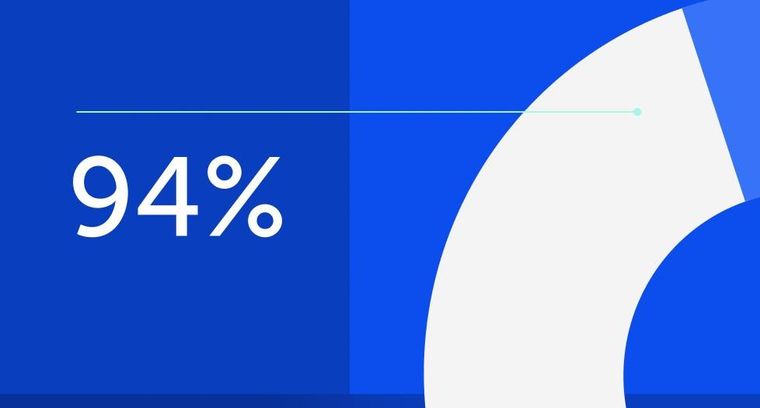
94% of researchers rate our articles as excellent or good
Learn more about the work of our research integrity team to safeguard the quality of each article we publish.
Find out more
REVIEW article
Front. Public Health, 12 October 2023
Sec. Planetary Health
Volume 11 - 2023 | https://doi.org/10.3389/fpubh.2023.1252910
This article is part of the Research TopicVaccine-preventable Diseases in Times of Climate Change, Economic Crisis and Pandemic Preparedness – a Call for New Approaches and Global Equity.View all 6 articles
Climate change represents an unprecedented threat to humanity and will be the ultimate challenge of the 21st century. As a public health consequence, the World Health Organization estimates an additional 250,000 deaths annually by 2030, with resource-poor countries being predominantly affected. Although climate change’s direct and indirect consequences on human health are manifold and far from fully explored, a growing body of evidence demonstrates its potential to exacerbate the frequency and spread of transmissible infectious diseases. Effective, high-impact mitigation measures are critical in combating this global crisis. While vaccines and vaccination are among the most cost-effective public health interventions, they have yet to be established as a major strategy in climate change-related health effect mitigation. In this narrative review, we synthesize the available evidence on the effect of climate change on vaccine-preventable diseases. This review examines the direct effect of climate change on water-related diseases such as cholera and other enteropathogens, helminthic infections and leptospirosis. It also explores the effects of rising temperatures on vector-borne diseases like dengue, chikungunya, and malaria, as well as the impact of temperature and humidity on airborne diseases like influenza and respiratory syncytial virus infection. Recent advances in global vaccine development facilitate the use of vaccines and vaccination as a mitigation strategy in the agenda against climate change consequences. A focused evaluation of vaccine research and development, funding, and distribution related to climate change is required.
Climate change is posing an unprecedented threat to humanity. Attributable to human activities and natural climate variability, climate change refers to the long-term changes in weather patterns, and often specifically to the rise in global temperatures. The last decades of progress in global development may be undermined by this crisis. While global temperatures have already seen a human-induced rise of about 1°C since pre-industrial times, the Intergovernmental Panel on Climate Change (IPCC) projects a rise of global temperatures by at least 1.5°C between 2030 and 2052, with a worst-case scenario of up to 5°C increase by 2100 (1, 2). Meanwhile, an estimated 3.3–3.6 billion people already “live in contexts that are highly vulnerable to climate change” (3). While climate change is estimated to have caused over 150,000 deaths globally in 2000, 83 million cumulative additional deaths have been projected by the year 2100 (4, 5). The World Health Organization (WHO) estimates the direct damage costs to health to be around 2–4 billion USD/year by 2030, while others estimate the health-related cost of air pollution and climate change to already have surpassed 800 billion USD/year for the United States alone (6, 7). These widely diverging figures may be attributed to different modeling approaches as well as varying inclusion variables, as the latter figure specifically relates not only to climate-caused, but also climate-sensitive health outcomes. Consequently, the wide range reflects the uncertainties related to accurately describing the effects of climate change.
The main direct and measurable effects of climate change are rising temperatures, extreme weather events such as heat waves, droughts, floods, and changes in precipitation patterns, as well as elevating sea- and greenhouse gas levels (1). These climate hazards can further lead to a cascade of numerous indirect events that may impact human health negatively. Extreme weather events can lead to the displacement of populations, limit access to healthcare services and the availability of clean water and food, thereby changing the social and environmental determinants of health (7, 8).
Climate change is a major driver of infectious disease dynamics through changes in temperature and precipitation. The effect of climate change on infectious diseases disproportionally affects low- and middle-income countries (LMIC) (9). Diseases with a significant global burden are thought to be aggravated by climate change, while the emergence and re-emergence of other infectious diseases are also anticipated (10, 11). A recent study on 375 infectious diseases worldwide found that 58% of them have been exacerbated by climate change through numerous pathways, most notably vector-borne and water-borne diseases and by bringing pathogens closer to people (12). Additionally, climate change is projected to alter the geographic range of mammals as well as humans and thereby facilitate pathogen sharing as there will be an increasing overlap in species range. This could lead to the emergence of zoonotic diseases of pandemic potential; similar to the spillover of HIV and SARS-CoV to humans enabled through wildlife host jumps; further, bats were identified as a major force facilitating future viral sharing (13). Notably, areas of high human population density are anticipated to emerge as future hotspots for these incidents, highlighting the impact of human interference on the alteration of ecological habitats (13, 14).
Mitigating climate-change-related infectious disease threats through climate change mitigation strategies will necessitate considerable long-term efforts and time to achieve impact. While measures to address climate change and its impact currently focus on political, economic, and social strategies, the potential role of vaccines as a strategy to mitigate the consequences of climate change has been poorly explored. The discovery of immunization and the subsequential development of vaccines for previously life-threatening infectious diseases has already saved millions of lives globally. Vaccines have become an indispensable tool in the last decades for preventing and controlling infectious diseases, as recently seen during the COVID-19 pandemic (15, 16). This paper reviews the leading vaccine-preventable infectious diseases associated with climate change, the vaccine development stages for those diseases, and their challenges.
Almost all pandemic-prone infectious diseases and diseases with high burden are being investigated for vaccines. While vaccines are available to prevent leading childhood infectious diseases, vaccines preventing other infectious diseases with high burden are still to be developed. Table 1 summarizes the climate change-associated vaccine-preventable (or possible) diseases and illustrates the status of vaccine development for those selected conditions.
Table 1. Climate change-associated major infectious diseases and corresponding vaccine development status.
Human health highly depends on access to safe drinking water, sanitation, and hygiene (WASH). WHO estimates 829,000 unsafe WASH-related diarrheal deaths per year, while the disease burden is particularly high in LMICs, where children under 5 years of age are predominantly affected (124). The major WASH-related pathogens are Vibrio cholera, Salmonella Typhi, and other diarrheal pathogens. Their occurrence has been linked to increasing temperatures and rainfall, thus affected directly by climate change (34, 125–129). The potential effect of extreme weather events exacerbated by climate change on waterborne diseases is becoming an increasing concern: The El Niño-Southern Oscillation (ENSO) is a complex natural and periodic climate pattern that occurs in the Pacific Ocean with extreme phases such as the El Niño and it has shown to be intensified through climate change (130, 131). ENSO causes droughts, floods, and hurricanes worldwide with an enhancement of endemic food- and water-borne diseases, as well as vector-borne diseases, through changes in pathogen survival and proliferation, vector survival, transmission, and ecology (132). Extreme weather events preceding outbreaks of food- and water-borne diseases are reported worldwide, such as typhoons in China, floods in Bangladesh and tsunamis in Thailand and Indonesia (133).
Cholera is at the forefront of vaccine-preventable WASH-related diseases, associated directly with climate change. Survival of the Vibrio cholera bacterium that causes cholera depends on environmental conditions such as temperature, pH, salinity, and plankton. Increasing sea surface temperature, a well-known impact of climate change, has been found to favor cholera outbreaks (19, 20). In addition, cholera cases have been shown to correlate with rainfall and temperatures while also exhibiting seasonal patterns (21–23). Extreme weather condition-related surges of cholera were documented following El Niño in the African continent, floods in Bangladesh and cyclones in Malawi (22, 24, 25, 134). Most recently, severe droughts in Somalia resulted in large displacement that exacerbated endemic cholera outbreaks. Humanitarian crises like these lead to disrupted health systems, worsened living conditions, limited access to clean water, and malnutrition; all of which may increase susceptibility to pathogens (135).
Predictive models suggest an increase in environmental suitability for Vibrio cholera in ocean waters as well as an increase in cholera cases under different Representation Concentration Pathway (RCP) scenarios (136, 137). The RCPs are commonly used for modeling studies and represent four future climate scenarios measured by emissions and classified as RCP 2.6, 4.5, 6.0, and 8.5 with increasing levels of severity, respectively (138). While less extensively than for cholera, similar positive relationships to temperature, rainfall, flooding, and seasonal effects have been reported for other common diarrheal pathogens like E. coli, rotavirus, S. typhi and other Salmonella (28, 29, 31–34, 37, 139). Pathogen-specific responses to climate variables are likely, as temperature sensitivity has been shown to vary between enteric pathogens (140). For instance, temperature may affect the expression of virulent genes, survival and growth of bacterial pathogens, which exhibit distinct optimal temperatures ranges. Meanwhile, fluctuations in rainfall can shape the ecological niche of enteric pathogens, and lead to contamination of water sources, enhancing their exposure to human populations (129, 140). Unfortunately, most studies do not further differentiate diarrheal diseases according to their causative pathogen (129).
Schistosomiasis and Hookworm are human helminth infections posing a major health threat to more than a billion people worldwide and are expected to be impacted by climate change. The effects of climate change on water reservoirs directly impact the immediate host of Schistosoma species, poikilothermic freshwater snails (46). Predictions for climate change scenarios suggest a shift in schistosomiasis incidence in African regions where schistosomiasis is currently highly prevalent (47). The burden of soil-transmitted helminthiasis such as hookworm infection, which is known to cause substantial global morbidity, may increase further as climate change impacts soil through increased surface temperatures and altered precipitation (44, 141, 142).
Other water-related zoonotic diseases are also expected to be impacted by climate change. Leptospirosis, the most globally widespread zoonotic disease, is associated with climatic factors. Outbreaks have been reported globally following extreme weather events like flooding (121, 143, 144). Increased rainfall affects environmental conditions for rodent populations, and may further alter transmission dynamics (122, 144).
The WHO estimates vector-borne diseases to account for 17% of infectious diseases, resulting in more than 700,000 deaths annually. The burden of diseases is highest in low-income settings, with the majority of cases occurring in tropical and subtropical regions (145). Further, the mortality rate from vector-borne diseases is estimated to be almost 300 times greater in LMICs than in high-income countries (146). Temperature significantly influences the survival and transmission of vectors and the pathogens they carry (147). The IPCC states, “risks from some vector-borne diseases, such as malaria and dengue fever, are projected to increase with warming from 1.5°C to 2°C, including potential shifts in their geographic range” (2).
Malaria remains one of the most prevalent and fatal infectious diseases worldwide. Nearly half the world’s population is at risk (148). Transmission of the Plasmodium parasites occurs through the Anopheles mosquito. Life cycle and disease transmission by Anopheles gambiae is influenced strongly by temperature, with an optimal temperature for transmission estimated around 25°C and temperatures between 17°C and 34°C required for survival (149–151). In the past century, Anopheles have shown a gradual movement to higher elevations and greater distances from the equator (50). Global warming is expected to contribute to a further northward expansion of transmission suitability and lengthened transmission seasons. Most significant effects are predicted for parts of Africa, South America, and South-East Asia (51, 52). Within the past 50 years, developing countries had a 39% increase in the number of months suitable for transmission, while effects varied between highland and lowland areas (53, 54). Precipitation patterns have also been shown to influence mosquito survival, as wet areas serve as breeding sites for mosquitos. However, the interaction with precipitation is not yet as clearly understood as it is for temperature (152). Regional decreases in malaria incidence and previously endemic areas becoming unsuitable are also projected in regions where temperatures may exceed optimal transmission temperatures due to the effects of global warming, indicating how climate change may also contribute to fighting malaria in Western Africa and South-East Asia (66). In addition, the expansion of Anopheles stephensi to Africa suggests urban transmission will further increase, as it is a competent urban vector with a wider temperature range (153, 154). Prediction models mainly focus on climate variables related to temperature and precipitation and often do not consider indirect factors, such as economic development and improved urban sanitation, the recently approved malaria vaccine, or the increasing resistance to malaria drugs, which may greatly mitigate or exacerbate health outcomes.
Dengue, transmitted mainly by mosquitos Aedes aegypti and Aedes albopictus, is a globally widespread vector-borne disease with rapidly increasing incidence (155). These two mosquitos are experiencing a steady increase in environmental suitability, as indicated by rising transmission rates, with the largest increase seen in countries with a high HDI (53). The risk of dengue fever has shown to be dependent on temperature and precipitation (156, 157). Transmission by Ae. aegypti peaks at 29°C, slightly higher than the peak temperature for malaria transmission. Global warming is expected to cause a shift in relative suitability and distribution of these diseases, with the sub-Saharan African region experiencing a shift from malaria to dengue (57). In Africa, where 15.2% of the total continent is already considered a highly suitable area for Ae. aegypti, an increase to 21.8% under RCP 4.5 and 23.3% under RCP 8.5 of suitable area is predicted by 2050 (58). An increased suitability for Ae. albopictus in Europe was predicted with 83% of urban areas and 68% of the entire European continent being suitable by 2050, up from 49% currently (59).
Zika and chikungunya viruses are also transmitted by the Aedes mosquito and have the highest burden in the Americas region (158). Their basic reproduction number has increased by around 12% and the length of their transmission season by around 6% compared to the 1950s (62). For the most recent Zika outbreak in 2015 with an estimated 130 million infected people, a link between transmission and El Niño has been suggested (159, 160). A Zika-specific temperature-dependent transmission model for A. aegypti predicted a net increase of 2.71 billion people at risk under RCP 8.5, with a projected year-round transmission risk for 915.9 million. Even under RCP 4.5, an additional 2.5 billion people are projected to be at risk as more parts of the world become suitable due to a warming climate. Under this model, the largest increase in transmission suitability was predicted in North America (63).
Chikungunya virus was first identified in Africa, where sporadic cases and outbreaks are reported frequently (161). In recent decades, there have been a few reports of local transmission in Europe (162). As it is transmitted by the same vectors as Zika and dengue, Ae. aegypti and Ae. albopictus, climate change scenarios project similar expansions due to increased climatic suitability (66, 67). Modeling under RCP 4.5 and RCP 8.5 showed expansion toward regions such as central Europe, China, and Central America; while some areas were also found to have declining suitability (68).
Yellow Fever is transmitted by the Aedes or Haemogogus mosquitos and is endemic in tropical regions of South America and Africa (163). The most recent modeling approach for yellow fever transmitted by Ae. aegypti projected an increased transmission intensity and an increase in the number of deaths in the African region, with heterogenous changes across the region, in line with predictions for other vector-borne diseases transmitted by Ae. aegypti. An expected increase of the number of deaths per year between 10.0 and 40.0% by 2070, depending on the severity of the anticipated climate change scenario, was reported (70). Meanwhile, another modeling study predicted a decrease in cases and future outbreak durations under RCP 4.5 and RCP 8.5 (71).
Rift Valley Fever (RVF) is another mosquito-borne disease of rising concern, a zoonotic, vector-borne disease that causes disease primarily in animals and humans with severe forms such as hemorrhagic fever. Transmission occurs through mosquitos, mainly Aedes, and the epidemiology of the virus and its vectors has shown to be influenced by climate; consequently, climate change is predicted to expand vector habitat and contribute to the emergence of risk areas (74, 75). While an increasing risk was associated with ENSO events; rainfall, population density and irrigation have further been identified as environmental drivers, underscoring the need for heightened surveillance efforts (76).
Vector-borne helminth infections are acknowledged as neglected tropical diseases (NTD).
Lymphatic filariasis is transmitted by several mosquito species, such as Anopheles and Culex, whose expanded range and breeding seasons are thought to lead to an estimated 1.86 billion people at risk of infection by 2050 (44, 79). For Culex, which also transmits the West Nile Virus, environmental suitability was identified for Europe and North Africa, as well as shifting distribution patterns under different RCP scenarios (80).
Leishmaniasis is transmitted by Phlebotomine sandflies, which carry the Leishmania parasite. This disease is endemic in many tropical regions; it is climate-sensitive as sandflies are dependent on high temperatures and therefore expected to expand their range under climate change. According to predictions, increased climate suitability could lead to further northward expansion of vector range in Europe; as well as a twofold increase in individuals at risk of Leishmaniasis in North America for 2080 (83, 84).
Ticks are efficient vectors to transmit a wide range of disease-causing pathogens. Important examples are Lyme disease, tick-borne-encephalitis (TBE) and Crimean-Congo Hemorrhagic Fever (CCHF).
Lyme disease is the most common tick-borne disease in the United States and Europe (164). The vector of Lyme disease, Ixodes ticks, have shown to be sensitive to environmental conditions, foremost temperature, rainfall, and humidity (165). Further, an index based on temperature, precipitation, vegetation, and soil moisture was able to correspond well to observed tick-borne infections, emphasizing the possibility of utilizing these climate variables as surveillance tools (166). Climate change effects are projected to cause an expansion of Ixodes distribution and increase the transmission risk, with high regional variability (86–88). Northward expansion of Ixodes has already been reported in Northern America and Scandinavia within the last decades, and was attributed to a warming climate in these regions (89, 90). An expansion to higher altitudes has also been projected, along with an increased abundance in regions where ticks are already present (91).
Tick-borne-encephalitis is less common than Lyme disease. A shift in distribution to higher altitudes, altered seasonality, and increased incidences were observed in Eastern Europe, as well as increased incidences that were attributed to increasing temperatures in Russia and Sweden (93–97). Furthermore, transmission is predicted to increase further, corresponding to expected temperature increases in Europe (98).
Crimean-Congo Hemorrhagic Fever (CCHF) is a disease of major global concern, transmitted by a highly pathogenic virus. Once limited to the 50° North latitude as the geographic range of its vector, CCHF has increased in endemic areas, such as Turkey, and further expanded to northern and western Europe, attributed to a warmer climate (100–102). The principal vector is Hyalomma ticks, most commonly H. marginatum. Expansion of suitable areas related to changing climatic variables has been observed for H. marginatum as well as predictions for further northward expansion with simultaneously reduced suitability in North Africa and Southern Iberia under RCP 4.5 (103, 167).
The effects of climate change on air-borne infectious diseases have been less well studied so far. There is limited evidence suggesting that climate change may influence the dynamics of air-borne infectious diseases. The dynamics of air-borne diseases and their relationship to climate change is complex and warrants further investigation.
Seasonal influenza, an acute respiratory infection that occurs in epidemics, is known to be influenced by region-specific climatic factors, foremost temperature and humidity (106, 107). Predictions show a trend where higher temperatures are associated with a lower risk of transmission, while associations with humidity suggest a similar pattern but are less clear (108–110). Consequently, a warming climate with milder winters itself could decrease the risk and intensity of influenza epidemics. However, the climate change-induced weather variability projected under RCP scenarios, with sudden large changes in temperature, may conversely increase the risk of influenza epidemics and result in shifting patterns (111, 112). Extreme weather events, resulting temperature fluctuations and the increasing risk of emerging zoonotic viruses may further contribute to future outbreaks (168). Ultimately, the “net effect” of climate change on influenza, also considering other factors such as vaccination and population density, is difficult to determine. As most current studies focus on temperature and humidity, the influence of other climate-dependent variables that may influence virus survival, replication and mutation should be considered in future studies.
Another important pathogen causing lower respiratory infections is the Respiratory Syncytial Virus (RSV). RSV epidemics are similar to influenza, with temperate regions usually experiencing a peak in winter months. It was found that precipitation and humidity both drive RSV transmission depending on location-specific climate conditions, with increased humidity under RCP 8.5 expected to lead to reduced transmission and precipitation-driven increased transmission under an overall northward shift (115). The role of temperature is less clear and often contradictory. In a study in Canada, warmer temperatures were associated with lower odds of RSV hospitalization (116). In LMICs, the association between temperature and increased or decreased RSV transmission varied by country, so other factors such as humidity and rainfall are likely interwoven (117).
The next decades will be shaped by the disruptive and destructive consequences of a warming climate, including the exacerbation of climate-sensitive infectious diseases, which poses a major global threat to human health. Figure 1 presents a summary of the impacts and consequences of climate change and subsequent mitigation factors. Taking action to prevent and mitigate potential consequences is critical.
Effective vaccines are highly cost-effective interventions that have substantially reduced the burden of some infectious diseases and eliminated others completely. For some infectious diseases identified in the scope of this review, there are already highly effective vaccines available. These could significantly reduce vulnerability in communities and help protect them from endemic as well as emerging infectious diseases that are driven by climate change. This could lead to strengthened health sector resilience and would help communities to better cope with other impacts of climate change. Further, widespread immunization presents a viable near-term strategy to improve overall health outcomes as opposed to realizing long-term, structural changes for public health in LMICs or combatting the climate crisis itself. In addition, the persisting threat of rising antimicrobial resistance, another major global health threat, could simultaneously be addressed, as vaccines can reduce antibiotic usage and are therefore considered a valuable tool to combat AMR (169).
Cholera illustrates well how an effective vaccine could mitigate the effects of climate change: There are three WHO pre-qualified oral cholera vaccines (OCV): Euvichol-Plus®, Shanchol™, and Dukoral®, which are recommended for use in areas of endemic cholera as well as during outbreaks and humanitarian crises with a high risk of infection (18). These OCVs significantly reduce cholera disease burden, can promote herd immunity, and are cost-effective (170–172). Currently, OCV administration occurs mostly reactively in emergency settings; for this purpose, the global GAVI-funded OCV stockpile has supplied more than 70 million doses for countries in need (173). The WHO announced a critical shortage of cholera vaccines in 2022 due to increased global demand following large outbreaks, which have also been attributed to climate change (174, 175). As the risk of outbreaks further increases, mitigating and preventing them through immunization in communities with poor living conditions, where cholera thrives, could have a substantial impact. Geographic and age-based targeting for vaccination may optimize the use of limited OCV supply by maximizing cost-effectiveness (176, 177). Expanded disease monitoring could help guide national and local vaccine allocation. Resource-limited areas often lack reliable data and diagnostic tools necessary to determine where vaccine distribution is most urgent and will have the greatest benefit (172).
Overcoming current supply constraints in the future could induce a crucial shift from reactive to preventive vaccination with the goal of achieving herd immunity, locally eradicating cholera, and averting epidemic outbreaks altogether. Endemic cholera could potentially be controlled with an estimated 50–70% OCV coverage, emphasizing the substantial benefits of mass vaccination (171). Malawi was one of the first countries to include OCV in their national cholera control plan and administered more than one million doses in the first year in a non-emergency setting, which could serve as a model for other countries (178).
Another example of a highly effective vaccine requiring higher coverage is the YF-17D yellow fever vaccine, a one-dose vaccine conferring lifelong protection that has been available since the 1930s (179). National vaccination campaigns have shown to reduce cases and the impact of vaccination significantly influences modeling outcomes for outbreaks (71, 180). However, the actual coverage is estimated at 44% in the African region and has substantially declined within the last decades. Outbreaks continue to occur, and up to 473 million people in risk areas would require vaccination to achieve sufficient population-level coverage (181, 182). There are several second-generation vaccines in development; if shown non-inferior to the YF-17D vaccine, they could provide support to the WHO’s ambitious goal of supplying 1.3 billion doses of vaccines to endemic countries by 2026 and help the global elimination of yellow fever (72, 183).
Developing effective vaccines for vector-borne diseases has proven to be a challenging endeavor that is becoming even more pressing with the climate-induced expansion of vectors. In 2021, after several decades of research and clinical trials, the long-awaited RTS,S/AS01 (Mosquirix™) became the first malaria vaccine and was recommended by the WHO for immunization of children in regions with risk of malaria transmission. More than 2 million doses have been administered in African countries so far (184). However, while it could prevent up to 30% of mortality in young children in endemic regions, efficacy varies between subgroups, is generally modest and protection significantly declines over time. In addition, the four-dose vaccination regime complicates successful implementation in endemic malaria settings (184–188). Weaknesses of the RTS,S vaccine should be considered for further vaccine developments. The R21/Matrix-M vaccine is regarded as a highly promising candidate. It is a modified form of RTS,S, cheaper and more potent, showing efficacy of 77% after the initial doses and up to 80% when administered with a high-dose adjuvant. Phase 3 trials are currently underway with the ambitious goal of obtaining licensure in 2023 (55, 189).
The expansion of dengue is similarly concerning. The only available vaccine CYD-TDV (Dengvaxia®), is licensed in several endemic countries, but its uptake and introduction to routine vaccination has been limited by its variable performance in different age groups, as well as the serotype-dependent response, with seronegative individuals even at higher risk for severe dengue (190). TAK-003 (QDENGA®), a live-attenuated vaccine candidate that is currently being studied in Phase 3 trials, showed high efficacy (80.2%) and reduced hospitalization in infected participants by 95.4% (191). It received a recommendation from the European Medicines Agency in October 2022 and was already approved in Indonesia, which could pave the way for global licensure (192). Effective dengue and malaria vaccines would become an invaluable asset for global public health; considering the projected developments under climate change, even partial protection from vaccines could be substantive and prevent further burden on communities that are already majorly affected.
Infectious diseases expected to be aggravated by climate change and for which no effective preventive vaccine is available yet should be prioritized for research and development (R&D). Limited investment in R&D for poverty-related diseases, which most climate-sensitive diseases currently are, is a fundamental problem expressed by the 10/90 gap. Less than 10% of health R&D expenditure is spent on diseases accounting for more than 90% of the world’s disease burden, the majority of which are infectious diseases. Since the 10/90 gap was first described, global health spending has seen a multifold increase; however, the principle of this gap persists (193). The spending disparities are also reflected in the low number of licensed drugs and vaccines available for these diseases, the result of pharmaceutical and public sector research concentrating on diseases of high-income countries (194). Most of the infectious diseases driven by climate change occur predominantly in developing countries and are therefore a prime example of this gap.
NTDs such as schistosomiasis and soil-transmitted helminths affect hundreds of millions of people and carry a high morbidity, but there are only a few drugs for treatment and no licensed vaccine available yet (195). A few vaccine candidates are currently under development, such as the hookworm recombinant vaccines Na-GST-1/Na-APR-1, in Phase 1 clinical studies (45). There are four prominent candidates with different targets for a Schistosomiasis vaccine that are currently in various clinical phases, including a Phase 1 clinical study for Sm-p80 GLA-SE (SchistoShield®), a leading vaccine candidate (48, 49). Nonetheless, it will take several more years until a licensed NTD vaccine is ready for roll-out and widespread administration, but once available, it has the potential to significantly lessen the burden and improve public health outcomes.
The IPCC has acknowledged the potential of vaccines to mitigate the effect of climate change on vector-borne disease, along with surveillance and warning systems (3). However, multiple challenges remain to leverage vaccinations as a global strategy to mitigate climate change effects, mainly related to vaccine development, supply, access and delivery.
The first step in climate-responsive strategies should be an improved understanding and anticipation of the dynamic, cascading effects of climate change. Refined spatial and temporal mapping of climate-sensitive diseases would be useful to determine disease burden, which is expected to shift significantly for various diseases; as well as anticipate climate change-fueled extreme weather events that could exacerbate outbreaks of infectious diseases. A proposed “Vaccine Risk Index,” which included variables related to climate, urban population, human development, and peace, could be a valuable tool to identify nations at risk for the emergence and re-emergence of vaccine-preventable diseases and help guide vaccination programs (196).
The climate-related burden assessment would provide further guidance for vaccine development. This review illustrates major gaps in vaccine development for several climate-sensitive diseases, in particular NTDs. Prioritizing research and development for diseases that do not yet have a vaccine and improving less-effective vaccines, while delivering existing vaccines to areas with low coverage should simultaneously be achieved. Research efforts should focus on continuous monitoring of genomic patterns of pathogens, surveillance of resistance to current treatments (e.g., antimicrobial resistance) and current vaccine-induced immune responses in the areas most affected by climate changes. The rapid evolution of SARS-CoV-2 and its variants has led to the development of multivalent (pan sarbecoviruses and zoonotic viruses) vaccines. Such a strategy may be adopted for other rapidly evolving pathogens and the benefit of existing vaccine platforms (e.g., mRNA) for rapid adaptation of vaccine design and development.
The shifting global distribution of diseases warrants a critical assessment of national routine immunization schedules, as some may require future adaptation to include protection against pathogens driven by climate change. In addition, combination vaccines could facilitate vaccine delivery in LMICs by increasing timeliness and efficiency (197). Climate-responsive vaccination strategies should not aspire to take a “one-size-fits-all” approach, rather, they will require dynamic reassessments to determine optimal regional, risk-based recommendations for immunization. Specifically, while vaccines remain a limited resource in several regions of the world, the most vulnerable and at-high-risk populations should be prioritized for vaccinations before large-scale campaigns and routine immunization strategies are implemented.
The threat of emerging infectious diseases and new pandemics may intensify under climate change (13). Vaccines remain the most effective means of controlling and mitigating outbreaks. The unprecedented, accelerated development of the COVID-19 vaccines with their novel platform technologies and rapid licensing process could serve as a blueprint for future pandemics, while shortcomings of the global roll-out process should be addressed (198). Notably, the United States National Institute of Allergy and Infectious Diseases has recently proposed a blueprint strategy detailing the prototype pathogen approach: developing vaccines directed against known viral families of concern to humans that may be easily and rapidly adapted to a newly identified pathogen and utilized to prevent pandemic outbreaks (199). Rigorous surveillance and disease modeling systems that incorporate anticipated climate change scenarios may help in assessing the expansion of pathogens and vectors, identifying high-risk areas, and predicting the next pandemic.
The recent focus on regional vaccine manufacturing, particularly in Africa, will over the long-term improve availability and accessibility of vaccines in vulnerable communities and LMICs. This will complement the efforts of key global health stakeholders, such as UNICEF and Gavi, The Vaccine Alliance, which currently supply more than 50 low-income countries with low-cost or no-cost vaccines (200–202). Notably, the impact of climate-change-related infectious diseases on populations in LMICs could also encourage the advancement of sustainable, regional vaccine manufacturing. Regardless, vaccines alone are insufficient if vaccination coverage is limited by health sector under-resourcing; most recently, the COVID-19 vaccine roll-out highlighted the importance of strengthening healthcare delivery in LMICs (197, 203). Improving health systems and expanding local and national healthcare infrastructure is crucial to achieve effective vaccine delivery and increase vaccination coverage rates. The global trend of declining vaccination rates, combined with the potential of climate change to undermine the future delivery of vaccines is alarming (204, 205). Vaccines are temperature-sensitive products and must be kept within a strict temperature range throughout their handling until the point of administration. Aware of the logistical challenges of vaccine delivery, considerable efforts are now underway to improve vaccine thermostability and long-term shelf-life at ambient temperature (206, 207). Consequently, implementing robust, climate-resilient vaccine delivery schemes must be a priority. Meanwhile, little is still known about the impact of climate change on host factors that may affect the risk for infectious diseases. For example, a recent study concluded that climate change is likely to affect the susceptibility of individuals to tuberculosis by increasing the prevalence of its underlying risk factors, particularly in LMICs (208). The potential impact of climate change on disease-specific risk host factors warrants further in-depth assessment.
Despite the proven safety and efficacy of established vaccines, there is a concerning trend of vaccine hesitancy. This has become increasingly evident during the global roll-out of COVID-19 vaccines and may counter-balance the climate change vaccination mitigation efforts. The WHO has identified vaccine hesitancy as one of the top ten threats to global health (209). Interestingly, in the case of the COVID-19 vaccines, acceptance was shown to be considerably higher for LMICs than high-income countries; this could imply that vaccination strategies should not be exclusive to LMICs but must also focus on high-income countries to overcome regional and national vaccination coverage gaps (210). Further, vaccine hesitancy has enabled resurgences of vaccine-preventable diseases such as measles, where even a small decrease in vaccine coverage could lead to significant increases in disease incidence (211).
Ultimately, the investment in vaccines will yield multiple beneficial downstream effects: these include the reduction of global disease burden, inequalities, poverty, antimicrobial resistance and potential future pandemics, as well as the improvement in quality of life, economic growth and planetary health; overall contributing to achieving the United Nation’s Sustainable Development Goals and ensuring health in a warming world (212).
The climate change crisis is undeniable and will be the ultimate challenge of the 21st century. Though still limited, evidence for the impact of climate change on infectious disease is accumulating. The impact of climate change on infectious diseases is confounded by interrelated covariates, including, but not limited to, human mitigation measures, geographic distribution, and variations within countries. Important non-climate determinants of disease transmission, such as globalization, public health systems, and socioeconomic conditions, may also be affected indirectly by climate change and should be addressed simultaneously.
Further research and monitoring in the form of large-scale, longitudinal studies are needed to better understand the health impacts of climate and environmental changes as they are constantly evolving. Meanwhile, collaborative surveillance activities are also essential measures to improve predictive models and outbreak preparedness, while guiding global and local policymaking for the introduction and prioritization of vaccination schemes.
Currently available vaccines should be distributed to achieve wider coverage, especially in LMICs. Whether single vaccines for specific diseases or combined “climate change” vaccines should be developed for better use are questions that deserve to be addressed urgently by stakeholders and developers. Considering the potentially severe effects of climate change on infectious diseases, health sector resilience should be strengthened. In this context, global vaccination strategies may serve as an important mitigation tool and must become a priority for research and development, supply, and delivery.
J-LE and JK designed the synopsis. CK and SA wrote the manuscript. FM, JK, and J-LE reviewed and edited the manuscript. All authors contributed to the article and approved the submitted version.
This work was funded entirely by the International Vaccine Institute.
The authors thank Ji Yeon Lee, librarian at IVI for helpful literature search and references.
The authors declare that the research was conducted in the absence of any commercial or financial relationships that could be construed as a potential conflict of interest.
All claims expressed in this article are solely those of the authors and do not necessarily represent those of their affiliated organizations, or those of the publisher, the editors and the reviewers. Any product that may be evaluated in this article, or claim that may be made by its manufacturer, is not guaranteed or endorsed by the publisher.
1. IPCC. Summary for policymakers In: V Masson-Delmotte, P Zhai, A Pirani, SL Connors, C Péan, and S Berger, et al., editors. Climate Change 2021: The physical science basis. Contribution of working group I to the sixth assessment report of the Intergovernmental Panel on Climate Change. (Cambridge, United Kingdom and New York, NY: Cambridge University Press) (2021). 3–32. doi: 10.1017/9781009157896.001
2. IPCC. Summary for policymakers. In: V Masson-Delmotte, P Zhai, H-O Pörtner, D Roberts, J Skea, and PR Shukla, et al., editors. Global warming of 1.5°C. an IPCC special report on the impacts of global warming of 1.5°C above pre-industrial levels and related global greenhouse gas emission pathways, in the context of strengthening the global response to the threat of climate change, sustainable development, and efforts to eradicate poverty. (Cambridge, United Kingdom and New York, NY, USA: Cambridge University Press) (2018). 3–24. doi: 10.1017/9781009157940.001
3. IPCC. Summary for policymakers. In: H-O Pörtner, DC Roberts, ES Poloczanska, K Mintenbeck, M Tignor, and A Alegría, et al., editors. Climate change 2022: Impacts, adaptation, and vulnerability. Contribution of working group II to the sixth assessment report of the Intergovernmental Panel on Climate Change. (Cambridge, United Kingdom and New York, NY, USA: Cambridge University Press) (2022). 3–33. doi: 10.1017/9781009325844.001
4. Sheffield, PE, and Landrigan, PJ. Global climate change and Children’s health: threats and strategies for prevention. Environ Health Perspect. (2011) 119:291–8. doi: 10.1289/EHP.1002233
5. Bressler, RD. The mortality cost of carbon. Nat Commun. (2021) 12:4467. doi: 10.1038/s41467-021-24487-w
6. De Alwis, D, and Limaye, VS. (2021). The costs of inaction: The economic burden of fossil fuels and climate change on health in the United States. Available at: https://www.nrdc.org/sites/default/files/costs-inaction-burden-health-report.pdf (Accessed February 7, 2023).
7. World Health Organization. (2021). Climate change and health. Fact sheet. Available at: https://www.who.int/news-room/fact-sheets/detail/climate-change-and-health (Accessed August 6, 2022).
8. Semenza, JC, Rocklöv, J, and Ebi, KL. Climate change and cascading risks from infectious disease. Infect Dis Ther. (2022) 11:1371–90. doi: 10.1007/s40121-022-00647-3
9. Shuman, EK. Global climate change and infectious diseases. N Engl J Med. (2010) 362:1061–5. doi: 10.1056/NEJMp0912931
10. Baker, RE, Mahmud, AS, Miller, IF, Rajeev, M, Rasambainarivo, F, Rice, BL, et al. Infectious disease in an era of global change. Nat Rev Microbiol. (2022) 20:193–205. doi: 10.1038/s41579-021-00639-z
11. Patz, JA, Epstein, PR, Burke, TA, and Balbus, JM. Global climate change and emerging infectious diseases. JAMA. (1996) 275:217–23. doi: 10.1001/JAMA.1996.03530270057032
12. Mora, C, McKenzie, T, Gaw, IM, Dean, JM, von Hammerstein, H, Knudson, TA, et al. Over half of known human pathogenic diseases can be aggravated by climate change. Nat Clim Chang. (2022) 12:869–75. doi: 10.1038/s41558-022-01426-1
13. Carlson, CJ, Albery, GF, Merow, C, Trisos, CH, Zipfel, CM, Eskew, EA, et al. Climate change increases cross-species viral transmission risk. Nat. (2022) 607:555–62. doi: 10.1038/s41586-022-04788-w
14. Johnson, CK, Hitchens, PL, Pandit, PS, Rushmore, J, Evans, TS, Young, CCW, et al. Global shifts in mammalian population trends reveal key predictors of virus spillover risk. Proc R Soc B. (2020) 287:20192736. doi: 10.1098/rspb.2019.2736
15. Toor, J, Echeverria-Londono, S, Li, X, Abbas, K, Carter, ED, Clapham, HE, et al. Lives saved with vaccination for 10 pathogens across 112 countries in a pre-COVID-19 world. elife. (2021) 10:e67635. doi: 10.7554/ELIFE.67635
16. Watson, OJ, Barnsley, G, Toor, J, Hogan, AB, Winskill, P, and Ghani, AC. Global impact of the first year of COVID-19 vaccination: a mathematical modelling study. Lancet Infect Dis. (2022) 22:1293–302. doi: 10.1016/S1473-3099(22)00320-6
17. World Health Organization. Prequalified vaccines | WHO - Prequalification of Medical Products (IVDs, Medicines, Vaccines and Immunization Devices, Vector Control). (2023). Available at: https://extranet.who.int/pqweb/vaccines/prequalifiedvaccines (Accessed March 10, 2023).
18. World Health Organization. (2022). Cholera. Available at: https://www.who.int/news-room/fact-sheets/detail/cholera?gclid=EAIaIQobChMIxYLB7daW-wIVmtB3Ch3DxQl3EAAYASAAEgIKLvD_BwE (Accessed November 5, 2022).
19. Christaki, E, Dimitriou, P, Pantavou, K, and Nikolopoulos, GK. The impact of climate change on cholera: a review on the global status and future challenges. Atmosphere (Basel). (2020) 11:449. doi: 10.3390/atmos11050449
20. Caminade, C, McIntyre, KM, and Jones, AE. Impact of recent and future climate change on vector-borne diseases. Ann N Y Acad Sci. (2019) 1436:157–73. doi: 10.1111/NYAS.13950
21. Hashizume, M, Armstrong, B, Hajat, S, Wagatsuma, Y, Faruque, ASG, Hayashi, T, et al. The effect of rainfall on the incidence of cholera in Bangladesh. Epidemiology. (2008) 19:103–10. doi: 10.1097/EDE.0B013E31815C09EA
22. Moore, SM, Azman, AS, Zaitchik, BF, Mintz, ED, Brunkard, J, Legros, D, et al. El Niño and the shifting geography of cholera in Africa. Proc Natl Acad Sci U S A. (2017) 114:4436–41. doi: 10.1073/pnas.1617218114
23. Reyburn, R, Kim, DR, Emch, M, Khatib, A, Von Seidlein, L, and Ali, M. Climate variability and the outbreaks of cholera in Zanzibar, East Africa: a time series analysis. Am J Trop Med Hyg. (2011) 84:862–9. doi: 10.4269/ajtmh.2011.10-0277
24. Schwartz, BS, Harris, JB, Khan, AI, LaRocque, RC, Sack, DA, Malek, MA, et al. Diarrheal epidemics in Dhaka, Bangladesh, during three consecutive floods: 1988, 1998, and 2004. Am J Trop Med Hyg. (2006) 74:1067–73. doi: 10.4269/ajtmh.2006.74.1067
25. Bagcchi, S. Malawi takes on cholera outbreak amid cyclone devastation. Lancet Microbe. (2022) 3:e480. doi: 10.1016/S2666-5247(22)00131-8
26. Holmgren, J. Modern history of cholera vaccines and the pivotal role of icddr,b. J Infect Dis. (2021) 224:S742–8. doi: 10.1093/infdis/jiab423
27. GBD Results Tool. (2022). GHDx. Available at: http://ghdx.healthdata.org/gbd-results-tool (Accessed November 7, 2022).
28. Dewan, AM, Corner, R, Hashizume, M, and Ongee, ET. Typhoid fever and its association with environmental factors in the Dhaka metropolitan area of Bangladesh: a spatial and time-series approach. PLoS Negl Trop Dis. (2013) 7:e1998. doi: 10.1371/journal.pntd.0001998
29. Liu, Z, Lao, J, Zhang, Y, Liu, Y, Zhang, J, Wang, H, et al. Association between floods and typhoid fever in Yongzhou, China: effects and vulnerable groups. Environ Res. (2018) 167:718–24. doi: 10.1016/j.envres.2018.08.030
30. Syed, KA, Saluja, T, Cho, H, Hsiao, A, Shaikh, H, Wartel, TA, et al. Review on the Recent Advances on Typhoid Vaccine Development and Challenges Ahead. Clin Infect Dis. (2020) 71:S141–S150. doi: 10.1093/cid/ciaa504
31. Thindwa, D, Chipeta, MG, Henrion, MYR, and Gordon, MA. Distinct climate influences on the risk of typhoid compared to invasive non-typhoid Salmonella disease in Blantyre, Malawi. Sci Reports. (2019) 9:20310. doi: 10.1038/s41598-019-56688-1
32. Baliban, SM, Lu, YJ, and Malley, R. Overview of the Nontyphoidal and Paratyphoidal Salmonella Vaccine Pipeline: Current Status and Future Prospects. Clin Infect Dis. (2020) 71:S151–S154. doi: 10.1093/cid/ciaa514
33. Khalil, IA, Troeger, C, Blacker, BF, Rao, PC, Brown, A, Atherly, DE, et al. Morbidity and mortality due to shigella and enterotoxigenic Escherichia coli diarrhoea: the global burden of disease study 1990–2016. Lancet Infect Dis. (2018) 18:1229. doi: 10.1016/S1473-3099(18)30475-4
34. Philipsborn, R, Ahmed, SM, Brosi, BJ, and Levy, K. Climatic drivers of Diarrheagenic Escherichia coli incidence: a systematic review and Meta-analysis. J Infect Dis. (2016) 214:6–15. doi: 10.1093/infdis/jiw081
35. Khalil, I, Walker, R, Porter, CK, Muhib, F, Chilengi, R, Cravioto, A, et al. Enterotoxigenic Escherichia coli (ETEC) vaccines: Priority activities to enable product development, licensure, and global access. Vaccine. (2021) 39:4266–77. doi: 10.1016/j.vaccine.2021.04.018
36. Troeger, C, Khalil, IA, Rao, PC, Cao, S, Blacker, BF, Ahmed, T, et al. Rotavirus Vaccination and the Global Burden of Rotavirus Diarrhea Among Children Younger Than 5 Years. JAMA Pediatr. (2018) 172:958–65. doi: 10.1001/jamapediatrics.2018.1960
37. Asare, EO, Al-Mamun, MA, Sarmin, M, Faruque, ASG, Ahmed, T, and Pitzer, VE. The influence of demographic and meteorological factors on temporal patterns of rotavirus infection in Dhaka, Bangladesh. Proc R Soc B Biol Sci. (2022) 289:20212727. doi: 10.1098/RSPB.2021.2727
38. Kirkwood, CD, Ma, LF, Carey, ME, and Steele, AD. The rotavirus vaccine development pipeline. Vaccine. (2019) 37:7328–35. doi: 10.1016/j.vaccine.2017.03.076
39. Keddy, KH. The considerable complexities of rotavirus vaccination. Lancet Infect Dis. (2022) 22:1520–2. doi: 10.1016/S1473-3099(22)00496-0
40. Song, YJ, Cheong, HK, Ki, M, Shin, JY, Hwang, SS, Park, M, et al. The epidemiological influence of climatic factors on shigellosis incidence rates in Korea. Int J Environ Res Public Health. (2018) 15:2209. doi: 10.3390/ijerph15102209
41. ClinicalTrials.gov. Efficacy, Immunogenicity and Safety of S. Flexneriza-S. Sonnei Bivalent Conjugate Vaccine in Volunteers Aged From 6 Months to 5 Years. (2022). Available at: https://clinicaltrials.gov/ct2/show/NCT05156528?cond=shigella&cntry=CN&draw=2&rank=1 (Accessed November 10, 2022).
42. MacLennan, CA, and Steele, AD. Frontiers in Shigella Vaccine Development. Vaccine. (2022) 10:1536. doi: 10.3390/vaccines10091536
43. Costa, F, Hagan, JE, Calcagno, J, Kane, M, Torgerson, P, Martinez-Silveira, MS, et al. Global Morbidity and Mortality of Leptospirosis: A Systematic Review. PLoS Negl Trop Dis. (2015) 9:e0003898. doi: 10.1371/journal.pntd.0003898
44. Blum, AJ, and Hotez, PJ. Global “worming”: climate change and its projected general impact on human helminth infections. PLoS Negl Trop Dis. (2018) 12:e0006370. doi: 10.1371/journal.pntd.0006370
45. Adegnika, AA, de Vries, SG, Zinsou, FJ, Honkepehedji, YJ, Dejon Agobé, JC, Vodonou, KG, et al. Safety and immunogenicity of co-administered hookworm vaccine candidates Na-GST-1 and Na-APR-1 in Gabonese adults: a randomised, controlled, double-blind, phase 1 dose-escalation trial. Lancet Infect Dis. (2021) 21:275–85. doi: 10.1016/S1473-3099(20)30288-7
46. De Leo, GA, Stensgaard, AS, Sokolow, SH, N’Goran, EK, Chamberlin, AJ, Yang, GJ, et al. Schistosomiasis and climate change. BMJ. (2020) 371:m4324. doi: 10.1136/BMJ.M4324
47. Stensgaard, AS, Vounatsou, P, Sengupta, ME, and Utzinger, J. Schistosomes, snails and climate change: current trends and future expectations. Acta Trop. (2019) 190:257–68. doi: 10.1016/j.actatropica.2018.09.013
48. Molehin, AJ, McManus, DP, and You, H. Vaccines for human schistosomiasis: recent Progress, new developments and future prospects. Int J Mol Sci. (2022) 23:2255. doi: 10.3390/ijms23042255
49. Panzner, U, Excler, J-L, Kim, JH, Marks, F, Carter, D, and Siddiqui, AA. Recent advances and methodological considerations on vaccine candidates for human schistosomiasis. Front Trop Dis. (2021) 2:9369. doi: 10.3389/FITD.2021.719369
50. Carlson, CJ, Bannon, E, Mendenhall, E, Newfield, T, and Bansal, S. Rapid range shifts in African Anopheles mosquitoes over the last century. Biol Lett. (2023) 19:365. doi: 10.1098/rsbl.2022.0365
51. Fischer, L, Gültekin, N, Kaelin, MB, Fehr, J, and Schlagenhauf, P. Rising temperature and its impact on receptivity to malaria transmission in Europe: a systematic review. Travel Med Infect Dis. (2020) 36:101815. doi: 10.1016/j.tmaid.2020.101815
52. Caminade, C, Kovats, S, Rocklov, J, Tompkins, AM, Morse, AP, Colón-González, FJ, et al. Impact of climate change on global malaria distribution. Proc Natl Acad Sci U S A. (2014) 111:3286–91. doi: 10.1073/pnas.1302089111
53. Romanello, M, McGushin, A, Di Napoli, C, Drummond, P, Hughes, N, Jamart, L, et al. The 2021 report of the lancet countdown on health and climate change: code red for a healthy future. Lancet. (2021) 398:1619–62. doi: 10.1016/S0140-6736(21)01787-6
54. Wang, Z, Liu, Y, Li, Y, Wang, G, Lourenço, J, Kraemer, M, et al. The relationship between rising temperatures and malaria incidence in Hainan, China, from 1984 to 2010: a longitudinal cohort study. Lancet Planet Heal. (2022) 6:e350–8. doi: 10.1016/S2542-5196(22)00039-0
55. Datoo, MS, Natama, MH, Somé, A, Traoré, O, Rouamba, T, Bellamy, D, et al. Efficacy of a low-dose candidate malaria vaccine, R21 in adjuvant matrix-M, with seasonal administration to children in Burkina Faso: a randomised controlled trial. Lancet. (2021) 397:1809–18. doi: 10.1016/S0140-6736(21)00943-0
56. RTS SCTP. Efficacy and safety of RTS,S/AS01 malaria vaccine with or without a booster dose in infants and children in Africa: final results of a phase 3, individually randomised, controlled trial. Lancet. (2015) 386:31–45. doi: 10.1016/S0140-6736(15)60721-8
57. Mordecai, EA, Ryan, SJ, Caldwell, JM, Shah, MM, and LaBeaud, AD. Climate change could shift disease burden from malaria to arboviruses in Africa. Lancet Planet Heal. (2020) 4:e416–23. doi: 10.1016/S2542-5196(20)30178-9
58. Sintayehu, DW, Tassie, N, and De Boer, WF. Present and future climatic suitability for dengue fever in Africa. Infect Ecol Epidemiol. (2020) 10:1782042. doi: 10.1080/20008686.2020.1782042
59. Oliveira, S, Rocha, J, Sousa, CA, and Capinha, C. Wide and increasing suitability for Aedes albopictus in Europe is congruent across distribution models. Sci Reports. (2021) 11:9916–9. doi: 10.1038/s41598-021-89096-5
60. Wang, WH, Urbina, AN, Lin, CY, Yang, ZS, Assavalapsakul, W, Thitithanyanont, A, et al. Targets and strategies for vaccine development against dengue viruses. Biomed Pharmacother. (2021) 144:112304. doi: 10.1016/j.biopha.2021.112304
61. World Health Organization. (2018). Vaccines and immunization: Dengue. Available at: https://www.who.int/news-room/questions-and-answers/item/dengue-vaccines (Accessed November 9, 2022).
62. Romanello, M, Di, NC, Drummond, P, Green, C, Kennard, H, Lampard, P, et al. The 2022 report of the lancet countdown on health and climate change: health at the mercy of fossil fuels. Lancet. (2022) 400:1619–54. doi: 10.1016/S0140-6736(22)01540-9
63. Ryan, SJ, Carlson, CJ, Tesla, B, Bonds, MH, Ngonghala, CN, Mordecai, EA, et al. Warming temperatures could expose more than 1.3 billion new people to Zika virus risk by 2050. Glob Chang Biol. (2021) 27:84–93. doi: 10.1111/gcb.15384
64. Makhluf, H, and Shresta, S. Development of Zika Virus Vaccines. Vaccines (Basel). (2018) 6:7. doi: 10.3390/vaccines6010007
65. World Health Organization. Global vector control response 2017–2030. Geneva: World Health Organization (2017).
66. Ryan, SJ, Carlson, CJ, Mordecai, EA, and Johnson, LR. Global expansion and redistribution of Aedes-borne virus transmission risk with climate change. PLoS Negl Trop Dis. (2019) 13:e0007213. doi: 10.1371/JOURNAL.PNTD.0007213
67. Fischer, D, Thomas, SM, Suk, JE, Sudre, B, Hess, A, Tjaden, NB, et al. Climate change effects on chikungunya transmission in Europe: geospatial analysis of vector’s climatic suitability and virus’ temperature requirements. Int J Health Geogr. (2013) 12:51. doi: 10.1186/1476-072X-12-51
68. Tjaden, NB, Suk, JE, Fischer, D, Thomas, SM, Beierkuhnlein, C, and Semenza, JC. Modelling the effects of global climate change on chikungunya transmission in the 21st century. Sci Rep. (2017) 7:3813. doi: 10.1038/s41598-017-03566-3
69. Schmidt, C, and Schnierle, BS. Chikungunya vaccine candidates: current landscape and future prospects. Drug Des Devel Ther. (2022) 16:3663–73. doi: 10.2147/DDDT.S366112
70. Gaythorpe, KAM, Hamlet, A, Cibrelus, L, Garske, T, and Ferguson, NM. The effect of climate change on yellow fever disease burden in Africa. elife. (2020) 9:55619. doi: 10.7554/eLife.55619
71. Sadeghieh, T, Sargeant, JM, Greer, AL, Berke, O, Dueymes, G, Gachon, P, et al. Yellow fever virus outbreak in Brazil under current and future climate. Infect Dis Model. (2021) 6:664–77. doi: 10.1016/j.idm.2021.04.002
72. Hansen, CA, and Barrett, ADT. The present and future of yellow fever vaccines. Pharmaceuticals. (2021) 14:891. doi: 10.3390/PH14090891
73. World Health Organization. Vaccines and vaccination against yellow fever. Wkly Epidemiol Rec. (2013) 88:269–83.
74. Mweya, CN, Mboera, LEG, and Kimera, SI. Climate influence on emerging risk areas for Rift Valley fever epidemics in Tanzania. Am J Trop Med Hyg. (2017) 97:109–14. doi: 10.4269/AJTMH.16-0444
75. Iacono, GL, Cunningham, AA, Bett, B, Grace, D, Redding, DW, and Wood, JLN. Environmental limits of Rift Valley fever revealed using ecoepidemiological mechanistic models. Proc Natl Acad Sci U S A. (2018) 115:E7448–56. doi: 10.1073/pnas.1803264115
76. Redding, DW, Tiedt, S, Lo Iacono, G, Bett, B, and Jones, KE. Spatial, seasonal and climatic predictive models of Rift Valley fever disease across Africa. Philos Trans R Soc B Biol Sci. (2017) 372:20160165. doi: 10.1098/RSTB.2016.0165
77. Jenkin, D, Wright, D, Folegatti, PM, Platt, A, Poulton, I, Lawrie, A, et al. Safety and immunogenicity of a ChAdOx1 vaccine against Rift Valley fever in UK adults: an open-label, non-randomised, first-in-human phase 1 clinical trial. Lancet Infect Dis. (2023) 23:956–64. doi: 10.1016/S1473-3099(23)00068-3
78. Local Burden of Disease 2019 Neglected Tropical Diseases Collaborators. The global distribution of lymphatic filariasis, 2000–18: a geospatial analysis. Lancet Glob Heal. (2020) 8:e1186–e1194. doi: 10.1016/S2214-109X(20)30286-2
79. Slater, H, and Michael, E. Predicting the current and future potential distributions of lymphatic Filariasis in Africa using maximum entropy ecological niche modelling. PLoS One. (2012) 7:e32202. doi: 10.1371/journal.pone.0032202
80. Samy, AM, Elaagip, AH, Kenawy, MA, Ayres, CFJ, Peterson, AT, and Soliman, DE. Climate change influences on the global potential distribution of the mosquito Culex quinquefasciatus, vector of West Nile virus and lymphatic Filariasis. PLoS One. (2016) 11:e0163863. doi: 10.1371/JOURNAL.PONE.0163863
81. Kalyanasundaram, R, Khatri, V, and Chauhan, N. Advances in vaccine development for human lymphatic Filariasis. Trends Parasitol. (2020) 36:195. doi: 10.1016/j.pt.2019.11.005
82. World Health Organization. (2023). Leishmaniasis. Available at: https://www.who.int/news-room/fact-sheets/detail/leishmaniasis (Accessed June 29, 2023).
83. González, C, Wang, O, Strutz, SE, González-Salazar, C, Sánchez-Cordero, V, and Sarkar, S. Climate change and risk of Leishmaniasis in North America: predictions from ecological niche models of vector and reservoir species. PLoS Negl Trop Dis. (2010) 4:e585. doi: 10.1371/journal.pntd.0000585
84. Koch, LK, Kochmann, J, Klimpel, S, and Cunze, S. Modeling the climatic suitability of leishmaniasis vector species in Europe. Sci Reports. (2017) 7:1–10. doi: 10.1038/s41598-017-13822-1
85. Gillespie, PM, Beaumier, CM, Strych, U, Hayward, T, Hotez, PJ, and Bottazzi, ME. Status of vaccine research and development of vaccines for leishmaniasis. Vaccine. (2016) 34:2992–5. doi: 10.1016/j.vaccine.2015.12.071
86. Couper, LI, MacDonald, AJ, and Mordecai, EA. Impact of prior and projected climate change on US Lyme disease incidence. Glob Chang Biol. (2021) 27:738–54. doi: 10.1111/GCB.15435
87. Ogden, NH, Maarouf, A, Barker, IK, Bigras-Poulin, M, Lindsay, LR, Morshed, MG, et al. Climate change and the potential for range expansion of the Lyme disease vector Ixodes scapularis in Canada. Int J Parasitol. (2006) 36:63–70. doi: 10.1016/j.ijpara.2005.08.016
88. McPherson, M, García-García, A, Cuesta-Valero, FJ, Beltrami, H, Hansen-Ketchum, P, Macdougall, D, et al. Expansion of the Lyme disease vector Ixodes Scapularis in Canada inferred from CMIP5 climate projections. Environ Health Perspect. (2017) 125:057008. doi: 10.1289/EHP57
89. Bouchard, C, Dibernardo, A, Koffi, J, Wood, H, Leighton, P, and Lindsay, L. Increased risk of tick-borne diseases with climate and environmental changes. Canada Commun Dis Rep. (2019) 45:83–9. doi: 10.14745/CCDR.V45I04A02
90. Jaenson, TGT, Jaenson, DGE, Eisen, L, Petersson, E, and Lindgren, E. Changes in the geographical distribution and abundance of the tick Ixodes ricinus during the past 30 years in Sweden. Parasites and Vectors. (2012) 5:1–15. doi: 10.1186/1756-3305-5-8
91. Ogden, NH, Ben Beard, C, Ginsberg, HS, and Tsao, JI. Possible effects of climate change on Ixodid ticks and the pathogens they transmit: predictions and observations. J Med Entomol. (2021) 58:1536–45. doi: 10.1093/jme/tjaa220
92. CDC. (2022). Lyme disease vaccine. Available at: https://www.cdc.gov/lyme/prev/vaccine.html (Accessed November 10, 2022).
93. Kriz, B, Maly, M, Benes, C, and Daniel, M. Epidemiology of tick-borne encephalitis in the Czech Republic 1970–2008. Vector Borne Zoonotic Dis. (2012) 12:994–9. doi: 10.1089/vbz.2011.0900
94. Lukan, M, Bullova, E, and Petko, B. Climate warming and tick-borne encephalitis. Slovakia Emerg Infect Dis. (2010) 16:524–6. doi: 10.3201/EID1603.081364
95. Daniel, M, Danielová, V, Fialová, A, Malý, M, Kříž, B, and Nuttall, PA. Increased relative risk of tick-borne encephalitis in warmer weather. Front Cell Infect Microbiol. (2018) 8:90. doi: 10.3389/FCIMB.2018.00090/BIBTEX
96. Lindgren, E, and Gustafson, R. Tick-borne encephalitis in Sweden and climate change. Lancet. (2001) 358:16–8. doi: 10.1016/S0140-6736(00)05250-8
97. Tokarevich, NK, Tronin, AA, Blinova, OV, Buzinov, RV, Boltenkov, VP, Yurasova, ED, et al. The impact of climate change on the expansion of Ixodes persulcatus habitat and the incidence of tick-borne encephalitis in the north of European Russia. Glob Health Action. (2011) 4:8448. doi: 10.3402/gha.v4i0.8448
98. Nah, K, Bede-Fazekas, Á, Trájer, AJ, and Wu, J. The potential impact of climate change on the transmission risk of tick-borne encephalitis in Hungary. BMC Infect Dis. (2020) 20:34. doi: 10.1186/s12879-019-4734-4
99. Kubinski, M, Beicht, J, Gerlach, T, Volz, A, Sutter, G, and Rimmelzwaan, GF. Tick-Borne Encephalitis Virus: A Quest for Better Vaccines against a Virus on the Rise. Vaccines (Basel). (2020) 8:451. doi: 10.3390/vaccines8030451
100. Temur, AI, Kuhn, JH, Pecor, DB, Apanaskevich, DA, and Keshtkar-Jahromi, M. Epidemiology of Crimean-Congo hemorrhagic fever (CCHF) in Africa—underestimated for decades. Am J Trop Med Hyg. (2021) 104:1978–90. doi: 10.4269/ajtmh.20-1413
101. Grandi, G, Chitimia-Dobler, L, Choklikitumnuey, P, Strube, C, Springer, A, Albihn, A, et al. First records of adult Hyalomma marginatum and H. rufipes ticks (Acari: Ixodidae) in Sweden. Ticks Tick Borne Dis. (2020) 11:101403. doi: 10.1016/j.ttbdis.2020.101403
102. Belobo, JTE, Kenmoe, S, Kengne-Nde, C, Emoh, CPD, Bowo-Ngandji, A, Tchatchouang, S, et al. Worldwide epidemiology of Crimean-Congo hemorrhagic fever virus in humans, ticks and other animal species, a systematic review and meta-analysis. PLoS Negl Trop Dis. (2021) 15:e0009299. doi: 10.1371/journal.pntd.0009299
103. Williams, HW, Cross, DE, Crump, HL, Drost, CJ, and Thomas, CJ. Climate suitability for European ticks: assessing species distribution models against null models and projection under AR5 climate. Parasit Vectors. (2015) 8:440. doi: 10.1186/S13071-015-1046-4
104. Tipih, T, and Burt, FJ. Crimean-Congo hemorrhagic fever virus: advances in vaccine development. Biores Open Access. (2020) 9:137–50. doi: 10.1089/BIORES.2019.0057
105. World Health Organization. (2018). Influenza (Seasonal). Available at: https://www.who.int/news-room/fact-sheets/detail/influenza-(seasonal) (Accessed September 13, 2022).
106. Lowen, AC, and Steel, J. Roles of humidity and temperature in shaping influenza seasonality. J Virol. (2014) 88:7692. doi: 10.1128/JVI.03544-13
107. Tamerius, JD, Shaman, J, Alonso, WJ, Bloom-Feshbach, K, Uejio, CK, Comrie, A, et al. Environmental predictors of seasonal influenza epidemics across temperate and tropical climates. PLoS Pathog. (2013) 9:e1003194. doi: 10.1371/JOURNAL.PPAT.1003194
108. Park, JE, Son, WS, Ryu, Y, Choi, SB, Kwon, O, and Ahn, I. Effects of temperature, humidity, and diurnal temperature range on influenza incidence in a temperate region. Influenza Other Respir Viruses. (2020) 14:11–8. doi: 10.1111/IRV.12682
109. Lane, MA, Walawender, M, Carter, J, Brownsword, EA, Landay, T, Gillespie, TR, et al. Climate change and influenza: a scoping review. J Clim Chang Heal. (2022) 5:100084. doi: 10.1016/J.JOCLIM.2021.100084
110. Qi, L, Liu, T, Gao, Y, Li, Q, Tang, W, Tian, D, et al. Effect of absolute humidity on influenza activity across different climate regions in China. Environ Sci Pollut Res. (2022) 29:49373–84. doi: 10.1007/S11356-022-19279-8
111. Bathiany, S, Dakos, V, Scheffer, M, and Lenton, TM. Climate models predict increasing temperature variability in poor countries. Sci Adv. (2018) 4:eaar5809. doi: 10.1126/SCIADV.AAR5809
112. Liu, Q, Tan, ZM, Sun, J, Hou, Y, Fu, C, and Wu, Z. Changing rapid weather variability increases influenza epidemic risk in a warming climate. Environ Res Lett. (2020) 15:044004. doi: 10.1088/1748-9326/AB70BC
113. Wei, C-J, Crank, MC, Shiver, J, Graham, BS, Mascola, JR, and Nabel, GJ. Next-generation influenza vaccines: opportunities and challenges. Nat Rev Drug Discov. (2020) 19:239–52. doi: 10.1038/s41573-020-0066-8
114. Li, Y, Wang, X, Blau, DM, Caballero, MT, Feikin, DR, Gill, CJ, et al. Global, regional, and national disease burden estimates of acute lower respiratory infections due to respiratory syncytial virus in children younger than 5 years in 2019: a systematic analysis. Lancet. (2022) 399:2047–64. doi: 10.1016/S0140-6736(22)00478-0
115. Baker, RE, Mahmud, AS, Wagner, CE, Yang, W, Pitzer, VE, Viboud, C, et al. Epidemic dynamics of respiratory syncytial virus in current and future climates. Nat Commun. (2019) 10:5512. doi: 10.1038/s41467-019-13562-y
116. Radhakrishnan, D, Ouedraogo, A, Shariff, SZ, McNally, JD, Benchimol, EI, and Clemens, KK. The association between climate, geography and respiratory syncitial virus hospitalizations among children in Ontario, Canada: a population-based study. BMC Infect Dis. (2020) 20:157. doi: 10.1186/S12879-020-4882-6
117. Suryadevara, M, and Domachowske, JB. Epidemiology and seasonality of childhood respiratory syncytial virus infections in the tropics. Viruses. (2021) 13:696. doi: 10.3390/V13040696
118. European Medicines Agency. (2023). First RSV vaccine to protect infants up to 6 months of age and older adults | European Medicines Agency. Available at: https://www.ema.europa.eu/en/news/first-rsv-vaccine-protect-infants-6-months-age-older-adults (Accessed August 17, 2023).
119. U.S. Food and Drug Administration. (2023). FDA approves first respiratory syncytial virus (RSV) vaccine | FDA. Available at: https://www.fda.gov/news-events/press-announcements/fda-approves-first-respiratory-syncytial-virus-rsv-vaccine (Accessed August 17, 2023.
120. Qiu, X, Xu, S, Lu, Y, Luo, Z, Yan, Y, Wang, C, et al. Development of mRNA vaccines against respiratory syncytial virus (RSV). Cytokine Growth Factor Rev. (2022) 68:37–53. doi: 10.1016/j.cytogfr.2022.10.001
121. Chadsuthi, S, Chalvet-Monfray, K, Wiratsudakul, A, and Modchang, C. The effects of flooding and weather conditions on leptospirosis transmission in Thailand. Sci Rep. (2021) 11:1486. doi: 10.1038/s41598-020-79546-x
122. Cunha, M, Costa, F, Ribeiro, GS, Carvalho, MS, Reis, RB, Nery, N, et al. Rainfall and other meteorological factors as drivers of urban transmission of leptospirosis. PLoS Negl Trop Dis. (2022) 16:e0007507. doi: 10.1371/journal.pntd.0007507
123. Xu, Y, and Ye, Q. Human leptospirosis vaccines in China. Hum Vaccin Immunother. (2018) 14:984–93. doi: 10.1080/21645515.2017.1405884
124. World Health Organization. (2022). Drinking-water. Available at: https://www.who.int/news-room/fact-sheets/detail/drinking-water (Accessed August 15, 2022).
125. Singh, RBK, Hales, S, De Wet, N, Raj, R, Hearnden, M, and Weinstein, P. The influence of climate variation and change on diarrheal disease in the Pacific Islands. Environ Health Perspect. (2001) 109:155–9. doi: 10.1289/ehp.01109155
126. Delahoy, MJ, Cárcamo, C, Huerta, A, Lavado, W, Escajadillo, Y, Ordoñez, L, et al. Meteorological factors and childhood diarrhea in Peru, 2005–2015: a time series analysis of historic associations, with implications for climate change. Environ Health. (2021) 20:22. doi: 10.1186/S12940-021-00703-4
127. Bhandari, D, Bi, P, Sherchand, JB, Dhimal, M, and Hanson-Easey, S. Assessing the effect of climate factors on childhood diarrhoea burden in Kathmandu. Nepal Int J Hyg Environ Health. (2020) 223:199–206. doi: 10.1016/J.IJHEH.2019.09.002
128. Dhimal, M, Bhandari, D, Karki, KB, Shrestha, SL, Khanal, M, Shrestha, RRP, et al. Effects of climatic factors on diarrheal diseases among children below 5 years of age at national and subnational levels in Nepal: an ecological study. Int J Environ Res Public Health. (2022) 19:6138. doi: 10.3390/IJERPH19106138
129. Levy, K, Woster, AP, Goldstein, RS, and Carlton, EJ. Untangling the impacts of climate change on waterborne diseases: a systematic review of relationships between diarrheal diseases and temperature, rainfall, flooding, and drought. Environ Sci Technol. (2016) 50:4905–22. doi: 10.1021/ACS.EST.5B06186
130. Wang, B, Luo, X, Yang, YM, Sun, W, Cane, MA, Cai, W, et al. Historical change of El Niño properties sheds light on future changes of extreme El Niño. Proc Natl Acad Sci U S A. (2019) 116:22512–7. doi: 10.1073/pnas.1911130116
131. Cai, W, Santoso, A, Collins, M, Dewitte, B, Karamperidou, C, Kug, JS, et al. Changing El Niño–southern oscillation in a warming climate. Nat Rev Earth Environ. (2021) 2:628–44. doi: 10.1038/s43017-021-00199-z
132. Anyamba, A, Chretien, JP, Britch, SC, Soebiyanto, RP, Small, JL, Jepsen, R, et al. Global disease outbreaks associated with the 2015–2016 El Niño event. Sci Rep. (2019) 9:1930. doi: 10.1038/s41598-018-38034-z
133. Kouadio, IK, Aljunid, S, Kamigaki, T, Hammad, K, and Oshitani, H. Infectious diseases following natural disasters: prevention and control measures. Expert Rev Anti Infect Ther. (2014) 10:95–104. doi: 10.1586/ERI.11.155
134. UN News. (2023). Tropical cyclone Freddy set to further weaken cholera-hit Malawi. Available at: https://news.un.org/en/story/2023/03/1134267 (Accessed March 18, 2023).
135. World Health Organization. (2022). Disease outbreak news; cholera in Somalia. Available at: https://www.who.int/emergencies/disease-outbreak-news/item/2022-DON398_1 (Accessed February 7, 2023).
136. Asadgol, Z, Mohammadi, H, Kermani, M, Badirzadeh, A, and Gholami, M. The effect of climate change on cholera disease: the road ahead using artificial neural network. PLoS One. (2019) 14:e0224813. doi: 10.1371/JOURNAL.PONE.0224813
137. Escobar, LE, Ryan, SJ, Stewart-Ibarra, AM, Finkelstein, JL, King, CA, Qiao, H, et al. A global map of suitability for coastal Vibrio cholerae under current and future climate conditions. Acta Trop. (2015) 149:202–11. doi: 10.1016/j.actatropica.2015.05.028
138. van Vuuren, DP, Edmonds, J, Kainuma, M, Riahi, K, Thomson, A, Hibbard, K, et al. The representative concentration pathways: an overview. Clim Chang. (2011) 109:5–31. doi: 10.1007/s10584-011-0148-z
139. Akil, L, Anwar Ahmad, H, and Reddy, RS. Effects of climate change on Salmonella infections. Foodborne Pathog Dis. (2014) 11:974–80. doi: 10.1089/fpd.2014.1802
140. Chua, PLC, Ng, CFS, Tobias, A, Seposo, XT, and Hashizume, M. Associations between ambient temperature and enteric infections by pathogen: a systematic review and meta-analysis. Lancet Planet Heal. (2022) 6:e202–18. doi: 10.1016/S2542-5196(22)00003-1
141. Short, EE, Caminade, C, and Thomas, BN. Climate change contribution to the emergence or re-emergence of parasitic diseases. Infect Dis (Auckl). (2017) 10:1178633617732296. doi: 10.1177/1178633617732296
142. Loukas, A, Hotez, PJ, Diemert, D, Yazdanbakhsh, M, McCarthy, JS, Correa-Oliveira, R, et al. Hookworm infection. Nat Rev Dis Prim. (2016) 2:1–18. doi: 10.1038/nrdp.2016.88
143. Douchet, L, Goarant, C, Mangeas, M, Menkes, C, Hinjoy, S, and Herbreteau, V. Unraveling the invisible leptospirosis in mainland Southeast Asia and its fate under climate change. Sci Total Environ. (2022) 832:155018. doi: 10.1016/J.SCITOTENV.2022.155018
144. Lau, CL, Smythe, LD, Craig, SB, and Weinstein, P. Climate change, flooding, urbanisation and leptospirosis: Fuelling the fire? Trans R Soc Trop Med Hyg. (2010) 104:631–8. doi: 10.1016/J.TRSTMH.2010.07.002
145. World Health Organization. (2020). Vector-borne diseases. Available at: https://www.who.int/news-room/fact-sheets/detail/vector-borne-diseases (Accessed August 6, 2022).
146. World Health Organization. (2009). Protecting health from climate change. Connecting science, policy, people. Geneva. Available at: https://apps.who.int/iris/handle/10665/44246
147. Bellone, R, and Failloux, AB. The role of temperature in shaping mosquito-borne viruses transmission. Front Microbiol. (2020) 11:2388. doi: 10.3389/FMICB.2020.584846
148. Liu, Q, Jing, W, Kang, L, Liu, J, and Liu, M. Trends of the global, regional and national incidence of malaria in 204 countries from 1990 to 2019 and implications for malaria prevention. J Travel Med. (2021) 28:46. doi: 10.1093/jtm/taab046
149. Cowman, AF, Healer, J, Marapana, D, and Marsh, K. Malaria: biology and disease. Cells. (2016) 167:610–24. doi: 10.1016/j.cell.2016.07.055
150. Mordecai, EA, Paaijmans, KP, Johnson, LR, Balzer, C, Ben-Horin, T, de Moor, E, et al. Optimal temperature for malaria transmission is dramatically lower than previously predicted. Ecol Lett. (2013) 16:22–30. doi: 10.1111/ele.12015
151. Christiansen-Jucht, C, Parham, PE, Saddler, A, Koella, JC, and Basáñez, MG. Temperature during larval development and adult maintenance influences the survival of Anopheles gambiae s.s. Parasit Vectors. (2014) 7:489. doi: 10.1186/s13071-014-0489-3
152. Rocklöv, J, and Dubrow, R. Climate change: an enduring challenge for vector-borne disease prevention and control. Nat Immunol. (2020) 21:479–83. doi: 10.1038/s41590-020-0648-y
153. Sinka, ME, Pironon, S, Massey, NC, Longbottom, J, Hemingway, J, Moyes, CL, et al. A new malaria vector in Africa: predicting the expansion range of Anopheles stephensi and identifying the urban populations at risk. Proc Natl Acad Sci U S A. (2020) 117:24900–8. doi: 10.1073/pnas.2003976117
154. Villena, OC, Ryan, SJ, Murdock, CC, and Johnson, LR. Temperature impacts the environmental suitability for malaria transmission by Anopheles gambiae and Anopheles stephensi. Ecology. (2022) 103:e3685. doi: 10.1002/ecy.3685
155. Bäck, AT, and Lundkvist, Å. Dengue viruses – an overview. Infect Ecol Epidemiol. (2013) 3:19839. doi: 10.3402/IEE.V3I0.19839
156. Ebi, KL, and Nealon, J. Dengue in a changing climate. Environ Res. (2016) 151:115–23. doi: 10.1016/j.envres.2016.07.026
157. Li, Y, Dou, Q, Lu, Y, Xiang, H, Yu, X, and Liu, S. Effects of ambient temperature and precipitation on the risk of dengue fever: a systematic review and updated meta-analysis. Environ Res. (2020) 191:110043. doi: 10.1016/j.envres.2020.110043
158. Puntasecca, CJ, King, CH, and Labeaud, AD. Measuring the global burden of chikungunya and Zika viruses: a systematic review. PLoS Negl Trop Dis. (2021) 15:e0009055. doi: 10.1371/journal.pntd.0009055
159. Caminade, C, Turner, J, Metelmann, S, Hesson, JC, Blagrove, MSC, Solomon, T, et al. Global risk model for vector-borne transmission of Zika virus reveals the role of El Niño 2015. Proc Natl Acad Sci U S A. (2017) 114:119–24. doi: 10.1073/pnas.1614303114
160. Moore, SM, Oidtman, RJ, James Soda, K, Siraj, AS, Reiner, RC, Barker, CM, et al. Leveraging multiple data types to estimate the size of the Zika epidemic in the Americas. PLoS Negl Trop Dis. (2020) 14:e0008640. doi: 10.1371/journal.pntd.0008640
161. Russo, G, Subissi, L, and Rezza, G. Chikungunya fever in Africa: a systematic review. Pathog Glob Health. (2020) 114:111–9. doi: 10.1080/20477724.2020.1748965
162. Bettis, AA, L’Azou Jackson, M, Yoon, IK, Breugelmans, JG, Goios, A, Gubler, DJ, et al. The global epidemiology of chikungunya from 1999 to 2020: a systematic literature review to inform the development and introduction of vaccines. PLoS Negl Trop Dis. (2022) 16:e0010069. doi: 10.1371/JOURNAL.PNTD.0010069
163. World Health Organization. Yellow fever. Key Facts. (2019). Available at: https://www.who.int/news-room/fact-sheets/detail/yellow-fever (Accessed August 7, 2022).
164. Marques, AR, Strle, F, and Wormser, GP. Comparison of Lyme disease in the United States and Europe. Emerg Infect Dis. (2021) 27:2017–24. doi: 10.3201/eid2708.204763
165. Süss, J, Klaus, C, Gerstengarbe, FW, and Werner, PC. What makes ticks tick? Climate change, ticks, and tick-borne diseases. J Travel Med. (2008) 15:39–45. doi: 10.1111/J.1708-8305.2007.00176.X
166. Lee, JS, and Chung, SY. The threat of climate change on tick-borne infections: rising trend of infections and geographical distribution of climate risk factors associated with ticks. J Infect Dis. (2022) 227:295–303. doi: 10.1093/INFDIS/JIAC300
167. Fernández-Ruiz, N, and Estrada-Peña, A. Towards new horizons: climate trends in Europe increase the environmental suitability for permanent populations of Hyalomma marginatum (Ixodidae). Pathogens. (2021) 10:95. doi: 10.3390/pathogens10020095
168. He, Y, Liu, WJ, Jia, N, Richardson, S, and Huang, C. Viral respiratory infections in a rapidly changing climate: the need to prepare for the next pandemic. EBioMedicine. (2023) 93:104593. doi: 10.1016/j.ebiom.2023.104593
169. Micoli, F, Bagnoli, F, Rappuoli, R, and Serruto, D. The role of vaccines in combatting antimicrobial resistance. Nat Rev Microbiol. (2021) 19:287–302. doi: 10.1038/s41579-020-00506-3
170. Teoh, SL, Kotirum, S, Hutubessy, RCW, and Chaiyakunapruk, N. Global economic evaluation of oral cholera vaccine: a systematic review. Hum Vaccin Immunother. (2018) 14:420–9. doi: 10.1080/21645515.2017.1392422
171. Longini, IM, Nizam, A, Ali, M, Yunus, M, Shenvi, N, and Clemens, JD. Controlling endemic cholera with Oral vaccines. PLoS Med. (2007) 4:e336. doi: 10.1371/journal.pmed.0040336
172. Wierzba, TF. Oral cholera vaccines and their impact on the global burden of disease. Hum Vaccin Immunother. (2019) 15:1294–301. doi: 10.1080/21645515.2018.1504155
173. GAVI The Vaccine Alliance. (2022). Oral cholera vaccine support. https://www.gavi.org/types-support/vaccine-support/oral-cholera (Accessed November 5, 2022).
174. World Health Organization. (2022). Shortage of cholera vaccines leads to temporary suspension of two-dose strategy, as cases rise worldwide. Available at: https://www.who.int/news/item/19-10-2022-shortage-of-cholera-vaccines-leads-to-temporary-suspension-of-two-dose-strategy--as-cases-rise-worldwide (Accessed November 5, 2022).
175. World Health Organization. (2022). Cholera – Global situation. Available at: https://www.who.int/emergencies/disease-outbreak-news/item/2022-DON426 (Accessed January 29, 2023).
176. Lee, EC, Azman, AS, Kaminsky, J, Moore, SM, McKay, HS, and Lessler, J. The projected impact of geographic targeting of oral cholera vaccination in sub-Saharan Africa: a modeling study. PLoS Med. (2019) 16:e1003003. doi: 10.1371/JOURNAL.PMED.1003003
177. Khan, AI, Levin, A, Chao, DL, DeRoeck, D, Dimitrov, DT, Khan, JAM, et al. The impact and cost-effectiveness of controlling cholera through the use of oral cholera vaccines in urban Bangladesh: a disease modeling and economic analysis. PLoS Negl Trop Dis. (2018) 12:e0006652. doi: 10.1371/JOURNAL.PNTD.0006652
178. M’Bangombe, M, Pezzoli, L, Reeder, B, Kabuluzi, S, Msyamboza, K, Masuku, H, et al. Oral cholera vaccine in cholera prevention and control. Malawi Bull World Health Organ. (2018) 96:428–35. doi: 10.2471/BLT.17.207175
179. Staples, JE, Barrett, ADT, Wilder-Smith, A, and Hombach, J. Review of data and knowledge gaps regarding yellow fever vaccine-induced immunity and duration of protection. npj Vaccines. (2020) 5:54. doi: 10.1038/s41541-020-0205-6
180. Zhao, S, Stone, L, Gao, D, and He, D. Modelling the large-scale yellow fever outbreak in Luanda, Angola, and the impact of vaccination. PLoS Negl Trop Dis. (2018) 12:e0006158. doi: 10.1371/JOURNAL.PNTD.0006158
181. Shearer, FM, Moyes, CL, Pigott, DM, Brady, OJ, Marinho, F, Deshpande, A, et al. Global yellow fever vaccination coverage from 1970 to 2016: an adjusted retrospective analysis. Lancet Infect Dis. (2017) 17:1209–17. doi: 10.1016/S1473-3099(17)30419-X
182. World Health Organization. (2021). Yellow fever – west and Central Africa. Available at: https://www.who.int/emergencies/disease-outbreak-news/item/yellow-fever---west-and-central-africa (Accessed August 15, 2022).
183. Piras-Douce, F, Raynal, F, Raquin, A, Girerd-Chambaz, Y, Gautheron, S, Sanchez, MEN, et al. Next generation live-attenuated yellow fever vaccine candidate: safety and immuno-efficacy in small animal models. Vaccine. (2021) 39:1846–56. doi: 10.1016/j.vaccine.2021.02.033
184. World Health Organization. (2021). WHO recommends groundbreaking malaria vaccine for children at risk. Available at: https://www.who.int/news/item/06-10-2021-who-recommends-groundbreaking-malaria-vaccine-for-children-at-risk (Accessed February 26, 2023).
185. Olotu, A, Fegan, G, Wambua, J, Nyangweso, G, Leach, A, Lievens, M, et al. Seven-year efficacy of RTS,S/AS01 malaria vaccine among Young African children. N Engl J Med. (2016) 374:2519–29. doi: 10.1056/NEJMoa1515257
186. Laurens, MB. RTS,S/AS01 vaccine (Mosquirix™): an overview. Hum Vaccin Immunother. (2020) 16:480–9. doi: 10.1080/21645515.2019.1669415
187. Zavala, F. RTS,S: the first malaria vaccine. J Clin Invest. (2022) 132:588. doi: 10.1172/JCI156588
188. Arora, N, Anbalagan, LC, and Pannu, AK. Towards eradication of malaria: is the WHO’s RTS,s/AS01 vaccination effective enough? Risk Manag Healthc Policy. (2021) 14:1033–9. doi: 10.2147/RMHP.S219294
189. Datoo, MS, Natama, HM, Somé, A, Bellamy, D, Traoré, O, Rouamba, T, et al. Efficacy and immunogenicity of R21/matrix-M vaccine against clinical malaria after 2 years’ follow-up in children in Burkina Faso: a phase 1/2b randomised controlled trial. Lancet Infect Dis. (2022) 22:1728–36. doi: 10.1016/S1473-3099(22)00442-X
190. Wilder-Smith, A. Dengue vaccine development by the year 2020: challenges and prospects. Curr Opin Virol. (2020) 43:71–78. doi: 10.1016/j.coviro.2020.09.004
191. Biswal, S, Reynales, H, Saez-Llorens, X, Lopez, P, Borja-Tabora, C, Kosalaraksa, P, et al. Efficacy of a tetravalent dengue vaccine in healthy children and adolescents. N Engl J Med. (2019) 381:2009–19. doi: 10.1056/NEJMoa1903869
192. Takeda. (2022). Takeda receives positive CHMP opinion for approval of dengue vaccine. Available at: https://www.takeda.com/newsroom/newsreleases/2022/Positive-CHMP-Opinion-Recommending-Approval-of-Dengue-vaccine/ (Accessed November 6, 2022).
193. Viergever, RF. The mismatch between the health research and development (R&D) that is needed and the R&D that is undertaken: an overview of the problem, the causes, and solutions. Glob Health Action. (2013) 6:22450. doi: 10.3402/gha.v6i0.22450
194. Yegros-Yegros, A, van de Klippe, W, Abad-Garcia, MF, and Rafols, I. Exploring why global health needs are unmet by research efforts: the potential influences of geography, industry and publication incentives. Heal Res policy Syst. (2020) 18:47. doi: 10.1186/S12961-020-00560-6
195. Hotez, PJ, Brindley, PJ, Bethony, JM, King, CH, Pearce, EJ, and Jacobson, J. Helminth infections: the great neglected tropical diseases. J Clin Invest. (2008) 118:1311–21. doi: 10.1172/JCI34261
196. Nuzhath, T, Hotez, PJ, Damania, A, Shuling Liu, P, and Colwell, B. Creation of a global vaccine risk index. PLoS One. (2022) 17:e0272784. doi: 10.1371/journal.pone.0272784
197. Excler, JL, Privor-Dumm, L, and Kim, JH. Supply and delivery of vaccines for global health. Curr Opin Immunol. (2021) 71:13–20. doi: 10.1016/j.coi.2021.03.009
198. Excler, JL, Saville, M, Berkley, S, and Kim, JH. Vaccine development for emerging infectious diseases. Nat Med. (2021) 2021:27, 591–600. doi: 10.1038/s41591-021-01301-0
199. Cassetti, MC, Pierson, TC, Patterson, LJ, Bok, K, DeRocco, AJ, Deschamps, AM, et al. Prototype pathogen approach for vaccine and monoclonal antibody development: a critical component of the NIAID plan for pandemic preparedness. J Infect Dis. (2023) 227:1433–41. doi: 10.1093/INFDIS/JIAC296
200. GAVI The Vaccine Alliance. (2022). Making Immunisation Sustainable – Eligibility. Available at: https://www.gavi.org/types-support/sustainability/eligibility (Accessed November 5, 2022).
201. World Health Organization. Global vaccine action plan monitoring, Evaluation & Accountability: Secretariat annual report 2020. Geneva: World Health Organization (2020).
202. GAVI The Vaccine Alliance. (2022). A new era of vaccine manufacturing in Africa. Available at: https://www.gavi.org/news-resources/knowledge-products/new-era-vaccine-manufacturing-africa#conclusion (Accessed March 17, 2023).
203. The PLOS Medicine. Vaccine equity: a fundamental imperative in the fight against COVID-19. PLoS Med. (2022) 19:e1003948. doi: 10.1371/journal.pmed.1003948
204. Nagata, JM, Epstein, A, Ganson, KT, Benmarhnia, T, and Weiser, SD. Drought and child vaccination coverage in 22 countries in sub-Saharan Africa: a retrospective analysis of national survey data from 2011 to 2019. PLoS Med. (2021) 18:e1003678. doi: 10.1371/journal.pmed.1003678
205. Eisenstein, M. Vaccination rates are falling, and its not just the COVID-19 vaccine that people are refusing. Nature. (2022) 612:S44–6. doi: 10.1038/d41586-022-04341-9
206. World Health Organization. Guidelines for the international packaging and shipping of vaccines. 6th ed. Geneva: World Health Organization (2020).
207. Kumar, R, Srivastava, V, Baindara, P, and Ahmad, A. Thermostable vaccines: an innovative concept in vaccine development. Expert Rev Vaccines. (2022) 21:811–24. doi: 10.1080/14760584.2022.2053678
208. Kharwadkar, S, Attanayake, V, Duncan, J, Navaratne, N, and Benson, J. The impact of climate change on the risk factors for tuberculosis: a systematic review. Environ Res. (2022) 212:113436. doi: 10.1016/j.envres.2022.113436
209. Galagali, PM, Kinikar, AA, and Kumar, VS. Vaccine hesitancy: obstacles and challenges. Curr Pediatr Rep. (2022) 10:241–8. doi: 10.1007/S40124-022-00278-9
210. Solís Arce, JS, Warren, SS, Meriggi, NF, Scacco, A, McMurry, N, Voors, M, et al. COVID-19 vaccine acceptance and hesitancy in low- and middle-income countries. Nat Med. (2021) 27:1385–94. doi: 10.1038/s41591-021-01454-y
211. Dimala, CA, Kadia, BM, Nji, MAM, and Bechem, NN. Factors associated with measles resurgence in the United States in the post-elimination era. Sci Reports. (2021) 11:51–10. doi: 10.1038/s41598-020-80214-3
Keywords: climate change, mitigation, vaccine-preventable diseases, vector-borne diseases, waterborne diseases, vaccine development, supply, delivery
Citation: Kim CL, Agampodi S, Marks F, Kim JH and Excler J-L (2023) Mitigating the effects of climate change on human health with vaccines and vaccinations. Front. Public Health. 11:1252910. doi: 10.3389/fpubh.2023.1252910
Received: 05 July 2023; Accepted: 04 September 2023;
Published: 12 October 2023.
Edited by:
Ellen Higginson, Wellcome Sanger Institute (WT), United KingdomReviewed by:
Pranita Sarangi, Indian Institute of Technology Roorkee, IndiaCopyright © 2023 Kim, Agampodi, Marks, Kim and Excler. This is an open-access article distributed under the terms of the Creative Commons Attribution License (CC BY). The use, distribution or reproduction in other forums is permitted, provided the original author(s) and the copyright owner(s) are credited and that the original publication in this journal is cited, in accordance with accepted academic practice. No use, distribution or reproduction is permitted which does not comply with these terms.
*Correspondence: Cara Lynn Kim, Y2wua2ltQG91dGxvb2suY29t
Disclaimer: All claims expressed in this article are solely those of the authors and do not necessarily represent those of their affiliated organizations, or those of the publisher, the editors and the reviewers. Any product that may be evaluated in this article or claim that may be made by its manufacturer is not guaranteed or endorsed by the publisher.
Research integrity at Frontiers
Learn more about the work of our research integrity team to safeguard the quality of each article we publish.