- 1Faculty of Medicine and Health Sciences, Universiti Sains Islam Malaysia, Nilai, Malaysia
- 2Faculty of Health Sciences, Universiti Teknologi Majlis Amanah Rakyat(MARA), Shah Alam, Malaysia
- 3Institute of Medical Science Technology, Universiti Kuala Lumpur, Kajang, Malaysia
- 4Department of Human & Clinical Anatomy, College of Medicine and Health Sciences, Muscat, Oman
In several regions of the world, the recent Coronavirus Disease-2019 (COVID-19) pandemic outbreak increased morbidity and mortality. The pandemic situation disrupted many workers' previously established lifestyles. The main aim of the present review was to describe the circadian disruption and occupational toxicant exposure affecting the immunity of shift workers during the SARS CoV-2 pandemic. We retrieved pertinent published literature from the Google Scholar, PubMed, and Scopus databases. In the present review, we discuss the circadian rhythm involving the hypothalamic-pituitary-adrenal (HPA) axis at the molecular level, its disruption, occupational toxicant exposure causing immunomodulatory effects, and the role of immunity during the SARS CoV-2 pandemic. The severity of the progression of the viral infection depends on multiple factors affecting immunity. Hence, shift workers may need to be aware of those factors such as circadian rhythm disruption as well as occupational toxicant exposure. The timing of shift workers' energy intake is also important concerning the shift of the workers. The information in the present review may be important for all workers who are at risk during the pandemic. In the absence of any published literature related to association of circadian rhythm disruption with occupational toxicant exposure, the present review may have greater importance.
Introduction
A cluster case of pneumonia with an unknown origin was reported in Wuhan, Hubei Province, China, at the end of December 2019 (1). Through isolation of the causative agent utilizing human airway epithelial cells from affected individuals, a novel coronavirus known as 2019-nCov (SARS-CoV 2) was found (2). The World Health Organization (WHO) formally termed it as unique Coronavirus Disease-2019 (COVID-19) on 11 February 2020. It was later declared as a worldwide pandemic when mortality and morbidity increased worldwide (3).
According to the WHO database, the total number of cases worldwide was 162,184,263 as of 16 May 2021, with a total number of 3,364,446 deaths (4). South-East Asia (53%) had the highest number of new cases in the seven days preceding the report, followed by the Americas (25%) (4). Meanwhile, there were 470,110 cases in Malaysia, with 1,902 deaths (4). Selangor had the highest confirmed cases (153,663), followed by Sabah (59,349) and Johor (50,419) (5). Despite non-pharmacological prophylactic measures implemented by the government, such as social distancing, mandatory rules on wearing masks in public areas, quarantine, and movement control order/restriction (6), the numbers continue to rise.
The majority of COVID-19 clusters found in a South-East Asian country like Malaysia were linked to workplace clusters, accounting for 52.3% of the cases (7). As a result, it is critical to comprehend the circumstances that influenced these workers' immunological status during the current pandemic. Workers in the healthcare and manufacturing industries were the most exposed to the virus (8, 9). While some of them worked during regular office hours, most of them were shift workers who worked on one of three shifts i.e. morning shift, evening shift, or night shift.
Immunity refers to the body's defense against any external organisms or toxins. Immunity is often compromised in shift workers. The severity of any infection depends on the immune status of the individual. The immune status can be affected by multiple factors, such as circadian disruption and exposure to certain toxicants. Keeping the above facts in mind, we focused on the circadian rhythm involving the hypothalamic-pituitary-adrenal (HPA) axis and its disruption, workplace toxicants that influence workers, and the function of immunity during the COVID-19 pandemic.
Materials and Methods
This was a narrative review. An extant literature search for relevant articles was conducted in May 2021 using three databases: Google Scholar, PubMed, and Scopus. Although it was a narrative review, the studies were identified based on information available in the title, abstract, and keywords. Relevant search terms included “circadian rhythm,” “shift work,” “occupational toxicants,” “COVID-19,” “shift work and circadian rhythm,” “immunity and COVID-19,” “circadian rhythm and immunity,” “occupational toxicants and immunity.”
We took into account all publications from 2011 until 2021. However, as COVID-19 began in 2019, all papers related to COVID-19 were limited from 2019 until 2021. One hundred seven studies out of 115 were included and were limited to publications in the English language.
The literature search was conducted by SHMF, NASMA, NIMFT, and SA. Articles were only accepted if all reviewers agreed that the article was relevant and of high quality. Any disagreement regarding the selection of studies was resolved by consensus. If necessary, 2nd, 6th, and 7th authors (NJ, IFA, and SD) were also consulted.
Results and Discussion
The results and discussion section depicted the spread of COVID-19, circadian rhythm and disruption, the role of body immunity and occupational toxicant exposure.
Spread of COVID-19 Infection
The SARS-CoV-2 virus belongs to the Coronaviriniae family and is classified as a beta coronavirus. It is a large, enveloped, spherical, single-stranded RNA virus genome measuring around 30 kb (10). It comprises four structural proteins, including nucleocapsid, envelops, membrane, and spike glycoprotein (11). During viral infection, neutralizing antibodies block viral particles from interacting with host cells. This virus exploits its spike glycoprotein to prevent neutralizing antibodies from working, allowing it to penetrate host cells (12). The spikes also prevent infected cells from undergoing apoptosis, ensuring their survival until the virus replication cycle is completed (13).
The transmission of COVID-19 occurs from person to person through respiratory droplets or contact with the infected patient's body fluids, such as saliva, tears, blood, urine, feces, and cerebrospinal fluid, with the mucosal lining of another individual (14). For example, respiratory droplets can be produced when an infected individual talks, coughs, or sneezes. These respiratory droplets can travel within 1 m up to 8 m (15–17). Fomite transmission is also possible as the virus remains alive and infectious on surfaces for up to 4–7 days, depending on the material of the surface (18).
According to multiple reviews, shift workers are at a higher risk of being infected by COVID-19 (19–21). Workers working in shift-work-based roles are reported to be 1.8 to 2 times more likely to be infected by COVID-19 compared to their counterparts (20). The severity predictors of COVID-19 depend on several factors, including age, pre-existing comorbidities, and immunity (22). Older age is associated with higher mortality and certain comorbidities such as cardiovascular disease, chronic kidney disease, chronic respiratory diseases such as asthma and chronic obstructive pulmonary disease, diabetes mellitus, hypertension, immunosuppression, and obesity. These are associated with unfavorable clinical courses, increased risk of intubation, as well as death (23–32). Several studies have concluded that shift workers are at a higher risk of developing these comorbidities, which directly increases the risk of them developing more severe COVID-19 complications (33–37).
Physiology of Circadian Rhythm in the Body, and Its Effect on Immunity Dysfunction
The circadian rhythm, also considered a biological clock, is a system that regulates and facilitates the ability of an individual to respond and adapt to daily changes in the external environment. It includes almost all physiological and behavioral functions in an organism (38, 39). It lasts for about 24 h before another cycle begins, affecting multiple systems, such as the cardiovascular, gastrointestinal, neurological, and immune systems (40–43). The circadian rhythm is reset daily by the master clock in the body, which is located in the suprachiasmatic nucleus (SCN) in the hypothalamus (39). The circadian rhythm regulation happens at the cellular, tissue, and systemic levels. It is synchronized with the environment by synchronizers (external factors) such as light-dark and fast-feed cycles (44). In addition, the master clock/pacemaker in the SCN regulates the peripheral clock using mediators such as hormones and neuronal signals.
Gene expression and protein production, which peak and trough according to the time of day, are regulated at the molecular level. This is accomplished by using two transcription-translation oscillator loops that can up-regulate and down-regulate gene expression in the nucleus and counter-regulate one another, resulting in the 24-h cycle (45–47).
The mammalian clock system revolves around three major physiological components: the input pathway, the master clock itself, and the output pathway (48). The input pathway transmits stimuli, such as light, through the retino-hypothalamic (RHT) tract to the master clock in the SCN. This, in return, transduces into neuronal and hormonal signals used to regulate the peripheral clock (49). In addition, a few elements can affect the circadian cycle, such as the sleep/wake cycle, temperature, fast/feeding cycle, and light/dark cycle (50). Thus, changes to any of these factors can cause misalignment of the circadian rhythm, resulting in circadian disruption.
A significant component regulated by the circadian rhythm is the HPA axis. The HPA axis is an interactive neuro-endocrine unit comprising the hypothalamus, pituitary gland, and adrenal gland (51). This axis is essential for preserving homeostasis, adapting to the external environment, and regulating human emotions and cognitive functions (43). Cortisol and melatonin are some of the most prominent endocrine manifestations of the HPA axis, showing the rhythmic cycle of this biological clock (42, 49). The adrenal glands secreted the cortisol into the bloodstream and reached its peak level in the morning around 7 a.m. until 8 a.m., halved by midnight, and becomes lowest around 2 a.m.−4 a.m. (43). It is known to have many functions in the body, such as metabolism regulation and stress response mediator functions (52). Meanwhile, melatonin is produced by the pineal gland located near the center of the brain, which increases in the evening, with a peak at night and the lowest in the morning (53, 54). Both cortisol and melatonin are deeply related to the sleep/wake cycle as melatonin serves as a time cue for darkness and promotes sleepiness.
In contrast, cortisol has a significant role in initiating wakefulness (43, 55, 56). As melatonin levels decrease with age, older adults are more prone to developing sleep disorders such as insomnia and sleep-related breathing disorders (57). Both these hormones' dysregulation is also associated with chronic illnesses such as obesity and type-2 diabetes mellitus (58–60). Obesity refers to any abnormal accumulation of fat which poses a risk to health, and according to WHO, a body mass index of >30, may be considered obese. Melatonin levels are negatively correlated with insulin and leptin concentrations (54). Impaired cortisol and melatonin signals have disrupted glucose metabolism, resulting in systemic insulin resistance, higher fat mass accumulation, and gaining body weight (61, 62). While studies regarding direct interactions between cortisol/melatonin and immunity or virus' proteins are few, individuals with chronic metabolic disease exhibit weaker immune activity and decreased endogenous antioxidant levels (63). As such, shift workers with circadian disruption are more susceptible to a more severe course of an event during the COVID-19 pandemic (63).
An earlier study showed that cortisol has an anti-inflammatory effect by inducing a decrease in the production of interferon-γ (IFNγ), interleukin-2 (IL-2), and tumor necrosis factor (TNF) as measured by the percentage of CD4+ and CD8+ T-cells, which express these cytokines. However, in circadian disruption, persistent activation of the HPA axis can induce glucocorticoid resistance of immune cells. Although the mechanisms causing glucocorticoid resistance are unclear, one of the possible mechanisms proposed is the accumulation of the dominant-negative β-isoform of the glucocorticoid receptor driven by inflammatory cytokines. Apart from that, psychological and physical stressors trigger the expression of endogenous damage-associated molecular patterns (DAMPs), which in turn activates the NOD-, LRR- and pyrin domain-containing protein 3 (NLRP3) inflammasome. These NLRP3 inflammasomes induce caspase-mediated cleavage of the glucocorticoid receptor, resulting in glucocorticoid resistance. Normally, both inflammatory and antiviral immune response genes (IRGs) are inhibited by cortisol. However, only antiviral IRGs are inhibited in the glucocorticoid resistance state, resulting in decreased expression of antiviral IRGs while increasing the expression of inflammatory IRGs (64). Figure 1 illustrates factors affecting the circadian rhythm and the effect of circadian disruption on different body systems, including cardiovascular, neurological, immunity, and metabolic functions. Figure 2 illustrates the cascading pattern of circadian disruption resulting in glucocorticoid resistance and immunity dysfunction.
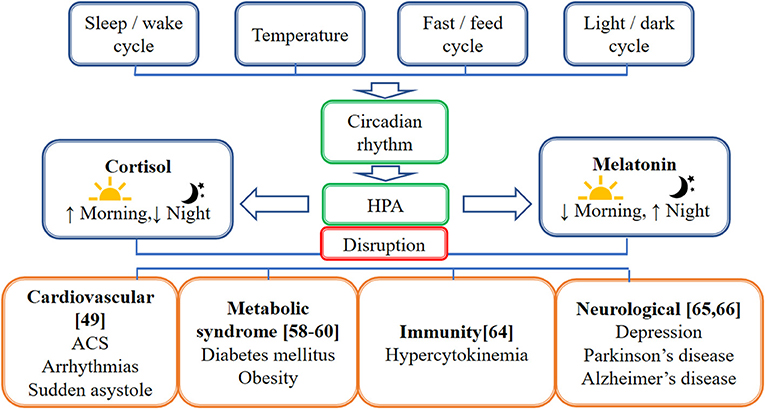
Figure 1. Schematic diagram on the factors affecting the circadian rhythm and the effect of circadian disruption on different body systems. This figure illustrates factors affecting the circadian rhythm which are the sleep/wake cycle, temperature, fast/feed cycle, and light/dark cycle. The hypothalamic-pituitary-adrenal axis (HPA) caused the cortisol to be high in the morning and lower in the night while melatonin is high in the night and lower in the morning. The circadian disruption affects different body systems, including cardiovascular, neurological, immunity, and metabolic functions.
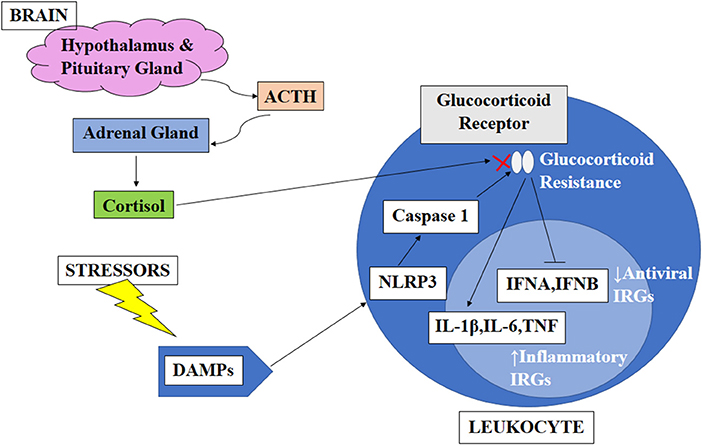
Figure 2. Schematic diagram on the cascading pattern of circadian disruption resulting in glucocorticoid resistance and immunity dysfunction. This figure illustrates the cascading pattern of circadian disruption resulting in glucocorticoid resistance and immunity dysfunction. The brain produces ACTH which caused the adrenal gland to release cortisol which has an anti-inflammatory effect by inducing a decrease in the production of interferon-γ (IFNγ), interleukin-2 (IL-2), and tumor necrosis factor (TNF). However, in circadian disruption, persistent activation of the HPA axis can induce glucocorticoid resistance of immune cells. Apart from that, psychological and physical stressors trigger the expression of endogenous damage-associated molecular patterns (DAMPs), which in turn activates the NOD-, LRR- and pyrin domain-containing protein 3 (NLRP3) inflammasome. These NLRP3 inflammasomes induce caspase-mediated cleavage of the glucocorticoid receptor, resulting in glucocorticoid resistance. Normally, both inflammatory and antiviral immune response genes (IRGs) are inhibited by cortisol. However, only antiviral IRGs are inhibited in the glucocorticoid resistance state, resulting in decreased expression of antiviral IRGs while increasing the expression of inflammatory IRGs (64).
Shift workers are at a higher risk of developing diet-related chronic illnesses such as obesity, type 2 diabetes mellitus, and cardiovascular disease compared to non-shift workers (65–67). About 70% of shift workers are overweight/obese (67). This is due to the disruption of circadian rhythm, as discussed before, which is also attributed to their poor food habits. Shift workers are more prone to eating on a 24 h basis compared to non-shift workers, who usually eat three meals a day, with the food mostly being consumed during the daytime (49). The sleep constraint also increases the levels of leptin and ghrelin, thus increasing appetite at night. Apart from hunger, other factors also drive shift workers to eat at night, such as break and time availability, social pressure to eat with colleagues, avoiding gastric upset, stress eating, and a means to stay awake and prevent sleepiness (68). During overnight shifts, the circadian rhythm that regulates the body's internal processes is disrupted, which in turn causes dysregulation of the energy metabolism and promotes weight gain. The body is in a fasting state during night sleep, promoting the release of stored glucose as well as lower insulin sensitivity and insulin secretion compared to the daytime. As such, eating at night when the body is programmed to be in a sleeping state disrupts the metabolic processing standard (69).
Occupational Toxicants Exposure in the Workplace and Its Effect on the Immune System
Occupational hygiene focuses on eliminating and controlling chemical, physical, or biological hazards. These hazards come in many forms, such as hazardous chemicals, gases, vapors, mist, dust, smoke, fumes, fibers, noise, vibration, heat, cold, ionizing radiation, non-ionizing radiation, and biological hazards such as bacteria, viruses, mold, or fungi. These workplace hazards are usually slow, and their effects are insidious on workers' health. There is an estimation of 2.4 million deaths linked to work-related illnesses annually throughout the world (70). Some of these toxicants have a detrimental effect on the immune system. In this pandemic, the industries with the highest exposure to COVID-19 are the factory and healthcare industries. The common types of occupational toxicants affecting the immune system are found in both industries, as summarized in Table 1.
Role of Body Immunity During SARS-CoV-2 Infection
The human body has the ability, called immunity, to resist almost all types of organisms or toxins, as well as tend to damaged tissues and organs. Classically, the body's immune response is divided into innate and adaptive immunity. While innate immunity responds rapidly and non-specifically toward pathogens, adaptive immunity reacts slower but in a specific manner, producing a long-lived immunological memory (77). Furthermore, as the circadian rhythm regulates almost all aspects of physiological and behavioral response, the immune system is not excluded from this response and fluctuates daily (45). As such, the circadian rhythm regulates both innate immune response, including neutrophils, eosinophils, monocytes, natural killer (NK) cells, macrophages, mast cells, and dendritic cells, as well as adaptive immune response, which includes CD4+ and CD8+ T cells and B cells (78, 79).
Innate immunity is mediated by humoral systems and innate immune cells, such as myeloid cells, innate lymphoid cells, and NK cells. In contrast, adaptive immunity is based on the immunoglobulin family and cells such as T- and B- lymphocytes (80). Following a new pathogen infection, innate immunity is triggered first to eliminate the infection (81). They secrete pro-inflammatory cytokines that inhibit viral replication, recruit other immune cells to the site of infection, and stimulate the adaptive immune response (82). However, the initial clearance of the pathogen can fail due to the high virulence or number of invading pathogens. This is where adaptive immunity is activated to allow specific recognition and elimination of the pathogen, creating the immunological memory to respond more effectively during reinfection (83).
During the SARS-CoV-2 infection, the adaptive immune response can target the four proteins of the virus: envelope, spike glycoprotein, membrane, and nucleocapsid, as well as the addition of 20 non-structural replicase and regulatory proteins that the virus encodes to clear out the infection and develop protection (84). However, there can be a mismatched or delayed response between innate and adaptive immunity following COVID-19 infection (85). As such, an effective antiviral response of the host's innate and adaptive immunity is needed for the production of various pro-inflammatory cytokines and the activation of T cells, including CD4+ and CD8+, to stop viral replication and limit the spread of viruses, inflammation, and clean the infected cells (86). It was revealed that the hyperinflammatory response to SARS-CoV-2 infection was the major cause of the disease's severity and death (87). Compared to mild COVID-19 cases, the critical cases showed enriched chronic hyperinflammatory monocytes and depleted alveolar macrophages, which are known for their antigen-presenting characteristics (88). In mild COVID-19 cases, the adaptive immune response was more potent compared to the critical cases where there was a depletion of T-cells (88).
Individuals with diabetes or obesity were shown to have chronic inflammation and immune dysregulation by adipocytes, which produce an excessive number of cytokines. The increase in the number and hypertrophy of the adipocytes caused heavy stress on the adipose tissue, which in response released cytokines and chemokines such as interleukin-6 (IL-6) and monocyte chemoattractant protein (MCP-1) into the surrounding area. This, in turn, drew immune cells, especially macrophages, into the adipose tissue and subsequently promoted further expression of pro-inflammatory proteins such as IL-6, IL-8, IL-1β, and tumor necrotizing factor α (TNFα) (61, 89). This chronic inflammation and hypercytokinemia state subsequently lead to defective innate immunity (90). Adaptive immunity was also affected by obesity and diabetes, with a decline in naïve CD4+ T cells and imbalances between CD4+ T helper cells toward Th17 and Th22 pro-inflammatory subsets (91, 92).
Individuals with circadian rhythm disruption have glucocorticoid resistance and metabolic diseases such as diabetes and obesity. As the baseline, these individuals with hypercytokinemia/hyperinflammatory state will cause COVID-19 to affect them more severely, leading to rather severe implications as it further increases the hypercytokinemia state, causing more severe implications in cytokine storm (82, 93). Figure 3 illustrates the effects of COVID-19 infection on the immune system in individuals with diabetes mellitus/obesity.
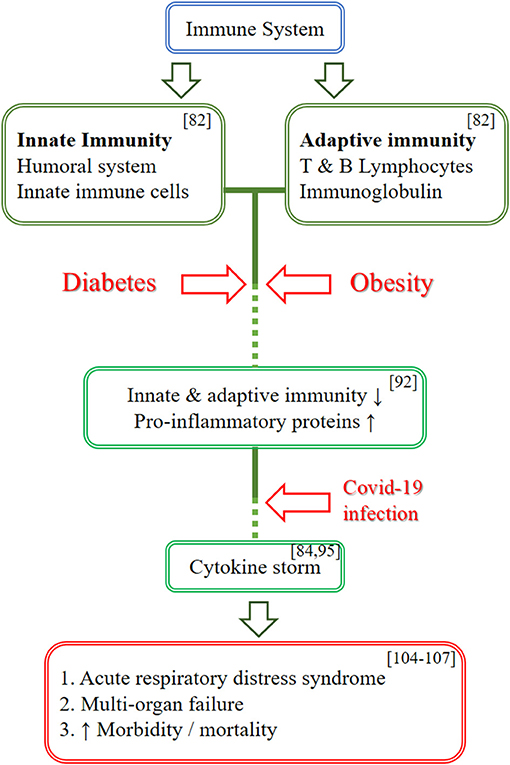
Figure 3. Schematic diagram on the effects of COVID-19 infection on the immune system in individuals with diabetes mellitus/obesity. This figure illustrates the effects of COVID-19 infection on the immune system in individuals with diabetes mellitus/obesity. The immune system is divided into innate and adaptive immunity. Diabetes and obesity caused the innate and adaptive immunity to be less affective and promote hypercytokinemia state as baseline by increasing the pro-inflammatory proteins. A COVID-19 infection further increases the cytokines level and leads to cytokine storm which in turn causing more severe complication such as ARDS and multi-organ failure, thus causing the increase in morbidity and mortality.
Link Between Circadian Rhythm and Severity of COVID-19 and Its Clinical Significance
Interestingly, circadian rhythm plays an essential role in the severity of COVID-19 infection. The defense system in the body follows a rhythmic pattern. Hence, the relationship between circadian timekeeping, host immunity, and host-virus interactions is considered the latest concept (94). The host susceptibility to the microorganism was reported to be dependent on the biological clock in the body (95). Previous reports suggested that the time of the day of infection determines the severity of the progression of influenza, respiratory syncytial, and parainfluenza type 3 viruses (96–98). A detailed, in-depth study showed that the uptake of the SARS-CoV-2 virus in monocytes of the human body varies with the time of the day (95, 99). Following SARS-CoV-2 infection, there is an activation of the inflammatory response involving interleukin signaling and the complement cascade. Both processes are regulated by the circadian rhythm in the body (100).
Individuals with a disturbance in their circadian clock due to old age, working night shifts, or irregular sleeping and eating habits have a compromised immune system. As a result, they could be more susceptible to viral respiratory diseases (101). Future drug targets could also be planned according to the host factor of circadian oscillation.
Conclusion
To summarize, immunity is an essential indicator of COVID-19 infection severity. An effective immunity response in innate and adaptive is important in any individual who are exposed to COVID-19 infection. The severity of infection is greater in individuals whose immunity is compromised. Immunity is affected by factors such as circadian rhythm misalignment and occupational toxicant exposure. As shift workers are more susceptible to circadian rhythm disruption and metabolic diseases, they are more likely to be infected by COVID-19 and develop rather severe complications such as ARDS, multi-organ failure, and death. There is a paucity of studies on the effect of occupational toxicant exposure on the immune system. Still, it is clear that occupational toxicant exposure worsens immunity in the long term. It is also not feasible to eliminate the shift work system in the current society in view of the expanding 24 h industry worldwide. In order to have the best possible work environment, the worker's health must be kept as a top priority in the employer's mind. Shift work is a modifiable risk factor for COVID-19 infection. Future studies must focus on suitable interventions for shift workers to reduce occupational toxicant exposure and circadian disruption. We also recommend future studies that should look into each worker's genetic polymorphisms and eventual epigenetic characteristics.
Author Contributions
NJ and SD: conceptualization of the main idea of the manuscript and in charge of overall direction. SM: writing—original draft preparation and editing. NJ, SD, NASM, NIM, IA, and SA: supervision and provide critical feedback. All authors have read and agreed to the published version of the manuscript.
Funding
This research was funded by Universiti Sains Islam Malaysia grants, grant numbers USIM/FRGS/FPSK/055002/50419 and USIM/MG/RSTECH/FPSK/055012/70619.
Conflict of Interest
The authors declare that the research was conducted in the absence of any commercial or financial relationships that could be construed as a potential conflict of interest.
Publisher's Note
All claims expressed in this article are solely those of the authors and do not necessarily represent those of their affiliated organizations, or those of the publisher, the editors and the reviewers. Any product that may be evaluated in this article, or claim that may be made by its manufacturer, is not guaranteed or endorsed by the publisher.
Acknowledgments
We wish to acknowledge the authors in this review and the collaborating universities.
References
1. Li Q, Guan X, Wu P, Wang X, Zhou L, Tong Y, et al. Early transmission dynamics in Wuhan, China, of novel coronavirus–infected pneumonia. N Engl J Med. (2020) 382:1199–207. doi: 10.1056/NEJMoa2001316
2. Zhu N, Zhang D, Wang W, Li X, Yang B, Song J, et al. A novel coronavirus from patients with pneumonia in China, 2019. N Engl J Med. (2020) 382:727–33. doi: 10.1056/NEJMoa2001017
3. World Health Organization (WHO). Timeline: WHO's COVID-19 Response. (2021). Available online at: https://www.who.int/emergencies/diseases/novel-coronavirus-2019/interactive-timeline# (accessed May 5, 2021).
4. World Health Organization (WHO). COVID-19 Weekly Epidemiological Update. Situation Reports. (2021). Available online at: https://www.who.int/publications/m/item/weekly-epidemiological-update-on-covid-19-−18-may-2021 (accessed May 20, 2021).
5. Kementerian Kesihatan Malaysia (2021). Available online at: http://covid-19.moh.gov.my/terkini/2021/05/situasi-terkini-covid-19-di-malaysia-16052021 (accessed May20, 2021).
6. Amer MS, Issam A, Mohd Hafiz M, Mohammad Subhi J, Mohammed Ali D. Scenario analysis of COVID-19 transmission dynamics in Malaysia with the possibility of reinfection and limited medical resources scenarios. Comput Biol Med. (2021) 133:104372. doi: 10.1016/j.compbiomed.2021.104372
7. Kementerian Kesihatan Malaysia,. Perbandingan Bilangan Kluster dan Jumlah Kes Dalam Kluster bagi tahun 2020 dan 2021 sehingga. (2021). Available online at: http://covid-19.moh.gov.my/semasa-kkm/2021/05/perbandingan-bilangan-kluster-dan-jumlah-kes-dalam-kluster-2020vs2021-21052021 (accessed May 22, 2021).
8. Sean S, John WC. Covid-19: protecting worker health. Ann Work Exposures Health. (2020) 64:461–4. doi: 10.1093/annweh/wxaa033
9. Toly C, Chi-Wei L. Smart and automation technologies for ensuring the long-term operation of a factory amid the COVID-19 pandemic: an evolving fuzzy assessment approach. Int J Adv Manufact Technol. (2020) 111:3545–58. doi: 10.1007/s00170-020-06097-w
10. Asita E. COVID-19 outbreak in Malaysia. Osong Public Health Res Perspect. (2020) 11:93–100. doi: 10.24171/j.phrp.2020.11.3.08
11. Anthony RF, Stanley P. Coronaviruses: an overview of their replication and pathogenesis. Methods Mol Biol. (2015) 1282:1−23. doi: 10.1007/978-1-4939-2438-7_1
12. Yvonne XL, Yan LN, James PT, Ding XL. Human coronaviruses: a review of virus-host interactions. Diseases. (2016) 4:26. doi: 10.3390/diseases4030026
13. Olena K, Olena L, Mykhailo I, Lyudmyla K, Serhiy K, Maryna S. SARS-CoV-2 spike protein fragment 674-685 protects mitochondria from releasing cytochrome c in response to apoptogenic influence. Biochem Biophys Res Commun. (2021) 561:14–8. doi: 10.1016/j.bbrc.2021.05.018
14. McArthur L, Sakthivel D, Ataide R, Chan F, Richards JS, Narh CA. Review of burden, clinical definitions, and management of COVID-19 cases. Am J Med Hyg. (2020) 103:625–38. doi: 10.4269/ajtmh.20-0564
15. World Health Organization. Mode of Transmission of Virus Causing COVID-19: Implications for IPC Precaution Recommendations. World Health Organization (2020). p. 1–3.
16. Sun CJ, Zhai ZQ. Sustainable cities and society, the efficacy of social distance and ventilation effectiveness in preventing COVID-19 transmission. Sustain Cities Soc. (2020) 62:102390. doi: 10.1016/j.scs.2020.102390
17. Bourouiba L. Turbulent gas clouds and respiratory pathogen emissions: potential implications for reducing transmission of COVID-19. J Am Med Assoc. (2020) 323:1837–8. doi: 10.1001/jama.2020.4756
18. Anand U, Cabreros C, Mal J, Ballesteros F, Sillanpaa M, Tripathi V, et al. Novel coronavirus disease 2019 (COVID-19) pandemic: from transmission to control with an interdisciplinary vision. Environ Res. (2021) 197:111126. doi: 10.1016/j.envres.2021.111126
19. Maidstone R, Anderson SG, Ray DW, Rutter MK, Durrington HJ, Blaikley JF. Shift work is associated with positive COVID-19 status in hospitalised patients. Thorax. (2021) 76:601–6. doi: 10.1101/2020.12.04.20244020
20. Fatima Y, Bucks RS, Mamun AA, Skinner I, Rosenzweig I, Leschziner G, et al. Shift work is associated with increased risk of COVID-19: findings from the UK Biobank cohort. J Sleep Res. (2021) 30:e13326. doi: 10.2139/ssrn.3684452
21. Rowlands AV, Gillies C, Chudasama Y, Davies MJ, Islam N, Kloecker DE, et al. Association of working shifts, inside and outside of healthcare, with severe COVID-19: an observational study. BMC Public Health. (2021) 21:773. doi: 10.1186/s12889-021-10839-0
22. Benjamin GM, Ghazal A, Katya L, Lanbo Y, Emily JS, Silvia SC, et al. Predictors of COVID-19 severity: a literature review. Rev Med Virol. (2020) 31:1–10. doi: 10.1002/rmv.2146
23. Imam Z, Odish F, Gill I, O'Connor J, Armstrong J, Vanood A, et al. Older age and comorbidity are independent mortality predictors in a large cohort of 1305 COVID-19 patients in Michigan, United States. J Intern Med. (2020) 288:469–76. doi: 10.1111/joim.13119
24. Huang S, Wang J, Liu F, Liu J, Cao G, Yang C, et al. COVID-19 patients with hypertension have more severe disease: a multicenter retrospective observational study. Hypertens Res. (2020) 43:824–31. doi: 10.1038/s41440-020-0485-2
25. Guo W, Li M, Dong Y, Zhou H, Zhang Z, Tian C, et al. Diabetes is a risk factor for the progression and prognosis of COVID-19. Diabetes Metab Res Rev. (2020) 36:e3319. doi: 10.1002/dmrr.3319
26. Li X, Xu S, Yu M, Wang K, Tao Y, Zhou Y, et al. Risk factors for severity and mortality in adult COVID-19 inpatients in Wuhan. J Allergy Clin Immunol. (2020) 146:110. doi: 10.1016/j.jaci.2020.04.006
27. Choi YJ, Park JY, Lee HS, Suh J, Song JY, Byun MK, et al. Effect of asthma and asthma medications on the prognosis of patients with COVID-19. Eur Respir J. (2021) 57:2002226. doi: 10.1183/13993003.02226-2020
28. Sarah Z, Andrew IM, Garry LRJ, Markus PS, Sally CI, Ruth A, et al. Cardiovascular disease and COVID-19: Australian and New Zealand consensus statement. Med J Aust. (2020) 213:182–7. doi: 10.5694/mja2.50714
29. Brandon MH, Giuseppe L. Chronic kidney disease is associated with severe coronavirus disease 2019 (COVID-19) infection. Int Urol Nephrol. (2020) 52:1193–4. doi: 10.1007/s11255-020-02451-9
30. Pranata R, Soeroto AY, Huang I, Lim MA, Santoso P, Permana H, et al. Effect of chronic obstructive pulmonary disease and smoking on the outcome of COVID-19. Int J Tuberc Lung Dis. (2020) 24:838–43. doi: 10.5588/ijtld.20.0278
31. Gao Y, Chen Y, Liu M, Shi S, Tian J. Impacts of immunosuppression and immunodeficiency on COVID-19: a systematic review and meta-analysis. J Infect. (2020) 81:93–5. doi: 10.1016/j.jinf.2020.05.017
32. David AK, Priya D, Oscar C. Obesity could shift severe COVID-19 disease to younger ages. Lancet. (2020) 395:1544–5. doi: 10.1016/S0140-6736(20)31024-2
33. Uhm JY, Kim HR, Kang GH, Choi YG, Park TH, Kim SY, et al. The association between shift work and chronic kidney disease in manual labor workers using data from the Korea National Health and Nutrition Examination Survey (KNHANES 2011-2014). Ann Occup Environ Med. (2018) 30:69. doi: 10.1186/s40557-018-0279-z
34. Celine V, Hassan SD, Jacqueline ML, Simon GA, Eva SS, Martin KR, et al. Night shift work, genetic risk, and type 2 diabetes in the UK Biobank. Diabetes Care. (2018) 14:762–9. doi: 10.2337/dc17-1933
35. Manohar S, Thongprayoon C, Cheungpasitporn W, Mao MA, Herrmann SM. Associations of rotational shift work and night shift status with hypertension: a systemic review and meta-analysis. J Hypertens. (2017) 35:1929–2937. doi: 10.1097/HJH.0000000000001442
36. Stephen MJ, Kimberly AH, Shobhan G, Hans PAVD. Shift work: disrupted circadian rhythms and sleep – implications for health and well-being. Curr Sleep Med Rep. (2017) 3:104–12. doi: 10.1007/s40675-017-0071-6
37. Robert JM, James T, Celine V, Hassan SD, Frank AJLS, Steven AS, et al. Night shift work is associated with an increased risk of asthma. Thorax. (2021) 76:53–60. doi: 10.1136/thoraxjnl-2020-215218
38. Natasha LS, Shane KM, Jane JP, Peter JM. Endocrine consequences of circadian rhythm disruption in early life. Curr Opin Endocr Metabol Res. (2020) 11:65–71. doi: 10.1016/j.coemr.2020.02.001
39. Jennifer AM, Carla BG, Joseph ST. Central and peripheral circadian clocks in mammal. Annu Rev Neurosci. (2012) 35:445–62. doi: 10.1146/annurev-neuro-060909-153128
40. Paola T, Christoph AT, Eran E, Paolo SC. Circadian coordination of antimicrobial response. Cell Host Microbe. (2017) 22:185–92. doi: 10.1016/j.chom.2017.07.007
41. Jessica AD, Sung YM, Michal T. Circadian disruption changes gut microbiome taxa and functional gene composition. Front Microbiol. (2018) 9:737. doi: 10.3389/fmicb.2018.00737
42. William HW, James CW, Courtney D, Randy JN. Circadian rhythm disruption and mental health. Transl Psychiatry. (2020) 10:28. doi: 10.1038/s41398-020-0694-0
43. Mohd Azmi NAS, Juliana J, Azmani S, Mohd Effendy N, Abu IF, Mohd Fahmi Teng NI, et al. Cortisol on circadian rhythm and its effect on cardiovascular system. Int J Environ Res Public Health. (2021) 18:676. doi: 10.3390/ijerph18020676
44. Jacopo AV, Giovanni L, Andi W, Giusppe B. Biological rhythms, chronodisruption and chrono-enhancement: the role of physical activity as synchroniser in correcting steroids circadian rhythm in metabolicdysfunctions and cancer. J Bio Med Rhythm Res. (2018) 35:1185–97. doi: 10.1080/07420528.2018.1475395
45. Sydney HA, Kali EM, Destynie M, Adam CS. Time is on the immune system's side, yes it is. Yale J Biol Med. (2019) 92:225–31.
46. Sarah SG, Caio TF, Richard MS. Chromo-immunology: progress and challenges in understanding between the circadian and immune system. Immunology. (2015) 146:349–58. doi: 10.1111/imm.12525
47. Yazit NA, Juliana N, Das S, Teng NIMF, Fahmy NM, Azmani S, et al. Association of micro RNA and postoperative cognitive dysfunction: a review. Mini Rev Med Chem. (2020) 20:1781–90. doi: 10.2174/1389557520666200621182717
48. Phillip LL, Joseph ST. Genetics of circadian rhythm in mammalian model organism. Adv Genet. (2011) 74:175–230. doi: 10.1016/B978-0-12-387690-4.00006-4
49. Mohd Azmi NAS, Juliana J, Mohd Fahmi Teng NI, Azmani S, Das S, Effendy N. Consequences of circadian disruption in shift workers on chrononutrition and their psychosocial well-being. Int J Environ Res Public Health. (2020) 17:2043. doi: 10.3390/ijerph17062043
50. Yanhong H, Chongtao X, Meirong H, Wenlong H, Kusheng W. Saliva cortisol, melatonin levels and circadian rhythm alterations in Chinese primary school children with dyslexia. Med Baltimore. (2020) 99:e19098. doi: 10.1097/MD.0000000000019098
51. Jennifer H. Hypothalamic-pituitary-adrenal axis. Encyclopedia Behav Med. (2013) 1017–8. doi: 10.1007/978-1-4419-1005-9_460
52. Oakley RH, Cidlowski JA. The biology of the glucocorticoid receptor: new signaling mechanisms in health and disease. J Allergy Clin Immunol. (2013) 132:1033–44. doi: 10.1016/j.jaci.2013.09.007
53. Calvo JR, Gonzalez-Yanes C, Maldonado MD. The role of melatonin in the cells of the innate immunity: a review. J Pineal Res. (2013) 55:103–20. doi: 10.1111/jpi.12075
54. Fan H, Shijia P, Pengfei X, Tinting X, Jialin W, Yuan G, et al. Melatonin orchestrates lipid homeostasis through the hepatointestinal circadian clock and microbiota during constant light exposure. MDPI Cells. (2020) 9:489. doi: 10.3390/cells9020489
55. Leena T, Timo P, Anu-Katriina P. Systemic review of light exposure impact on human circadian rhythm. J Biol Med Rhythm Res. (2019) 36:151–70. doi: 10.1080/07420528.2018.1527773
56. Nava Z. New perspectives on the role of melatonin in human sleep, circadian rhythms and their regulation. Br J Pharmacol. (2018) 175:3190–9. doi: 10.1111/bph.14116
57. Leger D, Laudon M, Zisapel N. Nocturnal 6-sulfatoxymelatonin excretion in insomnia and its relation to the response to melatonin replacement therapy. Am J Med. (2004) 116:91–5. doi: 10.1016/j.amjmed.2003.07.017
58. Robin O, Bjoern K, James BO, Justin BET, Mario S, Rita RK, et al. The association of morning serum cortisol with glucose metabolism and diabetes: the Jackson Heart Study. Pseudoneuroendocrinology. (2019) 103:25–32. doi: 10.1016/j.psyneuen.2018.12.237
59. Joshua JJ, Sherita HG. Cortisol dysregulation: the bidirectional link between stress, depression, and type 2 diabetes mellitus. Ann N Y Acad Sci. (2017) 1391:20–34. doi: 10.1111/nyas.13217
60. Angeliki K, Ralf J. Melatonin in type 2 diabetes mellitus and obesity. Nat Rev Endocrinol. (2019) 15:105–25. doi: 10.1038/s41574-018-0130-1
61. Nuttapong Y, Pirayat G, Chainarong T, Jiraporn T. Ischemic stroke, obesity, and the anti-inflammatory role of melatonin. Biofactors. (2021) 47:41–58. doi: 10.1002/biof.1690
62. Serin Y, Acar Tek N. Effect of circadian rhythm on metabolic processes and regulation of energy balance. Ann Nutr Metabol. (2019) 74:322–30. doi: 10.1159/000500071
63. Mohamed AE, Ziad MAE, Azza IO. Melatonin is a potential adjuvant to improve clinical outcomes in individuals with obesity and diabetes with coexistence of Covid-19. Eur J Pharmacol. (2020) 882:173329. doi: 10.1016/j.ejphar.2020.173329
64. Michael RI. Sleep and inflammation: partners in sickness and in health. Nat Rev Immunol. (2019) 19:702–15. doi: 10.1038/s41577-019-0190-z
65. Yong G, Chen Y, Xinyue T, Huilian S, Yingjie C, Xiaoxu Y, et al. Shift work and diabetes mellitus: a meta-analysis of observational studies. Occup Environ Med. (2015) 71:72–8. doi: 10.1136/oemed-2014-102150
67. Gerben H, Karin IP, Bette L, Heleen P, Johannes RA, Willem VM. The mediating role of lifestyle in the relationship between shift work, obesity and diabetes. Int Arch Occup Environ Health. (2021) 94:1287–95. doi: 10.1007/s00420-021-01662-6
68. Charlotte CG, Alison MC, Jill D, Siobhan B. The factors influencing the eating behaviour of shiftworkers: what, when, where and why. J-Stage Ind Health. (2019) 57:419–53. doi: 10.2486/indhealth.2018-0147
69. Emily KB, Catherine EH, Chris TH, Tracy AM, Claire P, Maxine PB. Influences on dietary choices during day versus night shift workers: A mixed methods study. MDPI Nutrients. (2017) 9:193. doi: 10.3390/nu9030193
70. Sue R, Dino P, Geze B. Principles of Occupational Health and Hygiene: An Introduction, 3rd Edn. London: Routledge (2020). p. 2–14.
71. Adeniyi NA, Omotosho IO, Onifade AA, Anetor JI. An assessment of the modulatory effect of zinc on humoral immune parameters in welders and cement block moulders in Ogbomosho, Nigeria. J Innov Res Health Sci Biotechnol. (2016) 1:186–94. doi: 10.18644/jiresh-biotech.0000022
72. Liangjiao C, Jia L, Yanli Z, Guilan Z, Yiyuan K, Aijie C, et al. The toxicity of silica nanoparticles to the immune system. Nanomedicine. (2018) 13:1939–62. doi: 10.2217/nnm-2018-0076
73. Jagzape A, Sawane M, Sawane A, Jagzape T. Impact of bioaerosol exposure on respiratory status of vegetable market workers in Nagpur, India. J Datta Meghe Inst Med Sci Univ. (2013) 8:158–63.
74. Emara AM, Alrasheedi KA, Alrashidi SD, Elgharabawy RM. Effect of inhaled anesthetic gases on immune status alterations in health care workers. J Immunotoxicol. (2021) 18:13–22. doi: 10.1080/1547691X.2020.1869872
75. Farahat SA, Mansour N, Sheta M, Ramadan MA. Immune-modulatory effect of ionising radiation on type 1 and type 2 immune responses among workers in cardiac catheterisation units. Br J Med Med Res. (2017) 19:1–10. doi: 10.9734/BJMMR/2017/29663
76. Jia X, Jia Q, Zhang Z, Gao W, Zhang X, Niu Y. Effects of formaldehyde on lymphocyte subsets and cytokines in the peripheral blood of exposed workers. PLoS ONE. (2014) 9:e104069. doi: 10.1371/journal.pone.0104069
77. Donna LF, Mihai GN, Andreas R, Klaus R, Rolf MZ. Immunological memory: lessons from the past and a look to the future. Nat Rev Immunol. (2016) 16:124–8. doi: 10.1038/nri.2016.13
78. Danping Z, Karina R, Eran E. Circadian influences of diet on the microbiome and immunity. Trends Immunol. (2020) 41:512–30. doi: 10.1016/j.it.2020.04.005
79. Sophia H, Stephan H, Christoph S. Molecular interactions between component of the circadian clock and the immune system. J Molecular Biol. (2020) 432:3700–13. doi: 10.1016/j.jmb.2019.12.044
80. Nadia D. The evolution of adaptive immunity. Adv Exp Med Biol. (2012) 738:218–35. doi: 10.1007/978-1-4614-1680-7_13
81. Mihai GN, Jessica Q, Jos WM. Trained immunity: a memory for innate host defense. Cell Host Microbe. (2011) 9:355–61. doi: 10.1016/j.chom.2011.04.006
82. Julia LM, Catherine AB. The innate immune system: fighting on the front lines or fanning the flames of COVID-19? Cell Host Microbe. (2020) 27:863–9. doi: 10.1016/j.chom.2020.05.009
83. Mihai GN, Andreas S, Katarzyna P, Leo ABJ, Joachim LS. Innate and adaptive immune memory: an evolutionary continuum in the host's response to pathogens. Cell Host Microbe. (2019) 25:13–26. doi: 10.1016/j.chom.2018.12.006
84. Diane EG. Are T cells helpful for COVID-19: the relationship between response and risk. J Clin Invest. (2020) 130:6222–4. doi: 10.1172/JCI142081
85. Federica R, Adel AM, Gian MC, Anella S, Francesco C, Raffaele S, et al. Interleukin-17A (IL-17A), a key molecule of innate and adaptive immunity, and its potential involvement in COVID-19 related thrombotic and vascular mechanism. Autoimmun Rev. (2020) 19:102572. doi: 10.1016/j.autrev.2020.102572
86. Abdurrahman T, Ashhan AV, Marco MC. COVID-19, immune system response, hyperinflammation and repurposing antirheumatic drugs. Turk J Med Sci. (2020) 50:620–32. doi: 10.3906/sag-2004-168
87. Diane MDV, Seunghee K, Hsin-Hui H, Noam DB, Sharon N, Bo W, et al. An inflammatory cytokine signature predicts COVID-19 severity and survival. Nat Med. (2020) 26:1636–43. doi: 10.1038/s41591-020-1051-9
88. Els W, Pierre VM, Abhishek DG, Sander J, Yannick VH, Lore V, et al. Discriminating mild from critical COVID-19 by innate and adaptive immune single-cell profiling of bronchoalveolar lavages. Cell Res. (2021) 31:272–90. doi: 10.1038/s41422-020-00455-9
89. Maeve AM, Orla MF, Ruth MO, Aoibheann MM, Heen MR. Mechanism of obesity-induced inflammation and insulin resistance: insights into the emerging role of nutritional strategies. Front Endocrinol. (2013) 4:52. doi: 10.3389/fendo.2013.00052
90. Evangelos JG, Mihai GN, Nikoletta R, Karolina A, Anastasia A, Nikolaos A, et al. Complex immune dysregulation in COVID-19 patients with severe respiratory failure. Cell Host Microbe. (2020) 27:992–1000. doi: 10.1016/j.chom.2020.04.009
91. Lynn MF, Guowu B, David EO, Peter AW, Matthew JD. Obesity and type 2 diabetes mellitus drive immune dysfunction, infection development, and sepsis mortality. J Leukoc Biol. (2018) 104:525–34. doi: 10.1002/JLB.5VMR0118-021RR
92. Tracey M, Shelley EA, Lei S, Edgar E. Role of innate and adaptive immunity in obesity-associated metabolic disease. J Clin Invest. (2017) 127:5–13. doi: 10.1172/JCI88876
93. Fu Y, Cheng Y, Wu Y. Understanding SARS-CoV-2-Mediated Inflammatory Responses: From Mechanisms to Potential Therapeutic Tools. Virol Sin. (2020) 35:266–71. doi: 10.1007/s12250-020-00207-4
94. Mazzoccoli G, Vinciguerra M, Carbone A, Relogio A. The circadian clock, the immune system, and viral infections: the intricate relationship between biological time and host-virus interaction. Pathogens. (2020) 9:83. doi: 10.3390/pathogens9020083
95. Diallo AB, Coiffard B, Leone M, Mezouar S. Mege, JL. For whom the clock ticks: clinical chronobiology for infectious diseases. Front Immunol. (2020) 9:1457. doi: 10.3389/fimmu.2020.01457
96. Majumdar T, Dhar J, Patel S, Kondratov R, Barik S. Circadian transcription factor BMAL1 regulates innate immunity against select RNA viruses. Innate Immun. (2017) 23:147–54. doi: 10.1177/1753425916681075
97. Edgar RS, Stangherlin A, Nagy AD, Nicoll MP, Efstathiou S, O'Neill JS, et al. Cell autonomous regulation of herpes and influenza virus infection by the circadian clock. Proc Natl Acad Sci U S A. (2016) 113:10085–90. doi: 10.1073/pnas.1601895113
98. Segupta S, Tang SY, Devine JC, Anderson ST, Nayak S, Zhang SL, et al. Circadian control of lung inflammation in influenza infection. Nat Commun. (2019) 10:4107. doi: 10.1038/s41467-019-11400-9
99. Diallo AB, Gay L, Coiffard B, Leone M, Mezouar S, Mege JL. Daytime variation in SARS-CoV-2 infection and cytokine production. Microb Pathog. (2021) 158:1–4. doi: 10.1016/j.micpath.2021.105067
100. Bojkova D, Klann K, Koch B, Widera M, Krause D, Ciesek S, et al. Proteomics of SARS-CoV-2-infected host cells reveals therapy targets. Nature. (2020) 583:469–72. doi: 10.1038/s41586-020-2332-7
101. Ray S, Reddy AS. COVID-19 management in light of the circadian clock. Nat Rev Mol Cell Biol. (2020) 21:494–5. doi: 10.1038/s41580-020-0275-3
102. Michelle W, Yong Z. Circadian rhythm and neurodegenerative disorders. Brain Sci Adv. (2020) 6:71–80. doi: 10.26599/BSA.2020.9050006
103. Tanu S. A review of coronavirus disease-2019 (COVID-19). Indian J Pediatr. (2020) 87:281–6. doi: 10.1007/s12098-020-03263-6
104. Zhou F, Yu T, Du R, Fan G, Liu Y, Liu Z, et al. Clinical course and risk factors for mortality of adult inpatients with COVID-19 in Wuhan, China: a retrospective cohort study. Lancet. (2020) 395:1054–62. doi: 10.1016/S0140-6736(20)30566-3
105. Senthil KS, Huanxing S, Yiwen L, Kuanpin S. Circadian rhythm and melatonin in the treatment of depression. Curr Pharm Des. (2018) 24:2549–55. doi: 10.2174/1381612824666180803112304
106. Adriano NK, Ana PT, Giovanni BF, Gaetano MF, Claudio T. Cardiac and arrhythmic complications in patients with COVID-19. J Cardiovasc Electrophysiol. (2020) 31:1003–8. doi: 10.1111/jce.14479
Keywords: circadian rhythm, occupational toxicants, shift workers, COVID-19, immunity
Citation: Mohd Fuad SH, Juliana N, Mohd Azmi NAS, Mohd Fahmi Teng NI, Azmani S, Abu IF and Das S (2022) Circadian Disruption and Occupational Toxicants Exposure Affecting the Immunity of Shift Workers During SARS CoV-2 Pandemic. Front. Public Health 10:829013. doi: 10.3389/fpubh.2022.829013
Received: 07 December 2021; Accepted: 21 February 2022;
Published: 22 March 2022.
Edited by:
Jaya Kumar, National University of Malaysia, MalaysiaReviewed by:
Diallo Aissatou Bailo, IHU Mediterranee Infection, FranceRashidi Mohamed, National University of Malaysia, Malaysia
Rafi Alnjadat, Al-Balqa Applied University, Jordan
Copyright © 2022 Mohd Fuad, Juliana, Mohd Azmi, Mohd Fahmi Teng, Azmani, Abu and Das. This is an open-access article distributed under the terms of the Creative Commons Attribution License (CC BY). The use, distribution or reproduction in other forums is permitted, provided the original author(s) and the copyright owner(s) are credited and that the original publication in this journal is cited, in accordance with accepted academic practice. No use, distribution or reproduction is permitted which does not comply with these terms.
*Correspondence: Norsham Juliana, bmp1bGlhbmEmI3gwMDA0MDt1c2ltLmVkdS5teQ==