- 1ICMR - Vector Control Research Centre, Puducherry, India
- 2Indian Council of Medical Research, Hqrs New Delhi, India
Plasmodium vivax is geographically the most widely dispersed human malaria parasite species. It has shown resilience and a great deal of adaptability. Genomic studies suggest that P. vivax originated from Asia or Africa and moved to the rest of the world. Although P. vivax is evolutionarily an older species than Plasmodium falciparum, its biology, transmission, pathology, and control still require better elucidation. P. vivax poses problems for malaria elimination because of the ability of a single primary infection to produce multiple relapses over months and years. P. vivax malaria elimination program needs early diagnosis, and prompt and complete radical treatment, which is challenging, to simultaneously exterminate the circulating parasites and dormant hypnozoites lodged in the hepatocytes of the host liver. As prompt surveillance and effective treatments are rolled out, preventing primaquine toxicity in the patients having glucose-6-phosphate dehydrogenase (G6PD) deficiency should be a priority for the vivax elimination program. This review sheds light on the burden of P. vivax, changing epidemiological patterns, the hurdles in elimination efforts, and the essential tools needed not just in India but globally. These tools encompass innovative treatments for eliminating dormant parasites, coping with evolving drug resistance, and the development of potential vaccines against the parasite.
1 Introduction
Plasmodium vivax is historically a resilient and the most widely distributed malaria parasite species. It coexists with Plasmodium falciparum in different proportions and geographical regions, and infrequently mixed infection of both species is also encountered (1, 2). P. vivax is known to cause extensive morbidity and low incidence of mortality across the world (3). Africa has the highest burden of malaria but mostly of P. falciparum. The endemic countries outside Africa have a sizable burden of P. vivax clinical malaria (4). The WHO Southeast Asia Region globally accounts for 53% of the P. vivax burden, with India accounting for the majority (i.e., 47%) in 2018 (5). In India, the proportion of P. vivax and P. falciparum malaria has been equal in the last two decades, although in recent years, vivax malaria has shown fluctuating trends being 38% in 2017 (6), 52% in 2018, 54% in 2019 and 36% in 2020 (7). Vivax malaria has certain distinctive characteristics that make it highly problematic such as multiple strains of P. vivax (Table 1) which are characterized by the pattern of relapses and duration of latency (8, 9). Jennison et al reported that P. vivax populations are more genetically diverse than P. falciparum, indicating greater resilience to environmental challenges and higher levels of interbreeding within and between distant parasite populations (10). P. vivax’s unique characteristics, such as relapse, provide opportunities for the exchange and dissemination of genetic material (11).The rapid development of immunity and high transmission potential of P. vivax is a unique characteristic of this malaria parasite and presents significant challenges to malaria control and elimination efforts. The species has a shorter growth phase in vectors, produces hypnozoites, and makes gametocytes earlier to the development of clinical manifestations in the patients, which allows the gametocytes to be picked up by the mosquito before the patient seeks treatment. These features and adaptabilities provide an edge to P. vivax to maintain a steady transmission via seasonal mosquito vectors, even at lower ambient temperatures and proves to be a major challenge in controlling transmission (12). Further, due to the prolonged latency in the liver phase, P. vivax ensures survival in colder regions, making it geographically the most widely distributed malaria parasite species capable of persisting in tropics, subtropics, and temperate climates (13). Children show greater morbidity in P. vivax endemic areas than adults who frequently have asymptomatic infections (14).
The global incidence of malaria has declined in the past 15 years, largely due to P. falciparum control. Malaria incidence (cases per 1,000 people at risk) fell from 81 in 2000 to 59 in 2015, and 56 in 2019 before rising to 59 in 2020. The increase in 2020 was mainly linked to the disruption in the surveillance and vector control services caused by the COVID-19 pandemic. Similar gains have been elusive in the case of P. vivax as it is less amenable to the routine malaria control interventions designed against falciparum malaria (15). Because P. vivax was inappropriately perceived as a benign infection, control of P. vivax has made slower progress relative to P. falciparum since 1960 (16). Justifiably, global malaria elimination efforts are focused against P. falciparum due to extensive morbidity and mortality caused by it in Africa and forested areas inhabited by ethnic tribes in Asia, particularly in India. The focus is more so on children and pregnant women, and therefore more resources are available for the control of P. falciparum than P. vivax, even though both species are generally co-endemic, especially in the Southeast Asia Region. It is a prevalent view among malariologists that eradicating P. vivax is technically more cumbersome than eliminating P. falciparum due to knowledge gaps and fewer reliable tools, especially for the prevention of recurring relapses (12, 16).
2 Global burden of Plasmodium vivax
Globally, P. vivax and P. falciparum account for most human malaria infections. Though P. falciparum is known for significant global morbidity and mortality, P. vivax is common among all human Plasmodia, and despite being benign, it also causes severe and even fatal infections (13, 17). P. vivax infection threatens one-third of the world’s population (~ 2.5 billion people) (17). In the 2021 WHO Report, there were ~ 241 million estimated malaria cases accountable for about 627,000 deaths; among them, 2.0% were attributed to P. vivax. In 2020, P. vivax accounted for roughly 2% of the worldwide malaria burden, marking a notable decline from its previous contribution of nearly 8% in 2000. The vivax burden was also reduced in WHO South-East Asian region from 47.7% (2000) to 36.3% (2020) (Figure 1). In 2020, India was responsible for nearly 83 percent of all malaria infections, with P. vivax accounting for more than a third (39%) of all cases in the South-East Asia region (18).
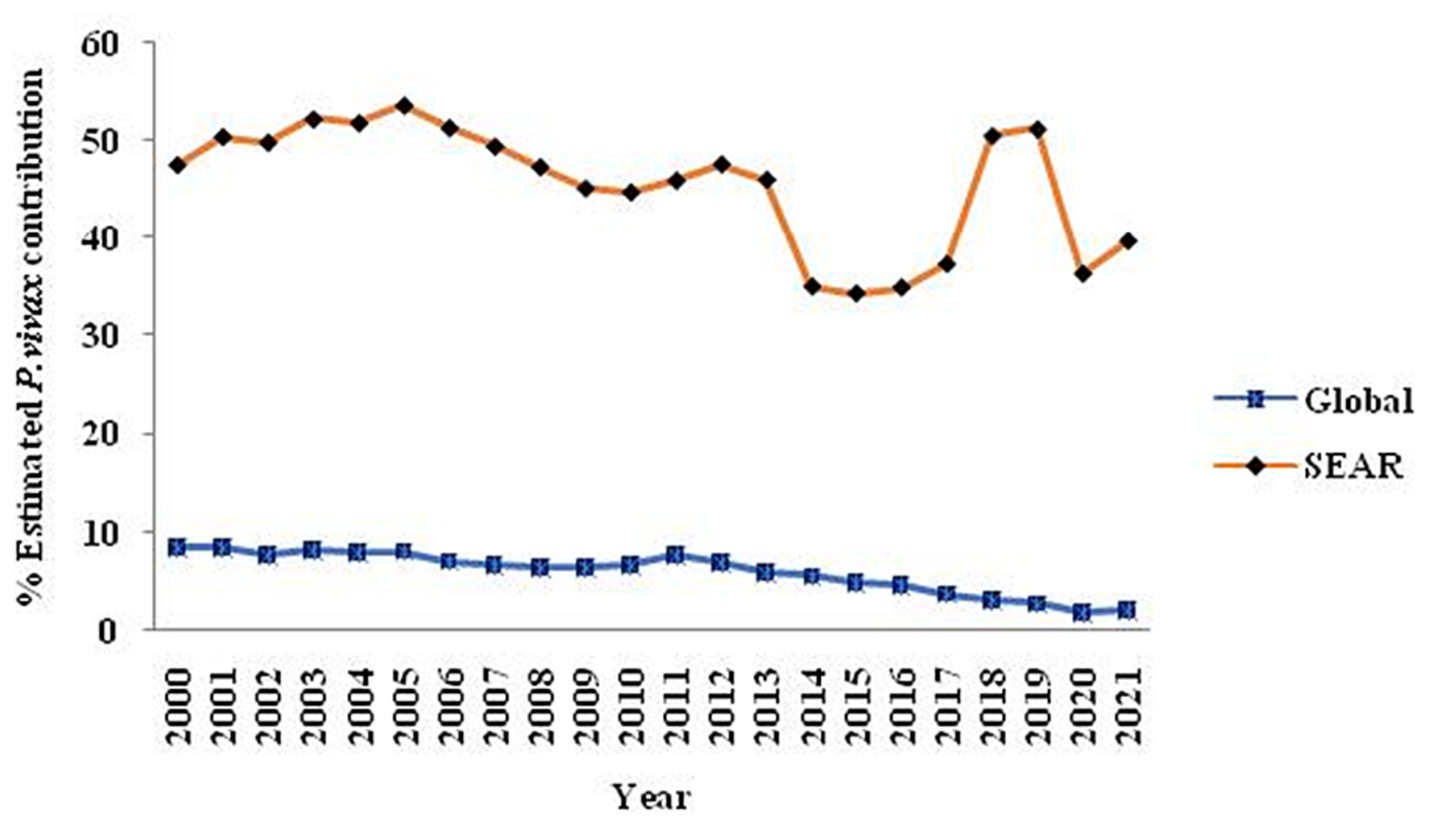
Figure 1. The trend over 20 years regarding the contribution of P. vivax globally and within the Southeast Asia Region is illustrated in a graph extracted from the WHO-2021 report, specifically on pages 23 and 28.
3 Plasmodium vivax burden in India
The two major malaria parasites in India are P. falciparum and P. vivax. P. vivax is mainly found in plains, but P. falciparum is present in woodland and bordering areas (19). India has a robust surveillance system that consistently collects and reports data. Over 100 million blood slides are examined in India from 2014–2021, making it an excellent source of data from all endemic districts. The slide positivity rate (SPR) is decreased from 0.89% in 2014 to 0.14% in 2021. The slide positive rate was higher in P. falciparum (0.09%) than P. vivax (0.05%) in the same period (Figure 2). As per the national malaria control program, vivax malaria declined to 37.3% in 2020 from 53.63% in 2019 (Figure 3). During 2021, P. vivax accounted for around ~38% (59087) of all malaria cases (158326) reported in the country (6). A surveillance-based national burden study conducted in 2015 and 2016 had estimated 1.08 million (28%) cases of P. vivax out of 3.875 million total malaria cases and 572 (3.0%) deaths out of 19,067 confirmed deaths attributed to the species in India, while the remaining cases and deaths were due to P. falciparum (20). The incidence of P. vivax and P. falciparum was almost equal nationally for nearly 15 years (1999–2014), probably due to chloroquine resistance against falciparum malaria (21). With the introduction of Artemisinin-based combination treatments (ACTs) for treatment of falciparum malaria in 2007, a decline in falciparum and overall malaria cases was observed. By 2014, the P. vivax proportion declined to 34%, with a high regional variation, which might be due to better management of both Plasmodium species. In 2014, about 380,000 P. vivax cases were recorded in India; which was approximately 16.6% of all P. vivax cases reported globally in 2014. Eleven out of 29 states in India accounted for around 95% of P. vivax cases, and of these 75% of cases were reported from seven states viz., Maharashtra, Madhya Pradesh, Jharkhand, Odisha, Gujarat, Chhattisgarh, and Uttar Pradesh. P. vivax has a much higher prevalence than P. falciparum in the urban areas of India (22) (Figure 4). Although reported incidence and mortality of malaria has declined due to the recent malaria elimination drive in India, in most states, it was largely due to the successful control of P. falciparum (23). In northeastern India, P. vivax is still a neglected infectious disease. Estimating recurrence patterns and transmission dynamics of P. vivax in different ecological settings are important prerequisites for malaria elimination programs in northeastern states (24).
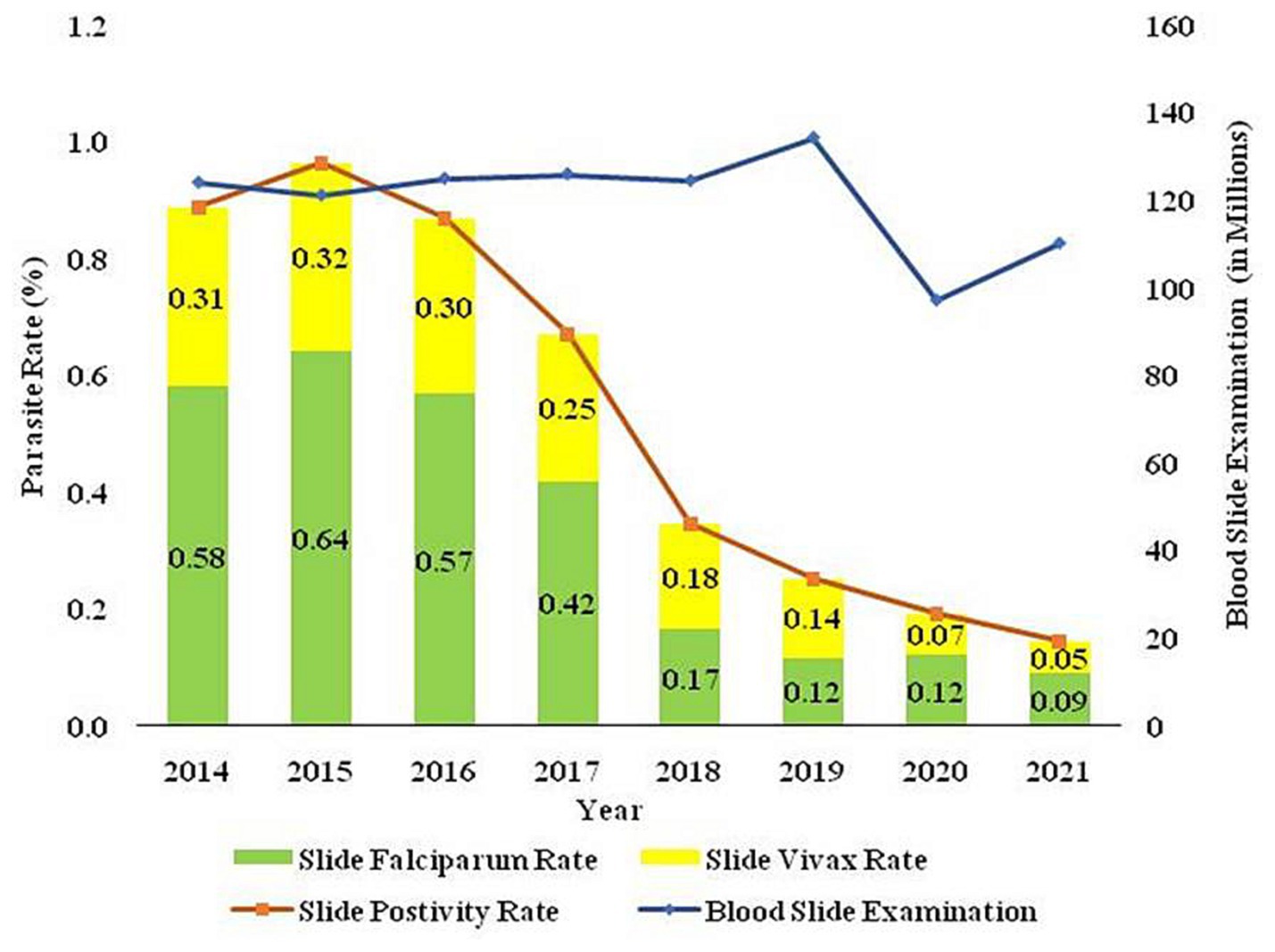
Figure 2. The trend of blood smears examined (BSE), Slide Positivity Rate (SPR), Slide falciparum rate (SFR), and Slide vivax rate (SVR) over the time in India is based on data sourced from the National Centre for Vector Borne Disease Control (NCVBDC), India.
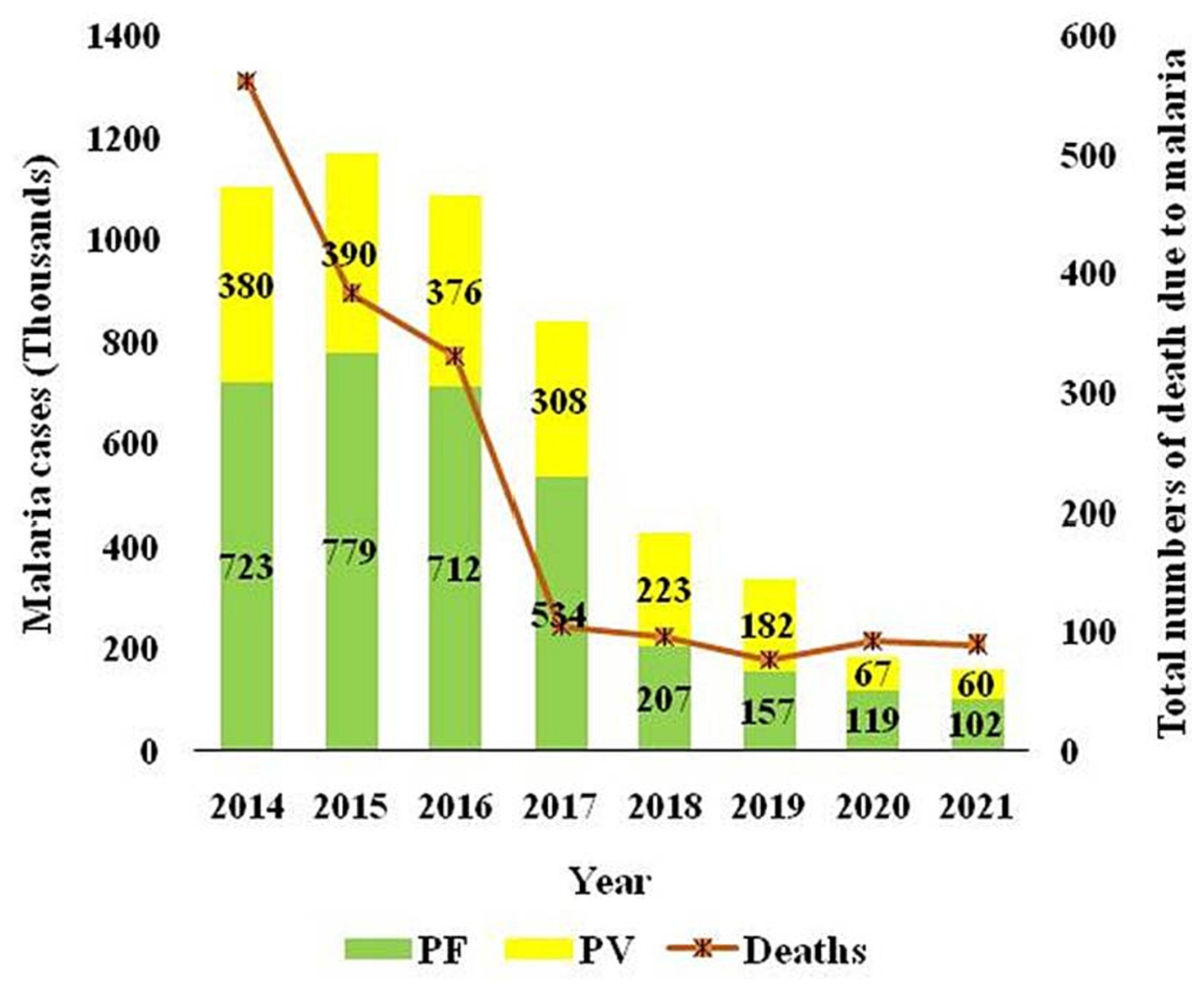
Figure 3. The trajectory of reported cases attributed to P. vivax and P. falciparum and total deaths due to malaria over the time in India is sourced from the National Centre for Vector Borne Disease Control, India.
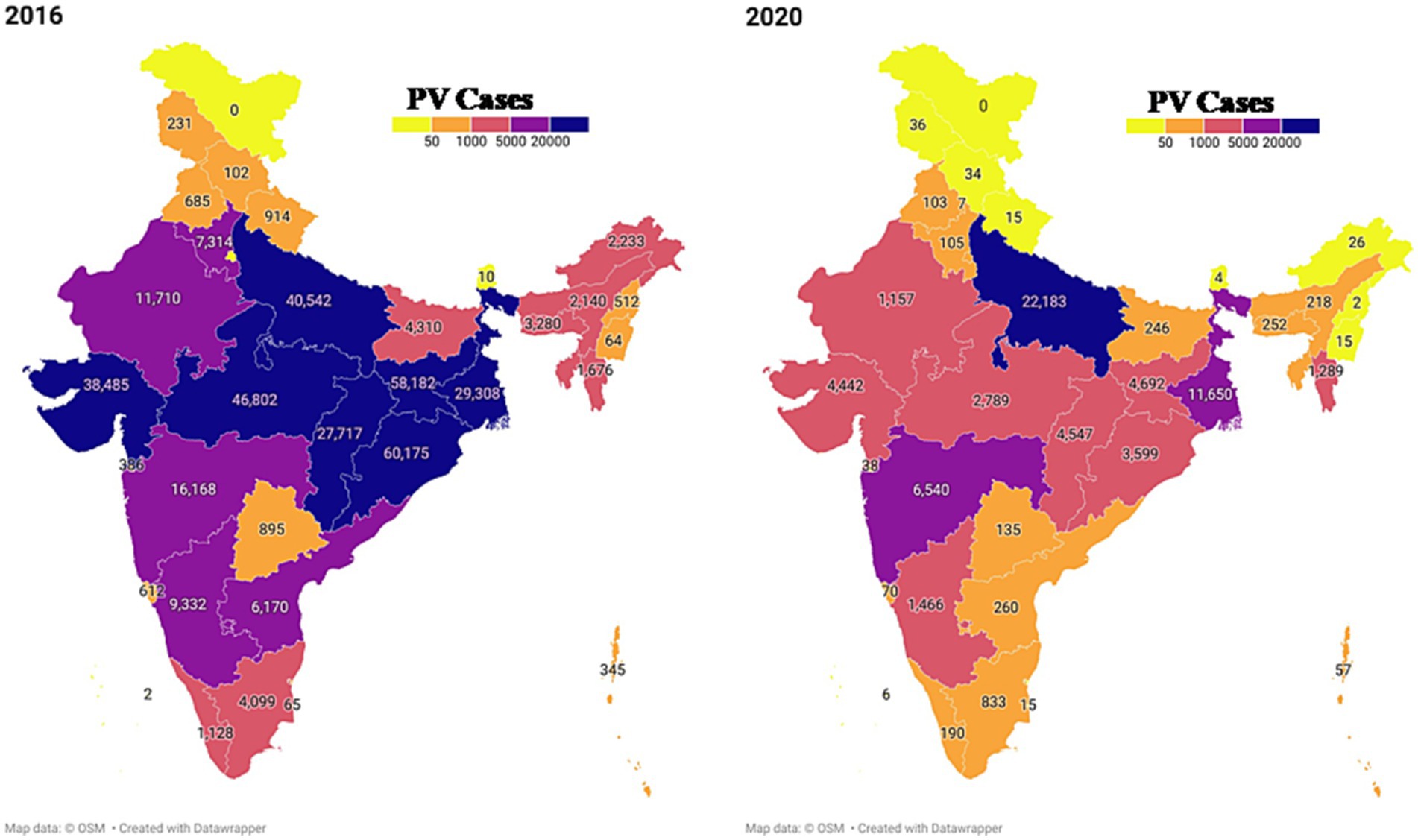
Figure 4. The geographical spread of P. vivax across India is depicted using data reported by NCVBDC for the years 2014 and 2020. The map was generated utilizing the available NCVBDC data through the data wrapper software.
4 Origin and dispersal of Plasmodium vivax
The origin and migration pattern of the malaria parasite, P. vivax, has always been a matter of debate. Understanding the origin, population migration pattern, and genetic diversity is important for improving strategies to fight against the malaria parasites and avert further spread. The routes of population migration and the origin of the parasites can be inferred through the evolutionary genomics approach adopted by evolutionary biologists (25).
The two evidence-based scenarios mainly discussed are whether this species originated in Asia or Africa. Based on 106 mitochondrial genome sequences from nine different countries, it was hypothesized that P. vivax had adapted itself, if not jumped from monkeys to humans, in South East Asia, and the species subsequently expanded in Asia and might have migrated to Africa through human population movements across the continents (25). Another study using the mitochondrial genome has revealed lesser genetic diversity in extant African P. vivax in comparison to the rest of the world and suggested a recent introduction in Africa from the Indian subcontinent (26). The Asian origin is further supported by one of the migration models utilizing 941 global mitogenomes of P. vivax (27). Recently, a study covering 28 locations in 20 countries reported the highest microsatellite genetic diversity in P. vivax populations in Southeast Asia and advocated the Asian origin of P. vivax (28).
With the discovery of P. vivax-like and several other parasites in African apes like chimpanzees and gorillas, it is now being hypothesized that P. vivax originally might have passed on from apes to humans in Africa instead of Asia (29, 30). A total of 5,000 ape faecal samples from 78 remote forest sites were screened for Plasmodium species throughout central Africa. It was an interesting discovery that the infection rates of P. vivax- like parasites were the same in the wild ape communities and in human populations, with stable parasite transmission (29). Considering that the above study was based on a few genes and partial genomes from the mitochondria, the observed phylogenetic patterns may be one-sided due to incomplete lineage sorting. This hypothesis is given further credence by the P. vivax parasite’s ability to infect Duffy-negative humans in Africa and South America (31–34). Recently, Van Dorp et al. (35) proposed an additional route of migration of P. vivax from Africa to Europe and then to South America using old blood smears of malaria patients who had malaria between 1942 and 1944 in the Ebro Delta of Spain. They have suggested utilizing historic medical collections for retrieving genomic information to infer the exact evolutionary history of the malaria parasite species.
The high genetic diversity of Asian P. vivax is an important factor to consider while suggesting the origin of the species. In addition, the phylogenetic closeness of P. vivax with fifteen Plasmodium species infecting wild Asian monkeys also needs to be considered. Recently, the discovery of the human malaria parasite, P. falciparum, in monkey species Macaca mulatta and M. Radiate (36) and non-human primates (P. coatneyi and P. fragile) specific variation reported in human P. falciparum in India (37). The non-human malaria parasite Plasmodium knowlesi has been observed in both human hosts and vectors across various regions in India, including Bihar, Delhi, Andaman & Nicobar Islands, and Uttar Pradesh, it’s crucial to base conclusions on robust scientific evidence (38–41). Additional evolutionary studies on malaria parasites of Asian monkeys will provide conclusive evidence to settle the debate on the origin of species from the academic point of view. To that effect, it is suggested to sequence the whole genome of P. vivax from worldwide populations to pinpoint the origin and dispersal pattern of the species.
5 Relapse of Plasmodium vivax
One P. vivax infective bite of a mosquito vector results in an attack within 2 weeks. It may be followed by multiple clinical episodes ranging from 2 months to 4 years after the initial infection (17). P. vivax produces dormant stages in the liver called hypnozoites, which, without any signs, become active and re-infect the bloodstream and trigger fresh episode of vivax malaria (Figure 5) which is the main setback for any malaria elimination program.
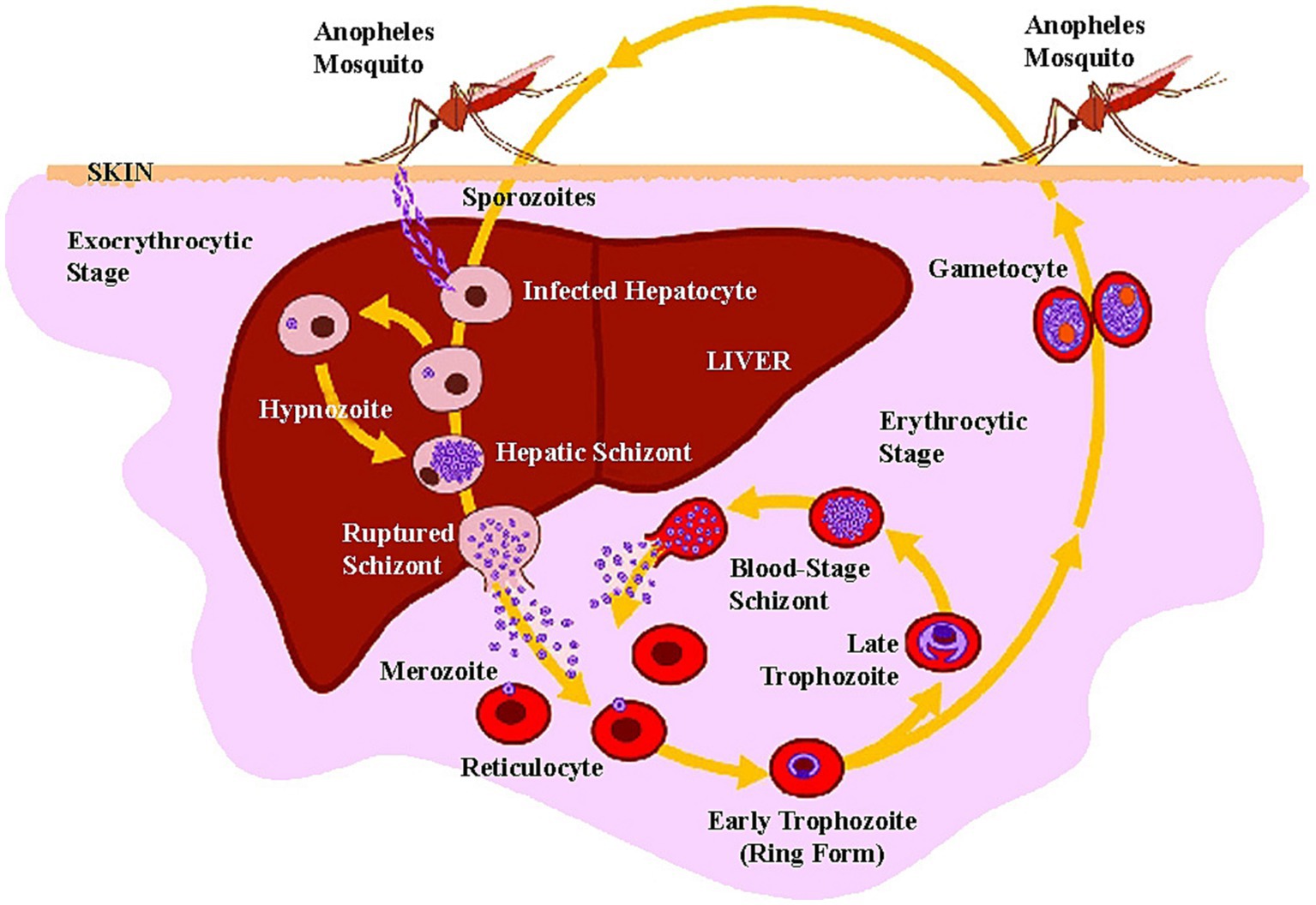
Figure 5. Life cycle of P. vivax in human host (adapted from “Major Histocompatibility Complex and Malaria: Focus on P. vivax Infection” by Lima-Junior JC and Pratt-Riccio LR (2016). Front. Immunol. 7:13. Page no-4. Copyright © 2016 Lima-Junior and Pratt-Riccio).
To target the pattern of relapse and prevent active transmission, different P. vivax strains in various parts of the world would require specific intervention strategies. Therefore, knowledge of the pattern of relapse is highly necessary to make an effective strategy for P. vivax elimination. It has been speculated that the relapse latency in different parasite populations depends on the type of strain, host biology, and climatic conditions (17). Variation in relapse patterns has been well studied in India. The strains with differing patterns of relapse coexisted, resulting in complications in transmission control measures. P. vivax populations have been identified as polymorphic for relapse in Delhi, India viz., Tropical type (those relapsing between 1 and 3 months); Sub-tropical types (relapsing between 12 and 20 weeks) and temperate type (relapsing between 24 and 28 weeks) (42, 43).
Globally, eight strains of P. vivax have been recognized (Table 1) viz., Chesson strain (with short latency and repeated relapses at short intervals), St. Elizabeth strain (long latency up to 2 years), Netherlands strain (long latency of ~8 months), North Korean strain (long latency of 8–11 months), sal 1 strain (long latency), Madagascar strain (long latency of 8–13 months), McCoy strain (long latency) and North Indian strain (long period of latency of 8–13 months) (8, 9). It should be noted that strains that relapse quickly are thought to originate in Southeast Asia. Long-latency strains are found in temperate and subtropical areas (9). Long-latency and frequently relapsing strains exist in the Indian subcontinent and South America (9). The dormancy of hypnozoites is usually longer in temperate zones, where vectors are active only for a few months during favourable weather (44).
It is still unclear what specific triggers are required for hypnozoite activation and reactivation though bacterial infections and infection with falciparum malaria are incriminated. Normal parasites and bacteriological infections can trigger hypnozoites of P. vivax (45).
6 Clinical presentation and complications
Pathogenesis of P. vivax malaria is consistent with RBC rupture in mature schizonts. In the early stages of P. vivax infection, fevers may be intermittent, and symptoms may be atypical, but if left untreated, these become intermittent (every other day) and typical. It causes the first appearance with hiccups (46), lack of fever sometimes, loss of taste, cough, pain while swallowing, and urinary discomfort (47). Infection may also lead to splenomegaly (48).
P. vivax is traditionally considered a benign species causes mild, uncomplicated, and self-limited illness (49). The public health value of controlling aggressive P. falciparum outshines P. vivax, as falciparum malaria leads to mortality due to complications and involvement of multiple organs. However, vivax malaria has also recently caused life-threatening complications, especially among children and pregnant women in endemic countries including India, Brazil, and Indonesia (49–54). Some severe malaria complications that have been reported include cerebral malaria, organ dysfunction, jaundice, acute kidney injury, thrombocytopenia, hypoglycemia, hepatic dysfunction, renal impairment, and hypotension (55–59). These observed symptoms could be due to arbitrary use of anti-malarial drugs, delayed treatment, increased resistance, and the change in the clinical spectrum of the disease (53, 55).
There are established reports that P. vivax causes severe anaemia among children in vivax-endemic countries (3, 49). It is well known that, unlike P. falciparum, the P. vivax merozoites prefer reticulocytes. The other causes of severe anaemia may be due to the release of glycosylphosphatidyl-inositol toxin in non-infected erythrocytes, the intensive haemolysis of circulating infected RBCs, other inducers of irritation such as haemozoin and dyserythropoiesis that occur due to the effect of different cytokines (60–62). A ruinous case of P. vivax malaria was reported in 2018 from the state of Goa, India. A 20-year-old otherwise healthy woman was diagnosed with vivax malaria with high parasitaemia (140,000/μl), confirmed microscopically followed by Polymerase Chain Reaction. She suffered from acute respiratory distress (ARDS) and succumbed to the so-called benign vivax malaria. Post-mortem showed congestion of alveolar capillaries, heavy monocytic infiltration and damage to the alveolar membranes consistent with ARDS (63).
7 Diagnosis of Plasmodium vivax
Currently, two diagnostic methods for malaria parasites in the point of care or clinical setting, i.e., Microscopy and rapid diagnostic tests based on lactate dehydrogenase (LDH) enzyme, are frequently used. Polymerase chain reaction (PCR) and other molecular tools are seemingly more feasible in the research facilities to confirm malaria species and to study intra-specific variations at the genomic level. Lately, their utility is also being recognized for surveillance in programmatic settings in certain scenarios like persistent malaria (64).
There are two biological characteristics of P. vivax, which make its detection very difficult. Firstly, the parasite prefers to attack young red blood cells (reticulocytes) in the blood flow resulting in lesser parasitaemia, thus requiring high microscopic abilities for accurate diagnosis. The second significant problem is the dormant liver stages of hypnozoites, when activated, can cause multiple “relapse infections” with low initial parasitaemia. When at low densities, the parasite may not be easily detectable in blood smears with light microscopy.
The age-old light microscopy for direct visualization of P. vivax parasites is hailed as the gold standard for identification of vivax malaria and calculating parasitaemia (65). Since the 1990s, point-of-care RDTs have become increasingly popular diagnostic tools (65). RDTs can detect Plasmodium antigens in the circulating blood. However, the experience shows that the sensitivity of RDT drops at parasite densities less than 500/microliter blood for P. falciparum and vivax which may produce false negative results, restricting their use in P. vivax diagnosis (66). The falciparum diagnostic protein (HRP-2) is absent in vivax malaria, which is the basis of malaria rapid detection tests (67). As a result, the RDT used for vivax identification depends on lactic acid dehydrogenase, which is less sensitive than HRP-2-based tests for falciparum malaria (68–70). However, the national program does not track malaria cases caused by P. malariae (Pm), P.ovale (Po) and P. knowlesi (Pk). Data on these non-PfPv infections are typically available only through published case reports and other studies, often as secondary outcomes (71–74). Chaturvedi et al. (41) reported that P. malariae and P.ovale infections, while less prevalent than P. vivax and P. falciparun, have been reported in India since 1930 and the 1980s, respectively. Plasmodium knowlesi infections were reported from 2004 onwards. Plasmodium malariae, in particular, is shown to persist in a single host for decades, even in the absence of symptoms, which provides an opportunity for sustained transmission. The study identifies potential hotspots for these neglected Plasmodium species, with Odisha and Bastar Chhattisgarh potentially being hotspots for Plasmodium malariae and Plasmodium ovale infections, respectively. Human Plasmodium knowlesi infections are more common in neighboring Southeast Asian countries like Malaysia, with only two studies reporting Plasmodium knowlesi infections in India. While existing anti-malarial drugs can be used to treat non-PfPv or mixed infections, the continued presence of these neglected parasites in the population, which often go undiagnosed, may pose challenges in achieving the goal of malaria elimination by 2030. Due to the lack of comprehensive data on overlooked (non-PfPv) infections in India and the pressing requirements for tailored treatments, disease prevention, an overall understanding of disease epidemiology, management of transmission dynamics, and the development of effective malaria control strategies is vital to integrate their surveillance and diagnosis within the national program. Hence, it is now required to develop improved diagnostic tools suited to low parasitaemia of non-falciparum species and probably non-LDH based (75). It is hopefully expected that the new technologies will eventually change this century-old diagnostic paradigm. However, it has been elusive despite the ongoing improvement in immuno-chromatographic strip tests. At the same time, proteomic techniques began to facilitate the identification of specific antigens of P. vivax (76). Discovering host responses, novel parasite antigens, and immune signatures using proteomic techniques can fill the diagnostic gap for P. vivax, which has proven difficult when parasitaemia is below the detection limit microscopic, and would play a vital role in global malaria elimination efforts (76). Based on serological markers and nucleic acid amplification techniques (NAATs), alternative diagnostic methods are also emerging nowadays (77). However, due to the high cost and advanced technology of NAAT-based approaches, their use in diagnosis is currently very limited.
8 Treatment
The treatment of P. vivax needs a combination of two antimalarials, a scizontocidal drug (4-aminoquinoline) to clear circulating stages and a hypnozoitocidal drug (8-aminoquinoline) for targeting hypnozoites in the liver. Chloroquine and primaquine are the only recommended drugs for treating P. vivax malaria, as per WHO guidelines. Confirmed cases of P. vivax has cured from the chloroquine with the dosage of 25 mg/kg for 3 days to remove an infection from the bloodstream, and primaquine with the dosage of 0.25 mg/kg for 14 days to cure hypnozoites, referred radical treatment. The treatment compliance of P. vivax is a challenge because of the 14 days treatment regimen with primaquine.
Primaquine is, however, contraindicated in pregnant women, infants, and persons with the deficiency of glucose-6-phosphate dehydrogenase (G6PD) (78–80). Because of the risk of foetal-haemolysis, it is strongly not recommended for pregnant women (79). This drug is also contraindicated in persons with met-haemoglobin reductase deficiency (79). Hence, individuals with G6PD deficiency must be recognized to evade drug-induced haemolysis prior to using primaquine (80). The adherence data of primaquine therapy is lacking (81). The want of access to radical treatment and poor adherence to a 14 days regimen will continuously fuel the number of P. vivax infections because of the many relapses which continue to cause P. vivax transmission. The development of drug resistance in P. vivax malaria is much less owing to its hypnozoite reservoir that maintains a pool of parasites drawn away from the bloodstream and maintained in the liver and protected from the drug effect because of their metabolic inactivity (82).
9 Improved drugs for control/elimination of Plasmodium vivax
There is a need for better drugs competent for killing parasites in all stages of Plasmodium, such as liver, blood, and mosquito. A single dose of tafenoquine, another 8-aminoquinoline, when taken with chloroquine, has been able to cure patients with P. vivax. Tafenoquine was recently approved by the United States Food and Drug Administration (FDA) for the eradication of P. vivax under the Krintafel label for individuals with G6PD activity greater than 70%. Tafenoquine has also been approved by the FDA for prophylaxis at various doses, under the Arakoda label, with a similar indication for G6PD deficiency. The single dose regime of tafenoquine, imparts an advantage in reducing non-compliance currently seen with long primaquine dosing schedules. However, tafenoquine, like primaquine is known to cause haemolysis in G6PD-deficient persons (83). Unlike primaquine, tafenoquine has a relatively long half-life of about 14 days. As a result, patients with G6PD deficiency may experience haemolysis for days (84). A companion qualitative diagnostic test is being made available with the drug to be used as a point of care diagnostic by community health workers before administering tafenoquine to the malaria positive. India can consider the deployment of drug after due regulatory considerations (85).
10 Drug resistance
Chloroquine is the primary first-line treatment for vivax malaria. The widespread resistance of vivax malaria to chloroquine in many countries in the Middle East, South Asia, Southeast Asia, East Africa and the Americas poses a challenge to control systems and control programs and highlights the critical need to develop new tools aimed specifically at treating P. vivax (86–91).
In Papua New Guinea and Indonesia, P. vivax was first reported to be resistant to chloroquine in 1989 and has since been identified in 12 countries. This resistance was reported after 30 years of recording chloroquine-resistant P. falciparum (86, 92). In 1989, the first documented instances of chloroquine-resistant P. vivax were recorded in Papua New Guinea. At that time, high-level chloroquine resistance had become prevalent in areas such as Indonesia and Oceania, which were recognized as epicenters of chloroquine resistance (92). Countries like Indonesia have shifted towards artemisinin-based combination therapies (ACTs) for treatment of P. vivax in the light of chloroquine resistance. Atovaquone-proguanil, quinine plus either tetracycline or mefloquine, or doxycycline have been proposed as therapeutic options in areas where P. vivax is resistant to chloroquine (49). It is also concerning that the new ACT medication has a lower impact on P. vivax than on P. falciparum (93). Dihydroartemisinin–piperaquine or Artemether–lumefantrine combinations are generally used, where a high level of resistance of P. vivax to chloroquine is detected (78). Therefore, there is an urgent need for revised P. vivax treatment guidelines to control and eliminate P. vivax malaria.
In India, the treatment of vivax malaria with chloroquine remains the secure and effective method (94, 95). Studies on drug efficacy are being routinely conducted in India to monitor the development of chloroquine resistance in this species (22). However, chloroquine resistance in some places was reported sporadically in the country in 2000 (96, 97). But extensive studies on therapeutic efficacy conducted regularly in India at various sites could not confirm the presence of chloroquine resistance (94, 95, 98).
11 Deficiency of glucose-6-phosphate dehydrogenase
G6PD deficiency is known to be associated with protection against malaria and related to P. vivax treatment (99). The risk–benefit ratio has changed because compared to the general population, the G6PD prevalence in the patient with infection of P. vivax patients is much lower (100). For the primaquine treatment guideline development, an evidence base of the geographical allele distribution of G6PD deficiency in P. vivax infected patients is necessary. This information on G6PD deficiency prevalence in any endemic areas provides support for the development of therapeutic strategies and screening for 8-aminoquinoline that reduce the risk of hemolysis caused by consumption of 8-aminoquinoline.
Primaquine can be given without blood testing in countries where there is essentially no G6PD deficiency, such as parts of Latin America and China. However, in India, the overall prevalence has been documented to be 1.9%, with a varied range from 0.8 to 6.3% (101). Its prevalence in the east (6.7%), central (6.1%), and north (5.8%) of India is higher than in the west (4.1%) and south (3.2%), according to a review of 224 studies in India. Scheduled tribes have the highest rate of G6PD deficiency among ethnic groups (5.5 percent) (22). In India, testing of G6PD deficiency facilities are not readily available, though incorporated as a priority for operational research under the National Framework for Malaria Elimination (19).
It is metabolized by the CYP2D6 isozyme of cytochrome P-450 (102). Individuals with exact CYP2D6 polymorphic alleles are unsuccessful in metabolizing primaquine and thus treatment may fail (102). It remains unclear as to what extent CYP2D6 polymorphisms can lead to treatment failure for parasite hypnozoites and later stages of the parasite, but the polymorphism is naturally occurring in the gene. CYP2D6 encoding leads to a wide range of metabolic activity, from above normal to none. Thus, cases of P. vivax with significant or no CYP2D6 depletion may relapse even with adequate adherence to good medication. The frequency of altered CYP2D6*10 allele (a specific genetic variant of the CYP2D6 gene) is relatively common (approximately 35%) in Southeast Asians (103). However, the distribution pattern of CYP2D6 alleles among different endemic regions for P. vivax is not fully understood. Hence, a better understanding of the CYP2D6–primaquine relationship in the different endemic areas for P. vivax will help to determine the failure rates of primaquine and to achieve better overall effectiveness of anti-relapse therapy (104).
12 Control of Plasmodium vivax
Hypnozoites impose a serious challenge to P. vivax elimination. At present, the only method of removing hypnozoites is the use of time-tested primaquine. Hypnozoite elimination was possible at the community level in large military populations where mass drug administration was enforced (105, 106). Vivax malaria elimination in large civilian populations will require some form of mass drug administration with a safe and effective drug.
India’s mosquito population is diverse, with approximately 415 species, including six major regional vectors. Anopheles culicifacies and Anopheles fluviatilis are the primary malaria vectors, responsible for 75–80% of the disease burden (107). An.culicifacies is a zoophilic species that adapts to changing ecological conditions, such as deforestation and population migration. Anopheles fluviatilis, a foothill species, shares habitat with An. culicifacies and is highly efficient in maintaining year-round malaria transmission. Anopheles minimus and Anopheles baimaii are highly efficient vectors in the eastern and northeastern regions, contributing to 5% of total cases. Anopheles stephensi, an urban vector, is found in metropolitan areas and contributes to about 10% of annual cases. Anopheles sundaicus, a brackish water species unique to the Andaman and Nicobar Islands, is primarily invasive and expanding with urbanization. Secondary vectors like An. annularis, An. subpictus, An. nivipes An. philippinensis, An. maculatus, and An. jeyporiensis also participate in malaria transmission in their areas of distribution. The increasing prevalence of insecticide-resistant malaria vectors in India has raised concerns. Anopheles culicifacies has shown resistance to all three insecticides, while other primary malaria vectors display resistance to both DDT and Malathion (108, 109). Anopheles minimus exhibits some resilience to DDT but remains susceptible to most other insecticides (110). However, there have been reports of behavioral avoidance in response to certain insecticides (111). To eliminate P. vivax malaria, vector control programs must run continuously as concerted anti-transmission measures, like mass distribution of insecticide impregnated nets (LLINs) targeting the local vectors at least for five years preferably beyond.
Moreover, P. vivax is a major public health issue in Indian cities. Malaria control in such areas is difficult due to the developmental activities, proliferation of shanties and migratory population. A separate program under the umbrella of national malaria control program namely, “Urban Malaria Scheme” covers these urban areas. The “Urban Malaria Scheme,” officially approved and sanctioned in November 1971, was dedicated to combat malaria in urban areas through a multifaceted approach (112). A fundamental element of this program involved the implementation of urban bylaws, which aimed to prevent mosquito breeding in residential blocks, government and commercial buildings, and construction sites by enforcing specific regulations. Additionally, the scheme introduced larvivorous fish into various water bodies, including streams, lakes, and ornamental ponds, where these fish effectively consumed mosquito larvae, offering a potent method for controlling mosquito populations. In areas where the use of fish was not practical, the scheme employed larvicides to eliminate mosquito larvae. Complementing these measures, awareness campaigns conducted by municipal bodies and urban area authorities played a vital role in educating and engaging the community in the collective effort to prevent and manage urban malaria. The malaria control strategies within this program include administering treatment through passive institutions such as medical facilities, hospitals, private and public sector pharmacies, and private healthcare providers. Dedicated malaria clinics are established in major urban centers, as well as in local government, railways, and defense services. In 2014, out of all the 4% of malaria cases, 12% PV cases were reported from urban areas. In the same year, P. vivax prevalence was 98 percent among all malaria cases in the Urban Malaria Scheme (112). Therefore, urban malaria schemes should be strengthened for the control of vectors resulting ultimately in the reduction of malaria cases.
13 Genetic diversity of Plasmodium vivax
Plasmodium vivax is genetically far more varied than P. falciparum, with the possibility of many clones present in a single infection, a phenomenon reported in several studies worldwide, even in areas with low-grade transmission (10, 11, 113–117). This scenario is in contrast to P. falciparum, the diversity of which is related to the regions of occurrence (118). High genetic variability in P. vivax is seen to confer it an ability to evade host immune response, increase drug resistance, and perhaps will be a critical challenge to the vaccine development program against this parasite. It also equips the species with greater adaptability to different anophelines and human hosts. Comparative genomic structure of the population of two species P. vivax and P. falciparum, showed a less structured population of P. vivax, suggesting a higher occurrence of gene flow between different geographic areas (11, 117). The main cause of the higher diversity of P. vivax could be due to relapse, which provides greater potential for multiple infections (113, 115). Immunity to P. vivax develops faster than P. falciparum. Studies are warranted to understand the relationship between genetic and antigenic variability crucial for the vaccine development program against P. vivax. Genetic diversity results from cross-mating between different co-existing strains of the parasite which, when passed on from the mosquito to the human hosts, are further propagated (10). Further, gametocyte specific pvs25 gene transcripts of P. vivax can be detected with molecular techniques (119). Almost 2,800 genes have been characterized, exclusive to P. vivax, possibly as a result of duplication within the P. vivax lineage providing it with the advantages mentioned earlier (120). Moreover, P. vivax is more antigenically conserved. The dynamics of polymorphism in P. vivax parasites need to be studied in order to understand its immune responses and evasion strategies.
14 Potential vaccine
In addition to other drugs that target the dormant stage of the liver, the development of vaccines against vivax malaria is of particular importance. A vaccine will protect against clinical illness, reduce transmission and potentially target each stage of the P. vivax life cycle. A vaccine against Plasmodium vivax (P. vivax) holds the potential to significantly reduce malaria transmission by targeting the liver-stage of the parasite, thereby lowering the parasite reservoir in humans and the risk of transmission to mosquitoes. This vaccine can work in conjunction with other malaria control measures, provide long-term protection, break the cycle of recurring infections, reduce the healthcare burden, and offer sustained immunity. It aligns with global health security goals and can yield economic benefits such as increased productivity and reduced healthcare costs. However, developing and deploying a P. vivax vaccine, especially in the context of India’s goal for malaria elimination by 2030, faces numerous challenges.The challenges in developing a P. vivax vaccine arise from the parasite’s high genetic diversity and complex life cycle. Potential vaccine candidates are categorized into three groups: preerythrocytic (PEVs), blood-stage (BVs), and transmission-blocking vaccines (TBV).
PEVs target sporozoite antigens and aim to prevent hepatocyte invasion and the development of hypnozoites. Promising candidates include circumsporozoite surface protein (CSP), thrombospondin-related anonymous protein (TRAP), and PvCelTOS. A multigenic vaccine combining PvCSP and PvTRAP has shown potential in animal models and is moving towards clinical trials (121, 122).
BVs focus on the asexual blood stage to alleviate malaria symptoms and reduce parasitemia and transmission. Key antigens include PvDBP-II (which interacts with the DARC/Duffy receptor), PvRBP-2b (which targets the TfR1/CD78 receptor), PvAMA-1, and the PvMSPfamily, which are involved in the invasion process. Others were recently described, such as GAMA, PvTRAg (which targets the Band 3 receptor or Basigin/CD147 receptor, such as TRAg-38), MAELB, ETRAMP, RON2 and EBP-2. They are expressed on the surface, microneme or rhoptry of the parasite and inhibit parasite invasion of reticulocytes (123–125).
TBVs target the sexual stages of the parasite in both the mosquito’s midgut and blood. Candidates such as Pvs25, Pvs28, Pvs45, Pvs48, and Pvs230 aim to block transmission by preventing the maturation of sexual phases in the mosquito host (126–130).
The Malaria Vaccine Technology Roadmap, developed by the world’s leading funders for malaria vaccine development, has envisaged the development of vaccines with protective efficacy of at least 75% against clinical malaria against both P. falciparum and P. vivax to reduce transmission of human malaria and accelerate malaria elimination globally by 2030 (131).
15 Challenges for Plasmodium vivax malaria elimination
• Diagnosis: The low density of P. vivax in the blood-stage are common (132, 133), making laboratory diagnosis particularly difficult. Since P. vivax usually infects reticulocytes and restricts parasite densities, diagnostic tests with high sensitivity are needed. Skilled microscopy is relied upon for blood smear-based diagnosis, but its use may be challenging in resource-poor settings. It has been observed that in certain areas, P. vivax infections, around 70%, were found to be below the detection limit by microscopy, posing an additional challenge to elimination efforts (133). Furthermore, sub-microscopic infections found in all settings seem to have greater comparative importance in low-incidence areas for achieving and maintaining transmission elimination.
• Presence of hypnozoite stage: P. vivax has a dormant hypnozoite stage in the liver, and limited treatment options are currently available. Despite scientific advancements, technology to recognize or detect the presence of hypnozoite is lacking (134).
• G6PD deficiency: Additionally, primaquine drug, which targets hypnozoites can potentially cause haemolysis in individuals who are G6PD deficient. Thus, testing for G6PD deficiency is desirable before the administration of primaquine (135). The testing facilities are not rampantly available in Indian public health settings. The 14 days treatment course of primaquine, with uncertain compliance and prevalence of G6PD deficient cases with the extensive genetic disorder, could cause severe haemolyticanaemia in approximately 8% of the people in a malaria endemic country (136).
• Genetic diversity and resistance to chloroquine: The failure of primaquine against latent P. vivax in individuals with damaged P450 2D6 alleles increases the substantial pool of primaquine ineligibles (137). When there is an acute natural polymorphism in the gene encoding CYP2D6, it results in various metabolic activities that confer chloroquine resistance (138). Also, acute regular polymorphisms in the CYP2D6 gene result in different metabolic activities that confer chloroquine resistance. As a result, many Asians may not be capable to fully metabolise the primaquine and achieve successful radical treatment (139). Alternative therapies such as ACTs are rarely assessed as a companion drug to primaquine in the crucial radical cure where P. vivax malaria is common. Hence alternate anti-relapse modalities and enabling strategies are needed to overcome this limitation to achieve P. vivax elimination.
• Outdoor transmission: Several vectors of P. vivax species exist in South-East Asia and South Asia, where >90% of infections occur. They flourish in diverse habitats, with adults preferring to rest indoors and outdoors and demonstrate a broad range of breeding and feeding behavior (140). Insecticide-impregnated bed nets and indoor residual spraying have been the primary malaria interventions. While bed nets and IRS provide high levels of protection indoors, no cost-effective, practicable, or scalable vector control techniques have been identified to provide similar levels of protection against outdoor biting mosquitoes.
16 Conclusion
There have been major gains against malaria, including P. vivax, globally over the last decade. However, there exist significant challenges for control of P. vivax, and its elimination may not be achievable with current malaria control tools. This parasite species has adapted to several vector species across varied ecologically diverse habitats and regions. P. vivax has a complex biology; it is difficult to detect the low parasite densities in infected individuals, the survival of hypnozoites causes relapses with polymorphism, and the appearance of gametocytes early in the course of infection all of which aid in infection transmission. Better P. vivax diagnostic tools are needed, particularly point-of-care rapid diagnostic tools with high sensitivity and specificity. Highly sensitive tests for detection of asymptomatic infection of P. vivax, and reliable tests for G6PD deficiency prior to dosing primaquine, may aid in better control and eventually elimination. New drugs that do not cause haemolysis are needed to replace primaquine, and anti-relapse therapeutics with shorter treatment regimens for better compliance, would greatly aid elimination efforts. As the world looks forward to malaria elimination, an effective surveillance system should be in place for monitoring, evaluation and evidence generation as the key interventions are rolled out and program are metamorphosed from control to achieve elimination goals. A multistage vaccine to prevent P. vivax infection and transmission would be a welcome development to achieve and sustain the elimination of malaria.
Author contributions
PPS and AK majorly drafted and edited different versions of the manuscript. SS, KH, ST, MR, and PPS significantly contributed with graphics, literature, provided technical inputs to the manuscripts for improvement. All authors contributed to the article and approved the submitted version.
Acknowledgments
Authors are thankful to Indian Council of Medical Research, New Delhi for the facilities.
Conflict of interest
The authors declare that the research was conducted in the absence of any commercial or financial relationships that could be construed as a potential conflict of interest.
Publisher’s note
All claims expressed in this article are solely those of the authors and do not necessarily represent those of their affiliated organizations, or those of the publisher, the editors and the reviewers. Any product that may be evaluated in this article, or claim that may be made by its manufacturer, is not guaranteed or endorsed by the publisher.
References
1. Douglas, NM, John, GK, von Seidlein, L, Anstey, NM, and Price, RN. Chemotherapeutic strategies for reducing transmission of plasmodium vivax malaria. Adv Parasitol. (2012) 80:271–300. doi: 10.1016/B978-0-12-397900-1.00005-0
2. Tajebe, A, Magoma, G, Aemero, M, and Kimani, F. Detection of mixed infection level of plasmodium falciparum and plasmodium vivax by SYBR Green I-based real-time PCR in North Gondar, north-West Ethiopia. Malar J. (2014) 13:411. doi: 10.1186/1475-2875-13-411
3. Genton, B, D'Acremont, V, Rare, L, Baea, K, Reeder, JC, Alpers, MP, et al. Plasmodium vivax and mixed infections are associated with severe malaria in children: a prospective cohort study from Papua New Guinea. PLoS Med. (2008) 5:e127. doi: 10.1371/journal.pmed.0050127
4. Battle, KE, Lucas, TCD, Nguyen, M, Howes, RE, Nandi, AK, Twohig, KA, et al. Mapping the global endemicity and clinical burden of plasmodium vivax, 2000–17: a spatial and temporal modelling study. Lancet. (2019) 394:332–43. doi: 10.1016/S0140-6736(19)31096-7
6. Malaria situation in India from 2014. (2014). Available at: https://nvbdcp.gov.in/index4.php?lang=1&level=0&linkid=564&lid=3867 (Accessed Apr 20, 2022).
7. Malaria situation in India from 2018. (2020). Available at: https://nvbdcp.gov.in/WriteReadData/l892s/41539703001661429664.pdf (Accessed Apr 20, 2022).
8. White, NJ. Determinants of relapse periodicity in plasmodium vivax malaria. Malar J. (2011) 10:297. doi: 10.1186/1475-2875-10-297
9. Battle, KE, Karhunen, MS, Bhatt, S, Gething, PW, Howes, RE, Golding, N, et al. Geographical variation in plasmodium vivax relapse. Malar J. (2014) 13:144. doi: 10.1186/1475-2875-13-144
10. Jennison, C, Arnott, A, Tessier, N, Tavul, L, Koepfli, C, Felger, I, et al. Plasmodium vivax populations are more genetically diverse and less structured than sympatric plasmodium falciparum populations. PLoS Negl Trop Dis. (2015) 9:e0003634. doi: 10.1371/journal.pntd.0003634
11. Neafsey, DE, Galinsky, K, Jiang, RH, Young, L, Sykes, SM, Saif, S, et al. The malaria parasite plasmodium vivax exhibits greater genetic diversity than plasmodium falciparum. Nat Genet. (2012) 44:1046–50. doi: 10.1038/ng.2373
12. Mueller, I, Galinski, MR, Baird, JK, Carlton, JM, Kochar, DK, Alonso, PL, et al. Key gaps in the knowledge of plasmodium vivax, a neglected human malaria parasite. Lancet Infect Dis. (2009) 9:555–66. doi: 10.1016/S1473-3099(09)70177-X
13. Gething, PW, Van Boeckel, TP, Smith, DL, Guerra, CA, Patil, AP, Snow, RW, et al. Modelling the global constraints of temperature on transmission of plasmodium falciparum and P. vivax. Parasit Vectors. (2011) 4:92. doi: 10.1186/1756-3305-4-92
14. Dayananda, KK, Achur, RN, and Gowda, DC. Epidemiology, drug resistance, and pathophysiology of plasmodium vivax malaria. J Vector Borne Dis. (2018) 55:1–8. doi: 10.4103/0972-9062.234620
15. Ding, XC, Ade, MP, Baird, JK, Cheng, Q, Cunningham, J, Dhorda, M, et al. Defining the next generation of plasmodium vivax diagnostic tests for control and elimination: target product profiles. PLoS Negl Trop Dis. (2017) 11:e0005516. doi: 10.1371/journal.pntd.0005516
16. Feachem, RGA. Shrinking the Malaria Map: a Guide on Malaria Elimination for Policy Makers. San Francisco: The Global Health Group, University of California, San Francisco. (2009).
17. Howes, RE, Battle, KE, Mendis, KN, Smith, DL, Cibulskis, RE, Baird, JK, et al. Global epidemiology of plasmodium vivax. Am J Trop Med Hyg. (2016) 95:15–34. doi: 10.4269/ajtmh.16-0141
18. World Health Organization. World malaria report 2021. Geneva: World Health Organization (2021). 2021 p.
19. National Framework for Malaria Elimination. 2016 – 2030, Directorate of National Vector Borne diseases, Directorate General of Health Services, Ministry of Health & Family Welfare Government of India. NVBDCP (2016).
20. Kumar, A, Chaturvedi, HK, Mohanty, AK, Sharma, SK, Malhotra, MS, and Pandey, A. Surveillance based estimation of burden of malaria in India, 2015–2016. Malar J. (2020) 19:156. doi: 10.1186/s12936-020-03223-7
22. Anvikar, AR, Shah, N, Dhariwal, AC, Sonal, GS, Pradhan, MM, Ghosh, SK, et al. Epidemiology of plasmodium vivax malaria in India. Am J Trop Med Hyg. (2016) 95:108–20. doi: 10.4269/ajtmh.16-0163
23. WHO. Malaria: factsheet 2022. Available at: https://www.who.int/news-room/fact-sheets/detail/malaria
24. Sharma, VP, Dev, V, and Phookan, S. Neglected plasmodium vivax malaria in northeastern states of India. Indian J Med Res. (2015) 141:546–55. doi: 10.4103/0971-5916.159511
25. Jongwutiwes, S, Putaporntip, C, Iwasaki, T, Ferreira, MU, Kanbara, H, and Hughes, AL. Mitochondrial genome sequences support ancient population expansion in plasmodium vivax. Mol Biol Evol. (2005) 22:1733–9. doi: 10.1093/molbev/msi168
26. Culleton, R, Coban, C, Zeyrek, FY, Cravo, P, Kaneko, A, Randrianarivelojosia, M, et al. The origins of African plasmodium vivax; insights from mitochondrial genome sequencing. PLoS One. (2011) 6:e29137. doi: 10.1371/journal.pone.0029137
27. Rodrigues, PT, Valdivia, HO, de Oliveira, TC, Alves, JMP, Duarte, A, Cerutti-Junior, C, et al. Human migration and the spread of malaria parasites to the New World. Sci Rep. (2018) 8:1993. doi: 10.1038/s41598-018-19554-0
28. Rougeron, V, Elguero, E, Arnathau, C, Acuna Hidalgo, B, Durand, P, Houze, S, et al. Human plasmodium vivax diversity, population structure and evolutionary origin. PLoS Negl Trop Dis. (2020) 14:e0008072. doi: 10.1371/journal.pntd.0008072
29. Liu, W, Li, Y, Shaw, KS, Learn, GH, Plenderleith, LJ, Malenke, JA, et al. African origin of the malaria parasite plasmodium vivax. Nat Commun. (2014) 5:3346. doi: 10.1038/ncomms4346
30. Loy, DE, Liu, W, Li, Y, Learn, GH, Plenderleith, LJ, Sundararaman, SA, et al. Out of Africa: origins and evolution of the human malaria parasites plasmodium falciparum and plasmodium vivax. Int J Parasitol. (2017) 47:87–97. doi: 10.1016/j.ijpara.2016.05.008
31. Abdelraheem, MH, Albsheer, MM, Mohamed, HS, Amin, M, and Mahdi Abdel Hamid, M. Transmission of plasmodium vivax in Duffy-negative individuals in Central Sudan. Trans R Soc Trop Med Hyg. (2016) 110:258–60. doi: 10.1093/trstmh/trw014
32. Carvalho, TA, Queiroz, MG, Cardoso, GL, Diniz, IG, Silva, AN, Pinto, AY, et al. Plasmodium vivax infection in Anajas, state of Para: no differential resistance profile among Duffy-negative and Duffy-positive individuals. Malar J. (2012) 11:430. doi: 10.1186/1475-2875-11-430
33. Menard, D, Barnadas, C, Bouchier, C, Henry-Halldin, C, Gray, LR, Ratsimbasoa, A, et al. Plasmodium vivax clinical malaria is commonly observed in Duffy-negative Malagasy people. Proc Natl Acad Sci U S A. (2010) 107:5967–71. doi: 10.1073/pnas.0912496107
34. Woldearegai, TG, Kremsner, PG, Kun, JF, and Mordmuller, B. Plasmodium vivax malaria in Duffy-negative individuals from Ethiopia. Trans R Soc Trop Med Hyg. (2013) 107:328–31. doi: 10.1093/trstmh/trt016
35. van Dorp, L, Gelabert, P, Rieux, A, de Manuel, M, De-Dios, T, Gopalakrishnan, S, et al. Plasmodium vivax malaria viewed through the Lens of an eradicated European strain. Mol Biol Evol. (2020) 37:773–85. doi: 10.1093/molbev/msz264
36. Dixit, J, Zachariah, APKS, Chandramohan, B, Shanmuganatham, V, and Karanth, KP. Reinvestigating the status of malaria parasite (plasmodium sp.) in Indian non-human primates. PLoS Negl Trop Dis. (2018) 12:e0006801. doi: 10.1371/journal.pntd.0006801
37. Tyagi, S, Pande, V, and Das, A. New insights into the evolutionary history of plasmodium falciparum from mitochondrial genome sequence analyses of Indian isolates. Mol Ecol. (2014) 23:2975–87. doi: 10.1111/mec.12800
38. Singh, S, Prakash, A, Yadav, RN, Mohapatra, PK, Sarma, NP, Sarma, DK, et al. Anopheles (Cellia) maculatus group: its spatial distribution and molecular characterization of member species in north-East India. Acta Trop. (2012) 124:62–70. doi: 10.1016/j.actatropica.2012.06.011
39. Tyagi, RK, Das, MK, Singh, SS, and Sharma, YD. Discordance in drug resistance-associated mutation patterns in marker genes of plasmodium falciparum and plasmodium knowlesi during coinfections. J Antimicrob Chemother. (2013) 68:1081–8. doi: 10.1093/jac/dks508
40. Vidhya, PT, Sunish, IP, Maile, A, and Zahid, AK. Anopheles sundaicus mosquitoes as vector for plasmodium knowlesi, Andaman and Nicobar Islands, India. Emerg Infect Dis. (2019) 25:817–20. doi: 10.3201/eid2504.181668
41. Chaturvedi, R, Deora, N, Bhandari, D, Parvez, S, Sinha, A, and Sharma, A. Trends of neglected plasmodium species infection in humans over the past century in India. One Health. (2021) 11:100190. doi: 10.1016/j.onehlt.2020.100190
42. Adak, T, Sharma, VP, and Orlov, VS. Studies on the plasmodium vivax relapse pattern in Delhi, India. Am J Trop Med Hyg. (1998) 59:175–9. doi: 10.4269/ajtmh.1998.59.175
43. Adak, T, Valecha, N, and Sharma, VP. Plasmodium vivax polymorphism in a clinical drug trial. Clin Diagn Lab Immunol. (2001) 8:891–4. doi: 10.1128/CDLI.8.5.891-894.2001
45. Shanks, GD, and White, NJ. The activation of vivax malaria hypnozoites by infectious diseases. Lancet Infect Dis. (2013) 13:900–6. doi: 10.1016/S1473-3099(13)70095-1
46. Guadarrama-Conzuelo, F, and Saad Manzanera, AD. Singultus as an unusual debut of plasmodium vivax malaria. Cureus. (2019) 11:e5548. doi: 10.7759/cureus.5548
47. Mohapatra, M. K., Padhiary, K. N., Mishra, D. P., and Sethy, G.. “Atypical manifestations of plasmodium vivax malaria”. Indian Journal of Malariology. (2002) 39:18–25.
48. Anderson, C. Pathogenic properties (virulence factors) of some common pathogens. (2010). https://faculty.mtsac.edu/canderson/pathogenic%20properties%20list%20.pdf
49. Tjitra, E, Anstey, NM, Sugiarto, P, Warikar, N, Kenangalem, E, Karyana, M, et al. Multidrug-resistant plasmodium vivax associated with severe and fatal malaria: a prospective study in Papua, Indonesia. PLoS Med. (2008) 5:e128. doi: 10.1371/journal.pmed.0050128
50. Alexandre, MA, Ferreira, CO, Siqueira, AM, Magalhaes, BL, Mourao, MP, Lacerda, MV, et al. Severe Plasmodium vivax malaria, Brazilian Amazon. Emerg Infect Dis. (2010) 16:1611–4. doi: 10.3201/eid1610.100685
51. Chaudhari, KS, Uttarwar, SP, Tambe, NN, Sharma, RS, and Takalkar, AA. Role of serum lactate and malarial retinopathy in prognosis and outcome of falciparum and vivax cerebral malaria: a prospective cohort study in adult Assamese tribes. J Global Infect Dis. (2016) 8:61–7. doi: 10.4103/0974-777X.177524
52. Chauhan, V, Raina, SK, and Thakur, S. State of the globe: the resurgence of vivax. J Global Infect Dis. (2016) 8:59–60. doi: 10.4103/0974-777X.182113
53. Gupta, A, Dhume, V, Puranik, GV, and Kavishwar, V. Autopsy study of febrile deaths during monsoon at a tertiary care institute in India: is malaria still a challenge? Niger Med J. (2015) 56:6–11. doi: 10.4103/0300-1652.149163
54. Kochar, DK, Das, A, Kochar, SK, Saxena, V, Sirohi, P, Garg, S, et al. Severe plasmodium vivax malaria: a report on serial cases from Bikaner in northwestern India. Am J Trop Med Hyg. (2009) 80:194–8. doi: 10.4269/ajtmh.2009.80.194
55. Kute, VB, Trivedi, HL, Vanikar, AV, Shah, PR, Gumber, MR, Patel, HV, et al. Plasmodium vivax malaria-associated acute kidney injury, India, 2010–2011. Emerg Infect Dis. (2012) 18:842–5. doi: 10.3201/eid1805.111442
56. Mahgoub, H, Gasim, GI, Musa, IR, and Adam, I. Severe plasmodium vivax malaria among sudanese children at new Halfa hospital, Eastern Sudan. Parasit Vectors. (2012) 5:154. doi: 10.1186/1756-3305-5-154
57. Manning, L, Laman, M, Law, I, Bona, C, Aipit, S, Teine, D, et al. Features and prognosis of severe malaria caused by plasmodium falciparum, plasmodium vivax and mixed plasmodium species in Papua new Guinean children. PLoS One. (2011) 6:e29203. doi: 10.1371/journal.pone.0029203
58. Osonuga, OAOA, Osonuga, IO, Osonuga, A, and Derkyi, KL. Prevalence of hypoglycemia among severe malaria children in a rural African population. Asian Pac J Trop Dis. (2011) 1:192–4. doi: 10.1016/S2222-1808(11)60026-1
59. Tanwar, GS, Khatri, PC, Sengar, GS, Kochar, A, Kochar, SK, Middha, S, et al. Clinical profiles of 13 children with plasmodium vivax cerebral malaria. Ann Trop Paediatr. (2011) 31:351–6. doi: 10.1179/1465328111Y.0000000040
60. Brattig, NW, Kowalsky, K, Liu, X, Burchard, GD, Kamena, F, and Seeberger, PH. Plasmodium falciparum glycosylphosphatidylinositol toxin interacts with the membrane of non-parasitized red blood cells: a putative mechanism contributing to malaria anemia. Microbes Infect. (2008) 10:885–91. doi: 10.1016/j.micinf.2008.05.002
61. Chasis, JA, and Mohandas, N. Erythroblastic islands: niches for erythropoiesis. Blood. (2008) 112:470–8. doi: 10.1182/blood-2008-03-077883
62. Haldar, K, Murphy, SC, Milner, DA, and Taylor, TE. Malaria: mechanisms of erythrocytic infection and pathological correlates of severe disease. Annu Rev Pathol. (2007) 2:217–49. doi: 10.1146/annurev.pathol.2.010506.091913
63. Valecha, N, Pinto, RG, Turner, GD, Kumar, A, Rodrigues, S, Dubhashi, NG, et al. Histopathology of fatal respiratory distress caused by plasmodium vivax malaria. Am J Trop Med Hyg. (2009) 81:758–62. doi: 10.4269/ajtmh.2009.09-0348
64. Rahi, M, Sharma, R, Saroha, P, Chaturvedi, R, Bharti, PK, and Sharma, A. Polymerase chain reaction-based malaria diagnosis can be increasingly adopted during current phase of malaria elimination in India. Am J Trop Med Hyg. (2022) 106:1005–12. doi: 10.4269/ajtmh.21-0966
65. Boyce, MR, and O'Meara, WP. Use of malaria RDTs in various health contexts across sub-Saharan Africa: a systematic review. BMC Public Health. (2017) 17:470. doi: 10.1186/s12889-017-4398-1
66. Abba, K, Kirkham, AJ, Olliaro, PL, Deeks, JJ, Donegan, S, Garner, P, et al. Rapid diagnostic tests for diagnosing uncomplicated non-falciparum or plasmodium vivax malaria in endemic countries. Cochrane Database Syst Rev. (2014) 2014:CD011431. doi: 10.1002/14651858.CD011431
67. Ly, AB, Tall, A, Perry, R, Baril, L, Badiane, A, Faye, J, et al. Use of HRP-2-based rapid diagnostic test for plasmodium falciparum malaria: assessing accuracy and cost-effectiveness in the villages of Dielmo and Ndiop, Senegal. Malar J. (2010) 9:153. doi: 10.1186/1475-2875-9-153
68. Ashley, EA, Touabi, M, Ahrer, M, Hutagalung, R, Htun, K, Luchavez, J, et al. Evaluation of three parasite lactate dehydrogenase-based rapid diagnostic tests for the diagnosis of falciparum and vivax malaria. Malar J. (2009) 8:241. doi: 10.1186/1475-2875-8-241
69. Ashton, RA, Kefyalew, T, Tesfaye, G, Counihan, H, Yadeta, D, Cundill, B, et al. Performance of three multi-species rapid diagnostic tests for diagnosis of plasmodium falciparum and plasmodium vivax malaria in Oromia regional state, Ethiopia. Malar J. (2010) 9:297. doi: 10.1186/1475-2875-9-297
70. Senn, N, Rarau, P, Manong, D, Salib, M, Siba, P, Robinson, LJ, et al. Rapid diagnostic test-based management of malaria: an effectiveness study in Papua new Guinean infants with plasmodium falciparum and plasmodium vivax malaria. Clin Infect Dis. (2012) 54:644–51. doi: 10.1093/cid/cir901
71. Singh, R, Jain, V, Singh, PP, Bharti, PK, Thomas, T, Basak, S, et al. First report of detection and molecular confirmation of plasmodium ovale from severe malaria cases in Central India. Tropical Med Int Health. (2013) 18:1416–20. doi: 10.1111/tmi.12184
72. Chaturvedi, N, Bhandari, S, Bharti, PK, Basak, SK, Singh, MP, and Singh, N. Sympatric distribution of plasmodium ovale curtisi and P. ovale wallikeri in India: implication for the diagnosis of malaria and its control. Trans R Soc Trop Med Hyg. (2015) 109:352–4. doi: 10.1093/trstmh/trv015
73. Singh, K, Bharti, PK, Devi, NC, Ahmed, N, and Sharma, A. Plasmodium malariae detected by microscopy in the international bordering area of Mizoram, a northeastern state of India. Diagnostics (Basel). (2022) 12:2015. doi: 10.3390/diagnostics12082015
74. Sharma, SK, Tyagi, PK, Padhan, K, Upadhyay, AK, Haque, MA, Nanda, N, et al. Epidemiology of malaria transmission in forest and plain ecotype villages in Sundargarh District, Orissa, India. Trans R Soc Trop Med Hyg. (2006) 100:917–25. doi: 10.1016/j.trstmh.2006.01.007
75. Harris, I, Sharrock, WW, Bain, LM, Gray, KA, Bobogare, A, Boaz, L, et al. A large proportion of asymptomatic plasmodium infections with low and sub-microscopic parasite densities in the low transmission setting of Temotu Province, Solomon Islands: challenges for malaria diagnostics in an elimination setting. Malar J. (2010) 9:254. doi: 10.1186/1475-2875-9-254
76. Patankar, S, Sharma, S, Rathod, PK, and Duraisingh, MT. Malaria in India: the need for new targets for diagnosis and detection of plasmodium vivax. Proteomics Clin Appl. (2018) 12:e1700024. doi: 10.1002/prca.201700024
77. World Health Organization.. WHO policy recommendation on malaria diagnostics in low transmission settings. World Health Organization. (2014). https://iris.who.int/handle/10665/338357
78. Baird, JK, and Hoffman, SL. Primaquine therapy for malaria. Clin Infect Dis. (2004) 39:1336–45. doi: 10.1086/424663
79. Hill, DR, Baird, JK, Parise, ME, Lewis, LS, Ryan, ET, and Magill, AJ. Primaquine: report from CDC expert meeting on malaria chemoprophylaxis I. Am J Trop Med Hyg. (2006) 75:402–15. doi: 10.4269/ajtmh.2006.75.402
80. Recht, J, Ashley, EA, and White, NJ. Use of primaquine and glucose-6-phosphate dehydrogenase deficiency testing: divergent policies and practices in malaria endemic countries. PLoS Negl Trop Dis. (2018) 12:e0006230. doi: 10.1371/journal.pntd.0006230
81. Barua, N, and Pandav, CS. The allure of the private practitioner: is this the only alternative for the urban poor in India? Indian J Public Health. (2011) 55:107–14. doi: 10.4103/0019-557X.85242
83. Shanks, GD, Oloo, AJ, Aleman, GM, Ohrt, C, Klotz, FW, Braitman, D, et al. A new primaquine analogue, tafenoquine (WR 238605), for prophylaxis against plasmodium falciparum malaria. Clin Infect Dis. (2001) 33:1968–74. doi: 10.1086/324081
84. WHO. New opportunities to prevent plasmodium vivax malaria relapse. (2019). https://www.who.int/news/item/25-02-2019-new-opportunities-to-prevent-p-vivax malariarelapse#:~:text=For%20the%20treatment%20of%20P,a%20full%202%2Dweek%20period
85. Ahmad, SS, Rahi, M, and Sharma, A. Relapses of plasmodium vivax malaria threaten disease elimination: time to deploy tafenoquine in India? BMJ Glob Health. (2021) 6:e004558. doi: 10.1136/bmjgh-2020-004558
86. Baird, JK, Basri, H, Purnomo,, Bangs, MJ, Subianto, B, Patchen, LC, et al. Resistance to chloroquine by plasmodium vivax in Irian Jaya, Indonesia. Am J Trop Med Hyg. (1991) 44:547–52. doi: 10.4269/ajtmh.1991.44.547
87. Ketema, T, Bacha, K, Birhanu, T, and Petros, B. Chloroquine-resistant plasmodium vivax malaria in Serbo town, Jimma zone, south-West Ethiopia. Malar J. (2009) 8:177. doi: 10.1186/1475-2875-8-177
88. Mohan, K, and Maithani, MM. Congenital malaria due to chloroquine-resistant Plasmodium vivax: a case report. J Trop Pediatr. (2010) 56:454–5. doi: 10.1093/tropej/fmq025
89. Rijken, MJ, Boel, ME, Russell, B, Imwong, M, Leimanis, ML, Phyo, AP, et al. Chloroquine resistant vivax malaria in a pregnant woman on the western border of Thailand. Malar J. (2011) 10:113. doi: 10.1186/1475-2875-10-113
90. Ruebush, TK 2nd, Zegarra, J, Cairo, J, Andersen, EM, Green, M, Pillai, DR, et al. Chloroquine-resistant Plasmodium vivax malaria in Peru. Am J Trop Med Hyg. (2003) 69:548–52. doi: 10.4269/ajtmh.2003.69.548
91. Teka, H, Petros, B, Yamuah, L, Tesfaye, G, Elhassan, I, Muchohi, S, et al. Chloroquine-resistant Plasmodium vivax malaria in Debre Zeit, Ethiopia. Malar J. (2008) 7:220. doi: 10.1186/1475-2875-7-220
92. Rieckmann, KH, Davis, DR, and Hutton, DC. Plasmodium vivax resistance to chloroquine? Lancet. (1989) 334:1183–4. doi: 10.1016/S0140-6736(89)91792-3
93. Carrara, VI, Sirilak, S, Thonglairuam, J, Rojanawatsirivet, C, Proux, S, Gilbos, V, et al. Deployment of early diagnosis and mefloquine-artesunate treatment of falciparum malaria in Thailand: the Tak malaria initiative. PLoS Med. (2006) 3:e183. doi: 10.1371/journal.pmed.0030183
94. Mishra, N, Singh, JP, Srivastava, B, Arora, U, Shah, NK, Ghosh, SK, et al. Monitoring antimalarial drug resistance in India via sentinel sites: outcomes and risk factors for treatment failure, 2009–2010. Bull World Health Organ. (2012) 90:895–904. doi: 10.2471/BLT.12.109124
95. Mishra, N, Singh, JP, Srivastava, B, Arora, U, Shah, NK, Ghosh, SK, et al. Monitoring antimalarial drug resistance in India via sentinel sites: outcomes and risk factors for treatment failure, 2009-2010. Bull World Health Organ. (2012) 90:895–904.
96. Singh, RK. Emergence of chloroquine-resistant vivax malaria in South Bihar (India). Trans R Soc Trop Med Hyg. (2000) 94:327. doi: 10.1016/S0035-9203(00)90344-4
97. Srivastava, HC, Yadav, RS, Joshi, H, Valecha, N, Mallick, PK, Prajapati, SK, et al. Therapeutic responses of plasmodium vivax and P. Falciparum to chloroquine, in an area of western India where P. vivax predominates. Ann Trop Med Parasitol. (2008) 102:471–80. doi: 10.1179/136485908X311759
98. Saravu, K, Kumar, R, Ashok, H, Kundapura, P, Kamath, V, Kamath, A, et al. Therapeutic assessment of chloroquine-Primaquine combined regimen in adult cohort of plasmodium vivax malaria from primary care Centres in southwestern India. PLoS One. (2016) 11:e0157666. doi: 10.1371/journal.pone.0157666
99. Louicharoen, C, Patin, E, Paul, R, Nuchprayoon, I, Witoonpanich, B, Peerapittayamongkol, C, et al. Positively selected G6PD-Mahidol mutation reduces plasmodium vivax density in southeast Asians. Science. (2009) 326:1546–9. doi: 10.1126/science.1178849
100. Leslie, T, Briceno, M, Mayan, I, Mohammed, N, Klinkenberg, E, Sibley, CH, et al. The impact of phenotypic and genotypic G6PD deficiency on risk of plasmodium vivax infection: a case-control study amongst afghan refugees in Pakistan. PLoS Med. (2010) 7:e1000283. doi: 10.1371/journal.pmed.1000283
101. Devendra, R, Gupta, V, Shanmugam, R, Singh, M, Patel, P, Valecha, N, et al. Prevalence and spectrum of mutations causing G6PD deficiency in Indian populations. Infect Genet Evol. (2020) 86:104597. doi: 10.1016/j.meegid.2020.104597
102. Bennett, JW, Pybus, BS, Yadava, A, Tosh, D, Sousa, JC, McCarthy, WF, et al. Primaquine failure and cytochrome P-450 2D6 in plasmodium vivax malaria. N Engl J Med. (2013) 369:1381–2. doi: 10.1056/NEJMc1301936
103. Sistonen, J, Fuselli, S, Palo, JU, Chauhan, N, Padh, H, and Sajantila, A. Pharmacogenetic variation at CYP2C9, CYP2C19, and CYP2D6 at global and microgeographic scales. Pharmacogenet Genomics. (2009) 19:170–9. doi: 10.1097/FPC.0b013e32831ebb30
104. Rahi, M, and Sharma, A. Malaria control initiatives that have the potential to be gamechangers in India's quest for malaria elimination. Lancet Reg Health Southeast Asia. (2022) 2:100009. doi: 10.1016/j.lansea.2022.04.005
105. Hill, E, and Amatuzio, DS. Southwest Pacific vivax malaria; clinical features and observations concerning duration of clinical activity. Am J Trop Med Hyg. (1949) s1-29:203–14. doi: 10.4269/ajtmh.1949.s1-29.203
106. Kaneko, A. A community-directed strategy for sustainable malaria elimination on islands: short-term MDA integrated with ITNs and robust surveillance. Acta Trop. (2010) 114:177–83. doi: 10.1016/j.actatropica.2010.01.012
107. Subbarao, SK, Nanda, N, Rahi, M, and Raghavendra, K. Biology and bionomics of malaria vectors in India: existing information and what more needs to be known for strategizing elimination of malaria. Malar J. (2019) 18:396. doi: 10.1186/s12936-019-3011-8
108. Raghavendra, K, Rahi, M, Verma, V, Velamuri, PS, Kamaraju, D, Baruah, K, et al. Insecticide resistance status of malaria vectors in the malaria endemic states of India: implications and way forward for malaria elimination. Heliyon. (2022) 8:e11902. doi: 10.1016/j.heliyon.2022.e11902
109. Raghavendra, K, Velamuri, PS, Verma, V, Elamathi, N, Barik, TK, Bhatt, RM, et al. Temporo-spatial distribution of insecticide-resistance in Indian malaria vectors in the last quarter-century: Need for regular resistance monitoring and management. J Vector Borne Dis. (2017) 54:111–30.
110. Das, MK, Rahi, M, Dhiman, RC, and Raghavendra, K. Insecticide susceptibility status of malaria vectors, Anopheles culicifacies, Anopheles fluviatilis and Anopheles minimus in the tribal districts of Jharkhand state of India. J Vector Borne Dis. (2021) 58:374–82. doi: 10.4103/0972-9062.325641
111. Jacob, M, Riveron, MT, Mugenzi, L, Menze, BD, Chiang, M-C, and Wondji, CS In: D SMaV, editor. Insecticide resistance in malaria vectors: an update at a global scale. United Kingdom: IntechOpen Limited (2018)
112. Urban Malaria Scheme (UMS). (2015). Available at: https://nvbdcp.gov.in/index4.php?lang=1&level=0&linkid=529&lid=3822 (Accessed September 03, 2022).
113. Chan, CW, Sakihama, N, Tachibana, S, Idris, ZM, Lum, JK, Tanabe, K, et al. Plasmodium vivax and plasmodium falciparum at the crossroads of exchange among islands in Vanuatu: implications for malaria elimination strategies. PLoS One. (2015) 10:e0119475. doi: 10.1371/journal.pone.0119475
114. Ferreira, MU, Karunaweera, ND, da Silva-Nunes, M, da Silva, NS, Wirth, DF, and Hartl, DL. Population structure and transmission dynamics of plasmodium vivax in rural Amazonia. J Infect Dis. (2007) 195:1218–26. doi: 10.1086/512685
115. Hupalo, DN, Luo, Z, Melnikov, A, Sutton, PL, Rogov, P, Escalante, A, et al. Population genomics studies identify signatures of global dispersal and drug resistance in plasmodium vivax. Nat Genet. (2016) 48:953–8. doi: 10.1038/ng.3588
116. Koepfli, C, Ross, A, Kiniboro, B, Smith, TA, Zimmerman, PA, Siba, P, et al. Multiplicity and diversity of plasmodium vivax infections in a highly endemic region in Papua New Guinea. PLoS Negl Trop Dis. (2011) 5:e1424. doi: 10.1371/journal.pntd.0001424
117. Orjuela-Sanchez, P, Sa, JM, Brandi, MC, Rodrigues, PT, Bastos, MS, Amaratunga, C, et al. Higher microsatellite diversity in plasmodium vivax than in sympatric plasmodium falciparum populations in Pursat, Western Cambodia. Exp Parasitol. (2013) 134:318–26. doi: 10.1016/j.exppara.2013.03.029
118. Barry, AE, Waltmann, A, Koepfli, C, Barnadas, C, and Mueller, I. Uncovering the transmission dynamics of plasmodium vivax using population genetics. Pathog Glob Health. (2015) 109:142–52. doi: 10.1179/2047773215Y.0000000012
119. Lima, NF, Bastos, MS, and Ferreira, MU. Plasmodium vivax: reverse transcriptase real-time PCR for gametocyte detection and quantitation in clinical samples. Exp Parasitol. (2012) 132:348–54. doi: 10.1016/j.exppara.2012.08.010
120. Cornejo, OE, Fisher, D, and Escalante, AA. Genome-wide patterns of genetic polymorphism and signatures of selection in plasmodium vivax. Genome Biol Evol. (2014) 7:106–19. doi: 10.1093/gbe/evu267
121. Bennett, JW, Yadava, A, Tosh, D, Sattabongkot, J, Komisar, J, Ware, LA, et al. Phase 1/2a trial of plasmodium vivax malaria vaccine candidate VMP001/AS01B in malaria-naive adults: safety, immunogenicity, and efficacy. PLoS Negl Trop Dis. (2016) 10:e0004423. doi: 10.1371/journal.pntd.0004423
122. Atcheson, E, Bauza, K, Salman, AM, Alves, E, Blight, J, Viveros-Sandoval, ME, et al. Tailoring a plasmodium vivax vaccine to enhance efficacy through a combination of a CSP virus-like particle and TRAP viral vectors. Infect Immun. (2018) 86:e00114-18. doi: 10.1128/IAI.00114-18
123. King, CL, Michon, P, Shakri, AR, Marcotty, A, Stanisic, D, Zimmerman, PA, et al. Naturally acquired Duffy-binding protein-specific binding inhibitory antibodies confer protection from blood-stage plasmodium vivax infection. Proc Natl Acad Sci U S A. (2008) 105:8363–8. doi: 10.1073/pnas.0800371105
124. Payne, RO, Silk, SE, Elias, SC, Milne, KH, Rawlinson, TA, Llewellyn, D, et al. Human vaccination against plasmodium vivax Duffy-binding protein induces strain-transcending antibodies. JCI. Insight. (2017) 2:e93683. doi: 10.1172/jci.insight.93683
125. Franca, CT, White, MT, He, WQ, Hostetler, JB, Brewster, J, Frato, G, et al. Identification of highly-protective combinations of plasmodium vivax recombinant proteins for vaccine development. elife. (2017) 6:6. doi: 10.7554/eLife.28673
126. Malkin, EM, Durbin, AP, Diemert, DJ, Sattabongkot, J, Wu, Y, Miura, K, et al. Phase 1 vaccine trial of Pvs25H: a transmission blocking vaccine for plasmodium vivax malaria. Vaccine. (2005) 23:3131–8. doi: 10.1016/j.vaccine.2004.12.019
127. Wu, Y, Ellis, RD, Shaffer, D, Fontes, E, Malkin, EM, Mahanty, S, et al. Phase 1 trial of malaria transmission blocking vaccine candidates Pfs25 and Pvs25 formulated with montanide ISA 51. PLoS One. (2008) 3:e2636. doi: 10.1371/journal.pone.0002636
128. MacDonald, NJ, Nguyen, V, Shimp, R, Reiter, K, Herrera, R, Burkhardt, M, et al. Structural and immunological characterization of recombinant 6-cysteine domains of the plasmodium falciparum sexual stage protein Pfs230. J Biol Chem. (2016) 291:19913–22. doi: 10.1074/jbc.M116.732305
129. Scaria, PV, Anderson, C, Muratova, O, Alani, N, Trinh, HV, Nadakal, ST, et al. Malaria transmission-blocking conjugate vaccine in ALFQ adjuvant induces durable functional immune responses in rhesus macaques. NPJ Vaccines. (2021) 6:148. doi: 10.1038/s41541-021-00407-3
130. Arevalo-Herrera, M, Miura, K, Cespedes, N, Echeverry, C, Solano, E, Castellanos, A, et al. Immunoreactivity of sera from low to moderate malaria-endemic areas against plasmodium vivax rPvs48/45 proteins produced in Escherichia coli and Chinese Hamster ovary systems. Front Immunol. (2021) 12:634738. doi: 10.3389/fimmu.2021.634738
131. Moorthy, VS, Newman, RD, and Okwo-Bele, JM. Malaria vaccine technology roadmap. Lancet. (2013) 382:1700–1. doi: 10.1016/S0140-6736(13)62238-2
132. Barbosa, S, Gozze, AB, Lima, NF, Batista, CL, Bastos Mda, S, Nicolete, VC, et al. Epidemiology of disappearing plasmodium vivax malaria: a case study in rural Amazonia. PLoS Negl Trop Dis. (2014) 8:e3109. doi: 10.1371/journal.pntd.0003109
133. Cheng, Q, Cunningham, J, and Gatton, ML. Systematic review of sub-microscopic P. vivax infections: prevalence and determining factors. PLoS Negl Trop Dis. (2015) 9:e3413. doi: 10.1371/journal.pntd.0003413
134. White, NJ, and Imwong, M. Relapse. Adv Parasitol. (2012) 80:113–50. doi: 10.1016/B978-0-12-397900-1.00002-5
135. von Seidlein, L, Auburn, S, Espino, F, Shanks, D, Cheng, Q, McCarthy, J, et al. Review of key knowledge gaps in glucose-6-phosphate dehydrogenase deficiency detection with regard to the safe clinical deployment of 8-aminoquinoline treatment regimens: a workshop report. Malar J. (2013) 12:112. doi: 10.1186/1475-2875-12-112
136. Howes, RE, Piel, FB, Patil, AP, Nyangiri, OA, Gething, PW, Dewi, M, et al. G6PD deficiency prevalence and estimates of affected populations in malaria endemic countries: a geostatistical model-based map. PLoS Med. (2012) 9:e1001339. doi: 10.1371/journal.pmed.1001339
137. Marcsisin, SR, Reichard, G, and Pybus, BS. Primaquine pharmacology in the context of CYP 2D6 pharmacogenomics: current state of the art. Pharmacol Ther. (2016) 161:1–10. doi: 10.1016/j.pharmthera.2016.03.011
138. Price, RN, Douglas, NM, and Anstey, NM. New developments in plasmodium vivax malaria: severe disease and the rise of chloroquine resistance. Curr Opin Infect Dis. (2009) 22:430–5. doi: 10.1097/QCO.0b013e32832f14c1
139. Baird, JK, Battle, KE, and Howes, RE. Primaquine ineligibility in anti-relapse therapy of plasmodium vivax malaria: the problem of G6PD deficiency and cytochrome P-450 2D6 polymorphisms. Malar J. (2018) 17:42. doi: 10.1186/s12936-018-2190-z
Keywords: Plasmodium vivax, relapse, hypnozoites, glucose-6-phosphate dehydrogenase, primaquine, vaccine, elimination
Citation: Kumar A, Singh PP, Tyagi S, Hari Kishan Raju K, Sahu SS and Rahi M (2024) Vivax malaria: a possible stumbling block for malaria elimination in India. Front. Public Health. 11:1228217. doi: 10.3389/fpubh.2023.1228217
Edited by:
Kinley Wangdi, The University of Queensland, AustraliaReviewed by:
Joseli Oliveira Ferreira, Oswaldo Cruz Foundation (Fiocruz), BrazilChetan Chitnis, Institut Pasteur, France
Copyright © 2024 Kumar, Singh, Tyagi, Hari Kishan Raju, Sahu and Rahi. This is an open-access article distributed under the terms of the Creative Commons Attribution License (CC BY). The use, distribution or reproduction in other forums is permitted, provided the original author(s) and the copyright owner(s) are credited and that the original publication in this journal is cited, in accordance with accepted academic practice. No use, distribution or reproduction is permitted which does not comply with these terms.
*Correspondence: Puspendra Pal Singh, cHVzaHBpaXRyQGdtYWlsLmNvbQ==; Manju Rahi, ZHJtYW5qdXJhaGlAZ21haWwuY29t