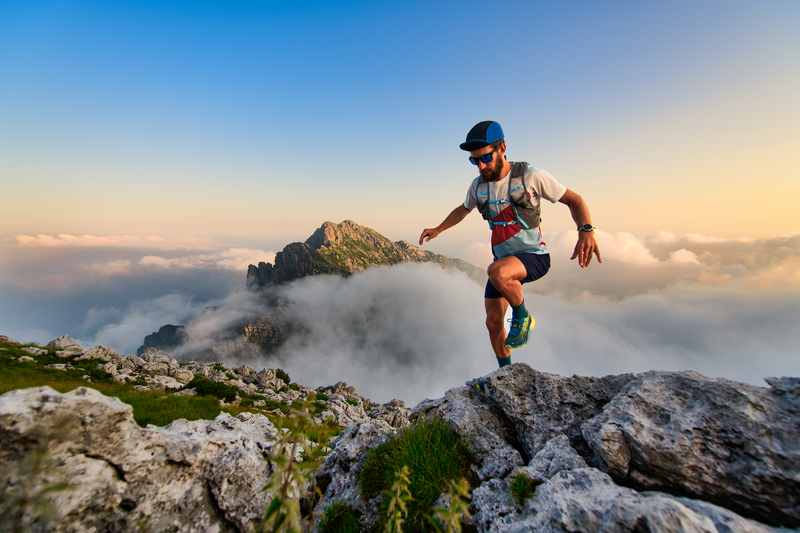
95% of researchers rate our articles as excellent or good
Learn more about the work of our research integrity team to safeguard the quality of each article we publish.
Find out more
REVIEW article
Front. Public Health , 21 December 2022
Sec. Infectious Diseases: Epidemiology and Prevention
Volume 10 - 2022 | https://doi.org/10.3389/fpubh.2022.1025633
This article is part of the Research Topic Antimicrobial Resistance and Antimicrobial Alternatives View all 12 articles
For around three decades, the fluoroquinolone (FQ) antibiotic ciprofloxacin has been used to treat a range of diseases, including chronic otorrhea, endocarditis, lower respiratory tract, gastrointestinal, skin and soft tissue, and urinary tract infections. Ciprofloxacin's main mode of action is to stop DNA replication by blocking the A subunit of DNA gyrase and having an extra impact on the substances in cell walls. Available in intravenous and oral formulations, ciprofloxacin reaches therapeutic concentrations in the majority of tissues and bodily fluids with a low possibility for side effects. Despite the outstanding qualities of this antibiotic, Salmonella typhi, Staphylococcus aureus, Escherichia coli, and Pseudomonas aeruginosa have all shown an increase in ciprofloxacin resistance over time. The rise of infections that are resistant to ciprofloxacin shows that new pharmacological synergisms and derivatives are required. To this end, ciprofloxacin may be more effective against the biofilm community of microorganisms and multi-drug resistant isolates when combined with a variety of antibacterial agents, such as antibiotics from various classes, nanoparticles, natural products, bacteriophages, and photodynamic therapy. This review focuses on the resistance mechanisms of bacteria against ciprofloxacin and new approaches for enhancing its efficacy.
A member of the fluoroquinolone (FQ) family of antibiotics, ciprofloxacin can be used to treat a variety of Gram-positive and Gram-negative bacteria. FQs regulate bacterial DNA supercoiling, a procedure necessary for DNA replication, recombination, and repair, by binding to and inhibiting DNA gyrase enzymes. The United States Food and Drug Administration (FDA) has given the drug approval for the treatment of gastrointestinal and lower respiratory tract infections, anthrax, plague, salmonellosis, skin, bone, and joint infections, prostatitis, typhoid fever, and sexually transmitted infections like gonorrhea and chancroid. It has also been recommended by World Health Organization (WHO) for treating tuberculosis (TB) as the second-line treatment for multidrug-resistant (MDR) TB (1, 2).
Nonetheless, there are increasing reports of ciprofloxacin resistance in Bacillus anthracis, Pseudomonas aeruginosa, Neisseria gonorrhoeae, Enterococci, Escherichia coli, and Klebsiella pneumoniae (3, 4). The resistance could develop by efflux pumps or mutations in DNA gyrase genes (gyrA) (3, 5). Ciprofloxacin can also be used in the treatment of malaria (6). In this regard, the review mainly concentrated on the various properties of ciprofloxacin, its clinical applications for the treatment of different microbial infections, and bacterial resistance mechanisms to this antibiotic, as well as new strategies for enhancing ciprofloxacin efficacy against MDR bacteria.
One-cyclopropyl-6-fluoro-4-oxo-7-(piperazine-1-yl)-1, 4-dihydroquinoline-3-carboxylic acid is the molecular name for the antibiotic (6). Its molecular weight is 331.34 g/mol and its chemical formula is C17H18FN3O3 (7). A quinolone, quinolin-4(1H)-one is the name of the antibiotic, and it has the functional groups cyclopropyl, carboxylic acid, fluoro, and piperazin-1-yl at positions 1, 3, 6, and 7, respectively (Figure 1) (7). The fluorine group at position C-6 and the piperazine group cause the expansion of the antimicrobial spectrum of ciprofloxacin. The piperazine group, also found in cefoperazone and piperacillin, increases ciprofloxacin activity against Pseudomonas. The cyclopropyl group is related to the high antibacterial activity of ciprofloxacin (8).
Figure 1. Chemical structure of ciprofloxacin (9).
The pharmacokinetic profile of ciprofloxacin has been investigated for absorption, distribution, metabolism, and clearance. Studies have been performed by testing healthy and patient volunteers. Ciprofloxacin is absorbed fast and well and penetrates the tissues very well after oral administration. It shows gastrointestinal absorption and bioavailability range between 60 and 85% (8). Time to the maximum concentration of drug in serum (Tmax) was approximately between 40 to 80 min, and the maximum concentration of drug in serum (Cmax) was around 1 mg/L for a dosage of 200 mg. In a comparison between fasting and non-fasting volunteers, it was found that fasting volunteers showed higher Cmax and shorter Tmax than non-fasting volunteers, which means the presence of food interferes with the absorption of ciprofloxacin *.
Ciprofloxacin has low serum binding protein; it shows a mean protein binding of 39% in 0.5, 1, 2, and 5 mg of ciprofloxacin per liter. Ciprofloxacin has great distribution and tissue penetration; accordingly, drug concentration in most tissues and body fluids is higher than in serum (8). Ciprofloxacin can be metabolized in four ways: the primary ways are oxo-ciprofloxacin and sulfo-ciprofloxacin, and two minor ways are ethylene ciprofloxacin and formyl-ciprofloxacin; they are excreted by urine and feces. Unchanged ciprofloxacin was the major molecule appearing in the urine and feces (5).
Ciprofloxacin is a broad-spectrum antibiotic that affects its target by inhibiting the DNA gyrase, which is known as topoisomerase II and topoisomerase IV (10). DNA gyrase contains subunits A and B. Quinolones such as ciprofloxacin are believed to prevent subunit A from resealing the DNA double-strand; therefore, single-stranded DNA may result in exonucleolytic degradation (5). In most studies, the effect of ciprofloxacin on DNA gyrase has been emphasized; however, a previous investigation has suggested that ciprofloxacin could affect Mycobacterium smegmatis cell wall compounds. It has also been demonstrated that ciprofloxacin, in addition to its effect on DNA gyrase, can cause reduction in the amount of DNA, RNA, and protein, as well as phospholipids, galactose, arabinose, glucosamine, and the mycolic acid of the M. smegmatis cell wall. However, these findings should be confirmed in the further studies (Figure 2) (11). Ciprofloxacin affects several Gram-positive bacteria such as Staphylococcus, Streptococcus, Enterococcus, Bacillus spp., and Mycobacterium. Furthermore, ciprofloxacin shows an acceptable in vitro activity against most Gram-negative bacteria strains such as most species of Enterobacteriaceae, N. gonorrhoeae, Neisseria meningitides, Haemophilus influenza, Moraxella catarrhalis, P. aeruginosa, and Legionella species (5, 12). According to a study, the rank order of in vitro activities of seven FQs against 140 clinical Acinetobacter baumannii isolates was in the following order: clinafloxacin > gatifloxacin > levofloxacin > trovafloxacin > gemifloxacin = moxifloxacin > ciprofloxacin *. Noteworthy, the inhibitory effects of ciprofloxacin against different Gram-positive and Gram-negative bacteria and a schematic view of this antibiotic's clinical usage are presented in Tables 1, 2, respectively.
Figure 2. Ciprofloxacin's mechanisms of action (13). Because ciprofloxacin blocks DNA gyrase and topoisomerase IV, DNA replication is slowed and double-stranded DNA breaks are created.
Table 1. The inhibitory effect of ciprofloxacin against various Gram-positive and Gram-negative bacteria.
Biofilm is made up of cell masses that are located in an environment in their extracellular matrix. This matrix contains polysaccharides, proteins, nucleic acids, and lipids. Biofilms are involved relatively in 80% of human infections (64). Biofilm is one of the most essential factors in developing tolerance against antimicrobial agents (65). Ciprofloxacin is an antibiotic agent that has the potential to control biofilm (66). Reffuveille et al. studied the anti-biofilm effect of ciprofloxacin on E. coli and P. aeruginosa. The percentage of biofilms remained in the primary biofilms after growth in a medium containing ciprofloxacin (320 ng/ml or 20 μM) + carboxy-TEMPO (4-carboxy-2, 2, 6, and 6- tetramethylpiperidine 1-oxyl) were 0.7 and 13% in P. aeruginosa PA14 and E. coli O157, respectively. However, using TEMPO without ciprofloxacin revealed that 40% of P. aeruginosa and 29% of E. coli remain. Hence, it can be concluded that the presence of ciprofloxacin is necessary for decreasing biofilm (67).
Verderosa et al. also evaluated the effect of ciprofloxacin-nitroxide and ciprofloxacin-methoxamine hybrids on P. aeruginosa PA14 biofilm at 20- and 40-μM concentrations. When using ciprofloxacin -nitroxide at 20 μM, 80% reduction was observed in the total biofilm; however, half of the biofilm biomass was composed of dead cells, which is suggestive of a 90% reduction in the live cell volume. The use of ciprofloxacin -methoxamine at 40 μg indicated a low reduction in biofilm bio-volume (41%). In addition, 91% of 59% of remaining biomass was composed of dead cells, corresponding to an overall reduction of 95% in live-cell volume, showing a 5% improvement compared to 20 μM. As a result, the higher doses of ciprofloxacin -nitroxide have more potential against P. aeruginosa but less capability in removing biofilm, which may be due to the release of cellular adhesive contents (such as DNA) into the environment. Ciprofloxacin-methoxamine reduce biofilm volume by 30% at 20 μM and by 35% at 40 μM concertation, which proves that it has less effect on biofilm than ciprofloxacin-nitroxide (68).
Therefore, recent studies have reported the antibiofilm effect for ciprofloxacin against Gram-negative bacteria. However, these data are limited, and the exact interaction of this antibiotic with bacterial biofilm is not reported. Therefore, future studies should be evaluated molecular and microscopic interactions of ciprofloxacin with the biofilm community of microorganisms; additionally, the anti-biofilm activity of ciprofloxacin should be assessed against multi-species biofilm.
Antibiotic resistance is one of the most severe public health issues facing the globe today. Antibiotic-resistant organisms can quickly spread, posing a hazard to populations in the form of novel infectious disease strains that are more difficult to cure and treat (69). Treatment failures may occur due to microbial resistance to effective broad-spectrum antibiotics. Treatment failures and difficult-to-treat infections could lead to a high death rate. Drug target mutations (DNA gyrase and DNA topoisomerase IV), mutations that limit drug accumulation, and plasmids that shield cells from ciprofloxacin's deadly effects are the three mechanisms of ciprofloxacin resistance that have been found (70).
Ciprofloxacin resistance in topoisomerase IV or gyrase can result from a single amino acid change. The amino-terminal domains of GyrA (residues 67 to Tyr122 for GyrA, Tyr120 for ParC) or ParC, which are covalently bound to DNA in an enzyme intermediate (106 for E. coli numbering), are where these resistance mutations are most frequently detected (residues 63–102). They are near the tyrosine active site. This domain is referred to as the quinolone resistance determining region (QRDR) of GyrA and ParC (71).
Quinolone resistance has also been linked to changes in specific domains of GyrB and ParE; however, these alterations are far less common in resistant clinical bacterial isolates than mutations in GyrA or ParC. Ciprofloxacin resistance has increased with sequential mutations in both target enzymes. High-level quinolone resistance is frequently associated with mutations in gyrase and topoisomerase IV in several species (72).
In Gram-positive bacteria, active efflux transporters are the main mechanism for reducing cytoplasmic drug concentrations. It has not been demonstrated that decreased diffusion through the cytoplasmic membrane is a form of resistance. Reduced outer membrane porin diffusion channels, which are necessary for ciprofloxacin to enter the periplasm, may be a factor in the development of resistance in Gram-negative bacteria and cooperate with basal or elevated expression of efflux transporters (72).
Porins are the main route for hydrophilic antibiotics like FQs to enter the bacterial outer membrane. Coexisting resistance mechanisms such as efflux pumps or antibiotic degrading enzymes are amplified by lower antibiotic uptake due to alterations in porin expression, resulting in high-level resistance (73).
Horizontal transference has been identified as the principal method for spreading quinolone resistance globally since 1998, when the primary plasmid-mediated quinolone resistance gene (PMQR) was first identified in a K. pneumoniae strain in the USA (74). The lowest inhibitory concentrations (MIC) of FQs, which typically prevent their in vitro detection, impart a modest growth in the presence of these resistance determinants. Furthermore, taking into account high-degree resistance to widen, PMQR might contribute to an increase in the occurrence of spontaneous mutations in QRDRs (72, 74, 75).
Inducing low susceptibility to these drugs by protecting the binding site in DNA-gyrase (qnr gene), modifying the drug enzymatically (aac(6')-Ib-cr gene), and expelling the agent from its site of action by coding for efflux pumps (oqxAB and qepA genes) are currently the three main mechanisms of resistance to quinolones related to PMQR that are recognized (74, 76).
PMQR genes consist of six qnr genes (qnrA, qnrB, qnrC, qnrD, qnrS, and qnrVC) encoding gyrase-protection repetitive peptides oqxAB, qepA, and qaqBIII encoding efflux pumps (77, 78); and aac(6′)-Ib-cr encoding an aminoglycoside and quinolone inactivating acetyl-transferase (79). These genes can synergize with chromosomal gyrA and parA mutations, increase the mutant prevention concentration of quinolones, interfere with quinolone action in apparently susceptible bacteria harboring them (80), and confer evolutionary fitness unrelated to quinolone resistance (Figure 3) (81).
Figure 3. Mechanisms of ciprofloxacin resistance carried by plasmids (82). Genes encoding the ciprofloxacin efflux pumps can be found in plasmids. Aac(6')-Ib-cr, an aminoglycoside-modifying acetyltransferase that acetylates and inactivates ciprofloxacin, or QepA or OqxAB, the Qnr protein, which binds gyrase.
In some Enterobacteriaceae species, the co-existence of mutations in the QRDR and PMQR genes can may occur. Additionally, QRDR mutations that increase FQs resistance can be encouraged by the presence of PMQR determinants (83). According to the findings of the Egyptian study, a high level of resistance to FQs is conferred by the accumulation of PMQR genes and QRDR mutations (84).
Single nucleotide polymorphisms (SNPs) in gyrA alone confer low- to intermediate-level resistance in N. gonorrhea, whereas high-level resistance necessitates one or more specific concurrent mutations in parC. These changes can be easily selected and transferred to other gonococci by exposing them to sub-inhibitory ciprofloxacin doses (85).
A missense mutation in gyrA (S91F) within the QRDR has been demonstrated to cause a 100-fold increase in ciprofloxacin resistance. A subsequent mutation at codon 95 (D95N) resulted in a two-fold increase in ciprofloxacin resistance. Higher levels of quinolone resistance required mutations in parC in addition to those in gyrA. These parC mutations were found in codons 88 (S88P) and 91 (E91K) of the parC gene (85). Additional GyrA/ParC amino acid change patterns were later discovered in ciprofloxacin-resistant bacteria worldwide (86). Ciprofloxacin resistance in Gonorrhea appears unaffected by mutations in the gyrB and parE genes (86).
As mentioned, the most common combinations of amino acid substitutions in the GyrA and ParC proteins conditioning resistance to FQs are S91F + D95G/A in GyrA and S87R in ParC (87). This combination was found in more than 40% of N. gonorrhoeae strains resistant to FQs and conditioned the MIC of ciprofloxacin from 4 to 32 mg/L (87). The frequency of individual mutations in the gyrA and parC genes varies (88). A mechanism increasing FQ MIC values, based on the overproduction of NorM membrane pump proteins, was also described in single N. gonorrhoeae strains (86–89).
In gonococci, four efflux pump systems (MtrCDE, MacAB, NorM, and FarAB) have been discovered in most strains. The MtrCDE, MacAB, NorM, and FarAB systems belong to the RND, ABC, MATE, and MF families, respectively, which have been proven to identify antimicrobials previously or currently approved for gonorrhea treatment (89, 90).
The MICs for ciprofloxacin-resistant N. meningitides isolates have been reported to range between 0.06 and 0.25 g/ml, with mutations in the QRDR of the gyrase-encoding gene gyrA being responsible for the majority of this resistance (91–93). According to a Chinese study, mutations in the QRDR of gyrA related with quinolone resistance substitutions were observed in all of the 51 ciprofloxacin-non susceptible N. meningitides strains, of which 49 strains harbored the typical substitution of threonine to isoleucine at amino acid position 91 (T91I). The other two ciprofloxacin-intermediate strains, harbored aspartate to asparagine substitutions at amino acid position 95 (D95N). No additional mutations were observed in the QRDRs of gyrB, parC, or parE. Furthermore, sixteen gyrA alleles (R1–R16), were defined in 51 ciprofloxacin-non susceptible isolates. Most of ciprofloxacin resistance-conferring alleles were transmitted through horizontal gene transfer (93).
The gyrA gene of N. meningitidis is 95% identical to the gyrA gene of N. gonorrhoeae. Mutations in the gyrA gene have been associated with ciprofloxacin resistance in N. meningitidis. The QRDR from the resistant N. meningitidis contained a mutation that resulted in an Asp95-to-Asn change. This known change in the N. gonorrhoeae gyrA gene's QRDR raises ciprofloxacin MICs to levels similar to those seen in this strain (94).
In the QRDR of gyrA, nearly all previously identified ciprofloxacin-resistant N. meningitidis isolates had Ile (I) or Phe (F) mutations at position 91 (92, 95–97). In N. meningitidis, further mutations in gyrA (D95N and T193A), as well as parC (D86N, S87R, and E91G), have been linked to increased ciprofloxacin MICs (92, 93, 97, 98). Chen et al. found that all quinolone-resistant N. meningitidis isolates contained mutations in T91 and/or D95 of GyrA, with seven isolates also possessing ParC mutations and displaying higher MICs. The specific Neisseria lactamica donors of seven mutation-carrying gyrA alleles (gyrA92, gyrA97, gyrA98, gyrA114, gyrA116, gyrA151, and gyrA230) and the Neisseria subflava donor isolate of gyrA171 were discovered by genomic analysis. Transformation of gyrA fragments from these donor strains into a meningococcal isolate raised its ciprofloxacin MIC from 0.004 g/ml to 0.125 or 0.19 g/ml and to 0.5 g/ml with the further transformation of an additional ParC mutation, according to their findings. Over fifty percent of quinolone-resistant N. meningitides strains acquired resistance through horizontal gene transfer from three commensal Neisseria species (97).
According to a study conducted in Brazil, all of the ciprofloxacin -resistant N. meningitides isolates possessed a Thr to Ile mutation at the QRDR of the gyrA gene's amino acid 91. No further mutations were identified in the gyrA or parC QRDRs (91). According to a study in Spain, single mutations in the gyrA (with Thr-91 to Ile being the most common substitution found) of ciprofloxacin-resistant N. meningitidis were the primary mechanism implicated. Four distinct gyrA substitutions were found in two meningococci. There were no changes in the parC and gyrB genes' QRDRs. However, three strains had a His-495 to Asn substitution in the parE gene. In addition, two distinct mutations in the mtrR gene that impact the expression of the MtrCDE efflux mechanism were discovered (99).
Two basic pathways of ciprofloxacin resistance in P. aeruginosa have been thoroughly explored. Major contributors to ciprofloxacin resistance in P. aeruginosa are mutations in the ciprofloxacin target-encoding genes gyrAB and parCE that decrease the affinity of DNA gyrase or topoisomerase for ciprofloxacin (13). Furthermore, overexpression of efflux pumps to lower antibiotic intracellular concentrations promotes ciprofloxacin expulsion from P. aeruginosa cells due to mutations in efflux pump regulatory genes. It has become apparent that a wide number of additional genes can play a role in ciprofloxacin resistance and that resistance evolves through a mix of alleles, underscoring the multifactorial character of the ciprofloxacin resistance development process (13). Bacteria with both target-site mutations and efflux overexpression were more resistant to ciprofloxacin than bacteria with only individual mutations (100).
Sequence variants in which the Thr at position 83 in GyrA is replaced by Ile and the Ser at position 87 in ParC is replaced by a Leu are the most commonly occurring alterations associated with ciprofloxacin resistance in P. aeruginosa isolates from patients and in vitro evolved isolates (100).
The second most common GyrA variation occurs at position 87, where Asn, Tyr, or Gly residues replace aspartate (13). The presence of alternative amino acid residues at these positions decreases gyrase's affinity for ciprofloxacin, providing a molecular explanation for the GyrA variations' increased ciprofloxacin resistance (101). GyrA and ParC variants are more common than GyrB and ParE variants, possibly because alterations in GyrB, and ParE sequences give lower-level ciprofloxacin resistance. In clinical isolates of P. aeruginosa, resistance alleles in both gyrA and parC give stronger ciprofloxacin resistance than resistance alleles in only gyrA (102–104).
Four efflux pumps in P. aeruginosa are known to efflux FQs: MexCD-OprJ, MexEF-OprN, MexAB-OprM, and MexXY-OprM (105–107). System-specific regulatory proteins regulate efflux pump gene expression, and mutations in these regulators cause efflux pump overexpression (108, 109). Two efflux pumps are overexpressed. Most typically, MexCD-OprJ and MexEF-oprN have been implicated with ciprofloxacin resistance. Overexpression of MexCD-OprJ occurs in P. aeruginosa isolates from Cystic Fibrosis (CF) and non-CF patients and is caused by mutations in the nfxB gene (110, 111).
Overexpression of MexEF-OprN occurs in isolates of P. aeruginosa from CF and non-CF patients due to mutations in the mexS gene, which result in overexpression of MexT. The MexEFoprN genes are regulated by the transcription factor MexT (106).
Furthermore, overexpression of MexXY-OprM in clinical isolates of P. aeruginosa has been demonstrated to confer ciprofloxacin resistance at lower levels. Mutations in the regulator gene mexZ have been blamed for most MexXY-OprM overexpression (100). Experiments demonstrate that a gyrA-resistant allele mutation is required for ciprofloxacin resistance, with other mutations enhancing resistance further (112). GyrA's great affinity for ciprofloxacin makes it possible for bacteria to harbor mutations in the regulatory genes of efflux pumps, yet a wild-type gyrA allele may still be vulnerable to the drug (100).
The most prevalent mechanism of ciprofloxacin resistance in Campylobacter is a single point mutation C257T in the gyrA gene, located within the QRDR resistance (113). This causes a Thr to Ile amino acid change in the Gyrase A subunit at position 86 (114). Other mutations in the gryA gene have been linked to increased ciprofloxacin resistance but at lower doses and frequencies (115–117). In Campylobacter spp., polymorphisms in the gyrB gene have been ruled out as a cause of quinolone resistance. The gyrA mutation interacts with the most frequent Campylobacter drug efflux pump, CmeABC, to promote the development of ciprofloxacin-resistant bacteria when its expression is raised (116, 117). Overexpression of the CmeABC efflux pump does not result in ciprofloxacin resistance without the gyrA gene mutation (114, 117, 118).
The 16-bp inverted repeat (IR) in the cmeR-cmeABC intergenic region is one more element that heightens resistance. The percentage of resistant isolates increases and the average ciprofloxacin MIC increases when this mutation coexists with the C257T-gyrA mutation. Recently found and spreading, RE-cmeABC is a variant of the cmeABC gene that increases ciprofloxacin resistance (118). Ciprofloxacin resistance may be indirectly impacted by changes in other genes. Variations in the mutant frequency decline gene (mfd), for instance, may be involved because silencing of this gene has been shown to 100-fold reduce mutation rates (117).
Ciprofloxacin resistance in H. influenzae is associated to chromosome-mediated mutations in the QRDRs of the genes producing DNA gyrase and topoisomerase IV, including gyrA, gyrB, parC, and parE. GyrA (at Ser84 and Asp88) and parC (at Gly82, Ser84, and Glu88) had more amino acid changes than gyrB and parE (119). Puig et al. found that strains with a single alteration in GyrA or one change in GyrA plus one in ParC had ciprofloxacin MICs of 0.12 to 2 g/ml.
In contrast, those with three or four changes (in GyrA, ParC, and ParE) had higher MICs (8–16 g/ml) (120). Ser84 to Leu or Tyr and Asp88 to Tyr, Asn, or Gly were the most common alterations in GyrA, which have been linked to resistance in H. influenza (119). In ParC, the most common changes were Ser84Ile and Glu88Lys (119) and Ser84Arg (119).
Ciprofloxacin resistance in Enterobacteriaceae has been extensively researched (121, 122). The accumulation of mutations in the genes encoding the two quinolone targets: DNA gyrase and topoisomerase IV in E. coli is a major contributor to resistance and decreased sensitivity to quinolones (123).
It may just take one change to the E. coli gene gyrA to result in large levels of nalidixic acid resistance. However, additional, progressive mutations in the topoisomerase IV or gyrA genes are necessary for high-level FQs resistance, including ciprofloxacin. Escherichia coli was the only species in a study of eight Enterobacteriaceae species where multiple mutations in gyrA were necessary for high-level FQ resistance. The most frequent gyrA mutations identified in clinical, veterinary, and laboratory strains of E. coli occur at codon 83. This Ser residue is most frequently changed to Leu in E. coli isolates with high levels of nalidixic acid resistance and lower susceptibility to FQs*.
Strains with a somewhat higher resistance to FQs had an extra mutation, most frequently at codon Asp87. The high occurrence of mutations at Ser83, however, has a plausible explanation because strains with a single mutation at Ser83 were considerably more resistant to FQs than those with a single mutation at Asp87*.
Increased drug extrusion caused by overexpression of AcrAB-TolC, the principal efflux pump reported in Enterobacteriaceae, on the other hand, is a major source of worry because it confers cross-resistance to a variety of unrelated chemicals, including antimicrobials. Other efflux systems, such as AcrEF and EmrAB, have been reported to engage in the extrusion of antimicrobial compounds to a lesser amount (124).
Increased efflux has been identified as the main mechanism for the development of quinolone resistance in Salmonella. On the other hand, in these bacteria, decreased OmpF porin synthesis has occasionally been linked to the MDR phenotype. Furthermore, according to a study, the ParC T57S substitution was common in strains exhibiting the lowest MICs of ciprofloxacin, while increased MICs depended on the type of GyrA mutation. PMQR genes represented a route for resistance development without target-site mutations (125).
According to Azargun et al., high-level ciprofloxacin resistance in Enterobacteriaceae is linked to DNA gyrase and topoisomerase IV mutations as a primary mechanism and PMQR genes acrB efflux pump gene expression, and outer membrane ompF gene expression. Ciprofloxacin resistance is increased due to twin mutations in gyrA and parC (124). PMQR genes are not the critical mechanism of ciprofloxacin resistance in uropathogenic E.coli in South Iran, according to Malekzadegan et al. (126).
Legionella pneumophila resistance to ciprofloxacin is most typically linked to changes in gyrA, gyrB, parC, and parE genes. Mutations affecting codons 83 and 87 of the gyrA QRDR have been linked to the in vitro selection of L. pneumophila strains with high-level ciprofloxacin resistance (127). However, mutations in gyrB and parC have also been identified (127). In vivo, only mutations at codon 83 of the gyrA gene have been described (128, 129).
Using next-generation DNA sequencing (NGS), Shadoud et al. demonstrated that the 248CT (T83I) mutation-carrying L. pneumophila mutant population was rapidly selected in vivo in two legionellosis patients treated with ciprofloxacin, increasing from 1.05% of the total L. pneumophila lung population at the time of diagnosis to 94% after a few days of FQ treatment (129).
According to a study, an amino acid substitution of Thr80 to Ile in GyrA causes M. catarrhalis to have low-level resistance to FQs (130). FQ targets gyr and par were also sequenced in another work on isolates with decreased FQ resistance that were produced by stepwise selection in levofloxacin. GyrA (D84Y, T594dup, and A722dup), GyrB (E479K and D439N), and ParE (Q395R) were shown to have six new mutations that contribute to M. catarrhalis resistance to FQs (131). According to a Polish study, M. catarrhalis FQ resistance is linked to amino acid changes in the gyrA and gyrB genes. G412C and four silent transition mutations were found in the gyrA gene. Two identical silent mutations and the substitution A1481G occurred in the gyrB gene (132).
Resistance to ciprofloxacin in Acinetobacter baumannii is advanced via unique techniques, one of which is the modifications that took place within the expression of the efflux pumps. The efflux pump in A. baumannii is the AdeABC pump and is of great significance in phrases of resistance advent (133).
This efflux pump has a three-part structure and is a member of the resistance-nodulation-cell department (RND) family: AdeB is a multidrug transporter, AdeC is an outer membrane protein, and AdeA is a membrane fusion protein. AdeS is a sensor kinase, while AdeR is a response regulator. Together, they form the -component system (AdeR-AdeS) that tightly controls the adeABC operon. Both point mutations in AdeRS and the insertion sequence (IS) Aba-1 insertion upstream of the adeABC operon have been implicated in the overexpression of the AdeABC efflux pump*.
The presence of quinolone resistance (qnr) genes on the plasmid, which results in a low-level resistance to quinolones, is another mechanism that results in resistance to ciprofloxacin (134). A mutation in the quinolone resistance-determining regions (QRDR), which affects the target enzymes of DNA gyrase (gyrA) and topoisomerase IV (parC), is another important mechanism. The main effects of quinolones are on target enzymes like DNA gyrase, which block the transcription process by attaching to and mutating this enzyme's gene (135).
Sequencing results in several studies revealed a serine to leucine mutation at position 83 of the gyrA subunit, indicating that Ser83Leu substitution is the primary mutation in A. baumannii for FQ resistance (136). In ciprofloxacin-resistant isolates from a different investigation, Ala84Pro or Gly81Val mutations in the gyrA gene were found. Quinolones' target in A. baumannii is topoisomerase IV, and mutations at parC residues Ser80 and Glu84 contribute to decreased fluoroquinolone sensitivity*.
Two clinical isolates from another study had mutations in parC without gyrA, suggesting that parC might not only be a secondary goal for quinolones but is as critical as gyrA to purpose a decreased susceptibility to FQs in A. baumannii (136). ParC mutations are typically in conjunction with mutations in gyrA and are needed to gather a high degree resistance to quinolones. Acinetobacter baumannii can resist FQs with just a single point mutation in DNA gyrase, but concurrent mutations in the QRDR regions of the gyrA and parC genes are projected to significantly contribute to high-degree FQs resistance (136).
A study found that the Serine 83 to Leucine mutation was present in the DNA gyrase subunit A's QRDR in isolates that were resistant to ciprofloxacin (GyrA). Furthermore, among isolates that were resistant to ciprofloxacin, researchers were unable to detect ParC mutations or plasmid-mediated quinolone resistance (qnrA). They came to the conclusion that a mutation in GyrA, with the presence of efflux pumps serving as a secondary motive, is the primary source of ciprofloxacin resistance in A. baumannii isolates from burn infections (137).
According other study in Iran, the prevalence rates of qnrA, qnrB, qnrS, AdeA, AdeB, and AdeC genes among A. baumannii isolates have been 0, 0, 3.9, 100, 100, and 100%, respectively. In all of the resistant isolates, mutation within the gyrA gene became discovered, however, no mutation became visible within the parC gene (138).
In Enterococci, ciprofloxacin resistance is mostly caused by chromosomal mutations in the genes encoding quinolone targets, DNA gyrase, and topoisomerase IV, which are mostly found in the QRDR (139). Resistance-associated mutations have been discovered in the gyrA gene (Ser83Arg, Ile, or Asn; Glu87Lys, Gly) and the parC gene in E. faecalis (Ser80Arg, or Ile; Glu84Ala). In Enterococcus faecium, mutations in the gyrA gene (Ser83Ala, Leu, Ile, Tyr, or Arg; Glu87Leu, Gly, or Lys) and the parC gene (Ser83Ala, Leu, Ile, Tyr, or Arg) have been identified (140).
Another well-known mechanism of quinolone resistance is antibiotic externalization via efflux pumps. NorA is described in E. faecium and EmeA in E. faecalis (141). A third resistance mechanism reported in E. faecalis is qnr, a protein with a series of pentapeptide repeats identical to the plasmid-borne quinolone resistance genes identified in Enterobacteriaceae. This protein protects DNA gyrase by preventing ciprofloxacin from binding to DNA and forming an antibiotic–gyrase complex (142).
It has been well established over the past few decades that the pathogen's capacity for resistance to antimicrobial drugs, particularly methicillin, may contribute to its persistence in the hospital and community (143). Methicillin-resistant S. aureus (MRSA) are a global health concern due to their growing resistance to macrolide, lincosamide, and streptogramin B treatments (144). In clinical isolates of S. aureus, resistance to ciprofloxacin is caused by both mutations in topoisomerases that impair drug binding effectiveness and increased production of endogenous efflux pumps (72). Amino acid substitutions in residues that make up the drug-binding site, also known as the quinolone resistance-determining area, are the most common types of mutations (72). ParC is the topoisomerase with the highest sensitivity in Staphylococci and is thus the major target. The secondary target is DNA gyrase, which is less sensitive. FQs are highly effective for Staphylococci; thus, alterations in both enzymes are required to build a resistance that exceeds the MIC breakpoint. A single amino acid substitution will often increase the MIC by 8–16 times (145, 146).
Clinical isolates with strong ciprofloxacin resistance frequently overexpress chromosomally encoded efflux pumps. NorA is responsible for the ciprofloxacin and norfloxacin resistant, while NorB and NorC are responsible for the sparfloxacin and moxifloxacin resistant. Therefore, the overexpression of an efflux pump (NorA) leads to the ciprofloxacin resistance in S. aureus (72).
After challenging 222 isolates of S. aureus with the antibiotic ciprofloxacin, Papkou et al. (147) discovered that a single efflux pump, norA, causes widespread variation in evaluability across isolates, and that chemical inhibition of NorA effectively prevents resistance evolution in all isolates. The frequency of efflux pump genes driving ciprofloxacin and antiseptic resistance in MRSA isolates was studied in a study conducted in Iran. According to their findings, the mdeA and qacA/B genes were detected with the highest (61.7%) and lowest (3.3%) frequency, respectively, among ciprofloxacin-resistant isolates (148).
The second-leading cause of death worldwide among infectious diseases is TB, an old infectious disease caused by M. tuberculosis and other species that are closely related to it. Each year, an estimated two–three million people die from TB and its associated complications worldwide (149). DNA gyrase mutations, drug efflux pumps, bacterial cell wall thickness, and pentapeptide proteins (MfpA)-mediated gyrase regulation in M. tuberculosis are the ciprofloxacin -resistant mechanism in Mtb (150). Because mycobacteria lack topoisomerase IV, ciprofloxacin resistance mutations are found in the genes encoding gyrase, most commonly in the QRDR of gyrA, but sometimes in the QRDR of gyrB. Mutations mainly cause FQ resistance in tuberculosis in the gyrA gene, the most prevalent at locations 90, 91, and 94, which are associated with high-level resistance (151).
The most prevalent mutations in gyrA are found in the QRDR codons 88–94, particularly codons 88, 90, 91, and 94. FQ resistance in gyrB is often linked to mutations in codons 500 and 538 (152).
The incidence of gyrA mutations does, however, vary geographically. A study's mutational examination of samples from pulmonary TB patients revealed that the majority of the mutations change codons 94 (changing Asp with Gly, D94G), and 90 (replacing Ala with Val A90V). In MDR and treatment failure instances, the D94G mutation was most frequently linked to resistance to FQs. However, many A90V mutations were discovered in recently diagnosed patients (153).
The Mmr efflux transporter is the only efflux pump from the small multidrug resistance (SMR) family in the Mtb genome. It has been linked to M. tuberculosis resistance to dyes and antibiotics such as FQs (154). In a systematic assessment of gyr mutations, 64% of FQ-resistant M. tuberculosis isolates contained mutations in the QRDR of gyrA. In 534 resistant isolates, the QRDR of gyrB was sequenced, but only 3% exhibited mutations. Eighty-one percent of the gyrA mutations were found inside the QRDR, whereas 19% were found outside. In 54% of FQ resistant isolates, mutations in gyrA codons 90, 91, and 94 were found (substitutions at amino acid 94 accounted for 37%). Only 44% of the gyrB mutations were found inside the QRDR (155). Two amino acid positions, 74 and 88, are related to less prevalent genetic variants in gyrA (156).
Multiple mutations and codons 94, 90, and 88 of gyrA provided high-level FQ resistance (157). The considerably less common gyrB mutations (up to 10%−15%) were generally, but not consistently, associated with lower levels of FQ resistance (155). Nevertheless, combined gyrA and gyrB mutations could result in a substantially higher resistance level (155, 158). Other efflux pumps that may be involved in FQ resistance include antiporters LfrA and Tap, in addition to the mycobacterial pentapeptide MfpA and the ATPase complex Rv2686c-Rv2687c-Rv2688c operon (159). According to a study, ciprofloxacin-resistant clinical isolates of M. tuberculosis had significant efflux pump pstB transcripts in a few isolates, implying that the pump plays a role in resistance (160).
It should be noted that all of the mentioned resistance mechanisms are summarized in Table 3.
Synergism of ciprofloxacin and amikacin for P. aeruginosa is as follows: total synergism–ciprofloxacin ¼ MIC + amikacin ¼ MIC, partite synergism–ciprofloxacin ½ MIC + amikacin 1/16 MIC or ciprofloxacin 1/16 MIC + amikacin ½ MIC. Time-kill assay affirmed the synergistic activity of ciprofloxacin and amikacin, apparent at as early as 4 h and kept up after that*. Combining gentamicin with ciprofloxacin against E. coli and P. aeruginosa displayed the ideal treatment alternative. However, more in vivo and clinical trials are needed to determine the potential treatment regimen based on the combination of these two antibiotics (161).
Additionally, unlike when each antibiotic was used alone, combining tobramycin with either azithromycin or ciprofloxacin enhanced the killing of planktonic K. pneumoniae cells and accelerated bacterial clearance in a mouse model of cutaneous abscess infection. Additionally, combining ciprofloxacin and tobramycin increased the bactericidal activity against cells linked to biofilms. In this regard, the antibiotic combinations reduced the number of bacteria from 108 to fewer than 10 colony forming units (CFU) ml−1; however, when each antibiotic was used alone, only 500 CFU ml−1 of bacteria were recovered (162).
In view of these findings, ciprofloxacin and tobramycin may be used in combination to treat both acute and persistent K. pneumoniae infections. The use of the aforementioned combination therapy can also lessen the emergence of resistance to individual or groups of antibiotics.
Finally, the results of another study indicated that the outcomes of ciprofloxacin -streptomycin combination with cefotaxime represented a synergic impact on MDR P. aeruginosa and a noteworthy lessening in the MIC value at a ratio of 1:3 for 20 strains with the percentage of 95.23% (163). Thus, synergistic results of cefotaxime or streptomycin– ciprofloxacin make this combination beneficial. However, more studies of P. aeruginosa in a clinical setting are required to assess this combination's interactions.
As mentioned, the efflux pump is one of the main resistance mechanisms of bacteria against CIP. In this concept, Pankey et al. proposed that gatifloxacin, an 8-methoxyfluoroquinolone, could boost CIP's efficacy by inhibiting the efflux pump. Synergy testing was performed by E-test and time-kill assay for 31 clinically one kind, plasmid DNA distinct, P. aeruginosa segregates. Based on the E-test method, ciprofloxacin and gatifloxacin combination demonstrated synergy in six (19%) out of 31 P. aeruginosa isolates utilizing a summation fractional inhibitory concentration (FIC) of ≤0.5 for synergy. Also, the time-kill assay illustrated synergy for 13 (42%)/31 isolates*. Hence, it seems gatifloxacin inhibits the efflux pump and increases the efficacy of ciprofloxacin against P. aeruginosa; however, in vitro synergy by ciprofloxacin plus gatifloxacin against MDR P. aeruginosa should be evaluated in clinical setting.
The combination use of cephalosporins with different FQ such as ciprofloxacin was considered by researchers. Mayer et al., reported that the combination of ciprofloxacin, ofloxacin, and pefloxacin with ceftazidime, examined by disc diffusion method, demonstrated synergy for only 3–5 isolates*. The three ciprofloxacin-β-lactam combinations, including ciprofloxacin + ceftazidime, ciprofloxacin + aztreonam, and ciprofloxacin + azlocillin, were evaluated against MDR isolates of P. aeruginosa. The frequency of synergy was subordinate to antibiotic susceptibilities. Based on the evidence, in case the organism was resistant to ciprofloxacin, synergy was found in more than 50% of the isolates, but if the organism was resistant to the β-lactam (excluding ceftazidime), synergy was commonly observed in <10% of the isolates*.
The combination of meropenem and ciprofloxacin seems more effective than either antibiotic alone in ICU infections due to P. aeruginosa strains. An earlier study examined 32 nosocomial-acquired P. aeruginosa strains between April 2001 and November 2001. Following the combination of ciprofloxacin with meropenem, an FIC index proposed synergy in two (6.2%) strains. The first strain was susceptible to ciprofloxacin but resistant to meropenem and imipenem; however, the second strain was both ciprofloxacin and carbapenems susceptible. Synergistic activity utilizing ciprofloxacin and imipenem happened in only one (3.1%) strain, which was susceptible to ciprofloxacin and imipenem*.
Time-kill synergy trials suggested that at 24 h, the sub-inhibitory meropenem and ciprofloxacin concentrations of 0.06–128 and 0.03–32 mg/L, respectively, indicated synergy against 34/51 P. aeruginosa strains, but that of 0.25–2 and 0.12–16 mg/L, respectively showed synergy against 18/52 Acinetobacter baumannii strains at the same period (164).
Rees et al. assessed bacterial killing and resistance suppression by combining meropenem with ciprofloxacin against P. aeruginosa in isolates collected from CF patients. Monotherapy with either meropenem or ciprofloxacin had a failure to suppress bacterial regrowth and the resistance of a hyper mutable clinical CF isolate at a high inoculum. However, the combination of 6 g of meropenem with 1.2 g of ciprofloxacin daily, both given periodically, achieved synergistic killing and resistance suppression over 8 days (165).
In another investigation, the authors separated two strongest extensive drug-resistant strains of P. aeruginosa, VIT PC 7 and VIT PC 9, from diabetic foot ulcer patients and tested their various resistance models utilizing whole genome sequencing. Susceptibility studies were applied using broth microdilution assay showing the impact of meropenem/ciprofloxacin susceptibility at higher concentrations, paving the way to design combinational drug examinations against these extensive drug resistance strains. The drug influence was significantly superior when the meropenem was utilized in combination with ciprofloxacin against VIT PC 7 and VIT PC 9, representing the increase of drug susceptibility by fourfold and eightfold (166).
In study performed by Pankuch et al. ciprofloxacin-meropenem combination was tested against 40 strains of A. baumannii. The micrograms per milliliter (MICs) of the antibiotics alone were as follows: ciprofloxacin 0.06–256 and meropenem 0.12–256. Ciprofloxacin plus meropenem, at 3 h, yielded synergy at sub-inhibitory concentrations (MICs) of ciprofloxacin (0.12 to 0.25) and meropenem (0.25) for two strains. At 24 h, the antibiotics indicated synergy against 18 strains at sub-inhibitory ciprofloxacin and meropenem concentrations of 0.12–16 and 0.25–2 μg/ml, respectively (164).
In other study by Lu et al. the in vitro antibacterial activity of meropenem combined with ciprofloxacin, was tested against clinically isolated XDR A. baumannii. The main actions of ciprofloxacin combined with meropenem were additive (56%) and indifference (44%) with synergistic and antagonistic effects (167). In the study of Sun et al. time-kill assay and checkerboard assay were conducted to study the combination effects in vitro. There was only one strain of A. baumannii for which ciprofloxacin plus meropenem indicated synergistic effect (168).
About Enterobacteriaceae spp. for example, in study performed by Ramadan et al. meropenem– ciprofloxacin combination showed indifferent effect (n = 52, 100%) on all carbapenem-resistant K. pneumoniae isolates, while meropenem–colistin combination indicated 25% synergism, and 59.6% indifference (169). Also in the study of Karki et al. the extensively drug resistant (XDR) isolates were tested for antimicrobial synergy and the results were interpreted as additive, synergistic, indifferent or antagonistic determining fractional inhibitory concentration (FIC) of the antibiotics. These isolates comprised E. coli, K. pneumoniae, Acinetobacter baumannii, and P. aeruginosa. All of the XDR isolates indicated “indifference” to the combination of meropenem- ciprofloxacin whereas few isolates indicated “antagonism” when tested with amikacin- ciprofloxacin and meropenem-colistin (170).
A combination of colistin with either of tobramycin or ciprofloxacin has displayed synergism (45.45%; five out of 11 isolates) against MDR K. pneumoniae isolates (171). The isolates' MICs ranged from 0.25 to 32 μg ml−1 for fosfomycin and from 1 to 1,024 μg ml−1 for CIP. The combination of fosfomycin with ciprofloxacin reflected 6% synergy on biofilm formation by MDR urinary isolates of E. coli. The combination also diminished the MIC of each antibiotic (22).
Synergistic interplays (interplays indices 0.69–0.83; P < 0.05) were found between amphotericin B (0.07–0.31 mg/L) and either ciprofloxacin (0.19–7.65 mg/L) or levofloxacin (0.41–32.88 mg/L) against Candida albicans and Aspergillus fumigatus. Synergy (interplays indices 0.56–0.87; P < 0.05) was also discovered between voriconazole (0.09–0.14 mg/L) and ciprofloxacin (0.22–11.41 mg/L), as well as between caspofungin (8.94–22.07 mg/L) and levofloxacin (0.14–5.17 mg/L) against A. fumigatus. Ciprofloxacin could elevate the activity of antifungal agents against both C. albicans and A. fumigatus (172).
In vitro and in vivo examinations have highlighted that tigecycline in combination with ciprofloxacin is a powerful choice for treating invasive Vibrio vulnificus infection. An in vitro time-kill assay manifested synergism between tigecycline and ciprofloxacin. The survival rate was remarkably higher in mice treated with tigecycline plus ciprofloxacin than those treated with cefotaxime plus minocycline. Vancomycin-ciprofloxacin combination can be synergic against enterococci resistant to both vancomycin and ciprofloxacin. Still, it would be unlikely to have any excellence in treating enterococcal infections due to the high concentrations needed (173).
Nanoparticles (NPs), particles with a size of 1–1,000 nm (commonly 5–350 nm in diameter), are made of any biocompatible substance. Different studies have reported acceptable antibacterial activity for NPs even against biofilm community of bacteria (174). To this end, the combined use of NPs with different antibiotics such as ciprofloxacin was considered by researchers for inhibition of bacterial growth and elimination of the biofilm community of these microorganisms.
In this concept, in the recently published study the authors synthesized embelin (Emb, isolated from Embelia tsjeriam-cottam)-chitosan-gold NPs (Emb-Chi-Au) were evaluated for their potential synergistic activity with ciprofloxacin by checker boarding assay and time-kill curve analysis. The NPs diminished the MIC of ciprofloxacin by 16- and 4-fold against MDR P. aeruginosa and E. coli strains, respectively. Furthermore, FIC records with ≤0.5 values affirmed the synergy between the ciprofloxacin and Emb-Chi-Au NPs, further confirmed at ½ MICs in both P. aeruginosa and E. coli, using time-kill curve analysis. In addition, Emb indicated the efflux pump-inhibitory potentials against both the organisms under consideration. Hence, the synergistic application of ciprofloxacin with Emb-Chi-Au NPs showed inhibitory impacts on two of the most MDR bacteria. To this end, the authors proposed that the inhibition of bacterial efflux pumps by NPs must have retained the concentrations of ciprofloxacin inside the cell, which acted against bacterial DNA topoisomerase/gyrase (175).
In addition to mentioned NPs, AgNPs are used in different studies to enhance ciprofloxacin efficacy. In one of these studies, the authors surveyed the synergistic bactericidal impact of AgNPs and ciprofloxacin on different bacteria such as Pseudomonas solanocearum, Pseudomonas syringae, Xanthomonas malvacearum, and Xanthomonas campestris. When 0.2 mM of AgNPs were combined with 1 μg of ciprofloxacin, the antiphytopathogenic activity was surprisingly expanded to 36, 40, 33, and 35 mm against all the mentioned bacteria, respectively. Similarly, MIC and minimum bactericidal concentration (MBC) values were diminished significantly, indicating the synergistic activity between AgNPs and ciprofloxacin (176).
In line with these results, Nikparast et al. reported that the combined antibacterial activity of ciprofloxacin with AgNPs declined the MIC of antibiotics from 0.125 to 0.0625 μg/ml toward P. aeruginosa. Ciprofloxacin MIC against P. syringae reduced from 0.25 to 0.0625 μg/ml in combination with 6.25, 12.5, and 25 μg/ml of AgNPs (177).
In addition to AgNPs, zinc oxide (ZnO) was another metal-NPs that was used in combination with ciprofloxacin for enhancement of antibacterial activity. To this end, the authors synthesized ZnONPs, functionalizing them by Glu and conjugating them with thiosemicarbazid (TSC) to increase their efficacy against ciprofloxacin -resistant S. aureus. The results showed the synergistic activity of ciprofloxacin and synthesized NPs against ciprofloxacin -resistant S. aureus. Thus, the authors introduced ZnO@Glu–TSC NPs as a promising new antibacterial agent for therapeutic and preventive purposes (178).
The exact interaction of metal-NPs and ciprofloxacin has not been reported yet. However, it seems that these NPs, after attachment to the bacterial cell membrane, lead to the formation of gap on the bacterial cell walls, and damage to the cell membrane, thereby allowing the ciprofloxacin to enter the periplasm of the bacterial cells. Therefore, the combination of ciprofloxacin and metal NPs yield novel antimicrobial agents with synergistic properties that could be exploited for higher antibacterial activity. However, due to the high toxicity of these NPs for human cells, further investigation needs to be performed to evaluate the safety of these NPs for medical applications.
It's noteworthy to mention that, other studies that have used of nanoplatforms for enhancement of ciprofloxacin efficacy are presented in Table 4. Based on this table and mentioned studies, ciprofloxacin delivery can be modified by encapsulating with or incorporating different polymeric NPs such as poly lactic-co-glycolic acid (PLGA), chitosan, arginine, albumin, and other organic and inorganic nanostructure systems (179). Furthermore, studies have also shown that nano-platforms could enhance the efficiency of ciprofloxacin against bacterial cells, interfere with the biofilm community, enhance the penetration and protect the drug from deactivation or efflux (Figure 4).
Table 4. The studies have used nano-platform for the enhancement of ciprofloxacin against different bacteria.
Figure 4. Used nano-platforms for enhancement of ciprofloxacin efficacy against bacteria. (A) Nanoparticles could boost the antibacterial function of ciprofloxacin by inhibition of efflux-pumps. (B) Nanoparticles increase the antibacterial activity and penetration of ciprofloxacin to the (B) dipper layers of biofilm and (C) body organs such as the skin.
Recent studies indicate that new antimicrobial agents are required to reduce the toxicity of conventional antimicrobial agents. Furthermore, combination therapy could improve the efficacy of different antimicrobials (199). In this regard, the combined ciprofloxacin and different natural products were considered to inhibit bacterial growth.
The recently published study used the checkerboard microdilution and evaluated in vitro interaction between Thymbra spicata L. extracts and certain antibiotics such as amikacin, cefotaxime, ampicillin, and ciprofloxacin against MDR K. pneumonia and S. aureus. The combination of amikacin, cefotaxime, and ampicillin plus plant extraction showed synergistic activity against S. aureus. In contrast, the joint activity of plant extract with ciprofloxacin indicated indifferent and additive activity. Furthermore, ciprofloxacin showed an indifference and additive effect with sensitive and resistant K. pneumoniae strains when combined with all T. spicata extracts (200).
Based on the checkerboard synergy technique, nbutanolic Cyclamen coum extract in combination with ciprofloxacin represented a synergistic effect against P. aeruginosa biofilms (ΣFBIC = 0.496) (201). The extricates of four customarily utilized therapeutic plants, i.e. Plumbago zeylanica (root), Hemidesmus indicus (stem), Acorus calamus (rhizome), and Holarrhena antidysenterica (bark), were examined against the clinical isolates of MRSA and methicillin-sensitive S. aureus, P. zeylanica and H. antidysenterica demonstrated synergism with ciprofloxacin (202).
Additionally, the MIC findings of another investigation uncovered that combinatorial impacts of Sami-Hyanglyun-Hwan ethanol extract (SHEE) with ciprofloxacin had 2–32-fold reduction in concentration as those needed by SHHE alone. The antibacterial activity of SHHE obviously declined the MICs of ciprofloxacin against S. aureus strains. The checkerboard method suggested that the combinations of SHHE with ciprofloxacin had a partial methicillin-resistant synergistic or synergistic impact on MRSA. The time-kill curves also proved that S. aureus in combination with SHHE and ciprofloxacin treatment, lessened the bacterial counts significantly after 24 h (203). Chrysoeriol had a notable synergistic impact when combined with ciprofloxacin and oxacillin against epidemic methicillin-resistant S. aureus 15 (EMRSA-15) and EMRSA-16, respectively, both of which are the UK epidemic MRSA strains (204). When biochanin A (BCA) was combined with ciprofloxacin, the FIC index data exhibited that there was synergy in all 12 of the S. aureus strains examined. The outcomes of time-kill tests and agar diffusion tests affirmed synergy between BCA and ciprofloxacin against S. aureus strains. These results proposed that BCA can be combined with FQs to produce a potent antimicrobial agent (205).
On the other hand, the results of another study showed that the combination of Propolis, a mixture of a complex chemical composition containing essential oils, balms, pollen, minerals, vitamins, and proteins, with ciprofloxacin has shown an antagonistic effect against MRSA. The ciprofloxacin action is diminished when combined with Propolis. In five of the seven strains studied, further growth of MRSA was found in combinations in concentrations of each substance separately applied. Hence, the combination of both substances is noxious (206).
Thus, combining ciprofloxacin with natural products could lead to several advantages such as boosted potency, a reduced dose of drugs needed and minimized toxicity, which ultimately helps inhibit different bacteria even MDR isolated. Although, the exact mechanism by which natural products synergizes with ciprofloxacin was not investigated in the studies mentioned above. Therefore, additional molecular and in vivo studies are needed to confirm the practical utility of these combinations. Finally, in addition to natural products, the combined use of ciprofloxacin with various natural compounds, which have antimicrobial properties, such as curcumin, eugenol, cinnamomum, and carvacrol, should be considered.
Photodynamic therapy (PDT) has been identified as an effective treatment for the inhibition of bacterial infections such as E. faecalis infection in root canal dentine (207). In this method, a specific wavelength excites a photosensitizer, photoactive dye, and leads to the generation of singlet oxygen or other reactive oxygen species (ROS) that can eliminate the target bacteria (208). Methylene blue (MB), due to various characteristics such as low molecular weight and toxicity in mammalian cells, and hydrophilicity, are reported as a potential photosensitizer for PDT (209). In recent years, different methods have been used to enhancement of PDT efficacy for the inhibition of bacterial infections. The use of antibiotics in combination with PDT is one of these methods. In this regard, researchers used ciprofloxacin to boost the performance of the PDT.
To this end, the findings of the recently published study showed that S. aureus, even with the lowest ciprofloxacin and MB concentrations (0.0625 and 6.25 μg/mL, respectively), bacterial killing was remarkably developed when compared to MB–PDT alone for the exact light dose. The best findings were achieved after the combination treatment of PDT with, followed by ciprofloxacin on biofilms, which enhanced bacterial diminishment on biofilms, resulting in a 5.4 log diminishment for S. aureus biofilm and approximately seven logs for E. coli biofilm (210). In another investigation also, the authors reported that essential oil obtained from Eugenia jambolana interferes with the action of antibiotics against bacteria exposed to LED lights. This trial showed that irradiation of E. coli and S. aureus with blue or red light in the presence of ciprofloxacin is more beneficial than antibiotic monotherapy (211). Therefore, PDT can destroy the bacterial community using various possible mechanisms such as interference with cellular hemostasis and membrane permeability, modulation of DNA and RNA synthesis, and alkalization of the cytoplasm and cell membrane depolarization. In this regard, the combine use of PDT and ciprofloxacin can be considered for treatment of bacterial infections especially infection that caused by MDR bacteria; however, the data about this kind of treatment is very limited and more confirmatory studies are needed.
Bacteriophages (phages), viruses that infected bacteria, were first discovered in the middle of the 20th century, and due to their great function in the inhibition of MDR bacteria, was considered by scientist as non-antibiotic approaches for the treatment of bacterial infections. Eukaryotic cells have no receptors for phages; therefore, they can be used to treat bacterial infections (212). A phage cocktail containing two or more bacteriophage mixtures with different host ranges in a single suspension could lead to a better antibacterial effect than single phage therapy (213, 214). Phages could penetrate the dipper layer of biofilm and damage its structure by producing natural enzymes. Additionally, endolysins are produced at the end of the lytic cycle of the phages. This enzyme could destroy bacterial cell walls by the cleavage of peptidoglycan (215, 216). To this end, combination therapy of antibiotics and phage not only causes a reduction in the number of bacteria but also can be related to the management of phage-resistant bacteria levels (217). Therefore, this section will discuss the combination therapy of ciprofloxacin and phage for treating bacterial infections (Figure 5).
Recently published studies reported additive or synergistic effects of ciprofloxacin and phage combination (134, 218–223). Gurney et al. reported that phages could interact with different structures of P. aeruginosa, such as lipopolysaccharide structure (LPS) and the efflux pumps. Therefore, phages could increase the permeability of bacteria and the drug dosage by inhibiting efflux pumps (134). Another study also indicated that combining phage cocktail and ciprofloxacin could increase the number of MDR P. aeruginosa strains' susceptibility to this antibiotic. This combination therapy also resulted in the re-sensitization of P. aeruginosa to ciprofloxacin. Noteworthy, the animal wound model result showed that phage-only treated mouse wounds had mutations for phage receptors; thus, these animals were resistant to infection with phage. However, these mutations were not detected in the combination treatment bacteria, suggesting that the treatment with phages and antibiotics reduced the incidence of the bacteria becoming resistant to the phage treatment (218). In another investigation, the authors reported that intratracheally treating mice (with acute lung infection) with phage- ciprofloxacin combination powder remarkably decreased the bacterial load in the lungs. In contrast, single treatments failed to reduce the bacterial count (221).
Therefore, the combination use of phage–antibiotic is a promising approach for in inhibition of MDR bacteria, especially P. aeruginosa. The phage- ciprofloxacin synergistic effect in killing bacterial cells could be due to a selective pressure under which the bacteria mutate in one trait to improve fitness while suffering a decrease in another trait. A recently published study reported an evolutionary trade-off effect when phage treatment imposed a selective pressure on MDR bacteria. When bacteria lose their receptor for phage binding, they resist to infection by phages. However, in this condition, bacteria regained sensitivity to a different antibiotic, such as ciprofloxacin. Another possible reason might be morphologic changes of bacterial cells when exposed to sub-inhibitory concentrations of antibiotics. In this circumstance, antibiotic exposure led to the elongation of bacterial cells but did not divide, which could improve phage assembly and maturation (221, 224, 225).
Additionally, as mentioned in previous parts of the manuscript, the biofilm community of bacteria is one of the most important challenges in treating infection. Given that, the combination uses of ciprofloxacin and phages have been considered by scientists for the elimination of bacterial biofilm. Tkhilaishvili et al. reported that a higher concentration of ciprofloxacin is required to suppress the growth of dual-species biofilms compared to monospecies biofilms. On the other hand, combining phages with ciprofloxacin significantly enhanced the anti-biofilm activity of both antimicrobials with complete eradication of S. aureus/P. aeruginosa biofilms (226). In line with these findings, a recently published study also reported that antibiotics such as ciprofloxacin and phages alone had a modest effect in killing bacteria in biofilm community.
Nonetheless, when these compounds were used at the same time, especially when ciprofloxacin was added sequentially after 6 h of phage treatment, a significant enhancement in the killing activity was detected (227). It seems phages via depolymerases could degrade the biofilm matrix, consequently enhancing antibiotic penetration into the deeper layers of the biofilm (227–229). However, depolymerases was not detected in some phages; therefore, the mentioned phenomenon might not have been responsible for the synergistic action of the phages and antibiotics combined therapy. In these cases, it's possible that phages using of biofilm void spaces could access the dipper layers of the biofilm. Afterward, phages replicate in the biofilm's deeper layer and interrupt the biofilm's extracellular matrix. The addition of antibiotics following this interruption causes an improved bacterial killing due to the deeper penetration of phages and antibiotics (227, 230).
Taken together, combining ciprofloxacin with phages can be synergistic in destroying the bacteria in the biofilm community; hence, this combination therapy is a promising candidate for treating infections are caused by MDR bacteria. However, some important challenges, such as the time of antibiotic application, the concentration of antibiotics, and the exact interaction of phages with eukaryotic cells, should be evaluated in further studies.
Finally, its noteworthy that recently published studies that have used various antibacterial agents to enhance ciprofloxacin efficacy against different bacterial infections in animal models and in vivo studies are presented in Table 5.
Table 5. Studies have used various approaches to enhance ciprofloxacin activity against bacterial infection in animal models and in vivo.
Ciprofloxacin's potential for the treatment of a large spectrum of bacterial infections led to the overuse of this drug in clinical practice and developed alarming levels of ciprofloxacin resistance as a consequence of heavy use. To preserve this beneficial agent, prescribers must ensure that ciprofloxacin is a proper choice and administer enough doses to limit the risk of selecting resistant mutant bacterial subpopulations. The increasing incidence of ciprofloxacin-resistant pathogens jeopardizes the continued empiric use of ciprofloxacin and raises the urgent need to develop novel ciprofloxacin derivatives potent against both drug-susceptible and drug-resistant pathogens and discover useful synergism between ciprofloxacin and other antibacterial agents. As mentioned earlier in this study, recent studies have reported a wide range of synergism between ciprofloxacin and other antibacterial agents. Therefore, the combination use of ciprofloxacin and other antibiotics and antibacterial agent should be considered in future studies because combination therapy could increase antibacterial performance of ciprofloxacin especially against MDR strains.
SK and MH conceived and designed the study. AS, MAr, MK, MAb, MG, MH, and SK contributed in comprehensive research. AS and MH participated in editing the manuscript. All authors have read and approved the manuscript.
We greatly appreciate the input from BioRender team (BioRender.com) for their collaboration with us in figures design.
The authors declare that the research was conducted in the absence of any commercial or financial relationships that could be construed as a potential conflict of interest.
All claims expressed in this article are solely those of the authors and do not necessarily represent those of their affiliated organizations, or those of the publisher, the editors and the reviewers. Any product that may be evaluated in this article, or claim that may be made by its manufacturer, is not guaranteed or endorsed by the publisher.
FQs, fluoroquinolones; FDA, Food and Drug Administration; UTI, urinary tract infection; WHO, World Health Organization; TB, tuberculosis; MDR, multidrug-resistant; gyrA, DNA gyrase genes; QRDR, quinolone resistance determining region; PMQR, plasmid-mediated quinolone resistance gene; MIC, minimal inhibitory concentrations; SNPs, single nucleotide polymorphisms; MRSA, methicillin-resistant Staphylococcus aureus; MBC, minimum bactericidal concentration; PDT, photodynamic therapy; ROS, reactive oxygen species; MB, methylene blue; LPS, lipopolysaccharide structure.
1. Hooper DC, Jacoby GA. Topoisomerase inhibitors: fluoroquinolone mechanisms of action and resistance. Cold Spring Harb Perspect Med. (2016) 6:a025320. doi: 10.1101/cshperspect.a025320
2. Yang Y, Niehaus KE, Walker TM, Iqbal Z, Walker AS, Wilson DJ, et al. Machine learning for classifying tuberculosis drug-resistance from DNA sequencing data. Bioinformatics. (2018) 34:1666–71. doi: 10.1093/bioinformatics/btx801
3. Heidary M, Nasiri MJ. Why has HIV/AIDS prevalence increased in Iran? Clin Infect Dis. (2016) 63:846. doi: 10.1093/cid/ciw361
4. Mabonga E, Parkes-Ratanshi R, Riedel S, Nabweyambo S, Mbabazi O, Taylor C, et al. Complete ciprofloxacin resistance in gonococcal isolates in an urban Ugandan clinic: findings from a cross-sectional study. Int J STD AIDS. (2019) 30:256–63. doi: 10.1177/0956462418799017
5. Campoli-Richards DM, Monk JP, Price A, Benfield P, Todd PA, Ward A. Ciprofloxacin. A review of its antibacterial activity, pharmacokinetic properties and therapeutic use. Drugs. (1988) 35:373–447. doi: 10.2165/00003495-198835040-00003
6. Zhang GF, Liu X, Zhang S, Pan B, Liu ML. Ciprofloxacin derivatives and their antibacterial activities. Eur J Med Chem. (2018) 146:599–612. doi: 10.1016/j.ejmech.2018.01.078
7. National Center for Biotechnology Information (2022). PubChem Compound Summary for CID 2764 CRF. (2022). Available online at: https://pubchem.ncbi.nlm.nih.gov/compound/2022
8. LeBel M. Ciprofloxacin: chemistry, mechanism of action, resistance, antimicrobial spectrum, pharmacokinetics, clinical trials, and adverse reactions. Pharmacotherapy. (1988) 8:3–33. doi: 10.1002/j.1875-9114.1988.tb04058.x
9. Bush NG, Diez-Santos I, Abbott LR, Maxwell A. Quinolones: mechanism, lethality and their contributions to antibiotic resistance. Molecules. (2020) 25:5662. doi: 10.3390/molecules25235662
10. Serizawa M, Sekizuka T, Okutani A, Banno S, Sata T, Inoue S, et al. Genomewide screening for novel genetic variations associated with ciprofloxacin resistance in Bacillus anthracis. Antimicrob Agents Chemother. (2010) 54:2787–92. doi: 10.1128/AAC.01405-09
11. Knoll KE, Lindeque Z, Adeniji AA, Oosthuizen CB, Lall N, Loots DT. Elucidating the antimycobacterial mechanism of action of ciprofloxacin using metabolomics. Microorganisms. (2021) 9:1158. doi: 10.20944/preprints202104.0443.v1
12. Liu C, Shi J, Dai Q, Yin X, Zhang X, Zheng A. In-vitro and in-vivo evaluation of ciprofloxacin liposomes for pulmonary administration. Drug Dev Ind Pharm. (2015) 41:272–8. doi: 10.3109/03639045.2013.858740
13. Rehman A, Patrick WM, Lamont IL. Mechanisms of ciprofloxacin resistance in Pseudomonas aeruginosa: new approaches to an old problem. J Med Microbiol. (2019) 68:1–10. doi: 10.1099/jmm.0.000873
14. Chin NX, Neu HC. Ciprofloxacin, a quinolone carboxylic acid compound active against aerobic and anaerobic bacteria. Antimicrob Agents Chemother. (1984) 25:319–26. doi: 10.1128/AAC.25.3.319
15. Zeiler HJ, Grohe K. The in vitro and in vivo activity of ciprofloxacin. Eur J Clin Microbiol. (1984) 3:339–43. doi: 10.1007/BF01977490
16. Eliopoulos GM, Gardella A, Moellering RC Jr. In vitro activity of ciprofloxacin, a new carboxyquinoline antimicrobial agent. Antimicrob Agents Chemother. (1984) 25:331–5. doi: 10.1128/AAC.25.3.331
17. Rodriguez JC, Ruiz M, Climent A, Royo G. In vitro activity of four fluoroquinolones against Mycobacterium tuberculosis. Int J Antimicrob Agents. (2001) 17:229–31. doi: 10.1016/S0924-8579(00)00337-X
18. Manzulli V, Fasanella A, Parisi A, Serrecchia L, Donatiello A, Rondinone V, et al. Evaluation of in vitro antimicrobial susceptibility of Bacillus anthracis strains isolated during anthrax outbreaks in Italy from 1984 to 2017. J Vet Sci. (2019) 20:58–62. doi: 10.4142/jvs.2019.20.1.58
19. Reeves DS, Bywater MJ, Holt HA, White LO. In-vitro studies with ciprofloxacin, a new 4-quinolone compound. J Antimicrob Chemother. (1984) 13:333–46. doi: 10.1093/jac/13.4.333
20. Shrire L, Saunders J, Traynor R, Koornhof HJ. A laboratory assessment of ciprofloxacin and comparable antimicrobial agents. Eur J Clin Microbiol. (1984) 3:328–32. doi: 10.1007/BF01977488
21. El-Wafa WMA, Ibrahim YM. In vitro activity of fosfomycin in double and triple combinations with imipenem, ciprofloxacin and tobramycin against multidrug-resistant Escherichia coli. Curr Microbiol. (2020) 77:755–61. doi: 10.1007/s00284-019-01871-w
22. Sugathan S, Mandal J. An in vitro experimental study of the effect of fosfomycin in combination with amikacin, ciprofloxacin or meropenem on biofilm formation by multidrug-resistant urinary isolates of Escherichia coli. J Med Microbiol. (2019) 68:1699–706. doi: 10.1099/jmm.0.001061
23. Drago L, De Vecchi E, Mombelli B, Nicola L, Valli M, Gismondo MR. Activity of levofloxacin and ciprofloxacin against urinary pathogens. J Antimicrob Chemother. (2001) 48:37–45. doi: 10.1093/jac/48.1.37
24. Kwiecinska-Pirog J, Skowron K, Bartczak W, Gospodarek-Komkowska E. The ciprofloxacin impact on biofilm formation by Proteus mirabilis and P. vulgaris strains. Jundishapur J Microbiol. (2016) 9:e32656. doi: 10.5812/jjm.32656
25. Hoogkamp-Korstanje JA. In-vitro activities of ciprofloxacin, levofloxacin, lomefloxacin, ofloxacin, pefloxacin, sparfloxacin and trovafloxacin against gram-positive and gram-negative pathogens from respiratory tract infections. J Antimicrob Chemother. (1997) 40:427–31. doi: 10.1093/jac/40.3.427
26. Group tCAS. Determination of the antimicrobial susceptibilities of Canadian isolates of Haemophilus influenzae, Streptococcus pneumoniae and Moraxella catarrhalis. J Antimicrob Chemother. (1999) 43(suppl_1):25–30. doi: 10.1093/jac/43.suppl_1.25
27. Hoogkamp-Korstanje JA, Dirks-Go SI, Kabel P, Manson WL, Stobberingh EE, Vreede RW, et al. Multicentre in-vitro evaluation of the susceptibility of Streptococcus pneumoniae, Haemophilus influenzae and Moraxella catarrhalis to ciprofloxacin, clarithromycin, co-amoxiclav and sparfloxacin. J Antimicrob Chemother. (1997) 39:411–4. doi: 10.1093/jac/39.3.411
28. Flamm RK, Rhomberg PR, Huband MD, Farrell DJ. In vitro activity of delafloxacin tested against isolates of Streptococcus pneumoniae, Haemophilus influenzae, and Moraxella catarrhalis. Antimicrob Agents Chemother. (2016) 60:6381–5. doi: 10.1128/AAC.00941-16
29. Saito A, Koga H, Shigeno H, Watanabe K, Mori K, Kohno S, et al. The antimicrobial activity of ciprofloxacin against Legionella species and the treatment of experimental Legionella pneumonia in guinea pigs. J Antimicrob Chemother. (1986) 18:251–60. doi: 10.1093/jac/18.2.251
30. Dubois J, St-Pierre C. In vitro activity of gatifloxacin, compared with ciprofloxacin, clarithromycin, erythromycin, and rifampin, against Legionella species. Diagn Microbiol Infect Dis. (1999) 33:261–5. doi: 10.1016/S0732-8893(98)00150-3
31. Stout JE, Arnold B, Yu VL. Comparative activity of ciprofloxacin, ofloxacin, levofloxacin, and erythromycin against Legionella species by broth microdilution and intracellular susceptibility testing in HL-60 cells. Diagn Microbiol Infect Dis. (1998) 30:37–43. doi: 10.1016/S0732-8893(97)00174-0
32. Blondeau JM, Yaschuk Y. In vitro activities of ciprofloxacin, cefotaxime, ceftriaxone, chloramphenicol, and rifampin against fully susceptible and moderately penicillin-resistant Neisseria meningitidis. Antimicrob Agents Chemother. (1995) 39:2577–9. doi: 10.1128/AAC.39.11.2577
33. Slaney L, Chubb H, Ronald A, Brunham R. In-vitro activity of azithromycin, erythromycin, ciprofloxacin and norfloxacin against Neisseria gonorrhoeae, Haemophilus ducreyi, and Chlamydia trachomatis. J Antimicrob Chemother. (1990) 25(Suppl A):1–5. doi: 10.1093/jac/25.suppl_A.1
34. Allen GP, Deao KM, Hill SA, Schipelliti SM, Tran T. In vitro evaluation of antimicrobial resistance selection in Neisseria gonorrhoeae. Int J Antimicrob Agents. (2021) 58:106417. doi: 10.1016/j.ijantimicag.2021.106417
35. Isenberg HD, Alperstein P, France K. In vitro activity of ciprofloxacin, levofloxacin, and trovafloxacin, alone and in combination with beta-lactams, against clinical isolates of Pseudomonas aeruginosa, Stenotrophomonas maltophilia, and Burkholderia cepacia. Diagn Microbiol Infect Dis. (1999) 33:81–6. doi: 10.1016/S0732-8893(98)00126-6
36. Klinger JD, Aronoff SC. In-vitro activity of ciprofloxacin and other antibacterial agents against Pseudomonas aeruginosa and Pseudomonas cepacia from cystic fibrosis patients. J Antimicrob Chemother. (1985) 15:679–84. doi: 10.1093/jac/15.6.679
37. Chalkley LJ, Koornhof HJ. Antimicrobial activity of ciprofloxacin against Pseudomonas aeruginosa, Escherichia coli, and Staphylococcus aureus determined by the killing curve method: antibiotic comparisons and synergistic interactions. Antimicrob Agents Chemother. (1985) 28:331–42. doi: 10.1128/AAC.28.2.331
38. Heinemann B, Wisplinghoff H, Edmond M, Seifert H. Comparative activities of ciprofloxacin, clinafloxacin, gatifloxacin, gemifloxacin, levofloxacin, moxifloxacin, and trovafloxacin against epidemiologically defined Acinetobacter baumannii strains. Antimicrob Agents Chemother. (2000) 44:2211–3. doi: 10.1128/AAC.44.8.2211-2213.2000
39. Kennedy N, Fox R, Kisyombe GM, Saruni AO, Uiso LO, Ramsay AR, et al. Early bactericidal and sterilizing activities of ciprofloxacin in pulmonary tuberculosis. Am Rev Respir Dis. (1993) 148:1547–51. doi: 10.1164/ajrccm/148.6_Pt_1.1547
40. Hoffner SE, Gezelius L, Olsson-Liljequist B. In-vitro activity of fluorinated quinolones and macrolides against drug-resistant Mycobacterium tuberculosis. J Antimicrob Chemother. (1997) 40:885–8. doi: 10.1093/jac/40.6.885
41. World Health Organization. WHO Consolidated Guidelines on Drug-resistant Tuberculosis Treatment. Geneva: World Health Organization (2019).
42. Chen TC, Lu PL, Lin CY, Lin WR, Chen YH. Fluoroquinolones are associated with delayed treatment and resistance in tuberculosis: a systematic review and meta-analysis. Int J Infect Dis. (2011) 15:e211–6. doi: 10.1016/j.ijid.2010.11.008
43. Chesson HW, Kirkcaldy RD, Gift TL, Owusu-Edusei K Jr, Weinstock HS. Ciprofloxacin resistance 1116 and gonorrhea incidence rates in 17 cities, United States, 1991-2006. Emerg Infect Dis. (2014) 20:612–9. doi: 10.3201/eid2004.131288
44. Andrade AA, de Pilla Varotti F, de Freitas IO, de Souza MV, Vasconcelos TR, Boechat N, et al. Enhanced activity of mefloquine and artesunic acid against Plasmodium falciparum in vitro and P. berghei in mice by combination with ciprofloxacin. Eur J Pharmacol. (2007) 558:194–8. doi: 10.1016/j.ejphar.2006.11.061
45. Afriyie DK, Adu LB, Dzradosi M, Amponsah SK, Ohene-Manu P, Manu-Ofei F. Comparative in vitro activity of ciprofloxacin and levofloxacin against isolated uropathogens in Ghana: a pilot study. Pan Afr Med J. (2018) 30:194. doi: 10.11604/pamj.2018.30.194.15457
46. Fasugba O, Gardner A, Mitchell BG, Mnatzaganian G. Ciprofloxacin resistance in community- and hospital-acquired Escherichia coli urinary tract infections: a systematic review and meta-analysis of observational studies. BMC Infect Dis. (2015) 15:545. doi: 10.1186/s12879-015-1282-4
47. Gupta K, Hooton TM, Naber KG, Wullt B, Colgan R, Miller LG, et al. International clinical practice guidelines for the treatment of acute uncomplicated cystitis and pyelonephritis in women: a 2010 update by the Infectious Diseases Society of America and the European Society for Microbiology and Infectious Diseases. Clin Infect Dis. (2011) 52:e103–20. doi: 10.1093/cid/ciq257
48. Kang CI, Kim J, Park DW, Kim BN, Ha US, Lee SJ, et al. Clinical practice guidelines for the antibiotic treatment of community-acquired urinary tract infections. Infect Chemother. (2018) 50:67–100. doi: 10.3947/ic.2018.50.1.67
49. Blaettler L, Mertz D, Frei R, Elzi L, Widmer AF, Battegay M, et al. Secular trend and risk factors for antimicrobial resistance in Escherichia coli isolates in Switzerland 1997-2007. Infection. (2009) 37:534–9. doi: 10.1007/s15010-009-8457-0
50. Hickerson AD, Carson CC. The treatment of urinary tract infections and use of ciprofloxacin extended release. Expert Opin Investig Drugs. (2006) 15:519–32. doi: 10.1517/13543784.15.5.519
51. Stass H, Nagelschmitz J, Willmann S, Delesen H, Gupta A, Baumann S. Inhalation of a dry powder ciprofloxacin formulation in healthy subjects: a phase I study. Clin Drug Investig. (2013) 33:419–27. doi: 10.1007/s40261-013-0082-0
52. Mogayzel PJ Jr, Naureckas ET, Robinson KA, Brady C, Guill M, Lahiri T, et al. Cystic Fibrosis Foundation pulmonary guideline pharmacologic approaches to prevention and eradication of initial Pseudomonas aeruginosa infection. Ann Am Thorac Soc. (2014) 11:1640–50. doi: 10.1513/AnnalsATS.201404-166OC
53. McShane PJ, Weers JG, Tarara TE, Haynes A, Durbha P, Miller DP, et al. Ciprofloxacin dry powder for inhalation (ciprofloxacin DPI): technical design and features of an efficient drug-device combination. Pulm Pharmacol Ther. (2018) 50:72–9. doi: 10.1016/j.pupt.2018.03.005
54. Dore MP, Tadeu V, Are B, Mura I, Fanciulli G, Massarelli G, et al. Efficacy of a “rescue” ciprofloxacin-based regimen for eradication of Helicobacter pylori infection after treatment failures. Gastroenterol Res Pract. (2012) 2012:484591. doi: 10.1155/2012/484591
55. Chen K, Chan EWC, Chen S. Evolution and transmission of a conjugative plasmid encoding both ciprofloxacin and ceftriaxone resistance in Salmonella. Emerg Microbes Infect. (2019) 8:396–403. doi: 10.1080/22221751.2019.1585965
56. Fong IW, Ledbetter WH, Vandenbroucke AC, Simbul M, Rahm V. Ciprofloxacin concentrations in bone and muscle after oral dosing. Antimicrob Agents Chemother. (1986) 29:405–8. doi: 10.1128/AAC.29.3.405
57. Kish TD, Chang MH, Fung HB. Treatment of skin and soft tissue infections in the elderly: a review. Am J Geriatr Pharmacother. (2010) 8:485–513. doi: 10.1016/S1543-5946(10)80002-9
58. Rosenfeld RM, Brown L, Cannon CR, Dolor RJ, Ganiats TG, Hannley M, et al. Clinical practice guideline: acute otitis externa. Otolaryngol Head Neck Surg. (2006) 134:S4–23. doi: 10.1016/j.otohns.2006.02.014
59. Noonan KY, Kim SY, Wong LY, Martin IW, Schwartzman JD, Saunders JE. Treatment of ciprofloxacin-resistant ear infections. Otol Neurotol. (2018) 39:e837–42. doi: 10.1097/MAO.0000000000001966
60. Mofatteh MR, Shahabian Moghaddam F, Yousefi M, Namaei MH. A study of bacterial pathogens and antibiotic susceptibility patterns in chronic suppurative otitis media. J Laryngol Otol. (2018) 132:41–5. doi: 10.1017/S0022215117002249
61. Herbert DA. Successful oral ciprofloxacin therapy of Neisseria elongata endocarditis. Ann Pharmacother. (2014) 48:1529–30. doi: 10.1177/1060028014545355
62. Avery LM, Felberbaum CB, Hasan M. Ciprofloxacin for the treatment of Cardiobacterium hominis prosthetic valve endocarditis. IDCases. (2018) 11:77–9. doi: 10.1016/j.idcr.2018.01.016
63. Heldman AW, Hartert TV, Ray SC, Daoud EG, Kowalski TE, Pompili VJ, et al. Oral antibiotic treatment of right-sided staphylococcal endocarditis in injection drug users: prospective randomized comparison with parenteral therapy. Am J Med. (1996) 101:68–76. doi: 10.1016/S0002-9343(96)00070-8
64. Yasir M, Dutta D, Willcox MDP. Activity of antimicrobial peptides and ciprofloxacin against Pseudomonas aeruginosa biofilms. Molecules. (2020) 25:3834. doi: 10.3390/molecules25173843
65. Flemming HC, Wingender J, Szewzyk U, Steinberg P, Rice SA, Kjelleberg S. Biofilms: an emergent form of bacterial life. Nat Rev Microbiol. (2016) 14:563–75. doi: 10.1038/nrmicro.2016.94
66. Verderosa AD, de la Fuente-Núñez C, Mansour SC, Cao J, Lu TK, Hancock REW, et al. Ciprofloxacin-nitroxide hybrids with potential for biofilm control. Eur J Med Chem. (2017) 138:590–601. doi: 10.1016/j.ejmech.2017.06.058
67. Reffuveille F, Fuente-Núñez Cde L, Fairfull-Smith KE, Hancock RE. Potentiation of ciprofloxacin action against Gram-negative bacterial biofilms by a nitroxide. Pathog Dis. (2015) 73:ftv016. doi: 10.1093/femspd/ftv016
68. Verderosa AD, Mansour SC, de la Fuente-Núñez C, Hancock RE, Fairfull-Smith KE. Synthesis and evaluation of ciprofloxacin-nitroxide conjugates as anti-biofilm agents. Molecules. (2016) 21:841. doi: 10.3390/molecules21070841
69. Li B, Webster TJ. Bacteria antibiotic resistance: new challenges and opportunities for implant-associated orthopedic infections. J Orthop Res. (2018) 36:22–32. doi: 10.1002/jor.23656
70. Aslam B, Wang W, Arshad MI, Khurshid M, Muzammil S, Rasool MH, et al. Antibiotic resistance: a rundown of a global crisis. Infect Drug Resist. (2018) 11:1645–58. doi: 10.2147/IDR.S173867
71. Laponogov I, Veselkov DA, Crevel IM-T, Pan X-S, Fisher LM, Sanderson MR. Structure of an ‘open'clamp type II topoisomerase-DNA complex provides a mechanism for DNA capture and transport. Nucleic Acids Res. (2013) 41:9911–23. doi: 10.1093/nar/gkt749
72. Hooper DC, Jacoby GA. Mechanisms of drug resistance: quinolone resistance. Ann N Y Acad Sci. (2015) 1354:12–31. doi: 10.1111/nyas.12830
73. Fernández L, Hancock RE. Adaptive and mutational resistance: role of porins and efflux pumps in drug resistance. Clin Microbiol Rev. (2012) 25:661–81. doi: 10.1128/CMR.00043-12
74. Ruiz J, Pons MJ, Gomes C. Transferable mechanisms of quinolone resistance. Int J Antimicrob Agents. (2012) 40:196–203. doi: 10.1016/j.ijantimicag.2012.02.011
75. Rodríguez-Martínez JM, Machuca J, Cano ME, Calvo J, Martínez-Martínez L, Pascual A. Plasmid-mediated quinolone resistance: two decades on. Drug Resist Updat. (2016) 29:13–29. doi: 10.1016/j.drup.2016.09.001
76. Heidary M, Bahramian A, Hashemi A, Goudarzi M, Omrani VF, Eslami G, et al. Detection of acrA, acrB, aac (6′)-Ib-cr, and qepA genes among clinical isolates of Escherichia coli and Klebsiella pneumoniae. Acta Microbiol Immunol Hung. (2017) 64:63–9. doi: 10.1556/030.63.2016.011
77. Nakaminami H, Noguchi N, Sasatsu M. Fluoroquinolone efflux by the plasmid-mediated multidrug efflux pump QacB variant QacBIII in Staphylococcus aureus. Antimicrob Agents Chemother. (2010) 54:4107–11. doi: 10.1128/AAC.01065-09
78. Fonseca EL, Vicente ACP. Epidemiology of qnrVC alleles and emergence out of the Vibrionaceae family. J Med Microbiol. (2013) 62(Pt 10):1628–30. doi: 10.1099/jmm.0.062661-0
79. Zhao J-Y, Dang H. Coastal seawater bacteria harbor a large reservoir of plasmid-mediated quinolone resistance determinants in Jiaozhou Bay, China. Microbial Ecol. (2012) 64:187–99. doi: 10.1007/s00248-012-0008-z
80. Domínguez-Herrera J, Velasco C, Docobo-Pérez F, Rodríguez-Martínez J, López-Rojas R, Briales A, et al. Impact of qnrA1, qnrB1 and qnrS1 on the efficacy of ciprofloxacin and levofloxacin in an experimental pneumonia model caused by Escherichia coli with or without the GyrA mutation Ser83Leu. J Antimicrob Chemother. (2013) 68:1609–15. doi: 10.1093/jac/dkt063
81. Michon A, Allou N, Chau F, Podglajen I, Fantin B, Cambau E. Plasmidic qnrA3 enhances Escherichia coli fitness in absence of antibiotic exposure. PLoS ONE. (2011) 6:e24552. doi: 10.1371/journal.pone.0024552
82. Conley ZC, Bodine TJ, Chou A, Zechiedrich L. Wicked: the untold story of ciprofloxacin. PLoS Pathog. (2018) 14:e1006805. doi: 10.1371/journal.ppat.1006805
83. Piekarska K, Wołkowicz T, Zacharczuk K, Rzeczkowska M, Chróst A, Bareja E, et al. Co-existence of plasmid-mediated quinolone resistance determinants and mutations in gyrA and parC among fluoroquinolone-resistant clinical Enterobacteriaceae isolated in a tertiary hospital in Warsaw, Poland. Int J Antimicrob Agents. (2015) 45:238–43. doi: 10.1016/j.ijantimicag.2014.09.019
84. Kotb DN, Mahdy WK, Mahmoud MS, Khairy RMM. Impact of co-existence of PMQR genes and QRDR mutations on fluoroquinolones resistance in Enterobacteriaceae strains isolated from community and hospital acquired UTIs. BMC Infect Dis. (2019) 19:979. doi: 10.1186/s12879-019-4606-y
85. Belland RJ, Morrison SG, Ison C, Huang WM. Neisseria gonorrhoeae acquires mutations in analogous regions of gyrA and parC in fluoroquinolone-resistant isolates. Mol Microbiol. (1994) 14:371–80. doi: 10.1111/j.1365-2958.1994.tb01297.x
86. Lindbäck E, Rahman M, Jalal S, Wretlind B. Mutations in gyrA, gyrB, parC, and parE in quinolone-resistant strains of Neisseria gonorrhoeae. Apmis. (2002) 110:651–7. doi: 10.1034/j.1600-0463.2002.1100909.x
87. Zhao L, Zhao S. Molecular basis of high-level ciprofloxacin resistance in Neisseria gonorrhoeae strains from Shandong Province, China. Braz J Microbiol. (2013) 44:273–6. doi: 10.1590/S1517-83822013005000020
88. Kulkarni S, Bala M, Sane S, Pandey S, Bhattacharya J, Risbud A. Mutations in the gyrA and parC genes of quinolone-resistant Neisseria gonorrhoeae isolates in India. Int J Antimicrob Agents. (2012) 40:549–53. doi: 10.1016/j.ijantimicag.2012.08.007
89. Młynarczyk-Bonikowska B, Majewska A, Malejczyk M, Młynarczyk G, Majewski S. Multiresistant Neisseria gonorrhoeae: a new threat in second decade of the XXI century. Med Microbiol Immunol. (2020) 209:95–108. doi: 10.1007/s00430-019-00651-4
90. Shafer WM, Yu EW, Rouquette-Loughlin C, Golparian D, Jerse AE, Unemo M. Efflux pumps in Neisseria gonorrhoeae: contributions to antimicrobial resistance and virulence. In: Li X-Z, Elkins CA, Zgurskaya HI, editors. Efflux-mediated Antimicrobial Resistance in Bacteria. New York. NY: Springer (2016), p. 439–69. doi: 10.1007/978-3-319-39658-3_17
91. Gorla MC, Cassiolato AP, Pinhata JMW, de Moraes C, Corso A, Gagetti P, et al. Emergence of resistance to ciprofloxacin in Neisseria meningitidis in Brazil. J Med Microbiol. (2018) 67:286–8. doi: 10.1099/jmm.0.000685
92. Hong E, Thulin Hedberg S, Abad R, Fazio C, Enriquez R, Deghmane AE, et al. Target gene sequencing to define the susceptibility of Neisseria meningitidis to ciprofloxacin. Antimicrob Agents Chemother. (2013) 57:1961–4. doi: 10.1128/AAC.02184-12
93. Chen M, Guo Q, Wang Y, Zou Y, Wang G, Zhang X, et al. Shifts in the antibiotic susceptibility, serogroups, and clonal complexes of Neisseria meningitidis in Shanghai, China: a time trend analysis of the pre-quinolone and quinolone eras. PLoS Med. (2015) 12:e1001838. discussion e1001838. doi: 10.1371/journal.pmed.1001838
94. Zhu B, Fan Y, Xu Z, Xu L, Du P, Gao Y, et al. Genetic diversity and clonal characteristics of ciprofloxacin-resistant meningococcal strains in China. J Med Microbiol. (2014) 63:1411–8. doi: 10.1099/jmm.0.078600-0
95. Harcourt BH, Anderson RD, Wu HM, Cohn AC, MacNeil JR, Taylor TH, et al. Population-based surveillance of Neisseria meningitidis antimicrobial resistance in the United States. Open Forum Infect Dis. (2015) 2:ofv117. doi: 10.1093/ofid/ofv117
96. Tsang RS, Law DK, Deng S, Hoang L. Ciprofloxacin-resistant Neisseria meningitidis in Canada: likely imported strains. Can J Microbiol. (2017) 63:265–8. doi: 10.1139/cjm-2016-0716
97. Chen M, Zhang C, Zhang X, Chen M. Meningococcal quinolone resistance originated from several commensal Neisseria species. Antimicrob Agents Chemother. (2020) 64:e01494–01419. doi: 10.1128/AAC.01494-19
98. Castanheira M, Deshpande LM, Jones RN, Farrell DJ. Evaluation of quinolone resistance–determining region mutations and efflux pump expression in Neisseria meningitidis resistant to fluoroquinolones. Diagn Microbiol Infect Dis. (2012) 72:263–6. doi: 10.1016/j.diagmicrobio.2011.12.001
99. Enríquez R, Abad R, Salcedo C, Pérez S, Vazquez JA. Fluoroquinolone resistance in Neisseria meningitidis in Spain. J Antimicrob Chemother. (2008) 61:286–90. doi: 10.1093/jac/dkm452
100. Bruchmann S, Dotsch A, Nouri B, Chaberny IF, Haussler S. Quantitative contributions of target alteration and decreased drug accumulation to Pseudomonas aeruginosa fluoroquinolone resistance. Antimicrob Agents Chemother. (2013) 57:1361–8. doi: 10.1128/AAC.01581-12
101. Aldred KJ, Kerns RJ, Osheroff N. Mechanism of quinolone action and resistance. Biochemistry. (2014) 53:1565–74. doi: 10.1021/bi5000564
102. Wang Y-T, Lee M-F, Peng C-F. Mutations in the quinolone resistance-determining regions associated with ciprofloxacin resistance in Pseudomonas aeruginosa isolates from Southern Taiwan. Biomark Genom Med. (2014) 6:79–83. doi: 10.1016/j.bgm.2014.03.003
103. Nouri R, Ahangarzadeh Rezaee M, Hasani A, Aghazadeh M, Asgharzadeh M. The role of gyrA and parC mutations in fluoroquinolones-resistant Pseudomonas aeruginosa isolates from Iran. Braz J Microbiol. (2016) 47:925–30. doi: 10.1016/j.bjm.2016.07.016
104. Cho HH, Kwon KC, Kim S, Koo SH. Correlation between virulence genotype and fluoroquinolone resistance in carbapenem-resistant Pseudomonas aeruginosa. Ann Lab Med. (2014) 34:286–92. doi: 10.3343/alm.2014.34.4.286
105. Goli HR, Nahaei MR, Rezaee MA, Hasani A, Samadi Kafil H, Aghazadeh M, et al. Contribution of mexAB-oprM and mexXY (-oprA) efflux operons in antibiotic resistance of clinical Pseudomonas aeruginosa isolates in Tabriz, Iran. Infect Genet Evol. (2016) 45:75–82. doi: 10.1016/j.meegid.2016.08.022
106. Llanes C, Köhler T, Patry I, Dehecq B, Van Delden C, Plésiat P. Role of the MexEF-OprN efflux system in low-level resistance of Pseudomonas aeruginosa to ciprofloxacin. Antimicrob Agents Chemother. (2011) 55:5676–84. doi: 10.1128/AAC.00101-11
107. Morita Y, Tomida J, Kawamura Y. Efflux-mediated fluoroquinolone resistance in the multidrug-resistant Pseudomonas aeruginosa clinical isolate PA7: identification of a novel MexS variant involved in upregulation of the mexEF-oprN multidrug efflux operon. Front Microbiol. (2015) 6:8. doi: 10.3389/fmicb.2015.00008
108. Tai AS, Bell SC, Kidd TJ, Trembizki E, Buckley C, Ramsay KA, et al. Genotypic diversity within a single Pseudomonas aeruginosa strain commonly shared by Australian patients with cystic fibrosis. PLoS ONE. (2015) 10:e0144022. doi: 10.1371/journal.pone.0144022
109. Sun J, Deng Z, Yan A. Bacterial multidrug efflux pumps: mechanisms, physiology and pharmacological exploitations. Biochem Biophys Res Commun. (2014) 453:254–67. doi: 10.1016/j.bbrc.2014.05.090
110. Jørgensen KM, Wassermann T, Jensen PØ, Hengzuang W, Molin S, Høiby N, et al. Sublethal ciprofloxacin treatment leads to rapid development of high-level ciprofloxacin resistance during long-term experimental evolution of Pseudomonas aeruginosa. Antimicrob Agents Chemother. (2013) 57:4215–21. doi: 10.1128/AAC.00493-13
111. Wong A, Kassen R. Parallel evolution and local differentiation in quinolone resistance in Pseudomonas aeruginosa. Microbiology. (2011) 157:937–44. doi: 10.1099/mic.0.046870-0
112. Feng Y, Jonker MJ, Moustakas I, Brul S, Ter Kuile BH. Dynamics of mutations during development of resistance by Pseudomonas aeruginosa against five antibiotics. Antimicrob Agents Chemother. (2016) 60:4229–36. doi: 10.1128/AAC.00434-16
113. Sproston EL, Wimalarathna HM, Sheppard SK. Trends in fluoroquinolone resistance in Campylobacter. Microb Genom. (2018) 4:e000198. doi: 10.1099/mgen.0.000198
114. Yang W, Zhang M, Zhou J, Pang L, Wang G, Hou F. The molecular mechanisms of ciprofloxacin resistance in clinical Campylobacter jejuni and their genotyping characteristics in Beijing, China. Foodborne Pathog Dis. (2017) 14:386–92. doi: 10.1089/fpd.2016.2223
115. Tang Y, Sahin O, Pavlovic N, LeJeune J, Carlson J, Wu Z, et al. Rising fluoroquinolone resistance in Campylobacter isolated from feedlot cattle in the United States. Sci Rep. (2017) 7:1–8. doi: 10.1038/s41598-017-00584-z
116. Luangtongkum T, Jeon B, Han J, Plummer P, Logue CM, Zhang Q. Antibiotic resistance in Campylobacter: emergence, transmission and persistence. Future Microbiol. (2009) 4:189–200. doi: 10.2217/17460913.4.2.189
117. Wieczorek K, Osek J. Antimicrobial resistance mechanisms among Campylobacter. BioMed Res Int. (2013) 2013:340605. doi: 10.1155/2013/340605
118. Yao H, Shen Z, Wang Y, Deng F, Liu D, Naren G, et al. Emergence of a potent multidrug efflux pump variant that enhances Campylobacter resistance to multiple antibiotics. MBio. (2016) 7:e01543-16. doi: 10.1128/mBio.01543-16
119. Shoji H, Shirakura T, Fukuchi K, Takuma T, Hanaki H, Tanaka K, et al. molecular analysis of quinolone-resistant Haemophilus influenzae: validation of the mutations in quinolone resistance-determining regions. J Infect Chemother. (2014) 20:250–5. doi: 10.1016/j.jiac.2013.12.007
120. Puig C, Tirado-Vélez JM, Calatayud L, Tubau F, Garmendia J, Ardanuy C, et al. Molecular characterization of fluoroquinolone resistance in nontypeable Haemophilus influenzae clinical isolates. Antimicrob Agents Chemother. (2015) 59:461–6. doi: 10.1128/AAC.04005-14
121. Khoshnood S, Heidary M, Hashemi A, Shahi F, Saki M, Kouhsari E, et al. Involvement of the AcrAB efflux pump in ciprofloxacin resistance in clinical Klebsiella pneumoniae isolates. Infect Disord Drug Targets. (2021) 21:564–71. doi: 10.2174/1871526520999200905121220
122. Heidary M, Goudarzi H, Hashemi A, Eslami G, Goudarzi M, Chirani AS, Amraei S. Prevalence of quinolone resistance genes in Klebsiella pneumoniae strains isolated from hospitalized patients during 2013-2014. Arch Pediatr Infect Dis. (2017) 5:e38343. doi: 10.5812/pedinfect.38343
123. Bansal S, Tandon V. Contribution of mutations in DNA gyrase and topoisomerase IV genes to ciprofloxacin resistance in Escherichia coli clinical isolates. Int J Antimicrob Agents. (2011) 37:253–5. doi: 10.1016/j.ijantimicag.2010.11.022
124. Azargun R, Sadeghi V, Leylabadlo HE, Alizadeh N, Ghotaslou R. Molecular mechanisms of fluoroquinolone resistance in Enterobacteriaceae clinical isolates in Azerbaijan, Iran. Gene Rep. (2020) 21:100924. doi: 10.1016/j.genrep.2020.100924
125. Chang MX, Zhang JF, Sun YH Li RS, Lin XL, Yang L, Webber MA, et al. Contribution of different mechanisms to ciprofloxacin resistance in Salmonella spp. Front Microbiol. (2021) 12:663731. doi: 10.3389/fmicb.2021.663731
126. Malekzadegan Y, Rastegar E, Moradi M, Heidari H, Ebrahim-Saraie HS. Prevalence of quinolone-resistant uropathogenic Escherichia coli in a tertiary care hospital in south Iran. Infect Drug Resist. (2019) 12:1683. doi: 10.2147/IDR.S206966
127. Almahmoud I, Kay E, Schneider D, Maurin M. Mutational paths towards increased fluoroquinolone resistance in Legionella pneumophila. J Antimicrob Chemother. (2009) 64:284–93. doi: 10.1093/jac/dkp173
128. Bruin JP, Koshkolda T, IJzerman EP, Luck C, Diederen BM, Den Boer JW, et al. Isolation of ciprofloxacin-resistant Legionella pneumophila in a patient with severe pneumonia. J Antimicrob Chemother. (2014) 69:2869–71. doi: 10.1093/jac/dku196
129. Shadoud L, Almahmoud I, Jarraud S, Etienne J, Larrat S, Schwebel C, et al. Hidden selection of bacterial resistance to fluoroquinolones in vivo: the case of Legionella pneumophila and humans. EBioMedicine. (2015) 2:1179–85. doi: 10.1016/j.ebiom.2015.07.018
130. Yamada K, Saito R. Molecular analysis of low-level fluoroquinolone resistance in clinical isolates of Moraxella catarrhalis. J Med Microbiol. (2014) 63:1066–70. doi: 10.1099/jmm.0.073734-0
131. Yamada K, Saito R, Muto S, Kashiwa M, Tamamori Y, Fujisaki S. Molecular characterization of fluoroquinolone-resistant Moraxella catarrhalis variants generated in vitro by stepwise selection. Antimicrob Agents Chemother. (2017) 61:e01336-17. doi: 10.1128/AAC.01336-17
132. Gergova R, Markovska R. Antimicrobial resistance of Bulgarian isolates Moraxella catarrhalis during the period 1999-2018. J IMAB Annu Proc Sci Papers. (2020) 26:3208–12. doi: 10.5272/jimab.2020262.3208
133. Lari AR, Ardebili A, Hashemi A. AdeR-AdeS mutations & overexpression of the AdeABC efflux system in ciprofloxacin-resistant Acinetobacter baumannii clinical isolates. Indian J Med Res. (2018) 147:413–21. doi: 10.4103/ijmr.IJMR_644_16
134. Gurney J, Pradier L, Griffin JS, Gougat-Barbera C, Chan BK, Turner PE, et al. Phage steering of antibiotic-resistance evolution in the bacterial pathogen, Pseudomonas aeruginosa. Evol Med Public Health. (2020) 2020:148–57. doi: 10.1093/emph/eoaa026
135. Lopes B, Amyes S. Insertion sequence disruption of adeR and ciprofloxacin resistance caused by efflux pumps and gyrA and parC mutations in Acinetobacter baumannii. Int J Antimicrob Agents. (2013) 41:117–21. doi: 10.1016/j.ijantimicag.2012.08.012
136. Ardebili A, Lari AR, Beheshti M, Lari ER. Association between mutations in gyrA and parC genes of Acinetobacter baumannii clinical isolates and ciprofloxacin resistance. Iran J Basic Med Sci. (2015) 18:623–6.
137. Maleki M-H, Jalilian FA, Khayat H, Mohammadi M, Pourahmad F, Asadollahi K, et al. Detection of highly ciprofloxacin resistance Acinetobacter baumannii isolated from patients with burn wound infections in presence and absence of efflux pump inhibitor. Maedica. (2014) 9:162–7.
138. Khayat H, Sadeghifard N, Pakzad I, Azimi L, Delfani S, Sayehmiri K, et al. Determination of different fluoroquinolone mechanisms among clinical isolates of Acinetobacter baumannii in Tehran, Iran. Iran Red Crescent Med J. (2017) 19. doi: 10.5812/ircmj.58798
139. López M, Tenorio C, Del Campo R, Zarazaga M, Torres C. Characterization of the mechanisms of fluoroquinolone resistance in vancomycin-resistant enterococci of different origins. J Chemother. (2011) 23:87–91. doi: 10.1179/joc.2011.23.2.87
140. Mlynarczyk B, Mlynarczyk A, Kmera-Muszynska M, Majewski S, Mlynarczyk G. Mechanisms of resistance to antimicrobial drugs in pathogenic Gram-positive cocci. Mini Rev Med Chem. (2010) 10:928–37. doi: 10.2174/138955710792007204
141. Miller WR, Munita JM, Arias CA. Mechanisms of antibiotic resistance in enterococci. Expert Rev Anti Infect Ther. (2014) 12:1221–36. doi: 10.1586/14787210.2014.956092
142. Arsène S, Leclercq R. Role of a qnr-like gene in the intrinsic resistance of Enterococcus faecalis to fluoroquinolones. Antimicrob Agents Chemother. (2007) 51:3254–8. doi: 10.1128/AAC.00274-07
143. Goudarzi M, Eslami G, Rezaee R, Heidary M, Khoshnood S, Sajadi Nia R. Clonal dissemination of Staphylococcus aureus isolates causing nosocomial infections, Tehran, Iran. Iran J Basic Med Sci. (2019) 22:238–45. doi: 10.22038/ijbms.2018.30067.7245
144. Khoshnood S, Shahi F, Jomehzadeh N, Montazeri EA, Saki M, Mortazavi SM, et al. Distribution of genes encoding resistance to macrolides, lincosamides, and streptogramins among methicillin-resistant Staphylococcus aureus strains isolated from burn patients. Acta Microbiol Immunol Hung. (2019) 66:387–98. doi: 10.1556/030.66.2019.015
145. Andersson DI, Hughes D. Microbiological effects of sublethal levels of antibiotics. Nat Rev Microbiol. (2014) 12:465–78. doi: 10.1038/nrmicro3270
146. Foster TJ. Antibiotic resistance in Staphylococcus aureus. Current status and future prospects. FEMS Microbiol Rev. (2017) 41:430–49. doi: 10.1093/femsre/fux007
147. Papkou A, Hedge J, Kapel N, Young B, MacLean RC. Efflux pump activity potentiates the evolution of antibiotic resistance across S. aureus isolates. Nat Commun. (2020) 11:1–15. doi: 10.1038/s41467-020-17735-y
148. Hassanzadeh S, Mashhadi R, Yousefi M, Askari E, Saniei M, Pourmand MR. Frequency of efflux pump genes mediating ciprofloxacin and antiseptic resistance in methicillin-resistant Staphylococcus aureus isolates. Microb Pathog. (2017) 111:71–4. doi: 10.1016/j.micpath.2017.08.026
149. Khoshnood S, Goudarzi M, Taki E, Darbandi A, Kouhsari E, Heidary M, et al. Bedaquiline: current status and future perspectives. J Glob Antimicrob Resist. (2021) 25:48–59. doi: 10.1016/j.jgar.2021.02.017
150. Heidary M, Shirani M, Moradi M, Goudarzi M, Pouriran R, Rezaeian T, et al. Tuberculosis challenges: resistance, co-infection, diagnosis, and treatment. Eur J Microbiol Immunol. (2022) 12:1–17. doi: 10.1556/1886.2021.00021
151. Cambau E, Viveiros M, Machado D, Raskine L, Ritter C, Tortoli E, et al. Revisiting susceptibility testing in MDR-TB by a standardized quantitative phenotypic assessment in a European multicentre study. J Antimicrob Chemother. (2015) 70:686–96. doi: 10.1093/jac/dku438
152. Avalos E, Catanzaro D, Catanzaro A, Ganiats T, Brodine S, Alcaraz J, et al. Frequency and geographic distribution of gyrA and gyrB mutations associated with fluoroquinolone resistance in clinical Mycobacterium tuberculosis isolates: a systematic review. PLoS ONE. (2015) 10:e0120470. doi: 10.1371/journal.pone.0120470
153. Kabir S, Tahir Z, Mukhtar N, Sohail M, Saqalein M, Rehman A. Fluoroquinolone resistance and mutational profile of gyrA in pulmonary MDR tuberculosis patients. BMC Pulm Med. (2020) 20:138. doi: 10.1186/s12890-020-1172-4
154. Rodrigues L, Villellas C, Bailo R, Viveiros M, Aínsa JA. Role of the Mmr efflux pump in drug resistance in Mycobacterium tuberculosis. Antimicrob Agents Chemother. (2013) 57:751–7. doi: 10.1128/AAC.01482-12
155. Maruri F, Sterling TR, Kaiga AW, Blackman A, van der Heijden YF, Mayer C, et al. systematic review of gyrase mutations associated with fluoroquinolone-resistant Mycobacterium tuberculosis and a proposed gyrase numbering system. J Antimicrob Chemother. (2012) 67:819–31. doi: 10.1093/jac/dkr566
156. Lau RW, Ho P-L, Kao RY, Yew W-W, Lau TC, Cheng VC, et al. Molecular characterization of fluoroquinolone resistance in Mycobacterium tuberculosis: functional analysis of gyrA mutation at position 74. Antimicrob Agents Chemother. (2011) 55:608–14. doi: 10.1128/AAC.00920-10
157. Zhang Z, Lu J, Wang Y, Pang Y, Zhao Y. Prevalence and molecular characterization of fluoroquinolone-resistant Mycobacterium tuberculosis isolates in China. Antimicrob Agents Chemother. (2014) 58:364–9. doi: 10.1128/AAC.01228-13
158. Long Q, Li W, Du Q, Fu Y, Liang Q, Huang H, et al. gyrA/B fluoroquinolone resistance allele profiles amongst Mycobacterium tuberculosis isolates from mainland China. Int J Antimicrob Agents. (2012) 39:486–9. doi: 10.1016/j.ijantimicag.2012.02.015
159. Takiff H, Guerrero E. Current prospects for the fluoroquinolones as first-line tuberculosis therapy. Antimicrob Agents Chemother. (2011) 55:5421–9. doi: 10.1128/AAC.00695-11
160. Lu J, Liu M, Wang Y, Pang Y, Zhao Z. Mechanisms of fluoroquinolone monoresistance in Mycobacterium tuberculosis. FEMS Microbiol Lett. (2014) 353:40–8. doi: 10.1111/1574-6968.12401
161. Wang L, Di Luca M, Tkhilaishvili T, Trampuz A, Gonzalez Moreno M. Synergistic activity of fosfomycin, ciprofloxacin, and gentamicin against Escherichia coli and Pseudomonas aeruginosa biofilms. Front Microbiol. (2019) 10:2522. doi: 10.3389/fmicb.2019.02522
162. Ren H, Zhang J, Zhou J, Xu C, Fan Z, Pan X, et al. Synergistic bactericidal activities of tobramycin with ciprofloxacin and azithromycin against Klebsiella pneumoniae. J Antibiot. (2021) 74:528–37. doi: 10.1038/s41429-021-00427-0
163. Abbas MK, Kadhum DA, Shabeeb AK, Mohammed SA. Combination effect of ciprofloxacin and streptomycin with cefotaxime against multi-drug resistant Pseudomonas aeruginosa from different clinical samples. Res J Pharm Technol. (2020) 13:4403–8. doi: 10.5958/0974-360X.2020.00779.9
164. Pankuch GA, Lin G, Seifert H, Appelbaum PC. Activity of meropenem with and without ciprofloxacin and colistin against Pseudomonas aeruginosa and Acinetobacter baumannii. Antimicrob Agents Chemother. (2008) 52:333–6. doi: 10.1128/AAC.00689-07
165. Rees VE, Yadav R, Rogers KE, Bulitta JB, Wirth V, Oliver A, et al. Meropenem combined with ciprofloxacin combats hypermutable Pseudomonas aeruginosa from respiratory infections of cystic fibrosis patients. Antimicrob Agents Chemother. (2018) 62:e01150–01118. doi: 10.1128/AAC.01150-18
166. Srivastava P, Sivashanmugam K. Efficacy of sub-MIC level of meropenem and ciprofloxacin against extensive drug-resistant (XDR) Pseudomonas aeruginosa isolates of diabetic foot ulcer patients. Infect Genet Evol. (2021) 92:104824. doi: 10.1016/j.meegid.2021.104824
167. Lu Y, Zhang Y, Zhou H, Yu F, Sun S, Rui Y. Combined drug sensitivity test of 50 strains of extensively drug-resistant Acinetobacter baumannii. J South Med Univ. (2014) 34:1697–701.
168. Sun Y, Wang L, Li J, Zhao C, Zhao J, Liu M, et al. Synergistic efficacy of meropenem and rifampicin in a murine model of sepsis caused by multidrug-resistant Acinetobacter baumannii. Eur J Pharmacol. (2014) 729:116–22. doi: 10.1016/j.ejphar.2014.02.015
169. Ramadan RA, Bedawy AM, Negm EM, Hassan TH, Ibrahim DA, ElSheikh SM, et al. Carbapenem-resistant Klebsiella pneumoniae among patients with ventilator-associated pneumonia: evaluation of antibiotic combinations and susceptibility to new antibiotics. Infect Drug Resist. (2022) 15:3537. doi: 10.2147/IDR.S371248
170. Karki R, Lamichhane S, Basnet BB, Dahal A, Awal BK, Mishra SK. In vitro antimicrobial synergy testing of extensively drug-resistant clinical isolates at an organ transplant center in Nepal. Infect Drug Resist. (2021) 14:1669. doi: 10.2147/IDR.S309531
171. Ontong JC, Ozioma NF, Voravuthikunchai SP, Chusri S. Synergistic antibacterial effects of colistin in combination with aminoglycoside, carbapenems, cephalosporins, fluoroquinolones, tetracyclines, fosfomycin, and piperacillin on multidrug resistant Klebsiella pneumoniae isolates. PLoS ONE. (2021) 16:e0244673. doi: 10.1371/journal.pone.0244673
172. Stergiopoulou T, Meletiadis J, Sein T, Papaioannidou P, Tsiouris I, Roilides E, et al. Comparative pharmacodynamic interaction analysis between ciprofloxacin, moxifloxacin and levofloxacin and antifungal agents against Candida albicans and Aspergillus fumigatus. J Antimicrob Chemother. (2009) 63:343–8. doi: 10.1093/jac/dkn473
173. Kim SE, Kim HK, Choi SM, Yu Y, Kim UJ, Darboe KS, et al. In vitro synergy and in vivo activity of tigecycline-ciprofloxacin combination therapy against Vibrio vulnificus sepsis. Antimicrob Agents Chemother. (2019) 63:e00310–00319. doi: 10.1128/AAC.00310-19
174. Shahmoradi S, Shariati A, Zargar N, Yadegari Z, Asnaashari M, Amini SM, et al. Antimicrobial effects of selenium nanoparticles in combination with photodynamic therapy against Enterococcus faecalis biofilm. Photodiagnosis Photodyn Ther. (2021) 35:102398. doi: 10.1016/j.pdpdt.2021.102398
175. Khare T, Mahalunkar S, Shriram V, Gosavi S, Kumar V. Embelin-loaded chitosan gold nanoparticles interact synergistically with ciprofloxacin by inhibiting efflux pumps in multidrug-resistant Pseudomonas aeruginosa and Escherichia coli. Environ Res. (2021) 199:111321. doi: 10.1016/j.envres.2021.111321
176. Mala R, Arunachalam P, Sivasankari M. Synergistic bactericidal activity of silver nanoparticles and ciprofloxacin against phytopathogens. J Cell Tissue Res. (2012) 12:3249.
177. Nikparast Y, Saliani M. Synergistic effect between phyto-syntesized silver nanoparticles and ciprofloxacin antibiotic on some pathogenic bacterial strains. J Med Bacteriol. (2018) 7:36–43.
178. Nejabatdoust A, Salehzadeh A, Zamani H, Moradi-Shoeili Z. Synthesis, characterization and functionalization of ZnO nanoparticles by glutamic acid (Glu) and conjugation of ZnO@Glu by thiosemicarbazide and its synergistic activity with ciprofloxacin against multi-drug resistant Staphylococcus aureus. J Clust Sci. (2019) 30:329–36. doi: 10.1007/s10876-018-01487-3
179. Yayehrad AT, Wondie GB, Marew T. Different nanotechnology approaches for ciprofloxacin delivery against multidrug-resistant microbes. Infect Drug Resist. (2022) 15:413–26. doi: 10.2147/IDR.S348643
180. Ibraheem DR, Hussein NN, Sulaiman GM, Mohammed HA, Khan RA, Al Rugaie O. Ciprofloxacin-loaded silver nanoparticles as potent nano-antibiotics against resistant pathogenic bacteria. Nanomaterials. (2022) 12:2808. doi: 10.3390/nano12162808
181. Anwar A, Imran M, Ramzan M, Khan FA, Ismail N, Hussain AI, et al. Chitosan-based Dy2O3/CuFe3O4 bio-nanocomposite development, characterization, and drug release kinetics. Int J Biol Macromol. (2022) 220:788–801. doi: 10.1016/j.ijbiomac.2022.08.119
182. Liu J, Ding H, Zhao M, Tu F, He T, Zhang L, et al. Functionalized erythrocyte membrane-coated nanoparticles for the treatment of Klebsiella pneumoniae-induced sepsis. Front Microbiol. (2022) 13:901979. doi: 10.3389/fmicb.2022.901979
183. Liu J, Song W, Algharib SA, Luo W, Chen W. Designing, structural determination, and antibacterial activity of injectable ciprofloxacin-loaded gelatin-sodium carboxymethyl cellulose composite nanogels against Staphylococcus aureus. Curr Drug Deliv. (2022). doi: 10.2174/1567201819666220513121219
184. Mehdizadeh M, Sheikhpour M, Salahshourifar I, Siadat SD, Saffarian P. An in vitro study of molecular effects of a combination treatment with antibiotics and nanofluid containing carbon nano-tubes on Klebsiella pneumoniae. Iran J Public Health. (2021) 50:2292–301. doi: 10.18502/ijph.v50i11.7585
185. Massey S, Iqbal F, Rehman AU, Iqbal MS, Iram F. Preparation, characterization and biological evaluation of silver nanoparticles and drug loaded composites for wound dressings formed from Lallemantia royleana seeds' mucilage. J Biomater Sci Polym Ed. (2022) 33:481–98. doi: 10.1080/09205063.2021.1992590
186. Zhu L, Chen L. Facile design and development of nano-clustery graphene-based macromolecular protein hydrogel loaded with ciprofloxacin to antibacterial improvement for the treatment of burn wound injury. Polym Bull. (2022) 79:7953–68. doi: 10.1007/s00289-021-03875-8
187. Mahkam M, Bazmi Zeynabad F, Alizadeh E, Rahimi M, Rahimi F, Salehi R. Novel methotrexate-ciprofloxacin loaded alginate-clay based nanocomposite as anticancer and antibacterial co-drug delivery system. Adv Pharm Bull. (2021) 11:477–89. doi: 10.34172/apb.2021.055
188. Arshad R, Tabish TA, Kiani MH, Ibrahim IM, Shahnaz G, Rahdar A, et al. A hyaluronic acid functionalized self-nano-emulsifying drug delivery system (SNEDDS) for enhancement in ciprofloxacin targeted delivery against intracellular infection. Nanomaterials. (2021) 11:1086. doi: 10.3390/nano11051086
189. Raouf M, Essa S, El Achy S, Essawy M, Rafik S, Baddour M. Evaluation of combined ciprofloxacin and azithromycin free and nano formulations to control biofilm producing Pseudomonas aeruginosa isolated from burn wounds. Indian J Med Microbiol. (2021) 39:81–7. doi: 10.1016/j.ijmmb.2021.01.004
190. Doymus B, Kerem G, Yazgan Karatas A, Kok FN, Onder S. A functional coating to enhance antibacterial and bioactivity properties of titanium implants and its performance in vitro. J Biomater Appl. (2021) 35:655–69. doi: 10.1177/0885328220977765
191. Orsu P, Matta S. Fabrication and characterization of carboxymethyl guar gum nanocomposite for application of wound healing. Int J Biol Macromol. (2020) 164:2267–76. doi: 10.1016/j.ijbiomac.2020.07.322
192. Benedini L, Laiuppa J, Santillan G, Baldini M, Messina P. Antibacterial alginate/nano-hydroxyapatite composites for bone tissue engineering: assessment of their bioactivity, biocompatibility, and antibacterial activity. Mater Sci Eng C Mater Biol Appl. (2020) 115:111101. doi: 10.1016/j.msec.2020.111101
193. Gunday C, Anand S, Gencer HB, Munafo S, Moroni L, Fusco A, et al. Ciprofloxacin-loaded polymeric nanoparticles incorporated electrospun fibers for drug delivery in tissue engineering applications. Drug Deliv Transl Res. (2020) 10:706–20. doi: 10.1007/s13346-020-00736-1
194. Sabzi M, Afshari MJ, Babaahmadi M, Shafagh N. pH-dependent swelling and antibiotic release from citric acid crosslinked poly(vinyl alcohol) (PVA)/nano silver hydrogels. Colloids Surf B Biointerfaces. (2020) 188:110757. doi: 10.1016/j.colsurfb.2019.110757
195. Esfahanian M, Ghasemzadeh MA, Razavian SMH. Synthesis, identification and application of the novel metal-organic framework Fe3O4@PAA@ZIF-8 for the drug delivery of ciprofloxacin and investigation of antibacterial activity. Artif Cells Nanomed Biotechnol. (2019) 47:2024–30. doi: 10.1080/21691401.2019.1617729
196. Zhang Y, Chang M, Bao F, Xing M, Wang E, Xu Q, et al. Multifunctional Zn doped hollow mesoporous silica/polycaprolactone electrospun membranes with enhanced hair follicle regeneration and antibacterial activity for wound healing. Nanoscale. (2019) 11:6315–33. doi: 10.1039/C8NR09818B
197. Farag MM, Al-Rashidy ZM, Ahmed MM. In vitro drug release behavior of Ce-doped nano-bioactive glass carriers under oxidative stress. J Mater Sci Mater Med. (2019) 30:18. doi: 10.1007/s10856-019-6220-3
198. Prusty K, Swain SK. Release of ciprofloxacin drugs by nano gold embedded cellulose grafted polyacrylamide hybrid nanocomposite hydrogels. Int J Biol Macromol. (2019) 126:765–75. doi: 10.1016/j.ijbiomac.2018.12.258
199. Ahmad A, Khan A, Khan LA, Manzoor N. In vitro synergy of eugenol and methyleugenol with fluconazole against clinical Candida isolates. J Med Microbiol. (2010) 59(Pt 10):1178–84. doi: 10.1099/jmm.0.020693-0
200. Haroun MF, Al-Kayali RS. Synergistic effect of Thymbra spicata L. extracts with antibiotics against multidrug- resistant Staphylococcus aureus and Klebsiella pneumoniae strains. Iran J Basic Med Sci. (2016) 19:1193–200.
201. Abdi Ali A, Shafiei M, Shahcheraghi F, Saboora A, Ghazanfari T. The study of synergistic effects of n. butanolic Cyclamen coum extract and ciprofloxacin on inhibition of Pseudomonas aeruginosa biofilm formation. Biol J Microorganism. (2015) 3:25–32.
202. Aqil F, Ahmad I, Owais M. Evaluation of anti-methicillin-resistant Staphylococcus aureus (MRSA) activity and synergy of some bioactive plant extracts. Biotechnol J. (2006) 1:1093–102. doi: 10.1002/biot.200600130
203. Choi JG, Choi JY, Mun SH, Kang OH, Bharaj P, Shin DW, et al. Antimicrobial activity and synergism of Sami-Hyanglyun-Hwan with ciprofloxacin against methicillin-resistant Staphylococcus aureus. Asian Pac J Trop Med. (2015) 8:538–42. doi: 10.1016/j.apjtm.2015.06.010
204. Lan J-E, Li X-J, Zhu X-F, Sun Z-L, He J-M, Zloh M, et al. Flavonoids from Artemisia rupestris and their synergistic antibacterial effects on drug-resistant Staphylococcus aureus. Nat Prod Res. (2021) 35:1881–6. doi: 10.1080/14786419.2019.1639182
205. Liu G, Liang J-C, Wang X-L, Li Z-H, Wang W, Guo N, et al. In vitro synergy of biochanin A and ciprofloxacin against clinical isolates of Staphylococcus aureus. Molecules. (2011) 16:6656–66. doi: 10.3390/molecules16086656
206. Guzmán EL, Cruz FJM. Combinations of extracts of propolis and other compounds against methicillin-resistant Staphylococcus aureus. In: El-Shemy HA, editor. Active Ingredients from Aromatic and Medicinal Plants. London: IntechOpen (2017). doi: 10.5772/66219
207. Siddiqui SH, Awan KH, Javed F. Bactericidal efficacy of photodynamic therapy against Enterococcus faecalis in infected root canals: a systematic literature review. Photodiagnosis Photodyn Ther. (2013) 10:632–43. doi: 10.1016/j.pdpdt.2013.07.006
208. Maisch T, Hackbarth S, Regensburger J, Felgenträger A, Bäumler W, Landthaler M, et al. Photodynamic inactivation of multi-resistant bacteria (PIB)–a new approach to treat superficial infections in the 21st century. J Dtsch Dermatol Ges. (2011) 9:360–6. doi: 10.1111/j.1610-0387.2010.07577.x
209. Rosa LP, da Silva FC, Nader SA, Meira GA, Viana MS. Antimicrobial photodynamic inactivation of Staphylococcus aureus biofilms in bone specimens using methylene blue, toluidine blue ortho and malachite green: an in vitro study. Arch Oral Biol. (2015) 60:675–80. doi: 10.1016/j.archoralbio.2015.02.010
210. Ronqui MR, de Aguiar TMSF, De Freitas LM, Miranda ET, Fontana CR. Synergistic antimicrobial effect of photodynamic therapy and ciprofloxacin. J Photochem Photobiol B Biol. (2016) 158:122–9. doi: 10.1016/j.jphotobiol.2016.02.036
211. Pereira NL, Aquino PE, Júnior JG, Cristo JS, Vieira Filho MA, Moura FF, et al. Antibacterial activity and antibiotic modulating potential of the essential oil obtained from Eugenia jambolana in association with led lights. J Photochem Photobiol B Biol. (2017) 174:144–9. doi: 10.1016/j.jphotobiol.2017.07.027
212. Moghadam MT, Amirmozafari N, Shariati A, Hallajzadeh M, Mirkalantari S, Khoshbayan A, et al. How phages overcome the challenges of drug resistant bacteria in clinical infections. Infect Drug Resist. (2020) 13:45. doi: 10.2147/IDR.S234353
213. Gu J, Liu X, Li Y, Han W, Lei L, Yang Y, et al. A method for generation phage cocktail with great therapeutic potential. PLoS ONE. (2012) 7:e31698. doi: 10.1371/journal.pone.0031698
214. Jaiswal A, Koley H, Ghosh A, Palit A, Sarkar B. Efficacy of cocktail phage therapy in treating Vibrio cholerae infection in rabbit model. Microbes Infect. (2013) 15:152–6. doi: 10.1016/j.micinf.2012.11.002
215. Łusiak-Szelachowska M, Weber-Dabrowska B, Górski A. Bacteriophages and lysins in biofilm control. Virol Sin. (2020) 35:125–33. doi: 10.1007/s12250-019-00192-3
216. Chegini Z, Khoshbayan A, Vesal S, Moradabadi A, Hashemi A, Shariati A. Bacteriophage therapy for inhibition of multi drug-resistant uropathogenic bacteria: a narrative review. Ann Clin Microbiol Antimicrob. (2021) 20:30. doi: 10.1186/s12941-021-00433-y
217. Valério N, Oliveira C, Jesus V, Branco T, Pereira C, Moreirinha C, et al. Effects of single and combined use of bacteriophages and antibiotics to inactivate Escherichia coli. Virus Res. (2017) 240:8–17. doi: 10.1016/j.virusres.2017.07.015
218. Engeman E, Freyberger HR, Corey BW, Ward AM, He Y, Nikolich MP, et al. Synergistic killing and re-sensitization of pseudomonas aeruginosa to antibiotics by phage-antibiotic combination treatment. Pharmaceuticals. (2021) 14:184. doi: 10.3390/ph14030184
219. Menon ND, Kumar MS, Satheesh Babu TG, Bose S, Vijayakumar G, Baswe M, et al. A novel N4-like bacteriophage isolated from a wastewater source in South India with activity against several multidrug-resistant clinical Pseudomonas aeruginosa isolates. mSphere. (2021) 6:e01215-20. doi: 10.1128/mSphere.01215-20
220. Jeon G, Ahn J. Evaluation of phage adsorption to Salmonella Typhimurium exposed to different levels of pH and antibiotic. Microb Pathog. (2021) 150:104726. doi: 10.1016/j.micpath.2020.104726
221. Lin Y, Quan D, Chang RYK, Chow MYT, Wang Y, Li M, et al. Synergistic activity of phage PEV20-ciprofloxacin combination powder formulation-A proof-of-principle study in a P. aeruginosa lung infection model. Eur J Pharm Biopharm. (2021) 158:166–71. doi: 10.1016/j.ejpb.2020.11.019
222. Nikolic I, Vukovic D, Gavric D, Cvetanovic J, Aleksic Sabo V, Gostimirovic S, et al. An optimized checkerboard method for phage-antibiotic synergy detection. Viruses. (2022) 14:1542. doi: 10.3390/v14071542
223. Hong HW, Kim YD, Jang J, Kim MS, Song M, Myung H. Combination effect of engineered endolysin EC340 with antibiotics. Front Microbiol. (2022) 13:821936. doi: 10.3389/fmicb.2022.821936
224. Chaudhry WN, Concepcion-Acevedo J, Park T, Andleeb S, Bull JJ, Levin BR. Synergy and order effects of antibiotics and phages in Killing Pseudomonas aeruginosa biofilms. PLoS ONE. (2017) 12:e0168615. doi: 10.1371/journal.pone.0168615
225. Chan BK, Sistrom M, Wertz JE, Kortright KE, Narayan D, Turner PE. Phage selection restores antibiotic sensitivity in MDR Pseudomonas aeruginosa. Sci Rep. (2016) 6:26717. doi: 10.1038/srep26717
226. Tkhilaishvili T, Wang L, Perka C, Trampuz A, Gonzalez Moreno M. Using bacteriophages as a trojan horse to the killing of dual-species biofilm formed by Pseudomonas aeruginosa and methicillin resistant Staphylococcus aureus. Front Microbiol. (2020) 11:695. doi: 10.3389/fmicb.2020.00695
227. Akturk E, Oliveira H, Santos SB, Costa S, Kuyumcu S, Melo LDR, et al. Synergistic action of phage and antibiotics: parameters to enhance the killing efficacy against mono and dual-species biofilms. Antibiotics. (2019) 8:103. doi: 10.3390/antibiotics8030103
228. Gutiérrez D, Briers Y, Rodríguez-Rubio L, Martínez B, Rodríguez A, Lavigne R, et al. Role of the pre-neck appendage protein (Dpo7) from phage vB_SepiS-phiIPLA7 as an anti-biofilm agent in Staphylococcal species. Front Microbiol. (2015) 6:1315. doi: 10.3389/fmicb.2015.01315
229. Lin DM, Koskella B, Lin HC. Phage therapy: an alternative to antibiotics in the age of multi-drug resistance. World J Gastrointest Pharmacol Ther. (2017) 8:162–73. doi: 10.4292/wjgpt.v8.i3.162
230. Vilas Boas D, Almeida C, Sillankorva S, Nicolau A, Azeredo J, Azevedo NF. Discrimination of bacteriophage infected cells using locked nucleic acid fluorescent in situ hybridization (LNA-FISH). Biofouling. (2016) 32:179–90. doi: 10.1080/08927014.2015.1131821
231. Ostapska H, Raju D, Corsini R, Lehoux M, Lacdao I, Gilbert S, et al. Preclinical evaluation of recombinant microbial glycoside hydrolases as antibiofilm agents in acute pulmonary Pseudomonas aeruginosa infection. Antimicrob Agents Chemother. (2022) 66:e0005222. doi: 10.1128/aac.00052-22
232. Aroso RT, Dias LD, Blanco KC, Soares JM, Alves F, da Silva GJ, et al. Synergic dual phototherapy: cationic imidazolyl photosensitizers and ciprofloxacin for eradication of in vitro and in vivo E. coli infections. J Photochem Photobiol B Biol. (2022) 233:112499. doi: 10.1016/j.jphotobiol.2022.112499
233. Chiang CY, Lane DJ, Zou Y, Hoffman T, Pan J, Hampton J, et al. A novel toll-like receptor 2 agonist protects mice in a prophylactic treatment model against challenge with Bacillus anthracis. Front Microbiol. (2022) 13:803041. doi: 10.3389/fmicb.2022.803041
234. Fujita K, Takata I, Yoshida I, Takashima H, Sugiyama H. TP0586532, a non-hydroxamate LpxC inhibitor, reduces LPS release and IL-6 production both in vitro and in vivo. J Antibiot. (2022) 75:136–45. doi: 10.1038/s41429-021-00498-z
235. Li Y, Liu Y, Ren Y, Su L, Li A, An Y, et al. Coating of a novel antimicrobial nanoparticle with a macrophage membrane for the selective entry into infected macrophages and killing of intracellular Staphylococci. Adv Funct Mater. (2020) 30:2004942. doi: 10.1002/adfm.202004942
236. Laulund AS, Schwartz F, Trøstrup H, Thomsen K, Christophersen L, Calum H, et al. Adjunctive S100A8/A9 immunomodulation hinders ciprofloxacin resistance in Pseudomonas aeruginosa in a murine biofilm wound model. Front Cell Infect Microbiol. (2021) 11:652012. doi: 10.3389/fcimb.2021.652012
237. Liao CC Yu HP, Yang SC, Alalaiwe A, Dai YS, Liu FC, Fang JY. Multifunctional lipid-based nanocarriers with antibacterial and anti-inflammatory activities for treating MRSA bacteremia in mice. J Nanobiotechnology. (2021) 19:48. doi: 10.1186/s12951-021-00789-5
238. Liu Y, Yang K, Jia Y, Shi J, Tong Z, Wang Z. Thymine sensitizes gram-negative pathogens to antibiotic killing. Front Microbiol. (2021) 12:622798. doi: 10.3389/fmicb.2021.622798
239. Moazzezy N, Asadi Karam MR, Rafati S, Bouzari S, Oloomi M. A synthetic peptide 2Abz(23)S(29) reduces bacterial titer and induces pro-inflammatory cytokines in a murine model of urinary tract infection. Drug Des Devel Ther. (2020) 14:2797–807. doi: 10.2147/DDDT.S259937
240. Yoshitani J, Kabata T, Arakawa H, Kato Y, Nojima T, Hayashi K, et al. Combinational therapy with antibiotics and antibiotic-loaded adipose-derived stem cells reduce abscess formation in implant-related infection in rats. Sci Rep. (2020) 10:11182. doi: 10.1038/s41598-020-68184-y
241. Sun M, Zhu C, Long J, Lu C, Pan X, Wu C, et al. microsphere-based composite hydrogel for dual delivery of ciprofloxacin and ginsenoside Rh2 to treat Staphylococcus aureus-induced skin infections. Drug Deliv. (2020) 27:632–41. doi: 10.1080/10717544.2020.1756985
242. Tambat R, Jangra M, Mahey N, Chandal N, Kaur M, Chaudhary S, et al. Microbe-derived indole metabolite demonstrates potent multidrug efflux pump inhibition in Staphylococcus aureus. Front Microbiol. (2019) 10:2153. doi: 10.3389/fmicb.2019.02153
243. Hazlett LD, Ekanayaka SA, McClellan SA, Francis R. Glycyrrhizin use for multi-drug resistant Pseudomonas aeruginosa: in vitro and in vivo studies. Invest Ophthalmol Vis Sci. (2019) 60:2978–89. doi: 10.1167/iovs.19-27200
244. Parquet MDC, Savage KA, Allan DS, Ang MTC, Chen W, Logan SM, et al. Antibiotic-resistant Acinetobacter baumannii is susceptible to the novel iron-sequestering anti-infective DIBI in vitro and in experimental pneumonia in mice. Antimicrob Agents Chemother. (2019) 63:e00855-19. doi: 10.1128/AAC.00855-19
Keywords: review, ciprofloxacin, resistance, new approach, antibacterial agents
Citation: Shariati A, Arshadi M, Khosrojerdi MA, Abedinzadeh M, Ganjalishahi M, Maleki A, Heidary M and Khoshnood S (2022) The resistance mechanisms of bacteria against ciprofloxacin and new approaches for enhancing the efficacy of this antibiotic. Front. Public Health 10:1025633. doi: 10.3389/fpubh.2022.1025633
Received: 23 August 2022; Accepted: 22 November 2022;
Published: 21 December 2022.
Edited by:
Marwan Osman, Cornell University, United StatesReviewed by:
Sarunyou Chusri, Prince of Songkla University, ThailandCopyright © 2022 Shariati, Arshadi, Khosrojerdi, Abedinzadeh, Ganjalishahi, Maleki, Heidary and Khoshnood. This is an open-access article distributed under the terms of the Creative Commons Attribution License (CC BY). The use, distribution or reproduction in other forums is permitted, provided the original author(s) and the copyright owner(s) are credited and that the original publication in this journal is cited, in accordance with accepted academic practice. No use, distribution or reproduction is permitted which does not comply with these terms.
*Correspondence: Mohsen Heidary, bW9oc2VuaGVpZGFyeTQwQGdtYWlsLmNvbQ==; Saeed Khoshnood, c2FlZWQua2hvc2hub29kMjJAZ21haWwuY29t
†These authors have contributed equally to this work
Disclaimer: All claims expressed in this article are solely those of the authors and do not necessarily represent those of their affiliated organizations, or those of the publisher, the editors and the reviewers. Any product that may be evaluated in this article or claim that may be made by its manufacturer is not guaranteed or endorsed by the publisher.
Research integrity at Frontiers
Learn more about the work of our research integrity team to safeguard the quality of each article we publish.