.2 g/kg body weight (BW) for healthy older individuals and 1.2–1.5 g/kg/BW for geriatric patients, containing ~2.5 g of leucine, to stimulate muscle protein synthesis (26). In addition, exercise and higher protein intake are recommended during weight loss, to avoid muscle wasting (27).
Caloric restriction, a lifestyle strategy to mitigate obesity and metabolic disease, which typically involves the consumption of 20–40% lower calories, shows beneficial effects on mitochondrial mass and function (28). However, this approach could also bring about unwanted reductions in lean mass, especially when the protein needs are not achieved, and may contradict dietary practices for optimizing skeletal muscle health in older persons (29). Thus, interventions to enhance the loss of fat while preserving muscle mass during energy restriction are of great importance to prevent sarcopenia in overweight older adults. Data indicate that, even in the presence of energy restriction, performance of RT with elevated daily protein ingestion (1.3 g/kg/BW) increases muscle protein synthesis and potentially supports muscle mass preservation during weight loss in obese older adults. In addition, short-term RT (over 2 weeks) stimulated mitochondrial protein synthesis as compared with energy restriction alone (30).
The few clinical trials of nutritional interventions on mitochondrial health in older healthy people or those with or at risk of malnutrition suggest that nutritional supplementation with branched-chain amino acids (BCAA) alone (31) or combined with 800 IU vitamin D3 per day (32) and omega-3 poly-unsaturated fatty acids (dosages from 3.3 to 3.9 g/day over a 4–6-month time period) (33, 34) may be useful in the prevention of sarcopenia. These strategies boost mitochondrial bioenergetic and redox capacities, potentially explaining the amelioration of muscular performance in older adults in the absence of exercise, which reflects the real-life situation of most community-dwelling older adults (18). Beta-hydroxy-beta-methylbutyrate (3 g/day), a metabolite of leucine, has been shown to concomitantly preserve muscle mass and mitochondrial gene expression in healthy older adults during 10 days of bed rest (35). Moreover, this supplementation improved mitochondrial content and dynamics over an 8-week RT rehabilitation period as compared with the placebo control (35).
Some micronutrients, such as zinc and selenium, may also contribute to mitochondrial health and reduce oxidative stress in sarcopenia, but the evidence is still too weak to promote these nutrients as treatments for sarcopenia (36).
Finally, probiotics may actively modulate the risk and progression of sarcopenia: Preclinical research findings suggest that Lactobacillus casei Shirota supplementation for 12 weeks enhances muscle function potentially through the gut–muscle axis via mitochondrial signaling (37, 38). Promoting a healthy gut microbiota also improves the bioavailability of dietary polyphenols. These compounds have been shown to benefit skeletal muscle cells and tissues, thus potentially representing effective components of a treatment strategy for reducing or reversing sarcopenia (39). Indeed, two studies detected improvements in mitochondrial density and oxidative phosphorylation capacity, accompanied by enhanced skeletal muscle morphology and better mobility in aged persons after 12 weeks of admission of resveratrol (500 mg/day) combined with exercise training (40, 41).
Taken together, the current evidence suggests that dietary interventions can be effective in the prevention and treatment of sarcopenia by improving various aspects of mitochondrial health. Adherence to a Mediterranean diet, which favors a high intake of proteins, fibers, and polyphenols, and nutritional supplementation with BCAA, omega-3 polyunsaturated fatty acids, and vitamin D should be considered in older adults to support exercise-induced adaptations and muscle health. Overall, it can be concluded that the combination of diet and exercise interventions due to synergistic and complementary effects may be is the most effective approach to protect mitochondria to ameliorate sarcopenia.
Epidemiological studies reveal that living at moderate altitudes (1,000–2,000 m) may increase human life expectancy (42, 43). Reduced mortality from cardiovascular diseases and certain cancer types are thought to be main mediators of this effect, and they thought to be a consequence of the lower oxygen partial pressure, and thus reduced oxygen availability (hypoxia), at these altitudes (43). This hypothesis is supported by evidence that exposure to mild chronic continuous environmental hypoxia extends the lifespan of various species, including worms (44), fruit flies (45), and mice (46).
Accumulating evidence suggests that brief and repeated exposures to mild or moderate hypoxia (hypoxia conditioning, HC) also induce physiological and cellular adaptations which protect individuals from subsequent, more severe hypoxic or ischemic insults and possibly from age-related diseases (47, 48). In contrast to the potential beneficial impact on healthy aging and life expectancy conferred by exposure to mild or moderate hypoxia, exposure to more severe hypoxia may even accelerate aging, potentially due to the augmentation of oxidative stress, inflammation, and mitochondrial dysfunction (48). Thus, major health benefits from hypoxia exposure may not result from hypoxia per se but rather from adaptations initiated by exposures to hypoxia at appropriate intensities, durations, and frequencies (49).
Mitochondria are key to adaptations involved in the induction of cellular stress responses, the upregulation of antioxidant pathways, and the optimization and reduction of oxidative metabolism rates (4, 50). Interventional studies have revealed the preventive and therapeutic effects and promotion of healthy aging by HC by, for example, improving exercise tolerance and cognitive performance in healthy older adults or those with pre-existing cardiovascular, pulmonary (51), or age-related neurological deficits (52). For example, after 3 weeks of intermittent hypoxia, VO2max increased by 6.2% in older men with and without coronary artery disease while no change was observed in the normoxic control (53).
Moreover, exercise training in athletes (twelve high-intensity treadmill sessions over 6 weeks, in addition to regular trainings) under normobaric hypoxic conditions (FiO2: 14.5%, 3,000 m) increased the gene expression of the mitochondrial biogenesis regulators PGC-1α and transcription factor A and elevated mitochondrial enzyme activity (i.e., of citrate synthase and cytochrome oxidases 1 and 4) (54). A recent meta-analysis revealed greater improvements in the body fat and body mass index of middle-aged and older adults when exercise was performed under normobaric hypoxic conditions as compared to normoxic conditions (55). The authors suggest that changes in cellular energy production and mitochondrial protein synthesis may be potential mechanisms associated with modifications in body composition (55). However, whether exercising in hypoxia benefits older people more than exercising in normoxia remains to be elucidated. A recent study in sedentary older individuals found no differences in mitochondrial and functional outcomes between these modalities after 8 weeks of aerobic exercise (56).
In summary, together with exercise and dietary interventions, HC represents a promising strategy to counteract skeletal and cardiac muscle dysfunction and conditions of sarcopenia, and thus to promote healthy aging. Which HC programs optimally improve specific mitochondrial functions and muscle health remain to be identified and will need to consider individual circumstances (e.g., physical and mental performance capabilities, co-morbidities, pharmacological therapy, and responsiveness to hypoxia exposure). A selection of studies linking exercise, dietary, hypoxia and combined interventions to mitochondrial and muscle or fitness outcomes are summarized in Table 1.
Increasing mitochondrial deficits (58), the associated oxidative stress (58), and inflammatory processes (59) are central processes in aging. Accordingly, mitochondrial health and the associated oxidative capacity also declines with age in skeletal muscle. This decline is correlated to reduced muscle performance and CRF (60, 61). It is thus plausible that aging mitochondria are involved in the development of age-related sarcopenia, although the specific concerned mitochondrial deficits (e.g., oxidative phosphorylation, biogenesis, dynamics, quality control) remain to be elucidated (62). Importantly, direct data on mitochondrial outcomes of lifestyle-interventions in sarcopenia are scarce and experimental confirmation on potential preventive and symptomatic benefits are urgently required.
The overlapping but also seemingly differential mitochondrial benefits of different lifestyle interventions might be harnessed to design optimized mixed lifestyle interventions that counteract the development of sarcopenia or alleviate its symptoms. These interactions among lifestyle factors and their results on mitochondrial activity, however, are still poorly known and controversially discussed. Different exercise modalities and intensities, for example, may differentially improve the mitochondrial biogenesis (resistance training, moderate endurance training) and dynamics (resistance training), antioxidant capacity (moderate endurance training), quality control/mitophagy (moderate endurance training), or oxidative phosphorylation capacity (intensive endurance training) (8, 9).
Like exercise, a mild caloric restriction and certain nutrients may help to preserve specific facets of mitochondrial health and might be suitable as a counteracting strategy for sarcopenia. These include the use of beta-hydroxy-beta-methylbutyrate supplements, which seem to effectively promote mitochondrial density and dynamics (35). In combination with exercise, resveratrol also appears to improve mitochondrial density and oxidative phosphorylation (40, 41).
The controlled variation in hypoxia levels (by climbing to different altitudes, spending time in hypoxia chambers/tents, breathing of defined gas mixtures, or performing breathing exercises) may also modulate specific mitochondrial functions, depending on the severity, duration, and frequency of the exposure (50). Cellular adaptations to hypoxia include increased oxidative phosphorylation efficiency and antioxidative capacities, but also enhanced cellular oxygen supply due to the improved oxygen transport in the blood (e.g., as a result of erythropoietin upregulation) and angiogenesis, as well as glucose transport and glycolysis upregulation, which reduce the reliance of ATP production on oxygen levels (50).To take full advantage of the potentially complementary and synergistic benefits of exercise, dietary strategies and hypoxia exposure, the distinct effects of these interventions need to be better understood (Figure 1).
Figure 1. Lifestyle interventions modulating mitochondrial and skeletal muscle health to prevent sarcopenia. Sedentary aging is associated with increasing mitochondrial deficits and functional decline of skeletal muscle and favors the development of sarcopenia, while the adoption of a healthy lifestyle provides some protection (A). On the molecular level, this protection is believed to be mediated by improvements in various mitochondrial functions and related oxygen utilization factors (B). Specific aspects of mitochondria and oxygen utilization discussed in the text are indicated by colored squares and letters a–e in (B), where the colors/letters correspond to lifestyle/environmental factors shown in (C).
Very few studies report small or no effects of higher physical activity levels and/or healthy dietary behaviors, such as Mediterranean diet, on sarcopenia prevalence [e.g., (63)], suggesting limitations of the preventive potential of healthy lifestyles.
Specifically, sarcopenic or malnourished older adults tend to develop an anabolic resistance to three fundamental anabolic stimuli [i.e., insulin signaling, BCAA (primarily leucine) blood concentration, and physical activity]. For these older people (64), individually optimized dietary protein intake combined with RT are required to maintain or improve muscular strength and mitochondrial function with aging. Physical activity, and predominantly endurance exercise, often potently counteracts sedentary aging associated with mitochondrial dysfunction, insulin resistance and obesity. But combinations with adequate dietary strategies, RT and hypoxia can further optimize mitochondrial health and muscle performance. Well-calibrated RT benefits almost all older people (65) and reduces the risk of sarcopenia in older adults adhering to aerobic moderate-to-vigorous physical activity guidelines even further (66).
Based on the high complexity of outcomes in lifestyle changes, the investigation of combined approaches (e.g., diet and exercise interventions) are challenging and individualized combinations of different training types and dietary regimes accompanied by monitoring and continuous program adaption will be important to guarantee success. Person-centered strategies are especially important for vulnerable populations to ensure exercise and/or hypoxia benefits and an appropriate nutritional status, while balancing these factors with associated risks (injury risk, oxidative stress, immune system consequences, and inflammation).
Other lifestyle and environmental factors that have not been considered in this review but may be similarly important (e.g., sleep or heat/cold acclimatization) also require further study. The complex physiological consequences of lifestyle and environmental changes also complicate efforts to compare the associated mitochondrial effects. The specific strategy outcomes, meanwhile, are determined by the application modalities (or supplement type), dose, and individual characteristics of the recipient (e.g., genetic makeup, fitness and health status). However, availability of experimental (e.g., OMICS) approaches together with increasingly powerful analytical/bioinformatic tools will pave the way for the development of a person-centered lifestyle medicine that can prevent sarcopenia and other age-related diseases.
JB: Writing—original draft, Writing—review & editing. BS: Writing—original draft, Writing—review & editing. MB: Writing—original draft, Writing—review & editing.
The author(s) declare that no financial support was received for the research, authorship, and/or publication of this article.
The authors declare that the research was conducted in the absence of any commercial or financial relationships that could be construed as a potential conflict of interest.
The author(s) declared that they were an editorial board member of Frontiers, at the time of submission. This had no impact on the peer review process and the final decision.
All claims expressed in this article are solely those of the authors and do not necessarily represent those of their affiliated organizations, or those of the publisher, the editors and the reviewers. Any product that may be evaluated in this article, or claim that may be made by its manufacturer, is not guaranteed or endorsed by the publisher.
1. Monzel AS, Enríquez JA, Picard M. Multifaceted mitochondria: moving mitochondrial science beyond function and dysfunction. Nat Metab. (2023) 5:546–62. doi: 10.1038/s42255-023-00783-1
2. Eisner V, Picard M, Hajnóczky G. Mitochondrial dynamics in adaptive and maladaptive cellular stress responses. Nat Cell Biol. (2018) 20:755–65. doi: 10.1038/s41556-018-0133-0
3. Cruz-Jentoft AJ, Bahat G, Bauer J, Boirie Y, Bruyère O, Cederholm T, et al. Sarcopenia: revised European consensus on definition and diagnosis. Age Ageing. (2019) 48:16–31. doi: 10.1093/ageing/afy169
4. Burtscher J, Romani M, Bernardo G, Popa T, Ziviani E, Hummel FC, et al. Boosting mitochondrial health to counteract neurodegeneration. Prog Neurobiol. (2022) 215:102289. doi: 10.1016/j.pneurobio.2022.102289
5. Pedersen BK, Saltin B. Exercise as medicine - evidence for prescribing exercise as therapy in 26 different chronic diseases. Scand J Med Sci Sports. (2015) 3(25 Suppl.):1–72. doi: 10.1111/sms.12581
6. Sorriento D, Di Vaia E, Iaccarino G. Physical exercise: a novel tool to protect mitochondrial health. Front Physiol. (2021) 12:660068. doi: 10.3389/fphys.2021.660068
7. Philp AM, Saner NJ, Lazarou M, Ganley IG, Philp A. The influence of aerobic exercise on mitochondrial quality control in skeletal muscle. J Physiol. (2021) 599:3463–76. doi: 10.1113/JP279411
8. Burtscher J, Burtscher M, Millet GP. The central role of mitochondrial fitness on antiviral defenses: an advocacy for physical activity during the COVID-19 pandemic. Redox Biol. (2021) 43:101976. doi: 10.1016/j.redox.2021.101976
9. Lippi L, de Sire A, Mezian K, Curci C, Perrero L, Turco A, et al. Impact of exercise training on muscle mitochondria modifications in older adults: a systematic review of randomized controlled trials. Aging Clin Exp Res. (2022) 34:1495–510. doi: 10.1007/s40520-021-02073-w
10. Ringholm S, Gudiksen A, Frey Halling J, Qoqaj A, Meizner Rasmussen P, Prats C, et al. Impact of aging and lifelong exercise training on mitochondrial function and network connectivity in human skeletal muscle. J Gerontol A Biol Sci Med Sci. (2023) 78:373–83. doi: 10.1093/gerona/glac164
11. Zampieri S, Pietrangelo L, Loefler S, Fruhmann H, Vogelauer M, Burggraf S, et al. Lifelong physical exercise delays age-associated skeletal muscle decline. J Gerontol A Biol Sci Med Sci. (2015) 70:163–73. doi: 10.1093/gerona/glu006
12. Harper C, Gopalan V, Goh J. Exercise rescues mitochondrial coupling in aged skeletal muscle: a comparison of different modalities in preventing sarcopenia. J Transl Med. (2021) 19:71. doi: 10.1186/s12967-021-02737-1
13. Egan B, Carson BP, Garcia-Roves PM, Chibalin AV, Sarsfield FM, Barron N, et al. Exercise intensity-dependent regulation of peroxisome proliferator-activated receptor coactivator-1 mRNA abundance is associated with differential activation of upstream signalling kinases in human skeletal muscle. J Physiol. (2010) 588:1779–90. doi: 10.1113/jphysiol.2010.188011
14. Blackwell JEM, Gharahdaghi N, Brook MS, Watanabe S, Boereboom CL, Doleman B, et al. The physiological impact of high-intensity interval training in octogenarians with comorbidities. J Cachexia Sarcopenia Muscle. (2021) 12:866–79. doi: 10.1002/jcsm.12724
15. Ruegsegger GN, Pataky MW, Simha S, Robinson MM, Klaus KA, Nair KS. High-intensity aerobic, but not resistance or combined, exercise training improves both cardiometabolic health and skeletal muscle mitochondrial dynamics. J Appl Physiol (1985). (2023) 135:763–74. doi: 10.1152/japplphysiol.00405.2023
16. Jubrias SA, Esselman PC, Price LB, Cress ME, Conley KE. Large energetic adaptations of elderly muscle to resistance and endurance training. J Appl Physiol (1985). (2001) 90:1663–70. doi: 10.1152/jappl.2001.90.5.1663
17. Marcangeli V, Youssef L, Dulac M, Carvalho LP, Hajj-Boutros G, Reynaud O, et al. Impact of high-intensity interval training with or without l-citrulline on physical performance, skeletal muscle, and adipose tissue in obese older adults. J Cachexia Sarcopenia Muscle. (2022) 13:1526–40. doi: 10.1002/jcsm.12955
18. Cochet C, Belloni G, Buondonno I, Chiara F, D'Amelio P. The role of nutrition in the treatment of sarcopenia in old patients: from restoration of mitochondrial activity to improvement of muscle performance, a systematic review. Nutrients. (2023) 15:3703. doi: 10.3390/nu15173703
19. Gielen E, Beckwée D, Delaere A, De Breucker S, Vandewoude M, Bautmans I. Nutritional interventions to improve muscle mass, muscle strength, and physical performance in older people: an umbrella review of systematic reviews and meta-analyses. Nutr Rev. (2021) 79:121–47. doi: 10.1093/nutrit/nuaa011
20. Robinson S, Granic A, Cruz-Jentoft AJ, Sayer AA. The role of nutrition in the prevention of sarcopenia. Am J Clin Nutr. (2023) 118:852–64. doi: 10.1016/j.ajcnut.2023.08.015
21. Strasser B, Wolters M, Weyh C, Krüger K, Ticinesi A. The effects of lifestyle and diet on gut microbiota composition, inflammation and muscle performance in our aging society. Nutrients. (2021) 13:2045. doi: 10.3390/nu13062045
22. Álvarez-Bustos A, Carnicero-Carreño JA, Davies B, Garcia-Garcia FJ, Rodríguez-Artalejo F, Rodríguez-Mañas L, et al. Role of sarcopenia in the frailty transitions in older adults: a population-based cohort study. J Cachexia Sarcopenia Muscle. (2022) 13:2352–60. doi: 10.1002/jcsm.13055
23. Morley JE, Vellas B, van Kan GA, Anker SD, Bauer JM, Bernabei R, et al. Frailty consensus: a call to action. J Am Med Dir Assoc. (2013) 14:392–7. doi: 10.1016/j.jamda.2013.03.022
24. Fried LP, Tangen CM, Walston J, Newman AB, Hirsch C, Gottdiener J, et al. Frailty in older adults: evidence for a phenotype. J Gerontol A Biol Sci Med Sci. (2001) 56:M146–56. doi: 10.1093/gerona/56.3.M146
25. van Dongen RJI, Haveman-Nies A, Doets EL, Dorhout BG, de Groot L. Effectiveness of a diet and resistance exercise intervention on muscle health in older adults: promuscle in practice. J Am Med Dir Assoc. (2020) 21:1065–72.e3. doi: 10.1016/j.jamda.2019.11.026
26. Bauer J, Biolo G, Cederholm T, Cesari M, Cruz-Jentoft AJ, Morley JE, et al. Evidence-based recommendations for optimal dietary protein intake in older people: a position paper from the PROT-AGE Study Group. J Am Med Dir Assoc. (2013) 14:542–59. doi: 10.1016/j.jamda.2013.05.021
27. Pasiakos SM, Cao JJ, Margolis LM, Sauter ER, Whigham LD, McClung JP, et al. Effects of high-protein diets on fat-free mass and muscle protein synthesis following weight loss: a randomized controlled trial. FASEB J. (2013) 27:3837–47. doi: 10.1096/fj.13-230227
28. López-Lluch G, Hunt N, Jones B, Zhu M, Jamieson H, Hilmer S, et al. Calorie restriction induces mitochondrial biogenesis and bioenergetic efficiency. Proc Natl Acad Sci USA. (2006) 103:1768–73. doi: 10.1073/pnas.0510452103
29. Robinson SM, Reginster JY, Rizzoli R, Shaw SC, Kanis JA, Bautmans I, et al. Does nutrition play a role in the prevention and management of sarcopenia? Clin Nutr. (2018) 37:1121–32. doi: 10.1016/j.clnu.2017.08.016
30. Murphy CH, Shankaran M, Churchward-Venne TA, Mitchell CJ, Kolar NM, Burke LM, et al. Effect of resistance training and protein intake pattern on myofibrillar protein synthesis and proteome kinetics in older men in energy restriction. J Physiol. (2018) 596:2091–120. doi: 10.1113/JP275246
31. Buondonno I, Sassi F, Carignano G, Dutto F, Ferreri C, Pili FG, et al. From mitochondria to healthy aging: the role of branched-chain amino acids treatment: MATeR a randomized study. Clin Nutr. (2020) 39:2080–91. doi: 10.1016/j.clnu.2019.10.013
32. Grootswagers P, Smeets E, Oteng AB, Groot L. A novel oral nutritional supplement improves gait speed and mitochondrial functioning compared to standard care in older adults with (or at risk of) undernutrition: results from a randomized controlled trial. Aging. (2021) 13:9398–418. doi: 10.18632/aging.202912
33. Lalia AZ, Dasari S, Robinson MM, Abid H, Morse DM, Klaus KA, et al. Influence of omega-3 fatty acids on skeletal muscle protein metabolism and mitochondrial bioenergetics in older adults. Aging. (2017) 9:1096–129. doi: 10.18632/aging.101210
34. Yoshino J, Smith GI, Kelly SC, Julliand S, Reeds DN, Mittendorfer B. Effect of dietary n-3 PUFA supplementation on the muscle transcriptome in older adults. Physiol Rep. (2016) 4:e12785. doi: 10.14814/phy2.12785
35. Standley RA, Distefano G, Pereira SL, Tian M, Kelly OJ, Coen PM, et al. Effects of β-hydroxy-β-methylbutyrate on skeletal muscle mitochondrial content and dynamics, and lipids after 10 days of bed rest in older adults. J Appl Physiol (1985). (2017) 123:1092–100. doi: 10.1152/japplphysiol.00192.2017
36. Romani M, Berger MM, D'Amelio P. From the bench to the bedside: branched amino acid and micronutrient strategies to improve mitochondrial dysfunction leading to sarcopenia. Nutrients. (2022) 14:483. doi: 10.3390/nu14030483
37. Burtscher J, Ticinesi A, Millet GP, Burtscher M, Strasser B. Exercise-microbiota interactions in aging-related sarcopenia. J Cachexia Sarcopenia Muscle. (2022) 13:775−80. doi: 10.1002/jcsm.12942
38. Chen LH, Chang SS, Chang HY, Wu CH, Pan CH, Chang CC, et al. Probiotic supplementation attenuates age-related sarcopenia via the gut-muscle axis in SAMP8 mice. J Cachexia Sarcopenia Muscle. (2022) 13:515–31. doi: 10.1002/jcsm.12849
39. Ticinesi A, Nouvenne A, Cerundolo N, Parise A, Meschi T. Accounting gut microbiota as the mediator of beneficial effects of dietary (poly)phenols on skeletal muscle in aging. Nutrients. (2023) 15:2367. doi: 10.3390/nu15102367
40. Alway SE, McCrory JL, Kearcher K, Vickers A, Frear B, Gilleland DL, et al. Resveratrol enhances exercise-induced cellular and functional adaptations of skeletal muscle in older men and women. J Gerontol A Biol Sci Med Sci. (2017) 72:1595–606. doi: 10.1093/gerona/glx089
41. Harper SA, Bassler JR, Peramsetty S, Yang Y, Roberts LM, Drummer D, et al. Resveratrol and exercise combined to treat functional limitations in late life: a pilot randomized controlled trial. Exp Gerontol. (2021) 143:111111. doi: 10.1016/j.exger.2020.111111
42. Faeh D, Gutzwiller F, Bopp M, Group NCS. Lower mortality from coronary heart disease and stroke at higher altitudes in Switzerland. Circulation. (2009) 120:495–501. doi: 10.1161/CIRCULATIONAHA.108.819250
43. Burtscher J, Millet GP, Burtscher M. Does living at moderate altitudes in Austria affect mortality rates of various causes? An ecological study. BMJ Open. (2021) 11:e048520. doi: 10.1136/bmjopen-2020-048520
44. Mehta R, Steinkraus KA, Sutphin GL, Ramos FJ, Shamieh LS, Huh A, et al. Proteasomal regulation of the hypoxic response modulates aging in C. elegans. Science. (2009) 324:1196–8. doi: 10.1126/science.1173507
45. Copeland JM, Cho J, Lo T Jr, Hur JH, Bahadorani S, Arabyan T, et al. Extension of Drosophila life span by RNAi of the mitochondrial respiratory chain. Curr Biol. (2009) 19:1591–8. doi: 10.1016/j.cub.2009.08.016
46. Rogers RS, Wang H, Durham TJ, Stefely JA, Owiti NA, Markhard AL, et al. Hypoxia extends lifespan and neurological function in a mouse model of aging. PLoS Biol. (2023) 21:e3002117. doi: 10.1371/journal.pbio.3002117
47. Mayfield KP, Hong EJ, Carney KM, D'Alecy LG. Potential adaptations to acute hypoxia: Hct, stress proteins, and set point for temperature regulation. Am J Physiol Regul Integr Comp Physiol. (1994) 266:R1615–22. doi: 10.1152/ajpregu.1994.266.5.R1615
48. Burtscher J, Mallet RT, Burtscher M, Millet GP. Hypoxia and brain aging: neurodegeneration or neuroprotection? Ageing Res Rev. (2021) 68:101343. doi: 10.1016/j.arr.2021.101343
49. Samaja M, Milano G. Editorial - hypoxia and reoxygenation: from basic science to bedside. Front Pediatr. (2015) 3:86. doi: 10.3389/fped.2015.00086
50. Burtscher J, Mallet RT, Pialoux V, Millet GP, Burtscher M. Adaptive responses to hypoxia and/or hyperoxia in humans. Antioxid Redox Signal. (2022). doi: 10.1089/ars.2021.0280
51. Burtscher M, Gatterer H, Szubski C, Pierantozzi E, Faulhaber M. Effects of interval hypoxia on exercise tolerance: special focus on patients with CAD or COPD. Sleep Breath. (2010) 14:209–20. doi: 10.1007/s11325-009-0289-8
52. Bayer U, Likar R, Pinter G, Stettner H, Demschar S, Trummer B, et al. Intermittent hypoxic–hyperoxic training on cognitive performance in geriatric patients. Alzheimers Dement. (2017) 3:114–22. doi: 10.1016/j.trci.2017.01.002
53. Burtscher M, Pachinger O, Ehrenbourg I, Mitterbauer G, Faulhaber M, Pühringer R, et al. Intermittent hypoxia increases exercise tolerance in elderly men with and without coronary artery disease. Int J Cardiol. (2004) 96:247–54. doi: 10.1016/j.ijcard.2003.07.021
54. Zoll J, Ponsot E, Dufour S, Doutreleau S, Ventura-Clapier R, Vogt M, et al. Exercise training in normobaric hypoxia in endurance runners. III. Muscular adjustments of selected gene transcripts. J Appl Physiol (1985). (2006) 100:1258–66. doi: 10.1152/japplphysiol.00359.2005
55. He Z, Qiang L, Liu Y, Gao W, Feng T, Li Y, et al. Effect of Hypoxia Conditioning on Body Composition in Middle-Aged and Older Adults: A Systematic Review and Meta-Analysis, Sports Med Open, ©. Switzerland: Springer Nature Switzerland AG (2023). p. 89.
56. Chobanyan-Jürgens K, Scheibe RJ, Potthast AB, Hein M, Smith A, Freund R, et al. Influences of hypoxia exercise on whole-body insulin sensitivity and oxidative metabolism in older individuals. J Clin Endocrinol Metab. (2019) 104:5238–48. doi: 10.1210/jc.2019-00411
57. Irving BA, Lanza IR, Henderson GC, Rao RR, Spiegelman BM, Nair KS. Combined training enhances skeletal muscle mitochondrial oxidative capacity independent of age. J Clin Endocrinol Metab. (2015) 100:1654–63. doi: 10.1210/jc.2014-3081
58. Kauppila TES, Kauppila JHK, Larsson N-G. Mammalian mitochondria and aging: an update. Cell Metab. (2017) 25:57–71. doi: 10.1016/j.cmet.2016.09.017
59. Franceschi C, Garagnani P, Vitale G, Capri M, Salvioli S. Inflammaging and ‘Garb-aging'. Trends Endocrinol Metab. (2017) 28:199–212. doi: 10.1016/j.tem.2016.09.005
60. Gonzalez-Freire M, Scalzo P, D'Agostino J, Moore ZA, Diaz-Ruiz A, Fabbri E, et al. Skeletal muscle ex vivo mitochondrial respiration parallels decline in vivo oxidative capacity, cardiorespiratory fitness, and muscle strength: The Baltimore Longitudinal Study of Aging. Aging Cell. (2018) 17:e12725. doi: 10.1111/acel.12725
61. Distefano G, Standley RA, Zhang X, Carnero EA, Yi F, Cornnell HH, et al. Physical activity unveils the relationship between mitochondrial energetics, muscle quality, and physical function in older adults. J Cachexia Sarcopenia Muscle. (2018) 9:279–94. doi: 10.1002/jcsm.12272
62. Gonzalez-Freire M, Adelnia F, Moaddel R, Ferrucci L. Searching for a mitochondrial root to the decline in muscle function with ageing. J Cachexia Sarcopenia Muscle. (2018) 9:435–40. doi: 10.1002/jcsm.12313
63. Coelho-Júnior HJ, Calvani R, Picca A, Cacciatore S, Tosato M, Landi F, et al. Combined aerobic training and mediterranean diet is not associated with a lower prevalence of sarcopenia in Italian older adults. Nutrients. (2023) 15:2963. doi: 10.3390/nu15132963
64. Cheng H, Kong J, Underwood C, Petocz P, Hirani V, Dawson B, et al. Systematic review and meta-analysis of the effect of protein and amino acid supplements in older adults with acute or chronic conditions. Br J Nutr. (2018) 119:527–42. doi: 10.1017/S0007114517003816
65. Churchward-Venne TA, Tieland M, Verdijk LB, Leenders M, Dirks ML, de Groot LC, et al. There are no nonresponders to resistance-type exercise training in older men and women. J Am Med Dir Assoc. (2015) 16:400–11. doi: 10.1016/j.jamda.2015.01.071
Keywords: aging, mitochondria, sarcopenia, exercise, diet, hypoxia, physical function
Citation: Burtscher J, Strasser B and Burtscher M (2024) A mito-centric view on muscle aging and function. Front. Public Health 11:1330131. doi: 10.3389/fpubh.2023.1330131
Received: 30 October 2023; Accepted: 19 December 2023;
Published: 10 January 2024.
Edited by:
Hiroyuki Sasai, Tokyo Metropolitan Institute of Gerontology, JapanReviewed by:
Takashi Shida, Tokyo Metropolitan Institute of Gerontology, JapanCopyright © 2024 Burtscher, Strasser and Burtscher. This is an open-access article distributed under the terms of the Creative Commons Attribution License (CC BY). The use, distribution or reproduction in other forums is permitted, provided the original author(s) and the copyright owner(s) are credited and that the original publication in this journal is cited, in accordance with accepted academic practice. No use, distribution or reproduction is permitted which does not comply with these terms.
*Correspondence: Barbara Strasser, YmFyYmFyYS5zdHJhc3NlckBtZWQuc2Z1LmFjLmF0
Disclaimer: All claims expressed in this article are solely those of the authors and do not necessarily represent those of their affiliated organizations, or those of the publisher, the editors and the reviewers. Any product that may be evaluated in this article or claim that may be made by its manufacturer is not guaranteed or endorsed by the publisher.
Research integrity at Frontiers
Learn more about the work of our research integrity team to safeguard the quality of each article we publish.