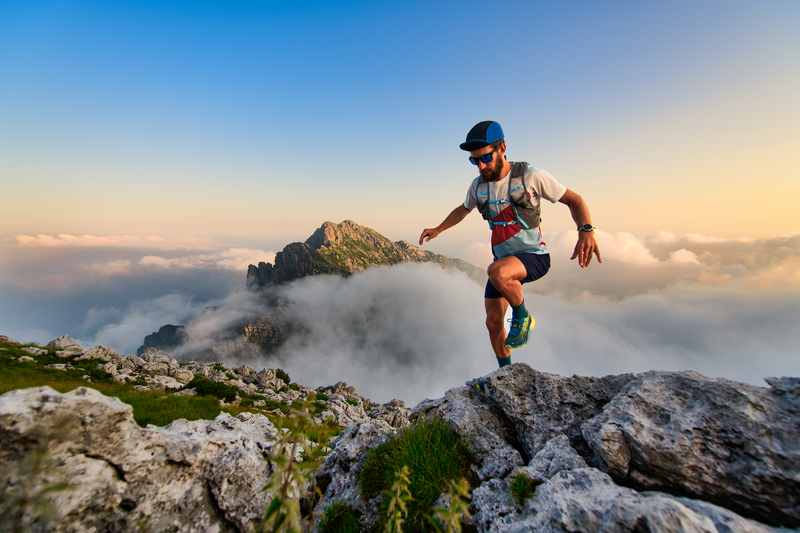
95% of researchers rate our articles as excellent or good
Learn more about the work of our research integrity team to safeguard the quality of each article we publish.
Find out more
REVIEW article
Front. Public Health , 24 February 2023
Sec. Environmental Health and Exposome
Volume 11 - 2023 | https://doi.org/10.3389/fpubh.2023.1140786
This article is part of the Research Topic Dietary Exposures to Environmental Pollutants: Integrated Multimedia Perspectives View all 16 articles
Inflammatory bowel disease (IBD) is becoming increasingly prevalent with the improvement of people's living standards in recent years, especially in urban areas. The emerging environmental contaminant is a newly-proposed concept in the progress of industrialization and modernization, referring to synthetic chemicals that were not noticed or researched before, which may lead to many chronic diseases, including IBD. The emerging contaminants mainly include microplastics, endocrine-disrupting chemicals, chemical herbicides, heavy metals, and persisting organic pollutants. In this review, we summarize the adverse health effect of these emerging contaminants on humans and their relationships with IBD. Therefore, we can better understand the impact of these new emerging contaminants on IBD, minimize their exposures, and lower the future incidence of IBD.
Inflammatory bowel disease (IBD) refers to chronic, relapsing inflammatory disorders of the gastrointestinal tract, and its pathogenesis includes heredity and environmental factors (1). The two major types of IBD are Crohn's disease (CD) and ulcerative colitis (UC) (2). The pathology of IBD involves impairment of the intestinal mucosal barrier, dysbiosis of the gut microenvironment, and the alteration of the gut immune response (3). Chronic abdominal pain and diarrhea are typical symptoms of IBD. Presently no effective treatments can fully cure IBD, and nearly 30% of IBD patients will require surgery within 10 years after their initial diagnosis (4, 5). The global spread of IBD appears to be associated with industrialization and changes in people's diets and environments, and environmental exposures are closely associated with the increased risk of IBD (6).
The new emerging environmental contaminant is a recently coined term that describes exposome to the environment. The emerging environmental contaminants including but are not limited to microplastics (MPs), endocrine-disrupting chemicals (EDCs), chemical herbicides, heavy metals, and persisting organic pollutants (POPs) (7, 8). The variable composition of these exposomes across regions, and the interaction among these exposures may contribute to the heterogeneous nature of the association between emerging environmental contaminants and IBD (9). These exposomes are not commonly monitored in nature, but have the potential to enter the environment and human body, and cause short-term and long-term adverse health effects. In the immediate dietary intake, the contaminants may cause acute abdominal pain or diarrhea, activating immediate intestinal inflammation (Figure 1). As in long-term exposure, these contaminants will cause chronic diseases like IBD and chronic renal failure, activate a series of chronic inflammation.
Figure 1. The common way and pollutants that general population in exposure of Emerging Contaminants (ECs) through digestive tract, respiratory tract and skin contact.
In this review, we summarize the current epidemiologic evidence and biological mechanism between new emerging contaminants exposure and the development of IBD. Also, we summarize the common exposure pathways of new emerging contaminants to public generations based on accumulated studies.
Microplastics (MPs) are tiny plastic particles under 5 millimeters in size (10). The primary sources of MPs in human life are plastic bottles, abrasives, and opacifiers (11), and they may be degraded into MPs by various factors like ultraviolet over time (12). The main types of MPs include polyethylene (PE), polypropylene (PP), polystyrene (PS), polyethylene terephthalate (PET), polyvinyl chloride (PVC), Polyurethane (PU), etc. (10, 13) (Table 1).
Human exposures to MPs occur through ingestion, inhalation, and dermal contact (21). MPs exist and exposit in all environments, especially underground and surface water, then the human food chain, and ultimately enter the body through ingestion, which is the major exposure way (11, 13, 22, 23). A recent review has summarized multiple types of food containing MPs, including fruit, vegetables, milk, meat, aquatic food, etc. (12, 24). Besides, the fast spread of takeaway foods accelerates the number of MPs ingested by humans globally, since they are usually packed with plastic products (12). The suggested weekly ingestion range of MPs is within 0.10–5 g/week (5, 7, 8). In a study of a small set of donors, which first measured the mass concentration of the polymetric component of plastic in human blood, the mean of the sum quantifiable concentration of plastic particles in blood was 1.6 μg/ml (25). In human feces, MPs were also detected in the order of 2 MP particles/g (5).
MPs could be detected in the human bloodstream probably due to the absorption of them into the blood by mucosal contact (either ingestion or inhalation) in a size-dependent manner (25). MPs may be transported to organs via the bloodstream, causing intestinal toxicity, metabolic disruption, reproductive toxicity, neurotoxicity, immunotoxicity through oxidative stress, apoptosis, and specific pathways, etc. (26). After dietary intake, MPs are usually absorbed in the digestive tract, liver, kidneys, and spleen (27–31). Once deposited, MPs will induce morphological changes, activate inflammatory responses, inhibit cell differentiation, and affect gene expression (27, 32–34). Several clinical trials found that exposure to MPs impairs the gut epithelial barrier, induces intestinal flora alteration, disturbs lipid metabolism, causes oxidative stress and the release of inflammatory factors (15, 35–37). Apart from passive intake, the intestinal tract absorbs MPs through multiple ways, including endocytosis of enterocytes and specialized M cells, paracellular uptake, and active absorption by the intestinal villus (38).
The increased exposure to MPs will also accelerate the pathogenesis of IBD. A recent study revealed that exposure to MPs may impair the antimicrobial capacity of blood clam by reducing plasma inhibition of bacterial growth, humoral immune effector levels, and chemotactic activity of hemocytes, which showed that MPs imposed significant oxidative stress on hemocytes, causing great immunotoxicity (39).
Researchers found that the average concentrations of MPs in the feces of IBD patients (41.8 items/g dm) were markedly higher than that of healthy people (28.0 items/g dm) (40). In IBD patients, the gut experiences repeating endothelial lesions, which increases the permeability of the intestinal epithelial barrier (41). This means in IBD patients, MPs are more likely to enter the injured intestinal epithelial cells, attach to them, and enhance their translocation to different systems (14, 42). MPs exposure also triggers the over-proliferation of intestinal stem cells, causing the imbalance of colonic epithelial homeostasis, which in turn elevates the occurrence and severity of DSS-induced colitis (43).
MPs will also alter the composition and diversity of intestinal microflora in animal models, which will trigger multiple follow-up effects, such as changing the ability in the differentiation of Treg cells, and activating the signal transduction pathways associated with intestinal mucosal immune function (17, 18, 44–46). In the DSS mouse model, additional exposure to polystyrene microplastics (PS-MPs) can aggravate the severity of colitis (14, 15) by reducing tight junction proteins such as Claudin1 and Occludin, and increasing intestinal permeability (47–49). Correspondingly, TNF-α, IL-1β, and IL-6 increase significantly in the colitis mice exposed to additional PS-MPs, demonstrating their proinflammatory properties (15, 16). After the exposure, the macrophage infiltration will increase in the liver, which further triggers immune cells to release proinflammatory and immune factors like IL-17α and IL-22 (16).
MPs can alter the structure of intestinal microbiota in mice, which may exacerbate intestinal inflammation. Previous studies showed the overgrowth of Staphylococci is related to IBD. Correspondingly, mice treated with MPs exhibited a marked increase in Staphylococcus genus abundance and a decrease in Parabacteroides genus abundance (P < 0.05) (18). Li et al. observed increased numbers of gut microbial species, bacterial abundance, and flora diversity in mice treated with a high concentration of MPs (50). An increased abundance of Staphylococcus and Bacteroidetes alongside a decreased abundance of Parabacteroides and Firmicutes are also documented in this research (18). Likewise, Lu et al. found that MPs exposure decreased the abundances of Firmicutes and α-Proteobacteria in the feces of mice, and altered the variety and diversity of gut microbes (17).
To sum up, exposure to MPs, either in the blood or digestive tract, may accelerate the occurrence and development of IBD, and cause serious harm to the human body. Therefore, it is necessary to reduce the amount of dietary intake of MPs, such as reducing the use of bottled water and takeaway food (12, 23). The government also needs to pay attention to more efficient ways to degrade MPs. The potential benefits of reducing the pollution of MPs at sources still deserve further exploration.
Endocrine disruptors (EDCs) are chemicals that interfere with the hormones in the human body through the endocrine system (51). EDCs can affect the way the body reacts to hormones, and change the gut microenvironment, which may cause immune vulnerability, decreased tolerance to food antigens (52), change in gut microbiota, and the occurrence of IBD (53).
EDCs, including phthalates, flame retardants, pharmaceutical agents, and phenols like bisphenol-A (BPA), ethyl-paraben, and methylparaben (54), are massively produced and used for food containers, personal care products, and other plastic objects (51). EDCs enter the human body mainly through dietary ingestion, inhalation, and dermal uptake, and are mostly bioaccumulated in the adipose tissue (55, 56). Most EDCs are lipophilic; therefore, they can induce microbial dysbiosis, and activate xenobiotic pathways and associated genes, enzymes, and metabolites (Table 2).
BPA is the most common and well-known EDC. It is linked with the active type of IBD. The direct intake of BPA-packaged food rarely causes an immediate intestinal response, as it mainly affects humans through chronic exposure and accumulation. BPA level is significantly increased in the serum of patients with the active period of IBD, compared to patients in the remission period, and regarding the disease phenotype, serum BPA levels were higher in colonic vs. ileal forms (62). It can change the level of microbiota and gut metabolites, increase the incidence of IBD, and accelerate IBD development (57, 59, 62).
BPA may change the metabolic way of gut microbiota and increase the risk of IBD. Many bacteria, such as Bacteroides, Mollicutes, Prevotellaceae, Erysipelotrichaceae, Akkermansia, Methanobrevibacter, and Sutterella, whose proportions increase with exposure to BPA, are associated with different diseases, such as IBD and colorectal cancer (58). Another American research shows BPA can affect the gut microbial composition in an age- and sex-dependent manner (61). In the epidemiological studies, dietary exposure to BPA reduces gut microbial diversity, and in the gut microbiota of CD patients, the population of Proteobacteria increased while Clostridium decreased (59). In the plasma of UC patients, the amount of BPA exposure is observed to have correlation with the altered level of plasma proteins involved in lipid-related metabolic processes and cytokine response, indicating BPA may serve as a biomarker in severe UC (63). BPA exposure may also change the level of gut metabolites, reducing butyric acid and tryptophan, and increasing fecal calprotectin, which indicates a correlation between its exposure and the severity of IBD (57, 62).
Aside from BPA and its analogs, many other EDCs also relate to the development of IBD, such as phthalates, triclosan, and perfluoroalkyl substances (64–66). For example, exposure to the BPA substitute, fluorene-9-bisphenol, can alter gut metabolites in mice and deregulates the sugar and fatty acid metabolisms in the gut (60). Several epidemiological studies have demonstrated the correlation between other EDCs and IBD (62, 66, 67). In a study estimating paraben and phenol exposure, approximately one quarter (25.5%) of all participants in this sub-set reported symptoms of chronic diarrhea, which is a typical complication of IBD (67). Notably, in IBD-specific cases, higher mean concentrations of urinary 4-tert-octylphenol were associated with the increased prevalence of IBD (67).
To reduce the adverse health effects of BPA, in 2015, the European Food Safety Authority (EFSA) reduced the tolerable daily intake (TDI) of BPA from 50 μg/kg body weight (bw)/d to 4 μg/kg bw/d (68). The estimated recommended daily intake of BPA ranges from < 1 to 5 μg/kg bw/d (69). To reduce EDCs exposure, it is essential to choose EDCs-free products and not heat food or store hot food in BPA containers marked with the recycling code 3 or 7 (70). From the present situation, it is important to strengthen the food safety policy, and use suitable materials in direct contact with food (56, 71). In the meantime, the necessary action is to reduce waste and use EDCs-free packaging, which may contribute to health improvement in food and reduce the risk of IBD.
Chemical herbicides are herbicides that inhibit the growth of unwanted plants like residential weeds and invasive species (72). Commercially used chemical herbicides can cause substantial mortality of non-target plants and insects. They contaminate soil and reside in water, and may accumulate in the environment over time (Table 3). Glyphosate is the most popular herbicide in America. Its concentration continued to soar in the world, with the level rising from 2 to 430 μg/L in natural water (79). The rising level in water causes severe pollution and threatens food safety.
Chemical herbicides affect humans mainly through intestinal absorption, skin exposure, and inhalation (69). They may enter the body through contaminated food, like crops, fruits, and vegetables (80). In a German survey, the urinary glyphosate concentrations of 3 to 17-year-old children were above the limit of quantification of 0.1 μg/L, and the overall exposure of the young population may relate to their vegetarian diet or consumption of cereals, pulses, or vegetables (81). Much research hasproven the adverse effects of chemical herbicides on non-human beings, such as mice, zebrafish, and livestock.
Herbicides, including glyphosate, 2,4-Dicholorophenoxy acetic acid (2,4-D), and dicamba, may damage the immune system and cause symptoms of IBD, like diarrhea, bowel inflammation, and maldigestion (77). Especially, people with weakened immune systems are more susceptible to chemical herbicide-related intestinal inflammation (82). Herbicides may disrupt the normal gut flora, and irritate the lining of the digestive tract, which can lead to gut inflammation and gastrointestinal diseases like IBD (83).
Glyphosate is an active ingredient in Roundup, the most widely-used herbicide (84, 85). Glyphosate exposure may be a critical environmental trigger in the etiology of diseases associated with gut microbiota dysbiosis, including IBD (73, 86, 87). Glyphosate can alter the structure of microbiota, interfere with the shikimate pathway in microbiomes, and hinder the production of aromatic amino acids (88, 89). And the dietary intake of aromatic amino acids may alleviate the antimicrobial effect of glyphosate (90). Glyphosate exposure induces inflammatory responses in the small intestine, and alters the gut microbial composition in rats, with the Lactobacillus significantly decreasing (73). It also destroys the intestinal mucosal barrier function, leading to dysbiosis and chronic inflammation.
Other essential elements in chemical herbicides include dicamba, 2,4-D, 2,4-Dinitrofluorobenzene, and 2,4,5-Trichlorophenoxyacetic acid. The sub-chronic low-dose 2,4-D exposure may influence gut microbiome homeostasis, significantly lower the acylcarnitine level, and decrease levels of plasma acylcarnitine (76). An IBD multi-omics research showed that the rising level of acylcarnitine is closely related to the development of IBD (91). But another explanation is that decreasing acylcarnitine levels can also produce toxicity, suggesting the perturbations in the fatty acid beta-oxidation pathway (92). Dicamba, 2,4-D, and glyphosate alone or in combination, account for genotoxicity in patients with gastrointestinal disorders, including DNA damage, oxidative stress, and unfolded protein response, which contributes to the development of IBD (77). Another herbicide, Propyzamide, can boost gut inflammation by upregulating NF-κB-driven C/EBPβ pro-inflammatory gene and inhibiting AHR signaling pathways, and further, inducing the development of IBD (78). Furthermore, an organic diet can reduce the human body's herbicide level, which is a possible solution to reduce herbicide residue and lower the risk of IBD.
Heavy metals are naturally occurring elements with high atomic weight and density (93). They are also called trace elements, usually detected in trace concentrations (ppb range to < 10 ppm) (94). The heavy metals we discuss are those accumulated in the food chain and are highly toxic to living organisms. Most of them come from natural resources and industry (95–98), including lead (Pb), manganese (Mn), arsenic (As), cadmium (Cd), mercury (Hg), and others. They are commonly used in people's daily life with widespread pollution (99, 100).
Human activities may increase the number of heavy metals residing in the environment, including metal processing, and the production of medical waste, plastic products, and electric wastes (101–104). Dietary is the main source of human exposure, with its detrimental effects including cardiovascular, neurological, reproductive, and intestinal disorders (105–108). Here we focus on five heavy metals: Pb, Mn, As, Cd, and Hg. They are not only ubiquitous in the environment, but also associated with gut microbiota dysbiosis and the severity of IBD (Table 4).
Most Pb emissions in life come from gasoline and enter the environment through burning exhausts. Pb enters the body mainly through dietary exposure, including food (65%) and water (20%) (117). For the Pb in the food, adults can absorb 10–15% of the ingested Pb, while children can absorb up to 50% through the gastrointestinal tract, which indicates children are more susceptible to Pb exposure.
Most Pb ingested accumulates in the kidneys, followed by the liver and other soft organs, like the heart and brain (94). When its dose is over 70 μg/dL, severe consequence happens (118). Pb can cause various disorders by inducing oxidative stress and breaking membrane integrity (119, 120). It also impairs gastrointestinal function and contributes to IBD pathogenies.
Epidemiological findings show that the level of Pb in IBD patients rises significantly (121). In CD patients, Pb in the scalp hair were significantly lower than that in healthy individuals, and its concentration in the serum is lower, which is consistent with the rising level in the tissue (122). In animals exposed to Pb, the amount of intestinal mucus increases, and the diversity and abundance of gut microbiota also change significantly (123, 124). Many metabolites related to glucolipid metabolism, amino acid metabolism, and nucleotide metabolism have changed after Pb exposure (109). Besides, Pb is highly toxic to Escherichia coli and Lactic acid bacteria, and long-term Pb exposure will induce chronic toxicity in a dose-dependent manner (11).
Developed countries have higher centration of Pb emission (125), which corresponds to the fact that IBD in developed countries shows a higher prevalence. Therefore, it is necessary for people in developed countries to take more precautions.
Manganese (Mn) is the 12th most abundant element on the Earth. It exists mainly in the chemical oxidation state (126), and it is necessary for normal body functioning (94). It can activate various enzymes in the body and is indispensable for the development of intestinal immune functions (126).
The content of Mn in vegetables is higher than that of animal food (127). Seafood, chocolate, nuts, fruits, rice, and spices are also essential sources of Mn (127). The average concentration of Mn in human tissue is 1 mg/kg (126). Excessive dietary intake may lead to impaired intestinal immune function and over-activate oxidative stress, which is closely associated with the inflammation process in IBD patients.
The serum Mn concentration is different between healthy individuals and IBD patients, with the extent much greater in IBD patients (121, 128, 129). The concentration of Mn in blood is markedly higher in CD patients (121). But animal experiments showed some contradictions. In Mn-deficient mice treated with DSS, the incidence of IBD increases, along with higher inflammatory cytokine levels, oxidative damage, and DNA damage, which indicates the level of Mn may be inversely correlated to the incidence of IBD.
Another two studies also indicate Mn's protective role in gut homeostasis. One shows that a decrease in absorption and accumulation of Mn will trigger the release of proinflammatory factors and exacerbate the severity of inflammation (111). Another study implies Mn can boost the immune system by enhancing the function of intestinal CD8+ T cells (130). Furthermore, some studies have indicated the protective role of Mn for IBD. A study on manganese metal-organic framework (Mn-MOF), is a practical application in the treatment of spontaneous IBD by scavenging ROS to relieve oxidative stress, and protect the intestinal barrier (131). The study on hollow MnO2 (hMnO2) carried out to achieve synergistic IBD therapy, is based on MnO2, which has highlighted SOD-like and CAT-like activities (132, 133). According to the aforementioned viewpoints, the role of Mn for IBD is contradictory. For IBD patients, higher serum Mn concentration may be a protective reaction to avoid producing rapid and excessive ROS. The assumption can be consistent with the fact that IBD is a chronic disease. Experimenting to test the changes in serum Mn concentration with time is valuable. These exposure may increase the risk of IBD through oxidative stress and other mechanism in the gut. It is necessary to come up with the hypotheses to sort out the contradiction.
Arsenic (As) is an essential and poisonous substance commonly found in contaminated soils and water (134). It is also rich in fish and marine mollusks (135). The roots of crops and vegetables also contain high-concentration As (118).
According to World Health Organization, the permissible limit for As in drinking water is 10 μg/L (136). And if the exposure dose is over 50 μg/L, it can lead to gastrointestinal tract dysfunction and multiple organ disorder (136–139). Long-term exposure or high ingestion doses may increase the accumulated As in the gut (140).
Mounting evidence has revealed some intrinsic connections between As exposure and IBD. It enters the body through dietary intake, and metabolites to arsenic trioxide (ATO) in the gut, which is toxic to the gut microenvironment (112). It not only induces intestinal damage and liver inflammatory cell infiltration, but also reduces gut microbiota diversity (112, 141). In ATO exposure, the expression of intestinal barrier-related proteins, such as Claudin-1, MUC2, ZO-1, and occludin, significantly decreases, resulting in increased intestinal permeability (142, 143). However, with exposure to inorganic As, the expression of Claudin-1 reduces, resulting in increased permeability and intestinal barrier disruption. ATO can also activate inflammasome NLRP3, and induce a cascade effect of the LPS/TLR4/NF-κB signaling pathway, which exacerbates the inflammatory severity (112). However, ATO can inhibit NF-κB expression, increase procaspase-3, and induce caspase-3 activation leading to apoptosis to eliminate inflamed cells, which indicates the anti-inflammatory effect of ATO. Another study shows that ATO exposure can alleviate the inflammatory extent in DSS-induced IBD mice by increasing catalase and GSH levels to enhance antioxidants (144). In the epidemiological study, the level of serum As concentration is higher in CD patients compared to healthy adults (121). The causes for the distinct results may include the various metabolism of As in different species, exposure to the diverse form of As (inorganic As or ATO), the various regulation approaches, and the dose of As.
Cadmium (Cd) is mainly used as an anticorrosion agent, and it naturally occurs in ores (93). Cd can enter the human body through contaminated food and water via the gastrointestinal tract, inhalation, and dermal tissues (145). Food is the most important source of Cd exposure in the general non-smoking population, which indicates the risk of dietary exposure. Recent studies have found that Cd is highly enriched in some aquatic animals like zebrafish and crabs. Ingestion of Cd is highly related to gastrointestinal disturbances such as diarrhea, nausea, and abdominal pain. Meanwhile, its chronic exposure can increase risks concerning multiple organ dysfunction, bone deformation, and contribute to cancer cell progression (146).
Cd exposure can also significantly affect the gut microenvironment. It can perturb the diversity and abundance of gut microflora, especially decreasing the number of Firmicutes and γ-proteobacteria (145). In addition, Cd exposure also elevates the level of TNF-α, IFN-γ, and IL-17 in the colon (147). Additionally, Cd exposure can increase intestinal permeability through decreasing mRNA expressions of ZO-1, ZO-2, occludin, and claudin-1 in the jejunum and colon, accompanied by intestinal histological changes (148).
However, research also indicates the potential protective role of Cd. Cd may interfere with LPS signaling, particularly disrupting macrophage inflammation by inhibiting the NF-κB pathway in the gut, inhibiting the pro-inflammatory effect of M1 macrophage (149). Short-term exposure to Cd exacerbates the symptoms of acute DSS- and TNBS-induced colitis, while sub-chronic exposure to Cd significantly alleviates some symptoms in DSS-induced colitis and reduces the severity of colitis in a dose-dependent manner. Its potential mechanisms include reversible reduction in epithelial permeability, stimulation of anti-oxidant pathways, upregulation of oxidative defense mechanism, and downregulation of Nf-κB and pro-inflammatory cytokine pathways (113). Moreover, the study also implies that the outcomes of Cd exposure may vary as a function of dose and exposure time. Along with other common heavy metals like Mn, As, and Pb, the Cd concentration is markedly higher in CD patients (121). Thus, further studies concerning Cd exposure relationship with IBD are needed to clear out the Cd dose, exposure time, and the synthetic effect of Cd regulating in different pathways.
Mercury (Hg) is a well-known component in medical apparatus like thermometers and other medical instruments (93). The absorptivity of Hg is 8–15% in the gastrointestinal tract (104). Human's primary exposure to Hg is dietary intake (125, 150–152).
Hg can accumulate in untreated wastewater from factory and agricultural runoff, which directly contaminates the crops and fish. Correspondingly, methyl mercury, a chemical substance converted from Hg, is discovered to be highly enriched in vegetables and fish, which implies the ability of Hg to accumulate in the food chain.
The toxicity of Hg can induce multiple organ failures when the dose of Hg exceeds 10 μg/L in blood or 20 μg/L in urine, such as lung injury, intestinal damage, proteinuria, allergies, and chronic poisoning.
Exposure to Hg is closely associated with IBD. Methyl mercury can accumulate in organs, and change the composition of gut microbes (116). Moreover, dietary exposure to Hg affects the growth of mice, partly due to changed gut microbiota (114). Mice exposed to Hg have a decreased abundance of Bacteroidetes and Proteobacteria, and an increased abundance of Clostridium, Lactobacillus, Treponema, and Helicobacter in the gut (115). The toxicity of Hg may also contribute to the development of IBD (115). It can directly break the calcium homeostasis and activate multiple enzymes by affecting the electron transport chains in mitochondria, producing superfluous reactive oxygen species (ROS) (115, 153, 154). Besides, ROS promotes mitosis, polyploid aberration, and susceptibility to DNA damage in the gut (155–157). Epidemiological studies have also shown altered enzyme activity in people exposed to Hg (29).
In conclusion, diet exposure is the common exposure route for heavy metals. Along with the food chain, heavy metals enter the human body, generating adverse health effects by various mechanisms. Gut injury caused by heavy metals is highly associated with the occurrence and development of IBD. Heavy metals can alter the gut microbiota by increasing some flora and decreasing other flora, then causing gut dysbiosis. In addition, some uncommon impacts include damaged intestinal barrier function, increased levels of inflammation cytokines, oxidative stress, etc. Meanwhile, other organ dysfunction can occur due to heavy metals exposure and accumulation. As heavy metals tend to accumulate in fish (107), people with a disease or hypo-immunity should reduce their eating frequency. And IBD patients should avoid dietary exposure to heavy metals. For relevant authorities, they should supervise factories' proper treatment of sewage and sludge to reduce heavy metals accumulation in crops (158–160). Bioremediation can also work by changing pollutants into food and energy (161, 162). Some novel ways also focus on dealing with heavy metal contamination, such as Particle Capture Systems, soil displacement/isolation, and Soil-flow-electrode capacitive deionization.
Persistent organic pollutants (POPs) are chemicals of global concern with the potential to persist in the environment. They can bio-accumulate and bio-magnify in ecosystems and threaten human health (71). POPs mainly include new pesticides, chemicals, and by-products of industrial production, which may lead to multiple effects on immune response and alter gut function (Table 5).
New pesticide is widely used to wipe out indoor and outdoor pests, such as imidacloprid, pyrethroids, and β-ketonitrile derivatives (169). It harms humans by taking the contaminated food and water (170), and causing intoxication through its accumulation in the food chain (171). Its exposure mainly includes the intake of vegetables, fruits, and grains. Among these, pesticide can residue more easily in grains. A food survey of Swedish adolescents showed that secondary school students who consumed grains had a higher exposure to pesticides than those who consumed vegetables and fruits, lending support to the findings (172).
Emerging links between pesticide residue and changes in the gut have emerged in recent years (173). Exposure to low-dose pesticide in diet seldom causes immediate health effect. Long-term exposure to chemicals in the pesticide can induce gut microbiota dysbiosis, alter the immune response in the gut, and contribute to the development of IBD (174, 175). Research on dietary exposure to chlorpyrifos, a widely used pesticide, suggests that dietary exposure can affect the population of immune-cell, induce inflammatory responses, and lead to severe tissue injury in DSS-induced colitis mice.
Another popular pesticide, imidacloprid (IMI), also adversely affects the gut microbiota (164, 165). IMI exposure can induce intestinal injury and oxidative stress in the gut of zebrafish (164). Additionally, it also results in a higher intestinal LPS level and the overexpression of inflammatory factors in the gut, as well as a rising level of the biochemical responses, transcriptome, and gut microbiota in the Pacific white shrimp. Human exposure to IMI is also observed in recent years. Currently, the maximum estimated daily intake of IMI [34.8 μg/kg bw/d] was lower than the chronic reference dose of IMI (57 μg/kg-bw/d) recommended by the United States Environmental Protection Agency (176). But IMI is already reported to have adverse effects on human semen quality parameters and the activation of macrophages in the body, which may increase the permeability of the intestine and impair the immune system (177, 178).
Another type of POPs is chemicals and by-products derivate from industrial production (179). These pollutants include Polychlorinated biphenyls (PCBs), Polybrominated dibenzo-p-dioxins and furans (PBDD/Fs), 2,3,7,8-Tetrachlorodibenzo-p-dioxin (TCDD), etc. (167). They can accumulate in the environment and exert a long-term adverse effect on human health (180). The food chain and the food web are the primary pathways of human exposure (180). Human exposure to them in diets mainly includes ingesting contaminated food, like fruits, vegetables, and grains, and eating polluted meat, milk, eggs, and fish, which is closely associated with IBD.
Polychlorinated biphenyls (PCBs) are synthetic organochlorine chemicals, which are mixtures of 209 different components (181). And PCBs are among the 12 initial POPs listed under the Stockholm Convention.
PCBs are mainly formed as by-products in manufacturing industries (182). They can reserve in soil and transfer to water surroundings, increasing the risk of human exposure via food chains (183, 184). Since PCBs are lipid-soluble, people who frequently eat animal fats can easily access PCBs (185). Contaminated meat and milk also show high concentrations of PCBs (186), and aquatic product consumption also increases the risk of PCBs exposure (181).
PCBs play a pro-inflammatory role in various diseases, which adversely affect IBD. They can induce oxidative stress by uncoupling CYP1A1 dose-dependently, and disrupt the normal endothelial barrier function (110, 187). Meanwhile, PCBs induce proinflammatory factors like IL-6 and vascular adhesion molecules such as VCAM-1, and then activate the NF-κB pathway (187–189). The expression of these molecules facilitates the recognition and migration of leukocytes, which are critical events of inflammatory responses (187). When exposed to PCBs, hosts can show disorders of gut microbiota, with reduced gut microbial diversity and variety (190, 191). In mice exposed to PCBs, the amount of Bacteroidales, Erysipelotrichales, Lactobacillales, Bifidobacteriales, Phyla Proteobacteria, Actinobacteria, Saccharibacteria, Deferribacteres, Firmicutes, and Verrucomicrobia increases significantly, while the level of Bacteroidetes decreases. Research in humans also shows that exposure to PCBs may interfere with the DNA hypomethylation of peripheral blood monocytes, inducing chronic inflammation (192).
Apart from PCBs, other POPs such as PBDD/Fs and TCDD are also related to IBD (193). These organics are by-products of industry, which are classified into unintentional POPs (194). Their primary exposure pathway is dietary intake.
Plant-based food is reported to show higher PBDD/F, and the overly dietary intakes of PBDD/F suggest some population groups, particularly young children, will exceed the tolerable weekly intake (2 pg TEQ/kg bw/week) (167). The metabolic mechanism of PBDD includes causing oxidative stress, apoptosis, and cell damage. It can induce gut inflammation and dysbiosis of the gut microenvironment, leading to IBD development.
TCDD is the most potent chemical carcinogen evaluated by the US Environmental Protection Agency (195). It has a long half-life of 5–10 years in humans, due to its high lipophilicity and low metabolism (195). Most TCDD released into the atmosphere eventually settles onto the plant, soil, and water surfaces. After being taken, it accumulates in blood serum and adipose tissue, which leads to further damage in the body (195). An animal experiment showed that maternal exposure to TCDD suppresses the differentiation of Type 3 innate lymphoid cells (ILC3s) in the offspring, and distinctly affects colonic ILC3 function (196). Since ILC3s play a significant role in the mucosal immune response in the pathogenesis of IBD, there is a close connection between TCDD exposure and the occurrence and development of IBD (197). Moreover, TCDD can impact the gut microbiota and metabolic pathways, such as upregulating harmful bacteria and downregulating beneficial bacteria (168).
In sum, people are exposed to POPs primarily through dietary intake. POPs can alter transcriptional and metabolic pathways in cecal bacterial mixtures, modify gut microbiota-host homeostasis, and affect the metabolism of individual bacteria in vitro (166). POPs can also alter the microbial community structure and metabolic activities, leading to host disorders (198). Some POPs can increase the amount of Proteus and proportion of Firmicutes/Bacteroidetes, and increase the synthesis of short-chain fatty acid, which is related to the inflammatory changes of IBD (191). Epidemiological studies also present the adverse health effects of POPs. The interplays between POPs and gut microbiota lead to intestinal inflammatory changes and resultant toxicity (198). That is to say, the variations in the microbial communities partially indicate the body's exposure to these pollutants. Therefore, it is essential to formulate some daily dietary interventions such as prebiotics, probiotics, or symbiotics, which could impede or alleviate detrimental impacts induced by exposure to POPs.
Mounting evidence of the underlying hazards of emerging contaminant exposures in IBD arouses increasing attention. However, the correlation of timing and frequency of such contaminant exposures to IBD incidence and disease phenotype was not specified. Environmental exposure may contribute to the pathogenesis of IBD through diet intake and the metabolic mechanism in the body. The currently accepted pathogenesis of IBD includes the interplay between genetic susceptibility and environmental factors, as well as the gut microbiome and the immune system.
The further relationship between these emerging contaminants and the risk of IBD deserves everyone's attention. Currently, these contaminants mainly exert long-term adverse effects by accumulating in the body and inducing chronic inflammation. Research in this field should focus more on the direct relationship and mechanism of these contaminants and the health of the human gut. And more epidemiological research about new emerging contaminants and their adverse health effects is needed.
In this review, we have summarized the standard ways of exposure and inclusion of emerging contaminants, and their adverse effects on IBD patients through various underlying mechanisms (Figure 2). Exposure to these pollutants will increase the risk of IBD in healthy individuals. And people with IBD should pay special attention to preventing daily exposure to these contaminants, as they may cause adverse health effects regardless of age and exposure time. Exposure to these new emerging contaminants may increase the risk of IBD and accelerate the process of IBD. Therefore, understanding the role of these contaminants, including how they enter the body, how they induce immune-related reactions, and how they affect certain inflammatory diseases like IBD, will enable the more comprehensive formation of policies concerning the prevention and control, and will reduce medical expenses and burdens on the families and countries.
Figure 2. The main ECs and their impacts on gut microenvironment. ECs damage the intestine by regulating the intensity of immune response, releasing proinflammatory factors, impairing the intestinal barrier, and increasing the intestinal permeability through various mechanism. The figure illustrates the potential mechanism of five ECs mentioned in the review. ECs, Emerging contaminants; EDCs, Endocrine-disrupting chemicals; POPs, Persistent organic pollutants; SCFA, Short-chain fatty acid; TLR4, Toll-like receptors 4; ILC-3, Innate lymphoid cells; VCAM 1, Vascular cell adhesion molecule 1.
XC: conceptualization, design, draft writing, and writing—review and editing. SW: design, draft writing, review, and writing—review and editing. XM: draft writing, review, and visualization. XX, AZ, YM, XY, and SP: methodology and review. SY: review and visualization. JC: methodology and writing—review and editing. XW: conceptualization, funding acquisition, and writing—review and editing. MD: conceptualization, design, methodology, and writing—review and editing. All authors contributed to the article and approved the submitted version.
This work was supported by the National Natural Science Foundation of China (81970494) and Key Project of Research and Development Plan of Hunan Province (2019SK2041).
The authors declare that the research was conducted in the absence of any commercial or financial relationships that could be construed as a potential conflict of interest.
All claims expressed in this article are solely those of the authors and do not necessarily represent those of their affiliated organizations, or those of the publisher, the editors and the reviewers. Any product that may be evaluated in this article, or claim that may be made by its manufacturer, is not guaranteed or endorsed by the publisher.
1. Kaplan GG. The global burden of Ibd: from 2015 to 2025. Nat Rev Gastroenterol Hepatol. (2015) 12:720–7. doi: 10.1038/nrgastro.2015.150
2. Baumgart DC, Carding SR. Inflammatory bowel disease: cause and immunobiology. Lancet. (2007) 369:1627–40. doi: 10.1016/S0140-6736(07)60750-8
3. Khor B, Gardet A, Xavier RJ. Genetics and pathogenesis of inflammatory bowel disease. Nature. (2011) 474:307–17. doi: 10.1038/nature10209
4. Bernstein CN, Loftus EV Jr, Ng SC, Lakatos PL, Moum B. Hospitalisations and surgery in Crohn's disease. Gut. (2012) 61:622–9. doi: 10.1136/gutjnl-2011-301397
5. Singh S, Feuerstein JD, Binion DG, Tremaine WJ. Aga technical review on the management of mild-to-moderate ulcerative colitis. Gastroenterology. (2019) 156:769–808.e29. doi: 10.1053/j.gastro.2018.12.008
6. Piovani D, Danese S, Peyrin-Biroulet L, Nikolopoulos GK, Lytras T, Bonovas S. Environmental risk factors for inflammatory bowel diseases: an umbrella review of meta-analyses. Gastroenterology. (2019) 157:647–59.e4. doi: 10.1053/j.gastro.2019.04.016
7. Bilal M, Adeel M, Rasheed T, Zhao Y, Iqbal HMN. Emerging contaminants of high concern and their enzyme-assisted biodegradation - a review. Environ Int. (2019) 124:336–53. doi: 10.1016/j.envint.2019.01.011
8. Landrigan PJ, Fuller R, Acosta NJR, Adeyi O, Arnold R, Basu N, et al. The lancet commission on pollution and health. Lancet. (2018) 391:462–512. doi: 10.1016/s0140-6736(17)32345-0
9. Ryu H, Li B, De Guise S, McCutcheon J, Lei Y. Recent progress in the detection of emerging contaminants Pfass. J Hazard Mater. (2021) 408:124437. doi: 10.1016/j.jhazmat.2020.124437
10. Thompson RC, Olsen Y, Mitchell RP, Davis A, Rowland SJ, John AW, et al. Lost at sea: where is all the plastic? Science. (2004) 304:838. doi: 10.1126/science.1094559
11. Yu L, Duan H, Yu Y, Zhang Q, Zhao J, Zhang H, et al. Dose-dependent effects of chronic lead toxicity in vivo: focusing on trace elements and gut microbiota. Chemosphere. (2022) 301:134670. doi: 10.1016/j.chemosphere.2022.134670
12. Liu Q, Chen Z, Chen Y, Yang F, Yao W, Xie Y. Microplastics and nanoplastics: emerging contaminants in food. J Agric Food Chem. (2021) 69:10450–68. doi: 10.1021/acs.jafc.1c04199
13. Kannan K, Vimalkumar K. A review of human exposure to microplastics and insights into microplastics as obesogens. Front Endocrinol. (2021) 12:724989. doi: 10.3389/fendo.2021.724989
14. Schwarzfischer M, Niechcial A, Lee SS, Sinnet B, Wawrzyniak M, Laimbacher A, et al. Ingested nano- and microsized polystyrene particles surpass the intestinal barrier and accumulate in the body. Nanoimpact. (2022) 25:100374. doi: 10.1016/j.impact.2021.100374
15. Luo T, Wang D, Zhao Y, Li X, Yang G, Jin Y. Polystyrene microplastics exacerbate experimental colitis in mice tightly associated with the occurrence of hepatic inflammation. Sci Total Environ. (2022) 844:156884. doi: 10.1016/j.scitotenv.2022.156884
16. Zheng H, Wang J, Wei X, Chang L, Liu S. Proinflammatory properties and lipid disturbance of polystyrene microplastics in the livers of mice with acute colitis. Sci Total Environ. (2021) 750:143085. doi: 10.1016/j.scitotenv.2020.143085
17. Lu L, Wan Z, Luo T, Fu Z, Jin Y. Polystyrene microplastics induce gut microbiota dysbiosis and hepatic lipid metabolism disorder in mice. Sci Total Environ. (2018) 631–2:449–58. doi: 10.1016/j.scitotenv.2018.03.051
18. Li B, Ding Y, Cheng X, Sheng D, Xu Z, Rong Q, et al. Polyethylene microplastics affect the distribution of gut microbiota and inflammation development in mice. Chemosphere. (2020) 244:125492. doi: 10.1016/j.chemosphere.2019.125492
19. Schwabl P, Koppel S, Konigshofer P, Bucsics T, Trauner M, Reiberger T, et al. Detection of various microplastics in human stool: a prospective case series. Ann Intern Med. (2019) 171:453–7. doi: 10.7326/M19-0618
20. Zhang N, Li YB, He HR, Zhang JF, Ma GS. You are what you eat: microplastics in the feces of young men living in Beijing. Sci Total Environ. (2021) 767:144345. doi: 10.1016/j.scitotenv.2020.144345
21. Hirt N, Body-Malapel M. Immunotoxicity and intestinal effects of nano- and microplastics: a review of the literature. Part Fibre Toxicol. (2020) 17:57. doi: 10.1186/s12989-020-00387-7
22. Hu K, Yang Y, Zuo J, Tian W, Wang Y, Duan X, et al. Emerging microplastics in the environment: properties, distributions, and impacts. Chemosphere. (2022) 297:134118. doi: 10.1016/j.chemosphere.2022.134118
23. Senathirajah K, Attwood S, Bhagwat G, Carbery M, Wilson S, Palanisami T. Estimation of the mass of microplastics ingested - a pivotal first step towards human health risk assessment. J Hazard Mater. (2021) 404(Pt B):124004. doi: 10.1016/j.jhazmat.2020.124004
24. Kwon JH, Kim JW, Pham TD, Tarafdar A, Hong S, Chun SH, et al. Microplastics in food: a review on analytical methods and challenges. Int J Environ Res Public Health. (2020) 17:6710. doi: 10.3390/ijerph17186710
25. Leslie HA, van Velzen MJM, Brandsma SH, Vethaak AD, Garcia-Vallejo JJ, Lamoree MH. Discovery and quantification of plastic particle pollution in human blood. Environ Int. (2022) 163:107199. doi: 10.1016/j.envint.2022.107199
26. Liu M, Liu J, Xiong F, Xu K, Pu Y, Huang J, et al. Research advances of microplastics and potential health risks of microplastics on terrestrial higher mammals: a bibliometric analysis and literature review. Environ Geochem Health. (2023):1–36. doi: 10.1007/s10653-022-01458-8
27. Prata JC, da Costa JP, Lopes I, Duarte AC, Rocha-Santos T. Environmental exposure to microplastics: an overview on possible human health effects. Sci Total Environ. (2020) 702:134455. doi: 10.1016/j.scitotenv.2019.134455
28. Agarwal KC, Vinayak VK, Ganguly NK, Kumar M, Chhuttani PN. Ecological effects of production of biogas from human excreta on the enteric pathogens. Indian J Med Res. (1978) 67:737–43.
29. Atis S, Tutluoglu B, Levent E, Ozturk C, Tunaci A, Sahin K, et al. The respiratory effects of occupational polypropylene flock exposure. Eur Respir J. (2005) 25:110–7. doi: 10.1183/09031936.04.00138403
30. Lu Y, Zhang Y, Deng Y, Jiang W, Zhao Y, Geng J, et al. Uptake and accumulation of polystyrene microplastics in zebrafish (Danio rerio) and toxic effects in liver. Environ Sci Technol. (2016) 50:4054–60. doi: 10.1021/acs.est.6b00183
31. Valavanidis A, Vlachogianni T, Fiotakis K, Loridas S. Pulmonary oxidative stress, inflammation and cancer: respirable particulate matter, fibrous dusts and ozone as major causes of lung carcinogenesis through reactive oxygen species mechanisms. Int J Environ Res Public Health. (2013) 10:3886–907. doi: 10.3390/ijerph10093886
32. Barboza LGA, Vieira LR, Branco V, Figueiredo N, Carvalho F, Carvalho C, et al. Microplastics cause neurotoxicity, oxidative damage and energy-related changes and interact with the bioaccumulation of mercury in the European seabass, dicentrarchus labrax (Linnaeus, 1758). Aquat Toxicol. (2018) 195:49–57. doi: 10.1016/j.aquatox.2017.12.008
33. Canesi L, Ciacci C, Bergami E, Monopoli MP, Dawson KA, Papa S, et al. Evidence for immunomodulation and apoptotic processes induced by cationic polystyrene nanoparticles in the hemocytes of the marine bivalve mytilus. Mar Environ Res. (2015) 111:34–40. doi: 10.1016/j.marenvres.2015.06.008
34. Forte M, Iachetta G, Tussellino M, Carotenuto R, Prisco M, De Falco M, et al. Polystyrene nanoparticles internalization in human gastric adenocarcinoma cells. Toxicol In Vitro. (2016) 31:126–36. doi: 10.1016/j.tiv.2015.11.006
35. Powell JJ, Thoree V, Pele LC. Dietary microparticles and their impact on tolerance and immune responsiveness of the gastrointestinal tract. Br J Nutr. (2007) 98(Suppl. 1):S59–63. doi: 10.1017/S0007114507832922
36. Salim SY, Kaplan GG, Madsen KL. Air pollution effects on the gut microbiota: a link between exposure and inflammatory disease. Gut Microbes. (2014) 5:215–9. doi: 10.4161/gmic.27251
37. Zhu D, Chen Q-L, An X-L, Yang X-R, Christie P, Ke X, et al. Exposure of soil collembolans to microplastics perturbs their gut microbiota and alters their isotopic composition. Soil Biol Biochem. (2018) 116:302–10. doi: 10.1016/j.soilbio.2017.10.027
38. Powell JJ, Faria N, Thomas-McKay E, Pele LC. Origin and fate of dietary nanoparticles and microparticles in the gastrointestinal tract. J Autoimmun. (2010) 34:J226–33. doi: 10.1016/j.jaut.2009.11.006
39. Tang Y, Han Y, Zhang W, Yu Y, Huang L, Zhou W, et al. Bisphenol a and microplastics weaken the antimicrobial ability of blood clams by disrupting humoral immune responses and suppressing hemocyte chemotactic activity. Environ Pollut. (2022) 307:119497. doi: 10.1016/j.envpol.2022.119497
40. Yan Z, Liu Y, Zhang T, Zhang F, Ren H, Zhang Y. Analysis of microplastics in human feces reveals a correlation between fecal microplastics and inflammatory bowel disease status. Environ Sci Technol. (2022) 56:414–21. doi: 10.1021/acs.est.1c03924
41. Feakins R, Torres J, Borralho-Nunes P, Burisch J, Cúrdia Gonçalves T, De Ridder L, et al. Ecco topical review on clinicopathological spectrum and differential diagnosis of inflammatory bowel disease. J Crohns Colitis. (2022) 16:343–68. doi: 10.1093/ecco-jcc/jjab141
42. Wright SL, Kelly FJ. Plastic and human health: a micro issue? Environmental Sci Technol. (2017) 51:6634–47. doi: 10.1021/acs.est.7b00423
43. Xie S, Zhang R, Li Z, Liu C, Chen Y, Yu Q. Microplastics perturb colonic epithelial homeostasis associated with intestinal overproliferation, exacerbating the severity of colitis. Environ Res. (2023) 217:114861. doi: 10.1016/j.envres.2022.114861
44. Qiao R, Sheng C, Lu Y, Zhang Y, Ren H, Lemos B. Microplastics induce intestinal inflammation, oxidative stress, and disorders of metabolome and microbiome in zebrafish. Sci Total Environ. (2019) 662:246–53. doi: 10.1016/j.scitotenv.2019.01.245
45. Fackelmann G, Sommer S. Microplastics and the gut microbiome: how chronically exposed species may suffer from gut dysbiosis. Mar Pollut Bull. (2019) 143:193–203. doi: 10.1016/j.marpolbul.2019.04.030
46. Kang HM, Byeon E, Jeong H, Kim MS, Chen Q, Lee JS. Different effects of nano- and microplastics on oxidative status and gut microbiota in the marine medaka oryzias melastigma. J Hazard Mater. (2021) 405:124207. doi: 10.1016/j.jhazmat.2020.124207
47. Yin K, Wang D, Zhao H, Wang Y, Zhang Y, Liu Y, et al. Polystyrene microplastics up-regulates liver glutamine and glutamate synthesis and promotes autophagy-dependent ferroptosis and apoptosis in the cerebellum through the liver-brain axis. Environ Pollut. (2022) 307:119449. doi: 10.1016/j.envpol.2022.119449
48. Huang Z, Weng Y, Shen Q, Zhao Y, Jin Y. Microplastic: a potential threat to human and animal health by interfering with the intestinal barrier function and changing the intestinal microenvironment. Sci Total Environ. (2021) 785:147365. doi: 10.1016/j.scitotenv.2021.147365
49. Qiao R, Deng Y, Zhang S, Wolosker MB, Zhu Q, Ren H, et al. Accumulation of different shapes of microplastics initiates intestinal injury and gut microbiota dysbiosis in the gut of zebrafish. Chemosphere. (2019) 236:124334. doi: 10.1016/j.chemosphere.2019.07.065
50. Natividad JM, Verdu EF. Modulation of intestinal barrier by intestinal microbiota: pathological and therapeutic implications. Pharmacol Res. (2013) 69:42–51. doi: 10.1016/j.phrs.2012.10.007
51. The Lancet Oncology. Endocrine disruptors—the lessons (not) learned. Lancet Oncol. (2021) 22:1483. doi: 10.1016/S1470-2045(21)00597-0
52. Casas M, Gascon M. Prenatal exposure to endocrine-disrupting chemicals and asthma and allergic diseases. J Investig Allergol Clin Immunol. (2020) 30:215–28. doi: 10.18176/jiaci.0580
53. Arbuckle TE, Agarwal A, MacPherson SH, Fraser WD, Sathyanarayana S, Ramsay T, et al. Prenatal exposure to phthalates and phenols and infant endocrine-sensitive outcomes: the mirec study. Environ Int. (2018) 120:572–83. doi: 10.1016/j.envint.2018.08.034
54. Kumar M, Sarma DK, Shubham S, Kumawat M, Verma V, Prakash A, et al. Environmental endocrine-disrupting chemical exposure: role in non-communicable diseases. Front Public Health. (2020) 8:553850. doi: 10.3389/fpubh.2020.553850
55. Yilmaz B, Terekeci H, Sandal S, Kelestimur F. Endocrine disrupting chemicals: exposure, effects on human health, mechanism of action, models for testing and strategies for prevention. Rev Endocr Metab Disord. (2020) 21:127–47. doi: 10.1007/s11154-019-09521-z
56. Ismanto A, Hadibarata T, Kristanti RA, Maslukah L, Safinatunnajah N, Kusumastuti W. Endocrine disrupting chemicals (Edcs) in environmental matrices: occurrence, fate, health impact, physio-chemical and bioremediation technology. Environ Pollut. (2022) 302:119061. doi: 10.1016/j.envpol.2022.119061
57. Malaise Y, Lencina C, Placide F, Bacquie V, Cartier C, Olier M, et al. Oral exposure to bisphenols induced food intolerance and colitis in vivo by modulating immune response in adult mice. Food Chem Toxicol. (2020) 146:111773. doi: 10.1016/j.fct.2020.111773
58. Javurek AB, Spollen WG, Johnson SA, Bivens NJ, Bromert KH, Givan SA, et al. Effects of exposure to bisphenol a and ethinyl estradiol on the gut microbiota of parents and their offspring in a rodent model. Gut Microbes. (2016) 7:471–85. doi: 10.1080/19490976.2016.1234657
59. Lai KP, Chung YT, Li R, Wan HT, Wong CK. Bisphenol a alters gut microbiome: comparative metagenomics analysis. Environ Pollut. (2016) 218:923–30. doi: 10.1016/j.envpol.2016.08.039
60. Yin F, Huang X, Lin X, Chan TF, Lai KP, Li R. Analyzing the synergistic adverse effects of bpa and its substitute, bhpf, on ulcerative colitis through comparative metabolomics. Chemosphere. (2022) 287(Pt 2):132160. doi: 10.1016/j.chemosphere.2021.132160
61. Diamante G, Cely I, Zamora Z, Ding J, Blencowe M, Lang J, et al. Systems toxicogenomics of prenatal low-dose bpa exposure on liver metabolic pathways, gut microbiota, and metabolic health in mice. Environ Int. (2021) 146:106260. doi: 10.1016/j.envint.2020.106260
62. Linares R, Fernandez MF, Gutierrez A, Garcia-Villalba R, Suarez B, Zapater P, et al. Endocrine disruption in crohn's disease: bisphenol a enhances systemic inflammatory response in patients with gut barrier translocation of dysbiotic microbiota products. FASEB J. (2021) 35:e21697. doi: 10.1096/fj.202100481R
63. Huang C, Wang Y, Lin X, Chan TF, Lai KP, Li R. Uncovering the functions of plasma proteins in ulcerative colitis and identifying biomarkers for bpa-induced severe ulcerative colitis: a plasma proteome analysis. Ecotoxicol Environ Saf. (2022) 242:113897. doi: 10.1016/j.ecoenv.2022.113897
64. Xu Y, Li Y, Scott K, Lindh CH, Jakobsson K, Fletcher T, et al. Inflammatory bowel disease and biomarkers of gut inflammation and permeability in a community with high exposure to perfluoroalkyl substances through drinking water. Environ Res. (2020) 181:108923. doi: 10.1016/j.envres.2019.108923
65. Lochhead P, Khalili H, Ananthakrishnan AN, Burke KE, Richter JM, Sun Q, et al. Plasma concentrations of perfluoroalkyl substances and risk of inflammatory bowel diseases in women: a nested case control analysis in the nurses' health study cohorts. Environ Res. (2022) 207:112222. doi: 10.1016/j.envres.2021.112222
66. Braun JM. Early-life exposure to edcs: role in childhood obesity and neurodevelopment. Nat Rev Endocrinol. (2017) 13:161–73. doi: 10.1038/nrendo.2016.186
67. de Silva PS, Yang X, Korzenik JR, Goldman RH, Arheart KL, Caban-Martinez AJ. Association of urinary phenolic compounds, inflammatory bowel disease and chronic diarrheal symptoms: evidence from the national health and nutrition examination survey. Environ Pollut. (2017) 229:621–6. doi: 10.1016/j.envpol.2017.06.023
68. Lopardo L, Petrie B, Proctor K, Youdan J, Barden R, Kasprzyk-Hordern B. Estimation of community-wide exposure to bisphenol a via water fingerprinting. Environ Int. (2019) 125:1–8. doi: 10.1016/j.envint.2018.12.048
69. Chen D, Kannan K, Tan H, Zheng Z, Feng YL, Wu Y, et al. Bisphenol analogues other than bpa: environmental occurrence, human exposure, and toxicity-a review. Environ Sci Technol. (2016) 50:5438–53. doi: 10.1021/acs.est.5b05387
70. Sokal A, Jarmakiewicz-Czaja S, Tabarkiewicz J, Filip R. Dietary intake of endocrine disrupting substances presents in environment and their impact on thyroid function. Nutrients. (2021) 13:867. doi: 10.3390/nu13030867
71. Casas G, Martinez-Varela A, Vila-Costa M, Jimenez B, Dachs J. Rain amplification of persistent organic pollutants. Environ Sci Technol. (2021) 55:12961–72. doi: 10.1021/acs.est.1c03295
72. Tyohemba RL, Pillay L, Humphries MS. Bioaccumulation of current-use herbicides in fish from a global biodiversity hotspot: Lake St Lucia, South Africa. Chemosphere. (2021) 284:131407. doi: 10.1016/j.chemosphere.2021.131407
73. Tang Q, Tang J, Ren X, Li C. Glyphosate exposure induces inflammatory responses in the small intestine and alters gut microbial composition in rats. Environ Pollut. (2020) 261:114129. doi: 10.1016/j.envpol.2020.114129
74. Suppa A, Kvist J, Li X, Dhandapani V, Almulla H, Tian AY, et al. Roundup causes embryonic development failure and alters metabolic pathways and gut microbiota functionality in non-target species. Microbiome. (2020) 8:170. doi: 10.1186/s40168-020-00943-5
75. Ding W, Shangguan Y, Zhu Y, Sultan Y, Feng Y, Zhang B, et al. Negative impacts of microcystin-Lr and glyphosate on zebrafish intestine: Linked with gut microbiota and micrornas? Environ Pollut. (2021) 286:117685. doi: 10.1016/j.envpol.2021.117685
76. Tu P, Gao B, Chi L, Lai Y, Bian X, Ru H, et al. Subchronic low-dose 2,4-D exposure changed plasma acylcarnitine levels and induced gut microbiome perturbations in mice. Sci Rep. (2019) 9:4363. doi: 10.1038/s41598-019-40776-3
77. Mesnage R, Brandsma I, Moelijker N, Zhang G, Antoniou MN. Genotoxicity Evaluation of 2,4-D, dicamba and glyphosate alone or in combination with cell reporter assays for DNA damage, oxidative stress and unfolded protein response. Food Chem Toxicol. (2021) 157:112601. doi: 10.1016/j.fct.2021.112601
78. Sanmarco LM, Chao CC, Wang YC, Kenison JE, Li Z, Rone JM, et al. Identification of environmental factors that promote intestinal inflammation. Nature (2022) 611:801–9. doi: 10.1038/s41586-022-05308-6
79. Van Bruggen AHC, He MM, Shin K, Mai V, Jeong KC, Finckh MR, et al. Environmental and health effects of the herbicide glyphosate. Sci Total Environ. (2018) 616–7:255–68. doi: 10.1016/j.scitotenv.2017.10.309
80. Supe Tulcan RX, Ouyang W, Gu X, Lin C, Tysklind M, Wang B. Typical herbicide residues, trophic transfer, bioconcentration, and health risk of marine organisms. Environ Int. (2021) 152:106500. doi: 10.1016/j.envint.2021.106500
81. Fagan J, Bohlen L, Patton S, Klein K. Organic diet intervention significantly reduces urinary glyphosate levels in U.S. children and adults. Environ Res. (2020) 189:109898. doi: 10.1016/j.envres.2020.109898
82. Belsey NA, Cordery SF, Bunge AL, Guy RH. Assessment of dermal exposure to pesticide residues during re-entry. Environ Sci Technol. (2011) 45:4609–15. doi: 10.1021/es200172q
83. Kruger M, Shehata AA, Schrodl W, Rodloff A. Glyphosate suppresses the antagonistic effect of Enterococcus spp. on clostridium botulinum. Anaerobe. (2013) 20:74–8. doi: 10.1016/j.anaerobe.2013.01.005
84. Zhou C, Luo X, Chen N, Zhang L, Gao J. C–P natural products as next-generation herbicides: chemistry and biology of glufosinate. J Agric Food Chem. (2020) 68:3344–53. doi: 10.1021/acs.jafc.0c00052
85. Sandermann H. Plant biotechnology: ecological case studies on herbicide resistance. Trends Plant Sci. (2006) 11:324–8. doi: 10.1016/j.tplants.2006.05.004
86. Matich EK, Laryea JA, Seely KA, Stahr S, Su LJ, Hsu PC. Association between pesticide exposure and colorectal cancer risk and incidence: a systematic review. Ecotoxicol Environ Saf. (2021) 219:112327. doi: 10.1016/j.ecoenv.2021.112327
87. Qiu S, Fu H, Zhou R, Yang Z, Bai G, Shi B. Toxic effects of glyphosate on intestinal morphology, antioxidant capacity and barrier function in weaned piglets. Ecotoxicol Environ Saf. (2020) 187:109846. doi: 10.1016/j.ecoenv.2019.109846
88. Samsel A, Seneff S. Glyphosate, pathways to modern diseases III: manganese, neurological diseases, and associated pathologies. Surg Neurol Int. (2015) 6:45. doi: 10.4103/2152-7806.153876
89. Del Castilo I, Neumann AS, Lemos FS, De Bastiani MA, Oliveira FL, Zimmer ER, et al. Lifelong exposure to a low-dose of the glyphosate-based herbicide roundup((R)) causes intestinal damage, gut dysbiosis, and behavioral changes in mice. Int J Mol Sci. (2022) 23:5583. doi: 10.3390/ijms23105583
90. Nielsen LN, Roager HM, Casas ME, Frandsen HL, Gosewinkel U, Bester K, et al. Glyphosate has limited short-term effects on commensal bacterial community composition in the gut environment due to sufficient aromatic amino acid levels. Environ Pollut. (2018) 233:364–76. doi: 10.1016/j.envpol.2017.10.016
91. Lloyd-Price J, Arze C, Ananthakrishnan AN, Schirmer M, Avila-Pacheco J, Poon TW, et al. Multi-omics of the gut microbial ecosystem in inflammatory bowel diseases. Nature. (2019) 569:655–62. doi: 10.1038/s41586-019-1237-9
92. McCoin CS, Knotts TA, Adams SH. Acylcarnitines–old actors auditioning for new roles in metabolic physiology. Nat Rev Endocrinol. (2015) 11:617–25. doi: 10.1038/nrendo.2015.129
93. Järup L. Hazards of heavy metal contamination. Br Med Bull. (2003) 68:167–82. doi: 10.1093/bmb/ldg032
94. Tchounwou PB, Yedjou CG, Patlolla AK, Sutton DJ. Heavy metal toxicity and the environment. Exp Suppl. (2012) 101:133–64. doi: 10.1007/978-3-7643-8340-4_6
95. Dietler D, Babu M, Cissé G, Halage AA, Malambala E, Fuhrimann S. Daily variation of heavy metal contamination and its potential sources along the major urban wastewater channel in Kampala, Uganda. Environ Monit Assess. (2019) 191:52. doi: 10.1007/s10661-018-7175-4
96. Shifaw E. Review of heavy metals pollution in china in agricultural and urban soils. J Health Pollut. (2018) 8:180607. doi: 10.5696/2156-9614-8.18.180607
97. Kumar V, Sharma A, Kaur P, Singh Sidhu GP, Bali AS, Bhardwaj R, et al. Pollution assessment of heavy metals in soils of india and ecological risk assessment: a state-of-the-art. Chemosphere. (2019) 216:449–62. doi: 10.1016/j.chemosphere.2018.10.066
98. Shammi SA, Salam A, Khan MAH. Assessment of heavy metal pollution in the agricultural soils, plants, and in the atmospheric particulate matter of a suburban industrial region in Dhaka, Bangladesh. Environ Monit Assess. (2021) 193:104. doi: 10.1007/s10661-021-08848-y
99. Suvarapu LN, Baek SO. Determination of heavy metals in the ambient atmosphere. Toxicol Ind Health. (2017) 33:79–96. doi: 10.1177/0748233716654827
100. Sall ML, Diaw AKD, Gningue-Sall D, Efremova Aaron S, Aaron JJ. Toxic heavy metals: impact on the environment and human health, and treatment with conducting organic polymers, a review. Environ Sci Pollut Res Int. (2020) 27:29927–42. doi: 10.1007/s11356-020-09354-3
101. He ZL, Yang XE, Stoffella PJ. Trace elements in agroecosystems and impacts on the environment. J Trace Elem Med Biol. (2005) 19:125–40. doi: 10.1016/j.jtemb.2005.02.010
102. Shallari S, Schwartz C, Hasko A, Morel JL. Heavy metals in soils and plants of serpentine and industrial sites of Albania. Sci Total Environ. (1998) 209:133–42. doi: 10.1016/S0048-9697(98)80104-6
103. Briffa J, Sinagra E, Blundell R. Heavy metal pollution in the environment and their toxicological effects on humans. Heliyon. (2020) 6:e04691. doi: 10.1016/j.heliyon.2020.e04691
104. Witkowska D, Słowik J, Chilicka K. Heavy metals and human health: possible exposure pathways and the competition for protein binding sites. Molecules. (2021) 26:6060. doi: 10.3390/molecules26196060
105. Ye F, Li X, Li F, Li J, Chang W, Yuan J, et al. Cyclosporin a protects against lead neurotoxicity through inhibiting mitochondrial permeability transition pore opening in nerve cells. Neurotoxicology. (2016) 57:203–13. doi: 10.1016/j.neuro.2016.10.004
106. Ladizinski B, Mistry N, Kundu RV. Widespread use of toxic skin lightening compounds: medical and psychosocial aspects. Dermatol Clin. (2011) 29:111–23. doi: 10.1016/j.det.2010.08.010
107. Siewit CL, Gengler B, Vegas E, Puckett R, Louie MC. Cadmium promotes breast cancer cell proliferation by potentiating the interaction between eralpha and C-Jun. Mol Endocrinol. (2010) 24:981–92. doi: 10.1210/me.2009-0410
108. Copan L, Fowles J, Barreau T, McGee N. Mercury toxicity and contamination of households from the use of skin creams adulterated with mercurous chloride (calomel). Int J Environ Res Public Health. (2015) 12:10943–54. doi: 10.3390/ijerph120910943
109. Xia J, Lu L, Jin C, Wang S, Zhou J, Ni Y, et al. Effects of short term lead exposure on gut microbiota and hepatic metabolism in adult zebrafish. Comp Biochem Physiol C Toxicol Pharmacol. (2018) 209:1–8. doi: 10.1016/j.cbpc.2018.03.007
110. Choi YJ, Seelbach MJ, Pu H, Eum SY, Chen L, Zhang B, et al. Polychlorinated biphenyls disrupt intestinal integrity via nadph oxidase-induced alterations of tight junction protein expression. Environ Health Perspect. (2010) 118:976–81. doi: 10.1289/ehp.0901751
111. Mitchell J, Kim SJ, Howe C, Lee S, Her JY, Patel M, et al. Chronic intestinal inflammation suppresses brain activity by inducing neuroinflammation in mice. Am J Pathol. (2022) 192:72–86. doi: 10.1016/j.ajpath.2021.09.006
112. Zhong G, Wan F, Lan J, Jiang X, Wu S, Pan J, et al. Arsenic exposure induces intestinal barrier damage and consequent activation of gut-liver axis leading to inflammation and pyroptosis of liver in ducks. Sci Total Environ. (2021) 788:147780. doi: 10.1016/j.scitotenv.2021.147780
113. Breton J, Daniel C, Vignal C, Body-Malapel M, Garat A, Plé C, et al. Does oral exposure to cadmium and lead mediate susceptibility to colitis? the dark-and-bright sides of heavy metals in gut ecology. Sci Rep. (2016) 6:19200. doi: 10.1038/srep19200
114. Zhao Y, Zhou C, Wu C, Guo X, Hu G, Wu Q, et al. Subchronic oral mercury caused intestinal injury and changed gut microbiota in mice. Sci Total Environ. (2020) 721:137639. doi: 10.1016/j.scitotenv.2020.137639
115. Zhao Y, Zhou C, Guo X, Hu G, Li G, Zhuang Y, et al. Exposed to mercury-induced oxidative stress, changes of intestinal microflora, and association between them in mice. Biol Trace Elem Res. (2021) 199:1900–7. doi: 10.1007/s12011-020-02300-x
116. Seki N, Akiyama M, Yamakawa H, Hase K, Kumagai Y, Kim YG. Adverse effects of methylmercury on gut bacteria and accelerated accumulation of mercury in organs due to disruption of gut microbiota. J Toxicol Sci. (2021) 46:91–7. doi: 10.2131/jts.46.91
117. Pratush A, Kumar A, Hu Z. Adverse effect of heavy metals (as, Pb, Hg, and Cr) on health and their bioremediation strategies: a review. Int Microbiol. (2018) 21:97–106. doi: 10.1007/s10123-018-0012-3
118. Rai PK, Lee SS, Zhang M, Tsang YF, Kim KH. Heavy metals in food crops: health risks, fate, mechanisms, and management. Environ Int. (2019) 125:365–85. doi: 10.1016/j.envint.2019.01.067
119. Zhou F, Yin G, Gao Y, Ouyang L, Liu S, Jia Q, et al. Insights into cognitive deficits caused by low-dose toxic heavy metal mixtures and their remediation through a postnatal enriched environment in rats. J Hazard Mater. (2020) 388:122081. doi: 10.1016/j.jhazmat.2020.122081
120. Annabi Berrahal A, Nehdi A, Hajjaji N, Gharbi N, El-Fazaa S. Antioxidant enzymes activities and bilirubin level in adult rat treated with lead. C R Biol. (2007) 330:581–8. doi: 10.1016/j.crvi.2007.05.007
121. Stojsavljević A, Sokić-Milutinović A, Rovčanin B, Tončev L, Manojlović D. Profiling of circulatory elements reveals alteration of essential and toxic trace metals in Crohn's disease. Biol Trace Elem Res. (2022) 200:2572–80. doi: 10.1007/s12011-021-02862-4
122. Ogasawara H, Hayasaka M, Maemoto A, Furukawa S, Ito T, Kimura O, et al. Levels of major and trace metals in the scalp hair of Crohn's disease patients: Correlations among transition metals. Biometals. (2021) 34:197–210. doi: 10.1007/s10534-020-00272-y
123. Breton J, Daniel C, Dewulf J, Pothion S, Froux N, Sauty M, et al. Gut microbiota limits heavy metals burden caused by chronic oral exposure. Toxicol Lett. (2013) 222:132–8. doi: 10.1016/j.toxlet.2013.07.021
124. Chi L, Xue J, Tu P, Lai Y, Ru H, Lu K. Gut microbiome disruption altered the biotransformation and liver toxicity of arsenic in mice. Arch Toxicol. (2019) 93:25–35. doi: 10.1007/s00204-018-2332-7
125. Cordier S, Grasmick C, Paquier-Passelaigue M, Mandereau L, Weber JP, Jouan M. Mercury exposure in french guiana: levels and determinants. Arch Environ Health. (1998) 53:299–303. doi: 10.1080/00039899809605712
126. O'Neal SL, Zheng W. Manganese toxicity upon overexposure: a decade in review. Curr Environ Health Rep. (2015) 2:315–28. doi: 10.1007/s40572-015-0056-x
127. Martins AC, Krum BN, Queirós L, Tinkov AA, Skalny AV, Bowman AB, et al. Manganese in the diet: bioaccessibility, adequate intake, and neurotoxicological effects. J Agric Food Chem. (2020) 68:12893–903. doi: 10.1021/acs.jafc.0c00641
128. Stochel-Gaudyn A, Fyderek K, Kościelniak P. Serum trace elements profile in the pediatric inflammatory bowel disease progress evaluation. J Trace Elem Med Biol. (2019) 55:121–6. doi: 10.1016/j.jtemb.2019.06.016
129. Cho JM, Yang HR. Hair mineral and trace element contents as reliable markers of nutritional status compared to serum levels of these elements in children newly diagnosed with inflammatory bowel disease. Biol Trace Elem Res. (2018) 185:20–9. doi: 10.1007/s12011-017-1225-6
130. Song Y, Liu Y, Teo HY, Hanafi ZB, Mei Y, Zhu Y, et al. Manganese enhances the antitumor function of Cd8(+) T cells by inducing type I interferon production. Cell Mol Immunol. (2021) 18:1571–4. doi: 10.1038/s41423-020-00524-4
131. Chen G, Yu Y, Fu X, Wang G, Wang Z, Wu X, et al. Microfluidic encapsulated manganese organic frameworks as enzyme mimetics for inflammatory bowel disease treatment. J Colloid Interface Sci. (2022) 607(Pt 2):1382–90. doi: 10.1016/j.jcis.2021.09.016
132. Qiu H, Gong H, Bao Y, Jiang H, Tong W. Reactive oxygen species-scavenging hollow Mno(2) nanozymes as carriers to deliver budesonide for synergistic inflammatory bowel disease Therapy. Biomater Sci. (2022) 10:457–66. doi: 10.1039/D1BM01525G
133. Li W, Liu Z, Liu C, Guan Y, Ren J, Qu X. Manganese dioxide nanozymes as responsive cytoprotective shells for individual living cell encapsulation. Angew Chem Int Ed Engl. (2017) 56:13661–5. doi: 10.1002/anie.201706910
134. Missimer TM, Teaf CM, Beeson WT, Maliva RG, Woolschlager J, Covert DJ. Natural background and anthropogenic arsenic enrichment in florida soils, surface water, and groundwater: a review with a discussion on public health risk. Int J Environ Res Public Health. (2018) 15:2278. doi: 10.3390/ijerph15102278
135. Hughes MF, Beck BD, Chen Y, Lewis AS, Thomas DJ. Arsenic exposure and toxicology: a historical perspective. Toxicol Sci. (2011) 123:305–32. doi: 10.1093/toxsci/kfr184
136. Rehman K, Fatima F, Waheed I, Akash MSH. Prevalence of exposure of heavy metals and their impact on health consequences. J Cell Biochem. (2018) 119:157–84. doi: 10.1002/jcb.26234
137. Wang C-H, Hsiao CK, Chen C-L, Hsu L-I, Chiou H-Y, Chen S-Y, et al. A review of the epidemiologic literature on the role of environmental arsenic exposure and cardiovascular diseases. Toxicol Appl Pharmacol. (2007) 222:315–26. doi: 10.1016/j.taap.2006.12.022
138. Civantos DP, López Rodríguez A, Aguado-Borruey JM, Narvaez JA. Fulminant malignant arrythmia and multiorgan failure in acute arsenic poisoning. Chest. (1995) 108:1774–5. doi: 10.1378/chest.108.6.1774-a
139. Chen Y, Parvez F, Gamble M, Islam T, Ahmed A, Argos M, et al. Arsenic exposure at low-to-moderate levels and skin lesions, arsenic metabolism, neurological functions, and biomarkers for respiratory and cardiovascular diseases: review of recent findings from the health effects of arsenic longitudinal study (heals) in Bangladesh. Toxicol Appl Pharmacol. (2009) 239:184–92. doi: 10.1016/j.taap.2009.01.010
140. Palma-Lara I, Martinez-Castillo M, Quintana-Perez JC, Arellano-Mendoza MG, Tamay-Cach F, Valenzuela-Limon OL, et al. Arsenic exposure: a public health problem leading to several cancers. Regul Toxicol Pharmacol. (2020) 110:104539. doi: 10.1016/j.yrtph.2019.104539
141. Chiocchetti GM, Domene A, Kühl AA, Zúñiga M, Vélez D, Devesa V, et al. In vivo evaluation of the effect of arsenite on the intestinal epithelium and associated microbiota in mice. Arch Toxicol. (2019) 93:2127–39. doi: 10.1007/s00204-019-02510-w
142. Slifer ZM, Blikslager AT. The integral role of tight junction proteins in the repair of injured intestinal epithelium. Int J Mol Sci. (2020) 21:972. doi: 10.3390/ijms21030972
143. Xie S-Z, Liu B, Ye H-Y, Li Q-M, Pan L-H, Zha X-Q, et al. Dendrobium huoshanense polysaccharide regionally regulates intestinal mucosal barrier function and intestinal microbiota in mice. Carbohydr Polym. (2019) 206:149–62. doi: 10.1016/j.carbpol.2018.11.002
144. Moulahoum H, Boumaza BMA, Ferrat M, Bounaama A, Djerdjouri B. Arsenic trioxide ameliorates murine colon inflammation through inflammatory cell enzymatic modulation. Naunyn Schmiedebergs Arch Pharmacol. (2019) 392:259–70. doi: 10.1007/s00210-018-1578-1
145. Zhang S, Jin Y, Zeng Z, Liu Z, Fu Z. Subchronic exposure of mice to cadmium perturbs their hepatic energy metabolism and gut microbiome. Chem Res Toxicol. (2015) 28:2000–9. doi: 10.1021/acs.chemrestox.5b00237
146. Junejo SH, Baig JA, Kazi TG, Afridi HI. Cadmium and lead hazardous impact assessment of pond fish species. Biol Trace Elem Res. (2019) 191:502–11. doi: 10.1007/s12011-018-1628-z
147. Ninkov M, Popov Aleksandrov A, Demenesku J, Mirkov I, Mileusnic D, Petrovic A, et al. Toxicity of oral cadmium intake: impact on gut immunity. Toxicol Lett. (2015) 237:89–99. doi: 10.1016/j.toxlet.2015.06.002
148. Zhai Q, Tian F, Zhao J, Zhang H, Narbad A, Chen W. Oral administration of probiotics inhibits absorption of the heavy metal cadmium by protecting the intestinal barrier. Appl Environ Microbiol. (2016) 82:4429–40. doi: 10.1128/AEM.00695-16
149. Cox JN, Rahman MA, Bao S, Liu M, Wheeler SE, Knoell DL. Cadmium attenuates the macrophage response to lps through inhibition of the Nf-Kb pathway. Am J Physiol Lung Cell Mol Physiol. (2016) 311:L754–65. doi: 10.1152/ajplung.00022.2016
150. Clarkson TW, Magos L. The toxicology of mercury and its chemical compounds. Crit Rev Toxicol. (2006) 36:609–62. doi: 10.1080/10408440600845619
151. Larose C, Canuel R, Lucotte M, Di Giulio RT. Toxicological effects of methylmercury on walleye (Sander vitreus) and perch (Perca flavescens) from lakes of the boreal forest. Comp Biochem Physiol C Toxicol Pharmacol. (2008) 147:139–49. doi: 10.1016/j.cbpc.2007.09.002
152. Webb J. Use of the ecosystem approach to population health: the case of mercury contamination in aquatic environments and Riparian populations, Andean Amazon, Napo River Valley, Ecuador. Can J Public Health. (2005) 96:44–6. doi: 10.1007/BF03404015
153. Liu B, Yu H, Baiyun R, Lu J, Li S, Bing Q, et al. Protective effects of dietary luteolin against mercuric chloride-induced lung injury in mice: involvement of Akt/Nrf2 and Nf-Kb pathways. Food Chem Toxicol. (2018) 113:296–302. doi: 10.1016/j.fct.2018.02.003
154. Ye F, Li X, Li L, Lyu L, Yuan J, Chen J. The role of Nrf2 in protection against Pb-induced oxidative stress and apoptosis in Sh-Sy5y cells. Food Chem Toxicol. (2015) 86:191–201. doi: 10.1016/j.fct.2015.10.009
155. Vieira HC, Bordalo MD, Rodrigues ACM, Pires SFS, Rocha RJM, Soares A, et al. Water temperature modulates mercury accumulation and oxidative stress status of common goby (Pomatoschistus microps). Environ Res. (2021) 193:110585. doi: 10.1016/j.envres.2020.110585
156. Wang G, Fowler BA. Roles of biomarkers in evaluating interactions among mixtures of lead, cadmium and arsenic. Toxicol Appl Pharmacol. (2008) 233:92–9. doi: 10.1016/j.taap.2008.01.017
157. Soares FA, Farina M, Santos FW, Souza D, Rocha JB, Nogueira CW. Interaction between metals and chelating agents affects glutamate binding on brain synaptic membranes. Neurochem Res. (2003) 28:1859–65. doi: 10.1023/a:1026175825871
158. Duan C, Fang L, Yang C, Chen W, Cui Y, Li S. Reveal the response of enzyme activities to heavy metals through in situ zymography. Ecotoxicol Environ Saf. (2018) 156:106–15. doi: 10.1016/j.ecoenv.2018.03.015
159. Singh RP, Agrawal M. Potential benefits and risks of land application of sewage sludge. Waste Manag. (2008) 28:347–58. doi: 10.1016/j.wasman.2006.12.010
160. Pathak A, Dastidar MG, Sreekrishnan TR. Bioleaching of heavy metals from sewage sludge: a review. J Environ Manag. (2009) 90:2343–53. doi: 10.1016/j.jenvman.2008.11.005
161. Achal V, Pan X, Fu Q, Zhang D. Biomineralization based remediation of as(III) contaminated soil by sporosarcina ginsengisoli. J Hazard Mater. (2012) 201–2:178–84. doi: 10.1016/j.jhazmat.2011.11.067
162. Li M, Cheng X, Guo H. Heavy metal removal by biomineralization of urease producing bacteria isolated from soil. Int Biodeterior Biodegrad. (2013) 76:81–5. doi: 10.1016/j.ibiod.2012.06.016
163. Huang HM, Pai MH, Liu JJ, Yeh SL, Hou YC. Effects of dietary exposure to chlorpyrifos on immune cell populations and inflammatory responses in mice with dextran sulfate sodium-induced colitis. Food Chem Toxicol. (2019) 131:110596. doi: 10.1016/j.fct.2019.110596
164. Luo T, Wang X, Jin Y. Low concentrations of imidacloprid exposure induced gut toxicity in adult zebrafish (Danio rerio). Comp Biochem Physiol C Toxicol Pharmacol. (2021) 241:108972. doi: 10.1016/j.cbpc.2020.108972
165. Fu Z, Han F, Huang K, Zhang J, Qin JG, Chen L, et al. Impact of imidacloprid exposure on the biochemical responses, transcriptome, gut microbiota and growth performance of the pacific white shrimp Litopenaeus vannamei. J Hazard Mater. (2022) 424(Pt B):127513. doi: 10.1016/j.jhazmat.2021.127513
166. Tian Y, Gui W, Rimal B, Koo I, Smith PB, Nichols RG, et al. Metabolic impact of persistent organic pollutants on gut microbiota. Gut Microbes. (2020) 12:1–16. doi: 10.1080/19490976.2020.1848209
167. Fernandes AR, Falandysz J. Polybrominated dibenzo-P-dioxins and furans (Pbdd/Fs): contamination in food, humans and dietary exposure. Sci Total Environ. (2021) 761:143191. doi: 10.1016/j.scitotenv.2020.143191
168. Li J, Li Y, Sha R, Zheng L, Xu L, Xie HQ, et al. Effects of perinatal tcdd exposure on colonic microbiota and metabolism in offspring and mother mice. Sci Total Environ. (2022) 832:154762. doi: 10.1016/j.scitotenv.2022.154762
169. Ge M, Wang X, Yang G, Wang Z, Li Z, Zhang X, et al. Persistent organic pollutants (pops) in deep-sea sediments of the tropical western pacific ocean. Chemosphere. (2021) 277:130267. doi: 10.1016/j.chemosphere.2021.130267
170. El-Nahhal Y, El-Nahhal I. Cardiotoxicity of some pesticides and their amelioration. Environ Sci Pollut Res Int. (2021) 28:44726–54. doi: 10.1007/s11356-021-14999-9
171. Knauer K, Homazava N, Junghans M, Werner I. The influence of particles on bioavailability and toxicity of pesticides in surface water. Integr Environ Assess Manag. (2017) 13:585–600. doi: 10.1002/ieam.1867
172. Noren E, Lindh C, Rylander L, Glynn A, Axelsson J, Littorin M, et al. Concentrations and temporal trends in pesticide biomarkers in urine of swedish adolescents, 2000–2017. J Expo Sci Environ Epidemiol. (2020) 30:756–67. doi: 10.1038/s41370-020-0212-8
173. Hurtado-Barroso S, Tresserra-Rimbau A, Vallverdu-Queralt A, Lamuela-Raventos RM. Organic food and the impact on human health. Crit Rev Food Sci Nutr. (2019) 59:704–14. doi: 10.1080/10408398.2017.1394815
174. Yuan X, Pan Z, Jin C, Ni Y, Fu Z, Jin Y. Gut microbiota: an underestimated and unintended recipient for pesticide-induced toxicity. Chemosphere. (2019) 227:425–34. doi: 10.1016/j.chemosphere.2019.04.088
175. Liang Y, Zhan J, Liu D, Luo M, Han J, Liu X, et al. Organophosphorus pesticide chlorpyrifos intake promotes obesity and insulin resistance through impacting gut and gut microbiota. Microbiome. (2019) 7:19. doi: 10.1186/s40168-019-0635-4
176. Mahai G, Wan Y, Xia W, Wang A, Qian X, Li Y, et al. Exposure assessment of neonicotinoid insecticides and their metabolites in Chinese women during pregnancy: a longitudinal study. Sci Total Environ. (2022) 818:151806. doi: 10.1016/j.scitotenv.2021.151806
177. Wang A, Wan Y, Zhou L, Xia W, Guo Y, Mahai G, et al. Neonicotinoid insecticide metabolites in seminal plasma: associations with semen quality. Sci Total Environ. (2022) 811:151407. doi: 10.1016/j.scitotenv.2021.151407
178. Walderdorff L, Laval-Gilly P, Wechtler L, Bonnefoy A, Falla-Angel J. Phagocytic activity of human macrophages and drosophila hemocytes after exposure to the neonicotinoid imidacloprid. Pestic Biochem Physiol. (2019) 160:95–101. doi: 10.1016/j.pestbp.2019.07.007
179. Jones KC. Persistent Organic Pollutants (Pops) and Related Chemicals in the Global Environment: Some Personal Reflections. Environ Sci Technol. (2021) 55:9400–12. doi: 10.1021/acs.est.0c08093
180. Castro-Jimenez J, Banaru D, Chen CT, Jimenez B, Munoz-Arnanz J, Deviller G, et al. Persistent organic pollutants burden, trophic magnification and risk in a pelagic food web from coastal Nw Mediterranean Sea. Environ Sci Technol. (2021) 55:9557–68. doi: 10.1021/acs.est.1c00904
181. Carpenter DO. Polychlorinated biphenyls (Pcbs): routes of exposure and effects on human health. Rev Environ Health. (2006) 21:1–23. doi: 10.1515/REVEH.2006.21.1.1
182. Mao S, Liu S, Zhou Y, An Q, Zhou X, Mao Z, et al. The occurrence and sources of polychlorinated biphenyls (Pcbs) in agricultural soils across China with an emphasis on unintentionally produced Pcbs. Environ Pollut. (2021) 271:116171. doi: 10.1016/j.envpol.2020.116171
183. Ravanipour M, Nabipour I, Yunesian M, Rastkari N, Mahvi AH. Exposure Sources of polychlorinated biphenyls (Pcbs) and health risk assessment: a systematic review in Iran. Environ Sci Pollut Res Int. (2022) 29:55437–56. doi: 10.1007/s11356-022-21274-y
184. Lü H, Cai Q-Y, Jones KC, Zeng Q-Y, Katsoyiannis A. Levels of organic pollutants in vegetables and human exposure through diet: a review. Crit Rev Environ Sci Technol. (2013) 44:1–33. doi: 10.1080/10643389.2012.710428
185. Wang S-L, Tsai P-C, Yang C-Y, Leon Guo Y. Increased risk of diabetes and polychlorinated biphenyls and dioxins: a 24-year follow-up study of the yucheng cohort. Diabetes Care. (2008) 31:1574–9. doi: 10.2337/dc07-2449
186. Fernández-González R, Yebra-Pimentel I, Martínez-Carballo E, Simal-Gándara J. A critical review about human exposure to polychlorinated dibenzo-P-dioxins (Pcdds), polychlorinated dibenzofurans (pcdfs) and polychlorinated biphenyls (Pcbs) through foods. Crit Rev Food Sci Nutr. (2015) 55:1590–617. doi: 10.1080/10408398.2012.710279
187. Hennig B, Meerarani P, Slim R, Toborek M, Daugherty A, Silverstone AE, et al. Proinflammatory properties of coplanar Pcbs: in vitro and in vivo evidence. Toxicol Appl Pharmacol. (2002) 181:174–83. doi: 10.1006/taap.2002.9408
188. Leijs M, Fietkau K, Merk HF, Schettgen T, Kraus T, Esser A. Upregulation of Ccl7, Ccl20, Cxcl2, Il-1β, Il-6 and Mmp-9 in skin samples of Pcb exposed individuals-a preliminary study. Int J Environ Res Public Health. (2021) 18:9711. doi: 10.3390/ijerph18189711
189. Leijs MM, Esser A, Amann PM, Schettgen T, Heise R, Fietkau K, et al. Expression of Cyp1a1, Cyp1b1 and Il-1β in Pbmcs and skin samples of Pcb exposed individuals. Sci Total Environ. (2018) 642:1429–38. doi: 10.1016/j.scitotenv.2018.06.136
190. Min L, Chi Y, Dong S. Gut microbiota health closely associates with Pcb153-derived risk of host diseases. Ecotoxicol Environ Saf. (2020) 203:111041. doi: 10.1016/j.ecoenv.2020.111041
191. Rude KM, Pusceddu MM, Keogh CE, Sladek JA, Rabasa G, Miller EN, et al. Developmental exposure to polychlorinated biphenyls (Pcbs) in the maternal diet causes host-microbe defects in weanling offspring mice. Environ Pollut. (2019) 253:708–21. doi: 10.1016/j.envpol.2019.07.066
192. Vidali MS, Dailianis S, Vlastos D, Georgiadis P. Pcb cause global DNA hypomethylation of human peripheral blood monocytes in vitro. Environ Toxicol Pharmacol. (2021) 87:103696. doi: 10.1016/j.etap.2021.103696
193. Patrizi B, Siciliani de Cumis M. Tcdd toxicity mediated by epigenetic mechanisms. Int J Mol Sci. (2018) 19:4101. doi: 10.3390/ijms19124101
194. Popli S, Badgujar PC, Agarwal T, Bhushan B, Mishra V. Persistent organic pollutants in foods, their interplay with gut microbiota and resultant toxicity. Sci Total Environ. (2022) 832:155084. doi: 10.1016/j.scitotenv.2022.155084
195. Sorg O, Zennegg M, Schmid P, Fedosyuk R, Valikhnovskyi R, Gaide O, et al. 2,3,7,8-tetrachlorodibenzo-P-dioxin (Tcdd) poisoning in victor yushchenko: identification and measurement of Tcdd metabolites. Lancet. (2009) 374:1179–85. doi: 10.1016/S0140-6736(09)60912-0
196. Li Y, Xie HQ, Zhang W, Wei Y, Sha R, Xu L, et al. Type 3 innate lymphoid cells are altered in colons of C57bl/6 mice with dioxin exposure. Sci Total Environ. (2019) 662:639–45. doi: 10.1016/j.scitotenv.2019.01.139
197. Sonnenberg GF, Artis D. Innate lymphoid cells in the initiation, regulation and resolution of inflammation. Nat Med. (2015) 21:698–708. doi: 10.1038/nm.3892
Keywords: inflammatory bowel disease, emerging contaminant (EC), exposome, adverse health effects (AHEs), gut dysbiosis
Citation: Chen X, Wang S, Mao X, Xiang X, Ye S, Chen J, Zhu A, Meng Y, Yang X, Peng S, Deng M and Wang X (2023) Adverse health effects of emerging contaminants on inflammatory bowel disease. Front. Public Health 11:1140786. doi: 10.3389/fpubh.2023.1140786
Received: 09 January 2023; Accepted: 06 February 2023;
Published: 24 February 2023.
Edited by:
Qun Xu, Chinese Academy of Medical Sciences and Peking Union Medical College, ChinaReviewed by:
Bingqing Zhao, Stanford University, United StatesCopyright © 2023 Chen, Wang, Mao, Xiang, Ye, Chen, Zhu, Meng, Yang, Peng, Deng and Wang. This is an open-access article distributed under the terms of the Creative Commons Attribution License (CC BY). The use, distribution or reproduction in other forums is permitted, provided the original author(s) and the copyright owner(s) are credited and that the original publication in this journal is cited, in accordance with accepted academic practice. No use, distribution or reproduction is permitted which does not comply with these terms.
*Correspondence: Minzi Deng, ZGVuZ216QGNzdS5lZHUuY24=; Xiaoyan Wang,
d2FuZ3hpYW95YW5AY3N1LmVkdS5jbg==
†These authors have contributed equally to this work and share first authorship
Disclaimer: All claims expressed in this article are solely those of the authors and do not necessarily represent those of their affiliated organizations, or those of the publisher, the editors and the reviewers. Any product that may be evaluated in this article or claim that may be made by its manufacturer is not guaranteed or endorsed by the publisher.
Research integrity at Frontiers
Learn more about the work of our research integrity team to safeguard the quality of each article we publish.