- 1Department of Toxicology, School of Public Health, Medical College of Soochow University, Suzhou, China
- 2Jiangsu Key Laboratory of Preventive and Translational Medicine for Geriatric Diseases, Suzhou, China
Objective: To examine whether exposure of mouse bone marrow stromal cells (BMSC) to 900 MHz radiofrequency fields used in mobile communication devices can induce mitochondrial unfolded protein response (UPRmt).
Methods: BMSCs were exposed to continuous wave 900 MHz radiofrequency fields (RF) at 120 μW/cm2 power intensity for 4 h/d for 5 consecutive days. Cells in sham group (SH) were cultured in RF exposure system, but without RF radiation. The positive control cells were irradiated with 6 Gy X-ray at a dose rate of 1.103 Gy/min (XR). To inhibit the upstream molecular JNK2 of UPRmt, cells in siRNA + RF, and siRNA + XR group were also pretreated with 100 nM siRNA-JNK2 for 48 h before RF/XR exposure. Thirty minutes, 4 h, and 24 h post-RF/XR exposure, cells were collected, the level of ROS was measured with flow cytometry, the expression levels of UPRmt-related proteins were detected using western blot analysis.
Results: Compared with Sham group, the level of ROS in RF and XR group was significantly increased 30min and 4 h post-RF/XR exposure (P < 0.05), however, the RF/XR-induced increase of ROS level reversed 24 h post-RF/XR exposure. Compared with Sham group, the expression levels of HSP10/HSP60/ClpP proteins in cells of RF and XR group increased significantly 30min and 4 h post-RF/XR exposure (P < 0.05), however, the RF/XR-induced increase of HSP10/HSP60/ClpP protein levels reversed 24 h post-RF exposure. After interfering with siRNA-JNK2, the RF/XR exposures could not induce the increase of HSP10/HSP60/ClpP protein levels any more.
Conclusions: The exposure of 900 MHz RF at 120 μW/cm2 power flux density could increase ROS level and activate a transient UPRmt in BMSC cells. Mitochondrial homeostasis in term of protein folding ability is restored 24 h post-RF exposure. Exposure to RF in our experimental condition did not cause permanent and severe mitochondrial dysfunctions. However, the detailed underlying molecular mechanism of RF-induced UPRmt remains to be further studied.
Introduction
Non-ionizing radiofrequency fields (RF) are ubiquitous in the environment. They are used in military, radio, and television broadcasting, wireless communications systems, industry, and medicine. The number of people exposed to RF increased exponentially with the introduction of wireless communication devices which transmit voice, data and images. The scientific information on the biological and health effects of exposure to RF is more extensive now than ever before (1). Nonetheless, the unfolded protein response (UPR) in mitochondria in cells exposed to RF received little attention of researchers. Mitochondria is the organelle with the closed bilayer membranes structure, which play a key role in cellular biosynthetic, intracellular oxidative phosphorylation and the regulation of calcium levels. Mitochondria contain specific heat shock proteins (HSP) and protease, which help to fold, unfold, or degrade other proteins for the protein equilibrium inside. When a large number of unfolded or misfolded proteins are accumulated in cells due to external stimulus, the reverse signaling pathway from mitochondria to nucleus will be activated to increase the expression of nuclear genes encoding mitochondrial proteins (2). The newly-synthesized mitochondrial proteins include chaperones HSP10 and HSP60 which facilitate the import and correct folding of unfolded proteins, and proteases ClpP and ClpX which help to degrade the unfolded and misfolded proteins. This process is called mitochondrial unfolded protein response (UPRmt) (3–7).
Mitochondria are the main source and one of the targets of RF-induced reactive oxygen species (ROS) (8). Electromagnetic field (EMF) directly targets the electron transport chain, which leads to mitochondrial dysfunction and overproduction of ROS. EMF can cause a disturbance of mitochondrial proton motive force, which then disrupts the balance between ROS production and ROS clearance. The severe and long-lasting oxidative stress may play a key role in mitochondrial damage that leads to some human health problems (9). However, mild ROS increase could lead to cellular defense mechanisms, including unfolded protein response (10). Studies showed that during mild mitochondrial dysfunction, UPRmt activation promotes development and prolonging longevity, suggesting that UPRmt activation may be a useful therapeutic approach for some mitochondria-related diseases. However, prolonged or dysregulation of UPRmt activation can exacerbate mitochondrial dysfunction caused by external stimuli (11–13).
Currently, there are few reports on the effect of RF-induced ROS on UPRmt. In view of the paucity of RF investigations on UPR in mitochondria, we have conducted the present study to investigate whether 900 MHz RF can induce UPRmt in BMSCs. Mouse bone marrow stem cells (BMSCs) were exposed to 900 MHz RF for 4 h/d for 5 d to examine if RF exposure can induce UPR in mitochondria. Sham-exposed (SH) control cells as well as those exposed to an acute dose of ionizing/X-rays radiation (XR) as positive control cells were included in the experiment. The expression levels of HSP10/HSP60/ClpP proteins involved in UPRmt were examined. To verify the induction of UPR in BMSCs, siRNA-JNK2 was used to inhibit the known signal molecular JNK2 of UPRmt in the study.
Materials and Methods
Bone Marrow Stromal Cells
The collection and culture of BMSCs were described in detail in our earlier paper (14). Single cell suspensions were prepared in complete IMDM medium (Iscove's modified Dulbecco's medium, Hyclone, Suzhou, China) containing 10% fetal bovine serum (FBS, Gibco, Shanghai, China), 100 units/ml penicillin and 100 μg/ml streptomycin (Bio Basic, Hangzhou, China). From each mouse, aliquots of 4 × 105 cells in 10 ml medium were placed in 100 mm petri dishes cultured in an incubator (Heal Force Bio-Meditech, Hong Kong, China) maintaining 37 ± 0.5°C with humidified atmosphere of 95% air and 5% carbon dioxide (CO2). Cells in 3–6 passages from a single mouse were used for different exposures described below.
Radiofrequency Fields/Sham Exposed Exposure
The exposure system was built in-house and consists of a signal generator (SN2130J6030, PMM, Cisano sul Neva, Italy), a power amplifier (SN1020, HD Communication, Ronkonkoma, NY), and a Gigahertz Transverse Electro-Magnetic (GTEM) chamber. The RF signal was generated, amplified and fed through an antenna (Southeast University, Nanjing, Jiangsu, China) and detected by a field strength meter (PMM, Cisano sul Neva, Italy). The specific operation principle and exposure protocols has been discussed in detail by the previous studies of our lab (14–16). The same GTEM without RF transmission, was used for SH-exposure of cells. During the RF/SH exposure, the culture medium was changed once and the external environment was maintained 37 ± 0.5°C with 87% relative humidity (without CO2). The peak and average SARs could be computed by either frequency or time domain method (17), and the estimated values were extremely low: they were 4.1 × 10−4 and 2.5 × 10−4 W/kg, respectively.
X-Ray Radiation
Irradiations were performed with an X-ray apparatus (Rad Source Technologies Inc., USA) operating at a dose rate of 1.103 Gy/min.
Group Design
Several 100 mm petri dishes, each containing ~4 × 105 cells/ml (total 10 ml medium), were used for the following exposure conditions: (a) kept in GTEM without RF (Sham, SH); (b) 900 MHz RF, 120 μW/cm2 power intensity for 4 h/d for 5 d (RF); (c) acute 6 Gy X-ray radiation (XR); (d) kept in GTEM without RF for 4 h/d for 5 d after siRNA transfection (si + SH); (e) 900 MHz RF for 4 h/d for 5 d after siRNA transfection (si + RF); (f) acute 6 Gy X-ray radiation after siRNA transfection (si + XR). Cells in each group were collected at 30min, 4 h, and 24 h post treatment for subsequent experiments. The entire investigation was repeated 3 times.
Measurement of Reactive Oxygen Species
ROS level was measured with reactive oxygen detection kit (Beyotime, Shanghai, China). After different treatments, the cells were gently washed twice with neutral phosphate buffer after digestion and centrifugation. Serum-free IMDM medium (Hyclone, USA) was used to prepare DCFH-DA solution with a final concentration of 10 μM. One mL of DCFH-DA solution was added into the centrifuge tubes of each group and thoroughly mixed. Centrifuge tube was incubated at 37°C for 20 min, mixed upside down every 3 min to promote maximum contact between the cells and the probe. At the end of incubation, supernatant was discarded, and the cells were washed with serum-free IMDM medium for 3 times. Then, the intracellular fluorescence intensity was measured at excitation wavelength of 488 nm and the emission wavelength of 525 nm.
siRNA Transfection
Transfection with siRNA was used to inhibit JNK2 expression, in a separate series of experiments, prior to sham, RF and X-rays exposure, cells grown to 60–70% confluence were transfected with 100 nM siRNA (ribo FECT− CP, Guangzhou, China) for 48 h, the target sequence used for JNK siRNA were GGCATCAAGCATCTGCATT. Subsequently, siRNA was washed out three times followed by the different exposure conditions. Transfection was performed for 48 h, and then the cells were collected. Quantitative real-time PCR was used to verify the transfection efficiency.
Western-Blot Analysis
In this study, HSP60/HSP10/ClpP protein, known UPRmt markers, were chosen as the indicators to verify whether UPRmt occurred (18–20). The expression levels of the heat shock protein HSP60/HSP10 and mitochondrial protease ClpP were detected with Western-Blot Analysis. Protein extracts were prepared by lysing the cells in lysis buffer containing 50 mM Tris (pH 7.4), 150 mM sodium chloride, 1%Triton X-100, 1% sodium deoxycholate, 0.1% sodium dodecyl sulfate, and 1 mM phenyl-methyl-sulfonyl fluoride (all obtained from Beyotime, Shanghai, China). The cell lysates were centrifuged at 14,000 × g for 5 min at 4°C and the supernatant containing solubilized proteins was collected. The protein concentration in all samples was determined using the BCA protein assay kit (Beyotime, Shanghai, China). From each sample, equal amount of protein (40 μg per lane) was loaded, separated by 10% sodium dodecyl sulfate polyacrylamide gel (SDS–PAGE) and then transferred to polyvinylidene difluoride (PVDF) membranes (Millipore Corporation, Billerica, MA, USA). The membranes were blocked for 2 h in 5% fat-free dry milk (Yili Industrial, Inner Mongolia, China) containing Tris Buffered Saline with Tween (TBST). The membranes were then incubated with primary antibodies (rabbit monoclonal anti-HSP10 antibody, rabbit monoclonal anti-HSP60 antibody, rabbit monoclonal anti-ClpP antibody, and rabbit monoclonal anti-GADPH, Abcam, Cambridge, USA) overnight at 4°C. They were washed three times in TBST and incubated further with horseradish peroxidase-conjugated antibodies (Beyotime, Shanghai, China) for 1.5 h at room temperature. This was followed by washing the membranes three times with TBST. The immunoreactive proteins on the membranes were detected using enhanced chemiluminescence reagents (Millipore Corporation) and G-BOX Chemi XRQ (Syngene, UK). The blots were quantified and normalized with the level of GADPH to correct the differences in loading of the proteins in different treatment cells.
Statistical Analyses
The results from three independent experiments were pooled and analyzed using GraphPad Prism 8.0 (GraphPad Software, San Diego, CA, USA). The results were subjected to One-way analysis of variance (ANOVA) to test differences between groups. A P < 0.05 was considered as significance difference between groups.
Results
Reactive Oxygen Species
The expression levels of ROS in different groups were shown in Figure 1. The level of ROS in the RF group and XR group was significantly increased at 30min and 4 h post-exposure (P < 0.05). The ROS level decreased to nearly those in SH cells 24 h post-RF and XR exposure. Compared with the cells exposed to RF, those exposed to XR showed significant increase in ROS at 30min, 4 h (P < 0.05). These results indicated that both RF and X-ray could induce the production of ROS in BMSCs for a certain period of time. The increase of ROS induced by X-ray was much higher than that induced by RF.
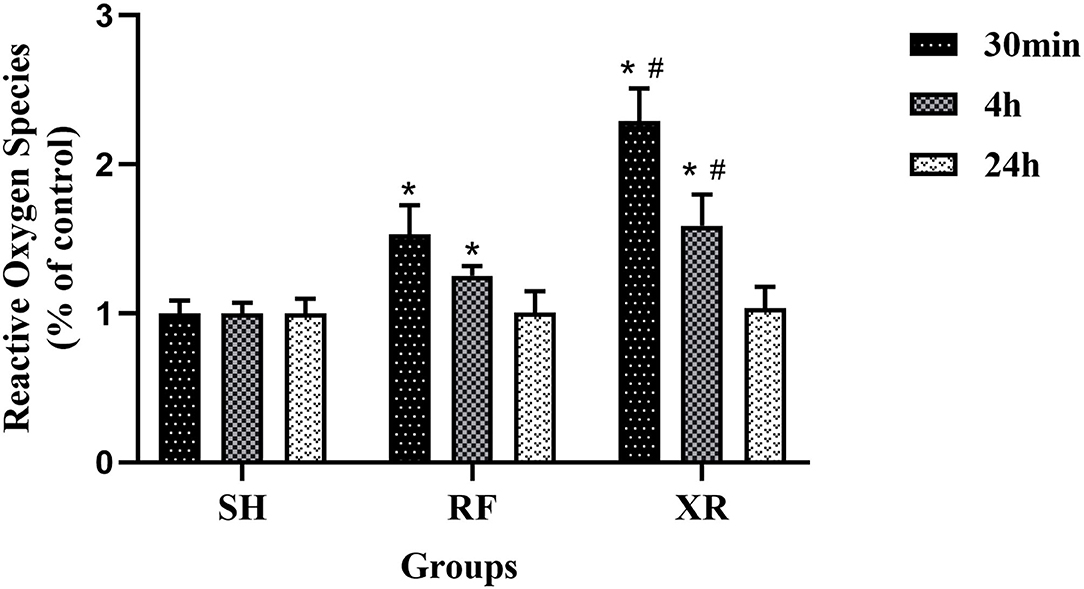
Figure 1. ROS levels in BMSCs at 30min, 4 h, and 24 h post exposure. SH, Sham; RF, RF-exposed; XR, X-rays irradiated. RF and XR vs. SH: *P < 0.05; XR vs. RF: #P < 0.05.
siRNA Transfection Efficiency
After siRNA transfection for 48 h, the expression level of JNK2 mRNA was shown in Figure 2. The expression level of JNK2 mRNA decreased significantly compared to sham group (P < 0.05). The knockdown efficiency of siRNA transfection is 50%.
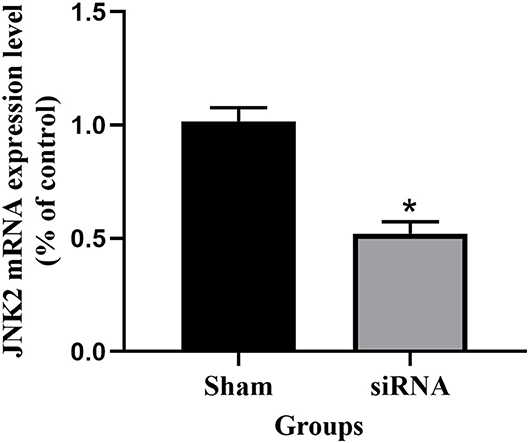
Figure 2. The expression level of JNK2 mRNA after siRNA transfection for 48 h. siRNA vs. Sham: *P < 0.05.
HSP10, HSP60, and ClpP Proteins (Western Blot Analysis)
The expression levels of HSP10, HSP60, ClpP proteins in different groups at 30min, 4 h, and 24 h were shown in Figure 3. Compared to the cells in SH group, the expression levels of HSP10, HSP60, ClpP proteins in the RF group increased significantly at 30min and 4 h (P < 0.05), then decreased gradually and returned to nearly those in SH cells at 24 h. These results indicate that low-dose Radio-frequency could induce UPRmt in BMSCs. After the interference of the upstream molecular JNK2 of UPRmt with siRNA, the expression levels of HSP10, HSP60, ClpP proteins in si + RF group were significantly decreased compared to the RF group (P < 0.05), indicated that RF activates UPRmt through the JNK2 signaling pathway.
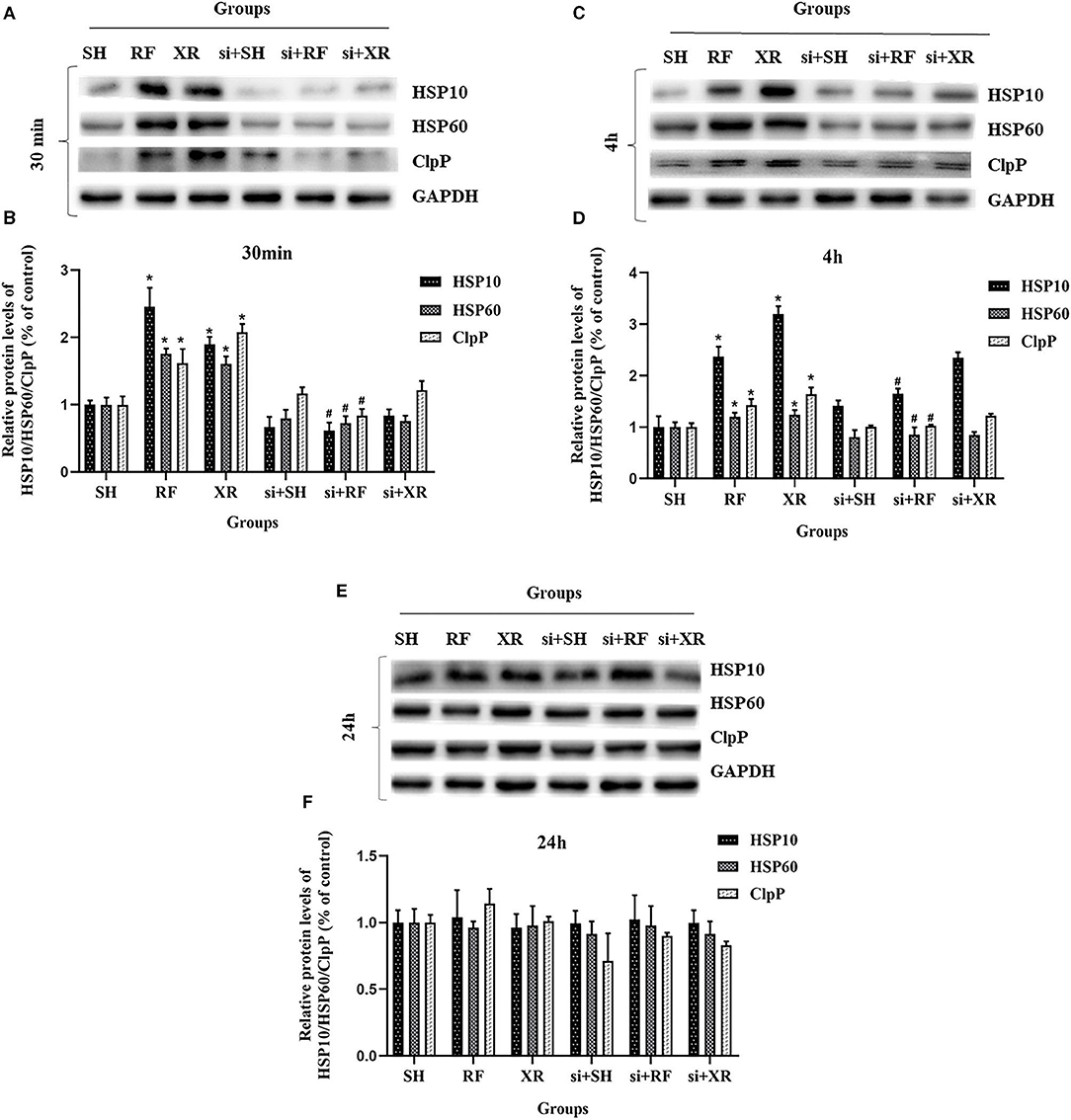
Figure 3. Protein levels of HSP10, HSP60, ClpP in BMSCs after exposure. (A) Western blot analysis of HSP10, HSP60, ClpP in BMSCs at 30min post exposure. (B) Relative expression on level of HSP10, HSP60, ClpP protein at 30min post exposure. (C) Western blot analysis of HSP10, HSP60, ClpP in BMSCs at 4 h post exposure. (D) Relative expression on level of HSP10, HSP60, ClpP protein at 4 h post exposure. (E) Western blot analysis of HSP10, HSP60, ClpP in BMSCs at 24 h post exposure. (F) Relative expression on level of HSP10, HSP60, ClpP protein at 24 h post exposure. SH, Sham; RF, RF-exposed; XR, X-rays irradiated; si + SH, siRNA + Sham; si + RF, siRNA + RF-exposed; si + XR, siRNA + X-rays irradiated. RF and XR vs. SH: *P < 0.05; si + RF vs. RF: #P < 0.05.
Discussion
Mitochondria are organelles that play important functions in cells and participate in a variety of physiological functions and biochemical reactions, including ATP production, iron-sulfur cluster biosynthesis, nucleotide and amino acid metabolism, and cell apoptosis (21–24). In order to maintain normal cellular physiological activities and functions, mitochondria have a complete set of molecular chaperone systems and quality control proteases, which promote the correct folding of proteins and degradation of misfolded/unfolded proteins (25, 26). Upon environmental stress, the accumulation of unfolded protein in the mitochondria could reach a certain threshold or severely damaged mitochondria appear in the cell, hence UPRmt can enhance the overall function of cellular mitochondria by upregulating protective molecular chaperones and proteases that promote protein folding or clearance of defective proteins within stressed mitochondria, restore and maintain mitochondria steady state, and ultimately prevent and/or reduce the damage of environmental factors to the cells.
Non-ionizing radiofrequency fields (RF, 300 MHz to 300 GHz) are ubiquitous in environment (27). 900 MHz RF is one of the frequencies commonly used in mobile communication. High doses or long duration of radiofrequency radiation will exert harmful health effects on living organisms. The power density of 120 μW/cm2 used in this study is below the exposure limit stipulated by the International Commission on Non-Ionizing Radiation Protection (ICNIRP), which is generally considered as low dose radiation. The biological effects and health impacts of long-term exposure to low-dose RF are a matter of widespread concern, and also a scientific issue that needs to be studied (28). In this study, we found that exposure to RF in our experimental condition did not cause permanent and severe mitochondrial dysfunctions. Our findings may shed light on dark areas of health effects of human exposure to radiofrequency radiation.
To the best of our knowledge, there have been very few peer-reviewed scientific publications in which ROS and mitochondrial UPR were examined in cells exposed to RF. Studies have shown that RF radiation can induce oxidative damage of mtDNA in cerebral cortical neurons of SD rats (29). It is reported that mitochondrial UPR could inhibit oxidative phosphorylation and ROS production (30). Increased protein level of chaperones HSP10, HSP60, and protease ClpP are considered to be the hallmark of UPRmt activation (2, 3). As a mitochondrial chaperone protein encoded by the nuclear genome, HSP60 mainly promotes the folding of relatively small soluble monomeric proteins (31, 32), and plays an important role in protein transport and folding in the mitochondria. HSP10 is widely present in a variety of mammalian tissues and can bind to unfolded proteins, effectively reducing energy barriers that occur during protein folding (33, 34). Mitochondrial ClpP is a serine protease located in the mitochondrial matrix. ClpP forms a proteolytic complex with AAA+ partner ClpX called ATP-dependent unfolding enzyme (ClpXP), which degrades misfolded or denatured proteins to participate in the quality control of mitochondrial proteins and maintain normal metabolic function (35). In this study, the expression levels of HSP10, HSP60, and ClpP in BMSCs were all upregulated within a certain period of time upon radio-frequency and X-rays exposure, indicating that both RF and X-rays could induce UPRmt in BMSCs, however, the level of unfolded protein response induced by ionizing X-rays radiation was much greater than non-ionizing radio frequency. This phenomenon possibly be explained by the more severe mitochondria damage and more misfolded protein aggregation caused by X-rays radiation.
Many literatures have reported that ionizing radiation and non-ionizing radiation could activate the JNK signaling pathway (36–39). The activation of JNK2 is closely related to the induction of UPR. Activation of JNK2 could trigger c-Jun binding to AP-1 elements to up-regulate CHOP and C/EBPβ transcription. Dimer of CHOP and C/EBPβ transcription factors binds to specific UPRmt promoter element and activates the target genes (40, 41). In this study, after interfering with siRNA-JNK2, the RF/XR exposures could not induce the increase of HSP10/HSP60/ClpP protein level, suggesting that JNK2 is involved in 900 MHz RF-induced UPRmt. Our results are in agreement with reported investigations (36–41).
To further investigate an association between RF exposure and activation of UPRmt, the level of ROS after RF and X-rays exposure were measured. The rationale for the study is that RF-induced ROS possibly causes mitochondrial damage, and hence the accumulation of misfolded/unfolded proteins which in turn activate UPRmt in BMSCs (2). One of the main sources of cellular ROS is the mitochondrial respiratory chain, when affected by external stimuli, ETC produces excessive ROS, disturbing the mitochondrial environment homeostasis (42, 43). When moderate ROS exists, it will play a beneficial physiological role, but when excessive ROS content increases, it will cause severe cell stress damage and even lead to cell death (44). For decades, people have been focused on the RF effect on the health of human body, many studies have tried to assess whether or not the RF can affect the production of ROS in cells. However, due to the differences in cell types, RF parameters and exposure time, etc., the effects of RF exposure on ROS levels are inconsistent (45). Some studies have shown that radio-frequency radiation could induce the increase of intracellular ROS content (46, 47). In this study, the increased ROS level are in line with the increased level of UPRmt-associated mitochondrial chaperone and protease. To date, very little is known about the mechanism underlying RF-induced UPRmt. Some investigations found that ROS increase is closely related to the induction of UPRmt, and UPRmt is in turn an indispensable and complex response that allows cells to buffer ROS (48, 49), suggesting that RF exposure could induce the occurrence of UPRmt via ROS production (50). The results of this research is consistent with the above reports.
Mitochondrial homeostasis determined the fate of cells. The functioning of the mitochondria are in turn tightly aligned to energy transduction and to the control of calcium and redox stress homeostasis (51). The observations obtained in our current study in BMSCs indicated that non-ionizing 900 MHz RF exposure at 120 μW/cm2 power density was capable of inducing UPRmt in response to mitochondria stress, increasing the expression of HSP10/HSP60/ClpP proteins, restoring mitochondrial homeostasis. Although RF exposure has already been demonstrated to activate UPRmt in this study, the detailed underlying mechanism remains to be further studied.
Data Availability Statement
The original contributions presented in the study are included in the article/supplementary material, further inquiries can be directed to the corresponding author/s.
Author Contributions
WX and RX conceived the manuscript, did the experiments, and wrote the draft of the manuscript. CF, CY, and HC reviewed the manuscript. YC designed the experiment and critically revised the manuscript. All authors listed have made a substantial, direct and intellectual contribution to the work, and approved it for publication.
Funding
This research was provided by the National Natural Science Foundation of China (Grant# 81373025) and Chinese Ministry of Science.
Conflict of Interest
The authors declare that the research was conducted in the absence of any commercial or financial relationships that could be construed as a potential conflict of interest.
Publisher's Note
All claims expressed in this article are solely those of the authors and do not necessarily represent those of their affiliated organizations, or those of the publisher, the editors and the reviewers. Any product that may be evaluated in this article, or claim that may be made by its manufacturer, is not guaranteed or endorsed by the publisher.
References
1. Yang L, Zhang C, Chen Z, Li C, Wu T. Functional and network analyses of human exposure to long-term evolution signal. Environ Sci Pollut Res Int. (2021) 28:5755–73. doi: 10.1007/s11356-020-10728-w
2. Haynes CM, Fiorese CJ, Lin YF. Evaluating and responding to mitochondrial dysfunction: the mitochondrial unfolded-protein response and beyond. Trends Cell Biol. (2013) 23:311–8. doi: 10.1016/j.tcb.2013.02.002
3. Haynes CM, Ron D. The mitochondrial UPR - protecting organelle protein homeostasis. J Cell Sci. (2010) 123:3849–55. doi: 10.1242/jcs.075119
4. Medinas DB, Hetz C. Protein homeostasis: modeling UPR adaptive responses. Nat Chem Biol. (2014) 10:879–80. doi: 10.1038/nchembio.1653
5. Quiros PM, Mottis A, Auwerx J. Mitonuclear communication in homeostasis and stress. Nat Rev Mol Cell Bio. (2016) 17:213–26. doi: 10.1038/nrm.2016.23
6. Yoneda T, Benedetti C, Urano F, Clark SG, Harding HP, Ron D. Compartment-specific perturbation of protein handling activates genes encoding mitochondrial chaperones. J Cell Sci. (2004) 117:4055–66. doi: 10.1242/jcs.01275
7. Pickles S, Vigie P, Youle RJ. Mitophagy and quality control mechanisms in mitochondrial maintenance. Curr Biol. (2018) 28:R170–85. doi: 10.1016/j.cub.2018.01.004
8. Sun Y, Zong L, Gao Z, Zhu S, Tong J, Cao Y. Mitochondrial DNA damage and oxidative damage in HL-60 cells exposed to 900MHz radiofrequency fields. Mutat Res. (2017) 797–9:7–14. doi: 10.1016/j.mrfmmm.2017.03.001
9. Santini SJ, Cordone V, Falone S, Mijit M, Tatone C, Amicarelli F. Role of mitochondria in the oxidative stress induced by electromagnetic fields: focus on reproductive systems. Oxid Med Cell Longev. (2018) :5076271. doi: 10.1155/2018/5076271
10. Tauffenberger A, Fiumelli H, Almustafa S, Magistretti PJ. Lactate and pyruvate promote oxidative stress resistance through hormetic ROS signaling. Cell Death Dis. (2019) 10:653. doi: 10.1038/s41419-019-1877-6
11. Melber A, Haynes CM. UPRmt regulation and output: a stress response mediated by mitochondrial-nuclear communication. Cell Res. (2018) 28:281–95. doi: 10.1038/cr.2018.16
12. Lin YF, Schulz AM, Pellegrino MW, Lu Y, Shaham S, Haynes CM. Maintenance and propagation of a deleterious mitochondrial genome by the mitochondrial unfolded protein response. Nature. (2016) 533:416–9. doi: 10.1038/nature17989
13. Gitschlag BL, Kirby CS, Samuels DC, Gangula RD, Mallal SA, Patel MR. Homeostatic responses regulate selfish mitochondrial genome dynamics in C. elegans. Cell Metab. (2016) 24:91–103. doi: 10.1016/j.cmet.2016.06.008
14. Ji Y, He Q, Sun Y, Tong J, Cao Y. Adaptive response in mouse bone-marrow stromal cells exposed to 900-MHz radiofrequency fields: Gamma-radiation-induced DNA strand breaks and repair. J Toxicol Environ Health A. (2016) 79:419–26. doi: 10.1080/15287394.2016.1176618
15. He Q, Zong L, Sun Y, Vijayalaxmi, Prihoda TJ, Tong J, et al. Adaptive response in mouse bone marrow stromal cells exposed to 900MHz radiofrequency fields: impact of poly (ADP-ribose) polymerase (PARP). Mutat Res. (2017) 820:19–25. doi: 10.1016/j.mrgentox.2017.05.007
16. Zong L, Gao Z, Xie W, Tong J, Cao Y. Role of NF-κB activation in mouse bone marrow stromal cells exposed to 900-MHz radiofrequency fields (RF). J Toxicol Environ Health A. (2019) 82:46–51. doi: 10.1080/15287394.2018.1553372
17. Li C, Yang L, Li CH, Xie Y, Wu T. Dosimetric variability of the rats' exposure to electromagnetic pulses. Electromagn Biol Med. (2015) 34:334–43. doi: 10.3109/15368378.2014.925472
18. Papa L, Germain D. Estrogen receptor mediates a distinct mitochondrial unfolded protein response. J Cell Sci. (2011) 124(Pt 9):1396–402. doi: 10.1242/jcs.078220
19. Papa L, Germain D. SirT3 regulates the mitochondrial unfolded protein response. Mol Cell Biol. (2014) 34:699–710. doi: 10.1128/MCB.01337-13
20. Zhao Q, Wang J, Levichkin IV, Stasinopoulos S, Ryan MT, Hoogenraad NJ. A mitochondrial specific stress response in mammalian cells. EMBO J. (2002) 21:4411–9. doi: 10.1093/emboj/cdf445
21. Mayevsky A, Rogatsky GG. Mitochondrial function in vivo evaluated by NADH fluorescence: from animal models to human studies. Am J Physiol Cell Physiol. (2007) 292:C615–40. doi: 10.1152/ajpcell.00249.2006
22. Yin F, Sancheti H, Liu Z, Cadenas E. Mitochondrial function in ageing: coordination with signalling and transcriptional pathways. J Physiol. (2016) 594:2025–42. doi: 10.1113/JP270541
23. Midzak AS, Chen H, Aon MA, Papadopoulos V, Zirkin BRJBoR. ATP synthesis, mitochondrial function, and steroid biosynthesis in rodent primary and tumor leydig cells. Biol Reprod. (2014) 84:976–85. doi: 10.1095/biolreprod.110.087460
24. Yang PM, Chen HC, Tsai JS, Lin LYJCRiT. Cadmium induces Ca2+-dependent necrotic cell death through calpain-triggered mitochondrial depolarization and reactive oxygen species-mediated inhibition of nuclear factor-kappaB activity. Chem Res Toxicol. (2014) 20:406–15. doi: 10.1021/tx060144c
25. Bukau B, Weissman J, Horwich A. Molecular chaperones and protein quality control. Cell. (2006) 125:443–51. doi: 10.1016/j.cell.2006.04.014
26. Pagliarini DJ, Calvo SE, Chang B, Sheth SA, Vafai SB, Ong SE, et al. A mitochondrial protein compendium elucidates complex I disease biology. Cell. (2008) 134:112–23. doi: 10.1016/j.cell.2008.06.016
27. Li CS, Xu CY, Wang RX, Yang L, Wu TN. Numerical evaluation of human exposure to 3.5-GHz electromagnetic field by considering the 3GPP-like channel features. Ann Telecommun. (2019) 74:25–33. doi: 10.1007/s12243-018-0682-z
28. Sarika S, Neeru K. Health implications of electromagnetic fields, mechanisms of action, and research needs. Adv Biol. (2014) 2014: 198609. doi: 10.1155/2014/198609
29. Xu S, Zhou Z, Zhang L, Yu Z, Zhang W, Wang Y, et al. Exposure to 1800 MHz radiofrequency radiation induces oxidative damage to mitochondrial DNA in primary cultured neurons. Brain Res. (2010) 1311:189–96. doi: 10.1016/j.brainres.2009.10.062
30. Zhu L, Luo X, Fu N, Chen L. Mitochondrial unfolded protein response: a novel pathway in metabolism and immunity. Pharmacol Res. (2021) 168:105603. doi: 10.1016/j.phrs.2021.105603
31. Cheng MY, Hartl FU, Martin J, Pollock RA, Kalousek F, Neupert W, et al. Mitochondrial heat-shock protein Hsp60 is essential for assembly of proteins imported into yeast mitochondria. Nature. (1989) 337:620–5. doi: 10.1038/337620a0
32. Yamano K, Kuroyanagi-Hasegawa M, Esaki M, Yokota M, Endo T. Step-size analyses of the mitochondrial Hsp70 import motor reveal the Brownian ratchet in operation. J Biol Chem. (2008) 283:27325–32. doi: 10.1074/jbc.M805249200
33. Höhfeld J, Hartl FU. Role of the chaperonin cofactor Hsp10 in protein folding and sorting in yeast mitochondria. J Cell Biol. (1994) 126:305–15. doi: 10.1083/jcb.126.2.305
34. Shan YX, Yang TL, Mestril R, Wang PH. Hsp10 and Hsp60 suppress ubiquitination of insulin-like growth factor-1 receptor and augment insulin-like growth factor-1 receptor signaling in cardiac muscle: implications on decreased myocardial protection in diabetic cardiomyopathy. J Biol Chem. (2003) 278:45492–8. doi: 10.1074/jbc.M304498200
35. Nouri K, Feng Y, Schimmer AD. Mitochondrial ClpP serine protease-biological function and emerging target for cancer therapy. Cell Death Dis. (2020) 11:841. doi: 10.1038/s41419-020-03062-z
36. Chen YR, Wang X, Templeton D, Davis RJ, Tan TH. The role of c-Jun N-terminal kinase (JNK) in apoptosis induced by ultraviolet C and gamma radiation. Duration of JNK activation may determine cell death and proliferation. J Biol Chem. (1996) 271:31929–36. doi: 10.1074/jbc.271.50.31929
37. Wong WY, Allie S, Limesand KH. PKCζ and JNK signaling regulate radiation-induced compensatory proliferation in parotid salivary glands. PLoS ONE. (2019) 14:e0219572. doi: 10.1371/journal.pone.0219572
38. Li F, Zheng X, Liu Y, Li P, Liu X, Ye F, et al. Different roles of CHOP and JNK in mediating radiation-induced autophagy and apoptosis in breast cancer cells. Radiat Res. (2016) 185:539–48. doi: 10.1667/RR14344.1
39. Buttiglione M, Roca L, Montemurno E, Vitiello F, Capozzi V, Cibelli G. Radiofrequency radiation (900 MHz) induces Egr-1 gene expression and affects cell-cycle control in human neuroblastoma cells. J Cell Physiol. (2007) 213:759–67. doi: 10.1002/jcp.21146
40. Mottis A, Jovaisaite V, Auwerx J. The mitochondrial unfolded protein response in mammalian physiology. Mamm Genome. (2014) 25:424–33. doi: 10.1007/s00335-014-9525-z
41. Barbour JA, Turner N. Mitochondrial stress signaling promotes cellular adaptations. Int J Cell Biol. (2014) 156020. doi: 10.1155/2014/156020
42. Yang S, Lian G. ROS and diseases: role in metabolism and energy supply. Mol Cell Biochem. (2020) 467:1–12. doi: 10.1007/s11010-019-03667-9
43. Castello PR, Drechsel DA, Patel M. Mitochondria are a major source of paraquat-induced reactive oxygen species production in the brain. J Biol Chem. (2007) 282:14186–93. doi: 10.1074/jbc.M700827200
44. Bertero E, Maack C. Calcium signaling and reactive oxygen species in mitochondria. Circ Res. (2018) 122:1460–78. doi: 10.1161/CIRCRESAHA.118.310082
45. Wang H, Zhang X. Magnetic fields and reactive oxygen species. Int J Mol Sci. (2017) 18:2175. doi: 10.3390/ijms18102175
46. Zhao L, Yang YF, Gao YB, Wang SM, Wang LF, Zuo HY, et al. Upregulation of HIF-1α via activation of ERK and PI3K pathway mediated protective response to microwave-induced mitochondrial injury in neuron-like cells. Mol Neurobiol. (2014) 50:1024–34. doi: 10.1007/s12035-014-8667-z
47. Houston BJ, Nixon B, King BV, Aitken RJ, De Iuliis GN. Probing the origins of 1,800 MHz radio frequency electromagnetic radiation induced damage in mouse immortalized germ cells and spermatozoa in vitro. Front Public Health. (2018) 6:270. doi: 10.3389/fpubh.2018.00270
48. Runkel ED, Liu S, Baumeister R, Schulze E. Surveillance-activated defenses block the ROS-induced mitochondrial unfolded protein response. PLoS Genet. (2013) 9:e1003346. doi: 10.1371/journal.pgen.1003346
49. Shao LW, Niu R, Liu Y. Neuropeptide signals cell non-autonomous mitochondrial unfolded protein response. Cell Res. (2016) 26:1182–96. doi: 10.1038/cr.2016.118
50. Runkel ED, Baumeister R, Schulze E. Mitochondrial stress: balancing friend and foe. Exp Gerontol. (2014) 56:194–201. doi: 10.1016/j.exger.2014.02.013
Keywords: mitochondria, radiofrequency fields, unfolded protein response, reactive oxygen species, microwave, heat shock protein
Citation: Xie W, Xu R, Fan C, Yang C, Chen H and Cao Y (2021) 900 MHz Radiofrequency Field Induces Mitochondrial Unfolded Protein Response in Mouse Bone Marrow Stem Cells. Front. Public Health 9:724239. doi: 10.3389/fpubh.2021.724239
Received: 12 June 2021; Accepted: 04 August 2021;
Published: 26 August 2021.
Edited by:
Tongning Wu, China Academy of Information and Communications Technology, ChinaCopyright © 2021 Xie, Xu, Fan, Yang, Chen and Cao. This is an open-access article distributed under the terms of the Creative Commons Attribution License (CC BY). The use, distribution or reproduction in other forums is permitted, provided the original author(s) and the copyright owner(s) are credited and that the original publication in this journal is cited, in accordance with accepted academic practice. No use, distribution or reproduction is permitted which does not comply with these terms.
*Correspondence: Yi Cao, eWljYW9Ac3VkYS5lZHUuY24=
†These authors have contributed equally to this work and share first authorship