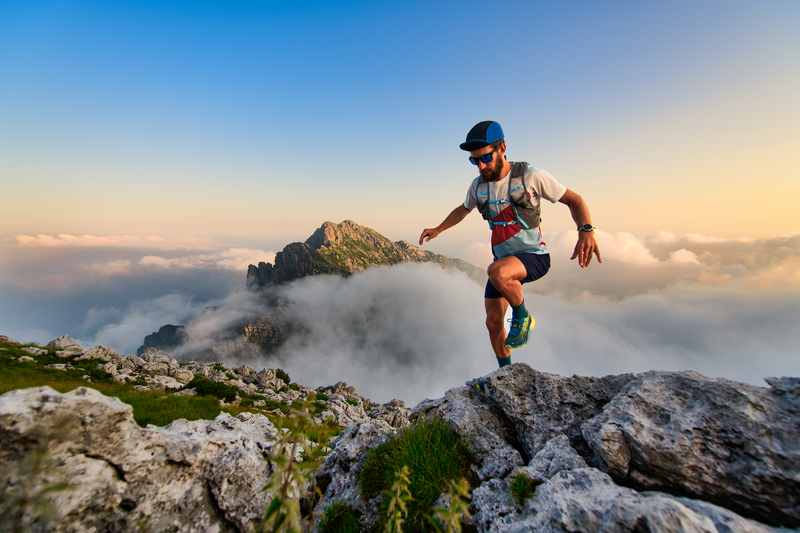
95% of researchers rate our articles as excellent or good
Learn more about the work of our research integrity team to safeguard the quality of each article we publish.
Find out more
CONCEPTUAL ANALYSIS article
Front. Public Health , 05 July 2021
Sec. Public Mental Health
Volume 9 - 2021 | https://doi.org/10.3389/fpubh.2021.664748
Nicotine is the primary pharmacologic component of tobacco, and its highly addictive nature is responsible for its widespread use and significant withdrawal effects that result in challenges to smoking cessation therapeutics. Nicotine addiction often begins in adolescence and this is at least partially attributed to the fact that adolescent brain is most susceptible to the neuro-inflammatory effects of nicotine. There is increasing evidence for the involvement of microglial cells, which are the brain's primary homeostatic sensor, in drug dependence and its associated behavioral manifestations particularly in the adolescent brain. A hallmark of neuro-inflammation is microglial activation and activation of microglia by nicotine during adolescent development, which may result in long-term addiction to nicotine. This non-systematic review examines multifactorial etiology of adolescent nicotine addiction, neurobiology of nicotine addiction and the potential mechanisms that underlie the effects of nicotine on inflammatory signaling in the microglia, understanding how nicotine affects the adolescent brain. We speculate, that modulating homeostatic balance in microglia, could have promising therapeutic potential in withdrawal, tolerance, and abstinence-related neural adaptations in nicotine addiction, in the adolescent brain. Further, we discuss nicotine addiction in the context of the sensitization-homeostasis model which provides a theoretical framework for addressing the potential role of microglial homeostasis in neural adaptations underlying nicotine abuse.
Nicotine addiction is the leading cause of preventable death and disease worldwide. Preclinical models and human studies have demonstrated that nicotine has cognitive-enhancing effects and these effects of nicotine may be an important factor in vulnerability to Tobacco Use Disorder (TUD) and may also contribute to difficulty in quitting smoking. The positive reinforcement effects of nicotine reflect nicotine's inherently rewarding effects that increase the probability of continued self-administration, and for both, the initiation and maintenance of tobacco use (1–3). Preclinical models typically used cell cultures or animal models that involve administration of nicotine to rodents. Preclinical models have consistently demonstrated that nicotine had both neuroprotective and anti-inflammatory effects depending on the nicotine dose administered and nicotine enhanced neurotrophic factors, increased cognition and impulsivity and developed neurotoxicity in the developing brain (2–4). Clinical studies evaluated the neurotoxic effects of tobacco smoking on the brain, and also evaluated the cognitive and behavioral assessments, as well as neuroimaging measures in the human brain, and have established that tobacco smoking decreases brain volume, increases neuro-inflammation and oxidative stress but enhances cognition and neural efficiency (4).
The National Institute of Drug Abuse (NIDA) reports that tobacco use is established primarily during adolescence, and evidence suggests that around 50% of those who start smoking in the adolescent years continue to smoke for 15–20 years (5). A National Youth Tobacco Survey (NYTS) found that 41.9% adolescents reported strong cravings for tobacco- a classic symptom of nicotine dependence (6). In adolescents, even infrequent smoking can result in an increased risk of dependence. In adolescents, monthly smoking can increase the likelihood of developing nicotine dependence by 10-fold as compared to adult smokers (7–10). The urge to smoke occurs early on after initiation, which drives the increase in frequency of use, exacerbating into nicotine dependence and a more rapid progression to addiction and a neurophysiologic dependence on nicotine (9). The risk of nicotine dependence in adolescents is associated with intensity of recent cigarette consumption, a slower nicotine metabolism and depression (11). The CDC warns that if cigarette smoking continues at the current rate among youth, 5.6 million of Americans younger than 18 will die early from a smoking-related illness (12).
Adolescence is a period of transition characterized by significant hormonal, psychosocial, and neural changes (13). This period is associated with development of social, emotional, and cognitive skills and also increased vulnerability to stress and risk-taking behaviors (14–16). The adolescent brain is undergoing maturation and is particularly vulnerable to the harmful effects of drugs of abuse, including tobacco and nicotine containing products. Nicotine binds to nicotinic acetylcholine receptors (nAChRs). nAChRs are widely distributed throughout the human brain and are critical in neurotransmitter release, brain maturation, reward processing, and cognition (17). Nicotine exposure during adolescence, disrupts the normal development, and expression of neuronal nAChRs, ultimately altering the function and pharmacology of the receptor subunits and changing the release of reward-related neurotransmitters (18).
E-cigarettes have emerged as the most common mode of nicotine delivery among youth across the U.S and its use is most prevalent among adolescents' and by vaping nicotine products, adolescents' do not have an awareness and understanding of nicotine and its presence within E-cigarettes products (19, 20). In adults, e-cigarettes are a potential cessation aid, while among adolescents who have never before smoked, e-cigarette use is associated with initiation or escalation of cigarette smoking (21, 22).
Smoking prevalence is a function of multiple parameters, such as initiation, cessation and relapse. Prevalence of adult smoking and cessation are both correlated with levels of childhood smoking intensity (23, 24). Adolescent smokers were the most likely to relapse and are more vulnerable to peer pressure which makes them more susceptible to smoking relapse after cessation (25). Adolescent smokers may underestimate the health consequences of smoking and therefore limit their determination to quit (26). A recent study that examined reuptake and relapse to tobacco use across a variety of tobacco products such as cigarettes, electronic nicotine delivery systems, cigars, hookah, and smokeless tobacco showed that for all the tobacco products reuptake occurred in 7.8% of adult previous users and 30.3% of adolescent previous users (27). These data affirm that preventive strategies should be designed early, so as to reduce, delay, or eliminate any youth access to cigarettes.
First-line pharmacologic therapies for smoking cessation includes nicotine replacement therapy (NRT), varenicline, and bupropion, however, the choice of therapy is based largely on patient preference. For those smokers willing to quit, a combination of behavioral support and pharmacologic therapy is the most effective in smoking cessation (28, 29). FDA has not approved cessation medications for adolescents, and NRT cannot be purchased over-the-counter by persons younger than 18 years of age (30, 31), but cessation medications can be prescribed for and used by adolescents under the supervision of a physician. A systematic meta-analysis study detected no significant efficacy of pharmacological therapy in adolescents, therefore, no definitive recommendations for pharmacotherapy for smoking cessation in adolescents could be made (32, 33). The tobacco cessation 5-A method (ask, advise, assess, assist, and arrange) that is used by adults, should be offered by the physician to all adolescents who smoke after assessment of the level of tobacco dependence in the adolescent using the Fagerström test for nicotine dependence (34). Therapies for adolescents should include counseling, nicotine replacement therapy, psychoactive medication (e.g., bupropion), and combination therapy (35).
Currently available cessation strategies include community interventions such as educational programs; anti-tobacco counter-advertising at the local, state, and national levels and curtailing access to tobacco via smoking bans at home and school and increased tobacco prices in combination with pharmacotherapy, all of which may be effective in decreasing tobacco use in adolescents. Novel smoking cessation experimental interventions using text messaging (36) peer mentoring (37) and digital or virtual self-help interventions (38) for adolescents may be more effective, however data supporting the effectiveness of such interventions at the current time are limited, however experts suggest that these novel strategies when used in combination with counseling and pharmacotherapy may be very effective (39).
Effects of nicotine are highly dependent on when exposure to the brain occurs and contributes to specific neural vulnerabilities at each brain developmental phase. Several studies have shown that prenatal, early postnatal, and adolescent brain maturation is physiologically regulated by acetylcholine (ACh) via activation of nicotinic acetylcholine receptors (nAChRs), and that nicotine exposure results in significant long-term deficits in the developing brain by interfering with the cholinergic regulatory processes (40–42). The dopaminergic system is dynamically changing during adolescence and stimulation by nicotine alters maturation of the mesocorticolimbic system via the nAChRs on dopaminergic neurons and microglia (43).
Given the susceptibility of the developing brain to nicotine as outlined above, preventing tobacco product use among youth is critical to ending the tobacco epidemic in the United States. Tobacco smoking continues to be the leading cause of preventable morbidity and mortality globally (44) which underscores the need for better therapeutics for nicotine dependence. In order to develop more effective therapeutic interventions, it is essential not only to understand the pathophysiology of addiction but also examine the adolescent neurobiology and the genetic predisposition that underlies the etiology of adolescent nicotine addiction.
We conducted a non-systematic literature review to examine in depth the multifactorial etiology of adolescent nicotine addiction. The review is largely based on a selection of current, high-quality articles in the field of neuroscience and epidemiology relevant to nicotine addiction with the goal of examining a potential relevant model, such as the sensitization-homeostasis model, which not only explains the development of nicotine addiction in adolescents, but is also strongly supported by scientific literature.
Epidemiological and clinical data have shown that exposure to tobacco or nicotine can lead to subsequent abuse of nicotine and other recreational drugs in adolescents, and this phenomenon is described as the gateway hypothesis (45). Parents can affect the health of their children through genetic factors, physical and mental health, health behaviors and socioeconomic status (11).
Nicotine dependence, depression, and parental socioeconomic factors, contribute significantly to poor health in early adulthood and adolescence (46).
Parental smoking and nicotine dependence directly increases child onset of smoking, daily smoking and nicotine addiction (47). Peer influence on the etiology and maintenance of smoking is enormous and predicts initiation, smoking persistence and dependence, and is also a mediator or progression to substance abuse (48). Although adolescent behavioral and personality characteristics may be associated with initiation, and continued use of cigarettes, individual genetic differences in initial sensitivity to nicotine may constitute a critical element in adolescent susceptibility to nicotine dependence (49).
Genetic Predisposition confers liability to nicotine dependence and variation in individual genes have been associated with nicotine dependence. The evidence for a significant role of genetic factors on nicotine dependence is substantial. Both linkage studies and genome wide association studies (GWAS) have identified candidate genes/genomic regions associated with nicotine dependence (50–53). Measured genetic variation are also associated with nicotine dependence treatment efficacy (54).
Genetic factors significantly influence both smoking initiation and persistence, a schematic of these influences are presented in Figure 1, these include genes associated with differences in nicotine's metabolic capacity and nicotine effects on central nervous system neurotransmitter functionality, specifically the dose that modulate direct and indirect effects on nAChR, dopaminergic and opioidergic activity, respectively. The candidate genes that play a key role in nicotine addiction include those associated with the dopaminergic neurotransmitter system (e.g., DRD2, DRD3, DRD4), cellular transport system (e.g., SLC1A2, SLC6A4), serotonergic neurotransmitter system (e.g., HTR2A), nicotinic neurotransmitter system (e.g., CHRNA4, CHRNA5, CHRNA3, CHRNA7, CHRNB4), opioidergic activity (e.g., OPRM1), and nicotine metabolism (e.g., CYP2A6) (55).
Figure 1. Schematic of Genetic factors that significantly influence both smoking initiation and persistence. Highlighted are genes associated with differences in nicotine's metabolic capacity and nicotine effects on central nervous system neurotransmitter functionality, specifically the those that modulate direct and indirect effects on nAChR, dopaminergic and opioidergic activity.
nAChRs are primary targets of nicotine, nicotine exerts direct and indirect effects on other receptor systems (e.g., opioid, serotonergic, glutamatergic) that also mediate nicotine-induced behavioral and neural changes in humans. Variation in the genes that code for the drug receptor proteins or that code for metabolic and catabolic enzymes that influence neurotransmitter levels, also represent the candidate genes for nicotine dependence and treatment.
The CYP2A6 genotype confers a slow nicotine metabolism increasing the risk of nicotine dependence (56). CYP2A6, is a genetically variable hepatic enzyme that is responsible for the majority of the metabolic inactivation of nicotine to cotinine. This enzyme mediates over 90% of the conversion of nicotine to cotinine, which is a major route of elimination of nicotine and therefore CYP2A6 activity is an important indicator of nicotine metabolism. A slow rate of nicotine conversion into cotinine results in a prolonged presence of higher nicotine concentrations in the bloodstream, thus increasing the exposure of nicotinic acetylcholine receptors in the brain to nicotine. Variant alleles of the CYP2A6 gene are associated with slower nicotine metabolism (57).
Nicotine from a smoked cigarette reaches the brain in as little as 7 s after inhalation (58). Inhalation of cigarette smoke results in nicotine quickly crosses the blood brain barrier and binding to nicotinic acetylcholine receptors (nAChRs) in the brain (59). Activation of nAChRs stimulates the mesocorticolimbic dopamine system which is the reward pathway thus producing the primary reinforcing effects of nicotine (60). Stimulation of dopamine neurons in the ventral tegmental area (VTA) by nicotine via high affinity α4β2 nAChRs causes increased firing in terminal dopaminergic fields, such as the nucleus accumbens (NAc), amygdala, and the prefrontal cortex (PFC) (61).
Exposure to nicotine in conjunction with environmental cues, causes lasting changes in dopaminergic function, which contribute to maintenance of smoking and the experience of withdrawal symptoms upon cessation (62–64). Disruption of dopaminergic activity via pharmacological blockade of dopamine receptors and disruption of nAChRs leads to decreased nicotine-induced reinforcement, suggesting a mediating role of these receptors in the reinforcing properties of nicotine (65).
Nicotine is a psychoactive and addictive substance that directly acts on brain areas involved in emotional and cognitive processing. Preclinical and clinical data suggests that although sociocultural influences significantly affect smoking adolescence, adolescent sensitivity to nicotine has strong neurobiological underpinnings (66).
Adolescence is a sensitive period for maturation of brain circuits that regulate cognition and emotion, with resulting vulnerability to the effects of nicotine and tobacco (67, 68). Adolescence is defined as a transitional period from childhood to adulthood that is conservatively estimated to last from 12 to 18 years of age in humans, however the boundaries of this period and what it encompasses is debatable and can vary widely depending on gender, socioeconomic status, and nutritional state (13).
Adolescence is marked by major physical changes in the body, however the hallmark of this period is a major reorganization of forebrain circuitry (13). During adolescence, the brain is sensitive to novel experiences with major experience-dependent plasticity occurring in the prefrontal cortex (PFC) region of the brain that is responsible for executive control and decision-making (69). Thus, dynamic structural and functional reorganization of the brain occurs during adolescence.
The structural changes in the adolescent brain include prolonged reorganization of gray matter, white matter, and associated neurochemical systems. During adolescence there is a significant decrease in the gray matter volume and density in the prefrontal cortex, parietal cortex and basal ganglia, which are critical brain regions for executive function, sensory processing, and motivation (70–72). On the other hand, there are corresponding increases in white matter, which reflect increased myelination and axonal diameter, and result in increased efficiency of impulse transduction (73). These changes in gray and white matter are not homogeneous and this imbalanced maturation of subcortical emotional and reward-focused systems as well as cortical executive and impulse control systems are believed to underlie the increased risk-taking behavior in adolescence (74, 75). These significant structural changes in the brain during adolescence, are accompanied by neurochemical changes which are paralleled by increases in functional connectivity, all of which synchronously play a significant role in the development of executive function and cognitive control attributed, to the maturation of the dopamine system (76, 77).
A functional MRI study examined the effects of nicotine dependence and tobacco consumption on brain structural changes in young adolescents and found that nicotine dependence was associated with distinct atrophy patterns on the brain volume (78). Mild nicotine dependence displayed more structural brain alternations than the heavy nicotine dependence and is attributed to the intensified neuroplasticity, a neural adaptation the adolescent brain undergoes against brain atrophy (79).
Studies have shown that micro-circuitry of the PFC and the NAc show developmental differences in dopamine function indicating that that cognitive processing within these regions is profoundly different in adolescence as compared to adulthood (66). Thus, rapidly maturing dopamine systems may be especially sensitive to disruption by environmental influences during adolescence, with long-term consequences on addiction behavior.
Smoking during adolescence increases the risk of developing psychiatric disorders and cognitive impairment in later life (80, 81). In addition, adolescent smokers suffer from attention deficits, which aggravate with the years of smoking (82–84). Recent studies in rodents reveal the molecular changes induced by adolescent nicotine exposure that alter the functioning of synapses in the PFC and that underlie the lasting effects on cognitive function (85). The PFC, is the brain area responsible for executive functions and attention performance, is one of the last brain areas to mature and is still developing during adolescence which makes the adolescent brain vulnerable to imbalance and therefore more susceptible to the influence of psychoactive substances such as nicotine (86). In prefrontal networks nicotine modulates information processing on multiple levels by activating and desensitizing nicotine receptors on different cell types and in this way affects cognition (87).
Comparison of smoking behavior of adolescents with that of adult's point to an enhanced sensitivity of the adolescent brain to addictive properties of nicotine. Adolescents report symptoms of dependence even at low levels of cigarette consumption (88, 89). Adolescents are uniquely sensitive to nicotine and therefore, understanding the distinct effects of nicotine use on the adolescent brain is critical to treating and preventing nicotine addiction. Nicotine interferes with adolescent brain maturation and causes persistent changes in neuronal signaling (41, 90). Nicotine exposure in adolescence modulates cortico-limbic processing and alters synaptic pruning patterns in reward-encoding brain regions (66, 91). Nicotine exposure may lead to higher levels of dependence by exerting neurotoxic effects in the prefrontal cortex (PFC) interfering with adolescent cognitive development, executive functioning, and inhibitory control (92). These effects are particularly evident under stressful or emotionally intense states and are most pronounced when smoking begins during early adolescence (93, 94). Neuronal nAChRs are central regulators of neurophysiology and signaling in addiction pathways and are widely distributed in neuroanatomical regions implicated in nicotine addiction (17). Adolescent nicotinic receptors in different neuroanatomical regions display significantly increased functionality compared with adult receptors (66, 95, 96). These data suggest that the underlying receptor mechanisms of nicotine tolerance differs between adults and adolescents, therefore the effectiveness of smoking cessation therapies differs between these group.
Adolescents also exhibit greater behavioral sensitivity and susceptibility to other drugs of abuse after nicotine exposure, contrary to that adults exposed to nicotine do not show enhanced behavioral sensitivity or susceptibility to other drugs of abuse (66, 97–99). The increased tolerance to nicotine in adolescents may contribute to an enhanced vulnerability, further the increase in adolescent nAChR functionality compared with adults may contribute to the shift in nicotine dependence (10, 41). Dopamine plays a large role in the rewarding effects of nicotine (66, 100). Since the dopaminergic system is still undergoing development during adolescence, nicotine-stimulated dopamine release is significantly higher during the early adolescent period (101).
In adults' dopamine release is attenuated during withdrawal, thus adolescents do not experience this same decrease in dopamine as adults and thus exhibit lower withdrawal symptoms and aversive effects (60, 102).
Nicotine withdrawal symptoms in adolescent smokers exhibit signs and symptoms that are characteristically associated with nicotine deprivation in adult smokers (103, 104). However, clinical studies suggests that the time course of withdrawal symptoms may be different for adolescents who are trying to achieve and maintain long-term abstinence and in those who have varying levels of nicotine dependence (10, 99).
Microglia are highly specialized resident immune cells of the brain and play a vital role in surveillance of the brain microenvironment, which enables them to detect and respond to perturbations by altering their own morphology based on the type of insult (105, 106). Recent studies have shown that microglia are critical mediators of anxiety-like behaviors in mice during nicotine withdrawal (107) and while microglia mediate both inflammatory responses in the brain and brain plasticity, little is known regarding their role in nicotine dependence and changes in microglial phenotypes in response to nicotine.
Adolescents are more to susceptible to microglial activation by nicotine as compared to adults which results in long term effects in terms of nicotine induced neuropathology and addiction (101, 108). Important structural and functional changes in synaptic plasticity and neural connectivity occur in different brain regions in adolescence (72, 74, 109). Most drugs of abuse activate microglia leading to a pro-inflammatory state which then alters neuro-circuits associated with reward and drug dependence (110–112).
Microglial activation phenotypes are described as (1) classic activation (M1 phenotype), (2) alternative activation (M2a phenotype), (3) alternative type II activation (M2b phenotype), and (4) acquired deactivation (M2c phenotype) (113, 114). M1 microglia are capable of producing reactive oxygen species (ROS) and produce cytokines such as tumor necrosis factor-α (TNF-α), IL-1β, IL-6, and IL-12, thereby mediating inflammatory tissue damage (115). The M1 phenotype is commonly referred to as neurotoxic (116, 117). M1 microglia regulate synaptic pruning (118) and exhibit limited phagocytic activity (119). M2a microglia exhibit significant phagocytic activity and respond to IL-4 and IL-13 stimulation by producing an insulin-like growth factor-1, anti-inflammatory cytokines such as IL-10; and to express G-CSF, GM-CSF, and CD209 (120–122). These microglia can stimulate tissue regeneration and can eliminate cellular debris. M2b microglia show increased IL-12, IL-10, and HLA-DR expression. M2b microglia also have significant phagocytic activity and an increased expression of CD32 and CD64. M2c also known as acquired deactivation phenotype is acquired as a result of stimulation with the anti-inflammatory cytokine IL-10 or glucocorticoids, shows increased expression of transforming growth factor (TGF), sphingosine kinase (SPHK1), and CD163 (123). The polarization of microglia toward the M2 phenotype occurs to resolve inflammation and degeneration as a whole; thus, this phenotype is characterized as neuroprotective (113, 114, 124, 125).
Nicotine induces both immunosuppressive and immuno-stimulatory effects in the CNS (126, 127). The translocator protein (TSPO) is used as a neuro-inflammatory marker as its expression is upregulated in reactive glial cells during CNS pathologies. However, it remains unclear in which microglial phenotypes TSPO levels are upregulated, as microglia can display a plethora of activation states that can be protective or detrimental to the brain. TSPO expression was selectively increased in M1 microglia but not M2 microglia. TSPO imaging reveals microgliosis in non-neurodegenerative brain pathologies, and this is perhaps reflected in the observation that cigarette smokers have decreased levels of TSPO suggesting that neuroprotective properties of nicotine and the anti-inflammatory responses of nicotine may be responsible for the decreased incidence in neurological diseases in smokers (128). Nicotine induced increases in brain inflammatory markers which are not only dose-dependent, but are also related to smoking intensity and time since smoking cessation (126). Neuroimaging studies, show gray-matter abnormalities throughout the brain, in smokers compared to non-smokers, and these are attributed to an upregulation of nicotinic acetylcholine receptors (nAChR) in the prefrontal cortex and the consequent differences in functional connectivity in the prefrontal cortex region in smokers compared to non-smokers (128, 129). Additional studies are needed to examine nicotine induced inflammatory responses and TSPO binding in human smokers during acute nicotine withdrawal in order to evaluate the therapeutic potential of microglial modulators as smoking cessation aids.
Adeluyi et al. showed that chronic nicotine treatment and nicotine withdrawal in adult mice both alter microglial morphology, however both conditions trigger different inflammatory responses in the brain NAc region (107). Chronic nicotine does not elicit a pro-inflammatory response in the NAc, but does induce microglial activation, while nicotine withdrawal does induce a pro-inflammatory response that involves the interaction between Nox-2, ROS, and TNFα in adult mice (107, 130). The NADPH oxidase (Nox) system is a major source of intracellular ROS production in the adult brain and the nicotine withdrawal induced activation of the Nox isoform-Nox-2 expression in microglia, which is believed to be the primary mechanism that results in increased ROS generation and pro-inflammatory response to nicotine withdrawal (131, 132).
In addition to their immune function, microglia are also involved in synaptic function and plasticity; therefore, we speculate that, their alterations within the NAc, which is a critical brain circuit for addictive processes, may enhance the development of aberrant synaptic connections and plasticity underlying nicotine dependency (111). Synaptic cues specific to the NAc during exposure to chronic nicotine or withdrawal from chronic nicotine distinctly influence the phenotype of its resident microglia. Nicotine induced neuro-plastic changes that contribute to nicotine addiction are triggered with initial exposure to nicotine and cause significant changes in brain physiology, structure and function, and changes in behavioral responses. Microglia play a critical role in synaptic remodeling and plasticity that underlies drug addiction (133, 134). Nicotine can directly modulate microglial morphology and function via interaction with nicotinic acetylcholine receptors (nAChRs) on microglia (135, 136). α7-nAChR is the only nAChR subtype expressed by microglia (137–142).
Activated microglia produce and release a variety of pro-inflammatory cytokines and augmenting the production of free radicals (143). Microglial cells express innate immune receptors, Toll like Receptors (TLRs) and cytoplasmic NOD-like immune receptors (NLRs) (144, 145), which react not only to pathogens (PAMPs, pathogen associated molecular patterns), but also to stress conditions, and to cell damage (DAMPS or damage-associated molecular patterns) (146). Activation of TLRs triggers signaling pathways, such as the activation of transcription factor NF-κB, which produces cytokines and inflammatory mediators (146). Several studies demonstrate the participation of these receptors in neuroinflammation and associated neuropathology is induced by nicotine abuse, particularly in adolescence (147).
Significant morphological differences exist between adult microglia and adolescent microglia, adult microglia were larger and have more complex morphology than adolescent microglia.
The transcriptional profile associated with immune activation is significantly different in adolescent microglia as compared to adult microglia (148). Nicotine treatment showed age-dependent effects on microglial marker Iba1 expression in the NAc and BLA which are actively maturing brain region during adolescence responsible for reward (66). Microglia express the receptor CX3CR1, which mediates developmental synaptic pruning through the neuronal ligand CX3CL1 (111).
Nicotine decreased overall expression of genes associated with microglial activation and nicotine alters the expression of these transcripts in an age-dependent manner which suggests that microglia are not fully mature by adolescence (101). A recent study showed that microglia are essential regulators of nicotine induced increases in cocaine seeking behavior (101) in adolescent microglia. Nicotine-induces microglial activation in the brain regions such as NAc, basolateral amygdala (BLA) which are responsible for reward (41, 66). The nicotine induced changes to microglial activation is mediated via the NAc localized D2 receptors and CX3CL1 signaling cascade suggesting that nicotine can induces significant changes to adolescent brain and behavior, and that microglial activation is a critical to this regulation (149). CX3CL1 not only mediates nicotine-induced increase in microglial activation, but increases the neuronal-microglial communication pathway via the CX3CL1-CX3CR1 interaction, after adolescent-nicotine exposure (149, 150).
Adult microglia treated with nicotine did not show either microglial proliferation nor activation demonstrating a neuroprotective effect of nicotine on adult microglia (151), however in the adolescent brain, nicotine treatment increased microglial activation (108) resulting in enhanced secretion of inflammatory cytokines, metabolic dysfunction, ineffective phagocytosis of proteins and neuronal debris, and alterations in neurochemical transmission producing long-term changes in limbic function (41, 152). The adolescence period is therefore a particularly vulnerable period during which, nicotine withdrawal induces microglial morphological changes in the nucleus accumbens (NAc) promoting microglial activation via Nox2-mediated increases in ROS.
Once activated microglia release pro-inflammatory cytokines TNFα and IL-1β, which correlate with increased withdrawal behaviors (107, 153). The increase in the pro-inflammatory cytokines occurs in both adolescents as well as adults, however, the increase in inflammatory cytokines in adolescents is significantly higher than that in adults (101, 154) (Figure 2).
Figure 2. Schematic that illustrates the effect of nicotine on microglial activation in adult microglia vs. adolescent microglia. M1 microglia represent a neurotoxic environment with increased levels of pro-inflammatory cytokines while M2 microglia are neuroprotective. Adolescent-nicotine exposed microglia show an increased reactive M1 activation and a pro-inflammatory response. Increased expression of pro-inflammatory cytokines CX3CR1, CXCL12, TNFrs11β, ROS, NOX-2, TNF-α, and TSPO are reported in nicotine exposed adolescent microglia as compared to nicotine exposed adult microglia.
Targeting the microglial potassium (KATP) channels has been shown to be effective in controlling inflammatory microglia activation, avoiding its toxic phenotype though a mitochondria-dependent mechanism (155). Such a strategy of modulating microglial activation and consequent neuroinflammation may be a novel therapeutic approach for treatment of nicotine withdrawal symptoms.
Nicotine withdrawal is associated with cognitive deficits including attention and episodic memory impairments. The presence of cognitive deficits correlated with microglial activation and the increased expression of neuro-inflammatory cytokines such as IL1β, TNFα and IFNγ, in the hippocampus and the prefrontal cortex regions of the brain (153). A non-steroidal anti-inflammatory drug (NSAID) such as indomethacin can prevent cognitive deficits and microglial activation during withdrawal, which suggests the potential use of anti-inflammatory agents to improve cognitive function during nicotine withdrawal (153).
The role of microglia in response to nicotine is further consolidated by experiments that show that microglial depletion reversed the microglial- related Nox2 and associated aberrant ROS production and also decreased anxiety-like behavior that is typical response to nicotine withdrawal (156).
Research investigating the role of microglia in nicotine dependence is limited and still novel, however, has potential implications in the development of more potent therapeutics to treat nicotine dependence and withdrawal.
Both the neurochemical and functional changes observed in adolescent brain regions are associated with dopamine modulation and the cerebral reward system, which are influenced by specific genes, suggesting that a genetic predisposition of the neural mechanisms is involved in the acquisition of dependence in nicotine addiction.
Identification of genes involved in the inheritance of specific smoking phenotypes may strengthen the selection of treatment options tailored to individual genotype (157). Although evidence for associations of CYP2A6 with smoking behavior and for the nicotine-metabolite ratio as a predictor of relapse are promising, cost effectiveness of implementing pharmacogenomics therapy would depend on the distribution of the relevant genetic polymorphisms in all smoking individuals (158). Pharmacogenomics and nicotine dependence is still an emerging science.
We speculate that neurodevelopmental changes may be modulated by pharmacotherapy targeted to activate change in microglial phenotype which may promote brain homeostasis and a neuro-adaptation that favors decreased dependence on nicotine thus microglia are a promising therapeutic target that need to be explored. Currently, data on role of microglial activation in nicotine cravings, withdrawal and tolerance is limited. We believe that the sensitization-homeostasis model (159–161), which highlights the concept that “nicotine's dependence liability derives from its ability to stimulate neural pathways responsible for the suppression of craving and due to sensitization,” will provide a theoretical framework for addressing the potential role of microglial homeostasis in withdrawal, tolerance and abstinence-related neural adaptations in nicotine addiction in both adults and adolescents. The sensitization-homeostasis model is unique in its extensive integration of clinical observations and basic science and its attribution of dependence to craving suppression and suggests that separate homeostatic mechanisms are responsible for abstinence, withdrawal, and tolerance (162).
Studies show that behavioral treatments particularly in adolescents are effective, whereas pharmacotherapies have only marginal success (28, 29, 32, 33). The side effect profiles for nicotine replacement therapy, bupropion, and varenicline in adolescents are similar to those reported in adult studies and none of these medications were efficacious in promoting long-term smoking cessation among adolescent smokers. The decision to use pharmacotherapy in adolescents should be individualized and should be administered in addition to cognitive-behavioral counseling and support.
Nicotine dependence over time can result in neuro-plastic changes in the brain (163), and therefore there is a possible concern for nicotine replacement therapy use during adolescence, which is that nicotine can change the neurodevelopmental trajectory. Therefore, understanding how nicotine affects the adolescent brain, and identifying novel therapeutics is essential to treating nicotine addiction in adolescents.
Cessation interventions utilizing mobile devices and social media also show promise in boosting tobacco cessation. Technology-based smoking cessation interventions such as the tobacco quitting helpline and other telehealth approaches are not only cost effective but increase the likelihood of adults and adolescents quitting, compared with no intervention.
Pharmacogenomics approaches hold promise for personalized treatment by increasing success rates in nicotine dependence treatment (82, 164–172). Thus, effective treatments that support tobacco cessation in both adults and adolescents should include both behavioral therapies and FDA-approved medications and further emphasis be placed on personalization of cessation treatments to increase the possibility of compliance and ensure success of the intervention.
Manuscript was written by SM and reviewed extensively and conceptualized by GH and AQ. All authors contributed significantly to this article.
The authors declare that the research was conducted in the absence of any commercial or financial relationships that could be construed as a potential conflict of interest.
1. LeSage MG, Smethells JR, Harris C. AStatus and future directions of preclinical behavioral pharmacology in tobacco regulatory science. Behav Anal. (2018) 18:252–74. doi: 10.1037/bar0000113
2. Jackson AB, Grobman S, Krishnan S. Recent findings in the pharmacology of inhaled nicotine: preclinical and clinical in vivo studies. Neuropharmacology. (2020) 176:108218. doi: 10.1016/j.neuropharm.2020.108218
3. Shoaib M, Perkins KA. Preclinical and clinical research on the discriminative stimulus effects of nicotine. Neuropharmacology. (2020) 170:108063. doi: 10.1016/j.neuropharm.2020.108063
4. Chang L, Liang H, Kandel SJJ. Independent and combined effects of nicotine or chronic tobacco smoking and HIV on the brain: a review of preclinical and clinical studies. J Neuroimmune Pharmacol. (2020) 15:658–93. doi: 10.1007/s11481-020-09963-2
5. Jamal AA, Gentzke S, Sean Hu KA, Cullen BJ, Apelberg DM, Hom BA, et al. Tobacco use among middle and high school students – United States, 2011-2016. MMWR Morb Mortal Wkly Rep. (2017) 66:597–603. doi: 10.15585/mmwr.mm6623a1
6. Apelberg BJ, Corey CG, Hoffman AC, Schroeder MJ, Husten CG, Caraballo RS, et al. Symptoms of tobacco dependence among middle and high school tobacco users: results from the 2012 National Youth Tobacco Survey. Am J Prev Med. (2014) 47(2 Suppl 1):S4–14. doi: 10.1016/j.amepre.2014.04.013
7. Ren M, Lotfipour S. Nicotine gateway effects on adolescent substance use. West J Emerg Med. (2019) 20:696–709. doi: 10.5811/westjem.2019.7.41661
8. Strong C, Juon HS, Ensminger ME. Effect of adolescent cigarette smoking on adulthood substance use and abuse: the mediating role of educational attainment. Subst Use Misuse. (2016) 51:141–54. doi: 10.3109/10826084.2015.1073323
9. DiFranza JR, Savageau JA, Rigotti NA. Development of symptoms of tobacco dependence in youths: 30 month follow up data from the DANDY study. Tob Control. (2002) 11:228–35. doi: 10.1136/tc.11.3.228
10. Siqueira MN, Committee On Substance Use and Prevention. Nicotine and tobacco as substances of abuse in children and adolescents. Pediatrics. (2017) 139:2016–3436. doi: 10.1542/peds.2016-3436
11. Griesler PC, Hu MC, Kandel DB. Nicotine dependence in adolescence and physical health symptoms in early adulthood. Nicotine Tob Res. (2016) 18:950–8. doi: 10.1093/ntr/ntv149
12. Singh T, Arrazola RA, Corey CG. Tobacco use among middle and high school students–United States, 2011–2015. MMWR Morb Mortal Wkly Rep. (2016) 65:361–7. doi: 10.15585/mmwr.mm6514a1
13. Spear LP. The adolescent brain and age-related behavioral manifestations. Neurosci Biobehav Rev. (2000) 24:417–63. doi: 10.1016/S0149-7634(00)00014-2
14. Steinberg L. A social neuroscience perspective on adolescent risk-taking. Dev Rev. (2008) 28:78–106. doi: 10.1016/j.dr.2007.08.002
15. Lipari R, Jean-Francois B. Trends in perception of risk and availability of substance use among full-time college students, The CBHSQ Report. Rockville, MD: Center for Behavioral Health Statistics and Quality, Substance Abuse and Mental Health Services Administration (2016).
16. Center for Behavioral Health Statistics and Quality. Behavioral Health Trends in the United States: Results From the 2014 National Survey on Drug Use and Health (HHS Publication No. SMA 15-4927, NSDUH Series H-50) (2015). Retrieved from: http://www.samhsa.gov/data/
17. Gotti C, Clementi F. Neuronal nicotinic receptors: from structure to pathology. Prog Neurobiol. (2004) 74:363–96. doi: 10.1016/j.pneurobio.2004.09.006
18. Adriani W, Macrì S, Pacifici R, Laviola G. Peculiar vulnerability to nicotine oral self-administration in mice during early adolescence. Neuropsychopharmacology. (2002) 27:212–24. doi: 10.1016/S0893-133X(02)00295-6
19. Fataar F, Hammond D. The prevalence of vaping and smoking as modes of delivery for nicotine and cannabis among youth in Canada, England and the United States. Int J Environ Res Public Health. (2019) 16:4111. doi: 10.3390/ijerph16214111
20. Perikleous EP, Steiropoulos P, Paraskakis E, Constantinidis TC, Nena E. E-cigarette use among adolescents: an overview of the literature and future perspectives. Front Public Health. (2018) 6:86. doi: 10.3389/fpubh.2018.00086
21. Choi K, Forster J. Characteristics associated with awareness, perceptions, and use of electronic nicotine delivery systems among young US Midwestern adults. Am J Public Health. (2013) 103:556–61. doi: 10.2105/AJPH.2012.300947
22. Pepper JK, Reiter PL, McRee AL, Cameron LD, Gilkey MB, Brewer NT. Adolescent males' awareness of and willingness to try electronic cigarettes. J Adolesc Health. (2013) 52:144–150. doi: 10.1016/j.jadohealth.2012.09.014
23. Alboksmaty A, Agaku IT, Odani S, Filippidis FT. Prevalence and determinants of cigarette smoking relapse among US adult smokers: a longitudinal study. BMJ Open. (2019) 9:e031676. doi: 10.1136/bmjopen-2019-031676
24. Hu TSL, Gall R, Widome LA, Bazzano TL, Burns SR, Daniels T, et al. Childhood/adolescent smoking and adult smoking and cessation: the international childhood cardiovascular cohort (i3C) consortium. J Am Heart Assoc. (2020) 9:e014381. doi: 10.1161/JAHA.119.014381
25. Collins SE, Witkiewitz K, Kirouac M, Marlatt GA. Preventing relapse following smoking cessation. Curr Cardiovasc Risk Rep. (2010) 4:421–8. doi: 10.1007/s12170-010-0124-6
26. El-Khoury Lesueur F, Bolze C, Melchior M. Factors associated with successful vs. unsuccessful smoking cessation: data from a nationally representative study. Addict Behav. (2018) 80:110–5. doi: 10.1016/j.addbeh.2018.01.016
27. Edwards KC, Kasza KA, Tang Z, Stanton CA, Stanton E, Sharma MJ, et al. Correlates of tobacco product reuptake and relapse among youth and adults in the USA: findings from the PATH Study Waves 1–3 (2013–2016). Tobacco Control. (2020) 29:s216–26. doi: 10.1136/tobaccocontrol-2020-055660
28. Maseeh A, Kwatra G. A review of smoking cessation interventions. Med Gen Med. (2005) 7:24. 10.1002/sim.2462]10.1002/sim.2462
29. Aubin HJ, Luquiens A, Berlin I. Pharmacotherapy for smoking cessation: pharmacological principles and clinical practice. Br J Clin Pharmacol. (2014) 77:324–36. doi: 10.1111/bcp.12116
30. Johnson KC, Klesges LM, Somes GW, Coday MC, DeBon M. Access of over-the-counter nicotine replacement therapy products to minors. Arch Pediatr Adolesc Med. (2004) 158:212–6. doi: 10.1001/archpedi.158.3.212
31. Karpinski JP, Timpe EM, Lubsch L. Smoking cessation treatment for adolescents. J Pediatr Pharmacol Ther. (2010) 15:249–63. doi: 10.5863/1551-6776-15.4.249
32. Kim Y, Myung SK, Jeon YJ, Lee EH, Park CH, Seo HG, et al. Effectiveness of pharmacologic therapy for smoking cessation in adolescent smokers: meta-analysis of randomized controlled trials. Am J Health Syst Pharm. (2011) 68:219–26. doi: 10.2146/ajhp100296
33. Bailey SR, Crew EE, Riske EC, Ammerman S, Robinson TN, Killen JD. Efficacy and tolerability of pharmacotherapies to aid smoking cessation in adolescents. Paediatr Drugs. (2012) 14:91–108. doi: 10.2165/11594370-000000000-00000
34. Heatherton TF, Kozlowski LT, Frecker RC, Fagerström KO. The Fagerström test for nicotine dependence: a revision of the Fagerstrom Tolerance Questionnaire. Br J Addict. (1991) 86:1125. doi: 10.1111/j.1360-0443.1991.tb01879.x
35. Leischow SJ, Muramoto ML, Matthews E, Floden LL, Grana RA. Adolescent smoking cessation with bupropion: the role of adherence, nicotine and tobacco research. Nicotine Tob Res. (2016) 18:1202–5. doi: 10.1093/ntr/ntv179
36. Hall AK, Cole-Lewis H, Bernhardt JM. Mobile text messaging for health: a and systematic review of reviews. Annu Rev Public Health. (2015) 36:393–415. doi: 10.1146/annurev-publhealth-031914-122855
37. Haines-Saah RJ, Kelly MT, Oliffe JL, Bottorff JL. Picture me smokefree: a qualitative study using social media and digital photography to engage young adults in tobacco reduction and cessation. J Med Internet Res. (2015) 7:e27. doi: 10.2196/jmir.4061
38. Carim-Todd L, Mitchell SH, Oken BS. Mind-body practices: an alternative, drug-free treatment for smoking cessation? A systematic review of the literature. Drug Alcohol Depend. (2013) 132:399–410. doi: 10.1016/j.drugalcdep.2013.04.014
39. Harvey J, Chadi N. Strategies to promote smoking cessation among adolescents. Paediatr Child Health. (2016) 21:201–8. doi: 10.1093/pch/21.4.201
40. Metherate R. Nicotinic acetylcholine receptors in sensory cortex. Learn Mem. (2004) 11:50–9. doi: 10.1101/lm.69904
41. Dwyer JB, McQuown SC, Leslie FM. The dynamic effects of nicotine on the developing brain. Pharmacol Ther. (2009) 122:125–39. doi: 10.1016/j.pharmthera.2009.02.003
42. Smith AM, Dwoskin LP, Pauly JR. Early exposure to nicotine during critical periods of brain development: mechanisms and consequences. J Pediatr Biochem. (2010) 1:125–41. doi: 10.1055/s-0036-1586367
43. Subramaniyan M, Dani JA. Dopaminergic and cholinergic learning mechanisms in nicotine addiction. Ann N Y Acad Sci. (2015) 1349:46–63. doi: 10.1111/nyas.12871
44. Samet JM. Tobacco smoking: the leading cause of preventable disease worldwide. Thorac Surg Clin. (2013) 23:103–12. doi: 10.1016/j.thorsurg.2013.01.009
45. Cross SJ, Lotfipour S, Leslie FM. Mechanisms and genetic factors underlying co-use of nicotine and alcohol or other drugs of abuse. Am J Drug Alcohol Abuse. (2017) 43:171–85 doi: 10.1080/00952990.2016.1209512
46. Lewinsohn PM, Olino TM, Klein DN. Psychosocial impairment in offspring of depressed parents. Psychol Med. (2005) 35:1493–503. doi: 10.1017/S0033291705005350
47. Kandel DB, Hu M-C, Griesler PC, Schaffran C. On the development of nicotine dependence in adolescence. Drug Alcohol Dependence. (2007) 91:26–39. doi: 10.1016/j.drugalcdep.2007.04.011
48. Brook JS, Whiteman M, Czeisler LJ, Shapiro J, Cohen P. Cigarette smoking in young adults. J Genet Psychol. (1997) 158:172–188. doi: 10.1080/00221329709596660
49. Eissenberg T, Balster RL. Initial tobacco use episodes in children and adolescents: current knowledge, future directions. Drug Alcohol Depend. (2000) 59:S41–60. doi: 10.1016/S0376-8716(99)00164-7
50. Gelernter J, Panhuysen C, Weiss R, Brady K, Poling J, Krauthammer M. Genomewide linkage scan for nicotine dependence: identification of a chromosome 5 risk locus. Biol Psychiatry. (2007) 61:119–26. doi: 10.1016/j.biopsych.2006.08.023
51. Swan GE, Hops H, Wilhelmsen KC, Lessov-Schlaggar CN, Cheng LS, Hudmon KS. A genome-wide screen for nicotine dependence susceptibility loci. Am J Med Genet B Neuropsychiatr Genet. (2006) 141:354–60. doi: 10.1002/ajmg.b.30315
52. Bierut LJ, Madden PA, Breslau N, Johnson EO, Hatsukami D, Pomerleau OF. Novel genes identified in a high-density genome wide association study for nicotine dependence. Hum Mol Genet. (2007) 16:24–35. doi: 10.1093/hmg/ddl441
53. Lerman C, Shields PG, Wileyto EP, Audrain J, Pinto A, Hawk L. Pharmacogenetic investigation of smoking cessation treatment. Pharmacogenetics. (2002) 12:627–34. doi: 10.1097/00008571-200211000-00007
54. Lee AM, Tyndale RF. Drugs and genotypes: how pharmacogenetic information could improve smoking cessation treatment. J Psychopharmacol. (2006) 20:7–14. doi: 10.1177/1359786806066039
55. Mackillop J, Obasi E, Amlung MT, McGeary JE, Knopik VS. The role of genetics in nicotine dependence: mapping the pathways from genome to syndrome. Curr Cardiovasc Risk Rep. (2010) 4:446–53. doi: 10.1007/s12170-010-0132-6
56. Karp I, O'Loughlin J, Hanley J, Tyndale RF, Paradis G. Risk factors for tobacco dependence in adolescent smokers. Tob Control. (2006) 15:199–204. doi: 10.1136/tc.2005.014118
57. Benowitz NL, Swan GE, Jacob P III, Lessov-Schlaggar CN, Tyndale RF. CYP2A6 genotype and the metabolism and disposition kinetics of nicotine. Clin Pharmacol Ther. (2006) 80:457–67. doi: 10.1016/j.clpt.2006.08.011
58. Maisto SA, Galizio M, Connors GJ, editors (2004). Drug Use and Abuse, 4th Edn. Belmont, CA, Wadsworth/Thompson Learning.
59. Dani JA, Heinemann S. Molecular and cellular aspects of nicotine abuse. Neuron. (1996) 16:905–8. doi: 10.1016/S0896-6273(00)80112-9
60. Di Chiara G. Role of dopamine in the behavioural actions of nicotine related to addiction. Eur J Pharmacol. (2000) 393:295–314 doi: 10.1016/S0014-2999(00)00122-9
61. Taber MT, Das S, Fibiger HC. Cortical regulation of subcortical dopamine release: mediation via the ventral tegmental area. J Neurochem. (1995) 65:1407–10. doi: 10.1046/j.1471-4159.1995.65031407.x
62. Mansvelder HD, McGehee DS. Long-term potentiation of excitatory inputs to brain reward areas by nicotine. Neuron. (2000) 27:349–57 doi: 10.1016/S0896-6273(00)00042-8
63. Miyata H, Yanagita T. Neurobiological mechanisms of nicotine craving. Alcohol. (2001) 24:87–93. doi: 10.1016/S0741-8329(01)00144-6
64. Balfour DJ. The neurobiology of tobacco dependence: a commentary. Respiration. (2002) 69:7–11. doi: 10.1159/000049362
65. Corrigall WA, Coen KM. Selective dopamine antagonists reduce nicotine self-administration. Psychopharmacology. (1991) 104:171–6. doi: 10.1007/BF02244174
66. Yuan M, Cross SJ, Loughlin SE, Leslie FM. Nicotine and the adolescent brain. J Physiol. (2015) 593:3397–412. doi: 10.1113/JP270492
67. Chambers RA, Taylor JR, Potenza MN. Developmental neurocircuitry of motivation in adolescence: a critical period of addiction vulnerability. Am J Psychiatry. (2003) 160:1041–52. doi: 10.1176/appi.ajp.160.6.1041
68. Crews F, He J, Hodge C. Adolescent cortical development: a critical period of vulnerability for addiction. Pharmacol Biochem Behav. (2007) 86:189–99. doi: 10.1016/j.pbb.2006.12.001
69. Bernheim A, Halfon O, Boutrel B. Controversies about the enhanced vulnerability of the adolescent brain to develop addiction. Front Pharmacol. (2013) 4:118. doi: 10.3389/fphar.2013.00118
70. Giedd JN, Blumenthal J, Jeffries NO, Castellanos FX, Liu H, Zijdenbos A, et al. Brain development during childhood and adolescence: a longitudinal MRI study. Nat Neurosci. (1999) 2:861–3. doi: 10.1038/13158
71. Sowell ER, Thompson PM, Holmes CJ, Batth R, Jernigan TL, Toga AW. Localizing age-related changes in brain structure between childhood and adolescence using statistical parametric mapping. Neuroimage. (1999) 9:587–97 doi: 10.1006/nimg.1999.0436
72. Sowell ER, Delis D, Stiles J, Jernigan TL. Improved memory functioning and frontal lobe maturation between childhood and adolescence: a structural MRI study. J Int Neuropsychol Soc. (2001) 7:312–22. doi: 10.1017/S135561770173305X
73. Paus T. Growth of white matter in the adolescent brain: myelin or axon? Brain Cogn. (2010) 72:26–35. doi: 10.1016/j.bandc.2009.06.002
74. Gogtay N, Giedd JN, Lusk L, Hayashi KM, Greenstein D, Vaituzis AC, et al. Dynamic mapping of human cortical development during childhood through early adulthood. Proc Natl Acad Sci USA. (2004) 101:8174–9. doi: 10.1073/pnas.0402680101
75. Casey B, Jones RM, Somerville LH. Braking and accelerating of the adolescent brain. J Res Adolesc. (2011) 21:21–33. doi: 10.1111/j.1532-7795.2010.00712.x
76. Luna B, Padmanabhan A, O'Hearn K. What has fMRI told us about the development of cognitive control through adolescence? Brain Cogn. (2010) 72:101–13. doi: 10.1016/j.bandc.2009.08.005
77. Padmanabhan A, Geier CF, Ordaz SJ, Teslovich T, Luna B. Developmental changes in brain function underlying the influence of reward processing on inhibitory control. Dev Cogn Neurosci. (2011) 1:517–29. doi: 10.1016/j.dcn.2011.06.004
78. Peng P, Wang Z, Jiang T, Chu S, Wang S, Xiao D. Brain-volume changes in young middle-aged smokers: a DARTEL-based voxel-based morphometry study. Clin Respir J. (2015) 11:621–31. doi: 10.1111/crj.12393
79. Peng P, Li M, Liu H, Tian YR, Chu SL, Van Halm-Lutterodt N, et al. Brain structure alterations in respect to tobacco consumption and nicotine dependence: a comparative voxel-based morphometry study. Front Neuroanat. (2018) 24:12:43. doi: 10.3389/fnana.2018.00043
80. Moylan S, Gustavson K, Karevold E, Øverland S, Jacka FN, Pasco JA, et al. The impact of smoking in adolescence on early adult anxiety symptoms and the relationship between infant vulnerability factors for anxiety and early adult anxiety symptoms: the TOPP study. PLoS ONE. (2013) 8:e63252. doi: 10.1371/journal.pone.0063252
81. Brown RA, Lewinsohn PM, Seeley JR, Wagner EF. Cigarette smoking, major depression, and other psychiatric disorders among adolescents. J Am Acad Child Psychiatry. (1996) 35:1602–1610 doi: 10.1097/00004583-199612000-00011
82. Andersson A, Hegvik T.-A., Chen Q, Rosenqvist MA, Kvalvik LG, et al. Attention-deficit/hyperactivity disorder and smoking habits in pregnant women. PLoS ONE. (2020) 15:e0234561. doi: 10.1371/journal.pone.0234561
83. Treur JL, Willemsen G, Bartels M, Geels LM, van Beek JHDA, Huppertz C, et al. Smoking during adolescence as a risk factor for attention problems. Biol Psychiatry. (2015) 78:656–63. doi: 10.1016/j.biopsych.2014.06.019
84. Liebrenz M, Fisher CE, Nellen R. Adult attention-deficit/hyperactivity disorder and nicotine withdrawal: a qualitative study of patient perceptions. BMC Psychiatry. (2016) 16:208. doi: 10.1186/s12888-016-0911-9
85. Adriani W, Spijker S, Deroche-Gamonet V, Laviola G, Le Moal M, Smit AB, et al. Evidence for enhanced neurobehavioral vulnerability to nicotine during periadolescence in rats. J Neurosci. (2003) 23:4712–16. doi: 10.1523/JNEUROSCI.23-11-04712.2003
86. Goriounova NA, Mansvelder HD. Short- and long-term consequences of nicotine exposure during adolescence for prefrontal cortex neuronal network function. Cold Spring Harbor Perspect Med. (2012) 2:a012120. doi: 10.1101/cshperspect.a012120
87. Goriounova NA, Mansvelder HD. Nicotine exposure during adolescence alters the rules for prefrontal cortical synaptic plasticity during adulthood. Front Synaptic Neurosci. (2012) 4:3. doi: 10.3389/fnsyn.2012.00003
88. Colby SM, Tiffany ST, Shiffman S, Niaura RS. Are adolescent smoker's dependent on nicotine? A review of the evidence. Drug Alcohol Depend. (2000) 59:S83–95. doi: 10.1016/S0376-8716(99)00166-0
89. Kandel DB, Chen K. Extent of smoking and nicotine dependence in the United States: 1991–1993. Nicotine Tob Res. (2000) 2:263–74 doi: 10.1080/14622200050147538
90. Huttenlocher PR. Synaptic density in human frontal cortex–developmental changes and effects of aging. Brain Res. (1979) 163:195–205. doi: 10.1016/0006-8993(79)90349-4
91. Selemon LD. A role for synaptic plasticity in the adolescent development of executive function. Transl Psychiatry. (2013) 13:2158–3188. doi: 10.1038/tp.2013.7
92. Masiero M, Cropley M, Pravettoni G. Increasing smoking cessation adherence: do we need to consider the role of executive function and rumination? Europe's J Psychol. (2020) 16:2279. doi: 10.5964/ejop.v16i1.2279
93. Flaudias V, Picot MC, Lopez-Castroman J, Llorca P.-M., Schmitt A, Perriot J, et al. Executive functions in tobacco dependence: importance of inhibitory capacities. PLoS ONE. (2016) 11:e0150940. doi: 10.1371/journal.pone.0150940
94. DeBry SC, Tiffany ST. Tobacco-induced neurotoxicity of adolescent cognitive development (TINACD): a proposed model for the development of impulsivity in nicotine dependence. Nicotine Tob Res. (2008) 10:11–25 doi: 10.1080/14622200701767811
95. Xiao C, Zhou CY, Jiang JH. Neural circuits and nicotinic acetylcholine receptors mediate the cholinergic regulation of midbrain dopaminergic neurons and nicotine dependence. Acta Pharmacol Sin. (2020) 41:1–9. doi: 10.1038/s41401-019-0299-4
96. Hoegberg BG, Lomazzo E, Lee NH, Perry DC. Regulation of α4β2α5 nicotinic acetylcholinergic receptors in rat cerebral cortex in early and late adolescence: Sex differences in response to chronic nicotine. Neuropharmacology. (2015) 99:347–55. doi: 10.1016/j.neuropharm.2015.08.015
97. Sinha R. Chronic stress, drug use, and vulnerability to addiction. Annals N Y Acad Sci. (2008) 1141:105–130. doi: 10.1196/annals.1441.030
98. Shram M, Funk D, Li Z. Nicotine self-administration, extinction responding and reinstatement in adolescent and adult male rats: evidence against a biological vulnerability to nicotine addiction during adolescence. Neuropsychopharmacol. (2008) 33:739–48. doi: 10.1038/sj.npp.1301454
99. Prochaska JJ, Benowitz NL. Current advances in research in treatment and recovery: nicotine addiction. Sci Adv. (2019) 5:9763. doi: 10.1126/sciadv.aay9763
100. Naneix F, Peters KZ, Young AMJ. Age-dependent effects of protein restriction on dopamine release. Neuropsychopharmacol. (2021) 46:394–403. doi: 10.1038/s41386-020-0783-z
101. Linker KE, Elabd MG, Tawadrous P. Microglial activation increases cocaine self-administration following adolescent nicotine exposure. Nat Commun. (2020) 11:306. doi: 10.1038/s41467-019-14173-3
102. Hildebrand BE, Nomikos GG, Hertel P, Schilstrom B, Svensson TH. Reduced dopamine output in the nucleus accumbens but not in the medial prefrontal cortex in rats displaying a mecamylamine-precipitated nicotine withdrawal syndrome. Brain Res. (1998) 779:214–25. doi: 10.1016/S0006-8993(97)01135-9
103. Jacobson LK, Krystal JH, Mencl EW, Westerveld M, Frost SJ, Pugh KR. Effects of smoking and smoking abstinence on cognition in adolescent tobacco smokers. Biological Psychiatry. (2005) 2005:57:56–66. doi: 10.1016/j.biopsych.2004.10.022
104. Killen JD, Ammerman S, Rojas N, Varady J, Haydel F, Robinson TN. Do adolescent smokers experience withdrawal effects when deprived of nicotine? Exp Clin Psychopharmacol. (2001) 9:176–82. doi: 10.1037/1064-1297.9.2.176
105. Evilsizor MN, Ray-Jones HF, Ellis TW. Microglia in experimental brain injury: implications on neuronal injury and circuit remodeling. In: Kobeissy FH, editor. Brain Neurotrauma: Molecular, Neuropsychological, Rehabilitation Aspects. Boca Raton, FL: CRC Press/Taylor and Francis; Chapter 8. (2015). Available online at: https://www.ncbi.nlm.nih.gov/books/NBK299191/
106. Kabba JA, Xu Y, Christian H. Microglia: housekeeper of the central nervous system. Cell Mol Neurobiol. (2018) 38:53–71. doi: 10.1007/s10571-017-0504-2
107. Adeluyi A, Guerin L, Fisher ML, Galloway A, Cole RD, Chan SSL, et al. Microglia morphology and proinflammatory signaling in the nucleus accumbens during nicotine withdrawal. Sci Adv. (2019) 5:eaax7031. doi: 10.1126/sciadv.aax7031
108. Linker KE, Cross SJ, Leslie FM. Glial mechanisms underlying substance use disorders. Addiction. (2019) 50:2574–89. doi: 10.1111/ejn.14163
109. Paolicelli RC. Synaptic pruning by microglia is necessary for normal brain development. Science. (2011) 333:1456–8. doi: 10.1126/science.1202529
110. Brenhouse HC, Schwarz JM. Immunoadolescence: neuroimmune development and adolescent behavior. Neurosci Biobehav Rev. (2016) 70:288–99. doi: 10.1016/j.neubiorev.2016.05.035
111. Lacagnina MJ, Rivera PD, Bilbo SD. Glial and neuroimmune mechanisms as critical modulators of drug use and abuse. Neuropsychopharmacology. (2017) 42:156–77. doi: 10.1038/npp.2016.121
112. Coller JK, Hutchinson MR. Implications of central immune signaling caused by drugs of abuse: mechanisms, mediators and new therapeutic approaches for prediction and treatment of drug dependence. Pharmacol Ther. (2012) 134:219–45. doi: 10.1016/j.pharmthera.2012.01.008
113. Hu X, Leak RK, Shi Y, Suenaga J, Gao Y, Zheng P, et al. Microglial and macrophage polarization—new prospects for brain repair. Nat Rev Neurol. (2015) 11:56–64. doi: 10.1038/nrneurol.2014.207
114. Lan X, Han X, Li Q. Modulators of microglial activation and polarization after intracerebral haemorrhage. Nat Rev Neurol. (2017) 13:420–33. doi: 10.1038/nrneurol.2017.69
115. Liu X, Quan N. Microglia and CNS interleukin-1: beyond immunological concepts. Front Neurol. (2018) 9:8. doi: 10.3389/fneur.2018.00008
116. Shechter R, Schwartz M. Harnessing monocyte-derived macrophages to control central nervous system pathologies: no longer ‘if' but ‘how'. J Pathol. (2013) 229:332–46. doi: 10.1002/path.4106
117. Fan H, Zhang K, Shan L, Kuang F, Chen K, Zhu K. Reactive astrocytes undergo M1 microglia/macrophages-induced necroptosis in spinal cord injury. Mol Neurodegener. (2016) 11:14. doi: 10.1186/s13024-016-0081-8
118. Schafer DP, Lehrman EK, Kautzman AG, Koyama R, Mardinly AR, Yamasaki R, et al. (2012). Microglia sculpt postnatal neural circuits in an activity and complement-dependent manner. Neuron. 74:691–705. doi: 10.1016/j.neuron.2012.03.026
119. Durafourt BA, Moore CS, Zammit DA, Johnson TA, Zaguia F, Guiot MC. Comparison of polarization properties of human adult microglia and blood-derived macrophages. GLI60 A. (2012) 60:717–27. doi: 10.1002/glia.22298
120. Martinez FO, Gordon S. The M1 and M2 paradigm of macrophage activation: time for reassessment. F1000prime Rep. (2014) 6:13. doi: 10.12703/P6-13
121. Franco R, Fernandez-Suarez D. Alternatively activated microglia and macrophages in the central nervous system. Prog Neurobiol. (2015) 131:65–86. doi: 10.1016/j.pneurobio.2015.05.003
122. Peferoen LA, Vogel DY, Ummenthum K, Breur M, Heijnen PD, Gerritsen WH. Activation status of human microglia is dependent on lesion formation stage and remyelination in multiple sclerosis. J Neuropathol Exp Neurol. (2015) 74:48–63. doi: 10.1097/NEN.0000000000000149
123. Wilcock DM. Neuroinflammatory phenotypes and their roles in Alzheimer's disease. Neurodegener Dis. (2014) 13:183–5. doi: 10.1159/000354228
124. Orihuela R, McPherson CA, Harry GJ. Microglial M1/M2 polarization and metabolic states. Br J Pharmacol. (2016) 173:649–65. doi: 10.1111/bph.13139
125. Zheng ZV, Wong KCG. Microglial activation and polarization after subarachnoid hemorrhage. Neuroimmunol Neuroinflammation. (2019) 6:1. doi: 10.20517/2347-8659.2018.52
126. Sopori M. Effects of cigarette smoke on the immune system. Nat Rev Immunol. (2002) 2:372–7. doi: 10.1038/nri803
127. Sopori ML, Kozak W. Immunomodulatory effects of cigarette smoke. J Neuroimmunol. (1998) 83:148–56. doi: 10.1016/S0165-5728(97)00231-2
128. Brody AL, Hubert R, Enoki R, Garcia LY, Mamoun MS, Okita K, et al. Effect of cigarette smoking on a marker for neuroinflammation: a [(11)C]DAA1106 positron emission tomography study. Neuropsychopharmacology. (2017) 42:1630–9. doi: 10.1038/npp.2017.48
129. Liao Y, Tang J, Liu T, Chen X, Hao W. Differences between smokers and non-smokers in regional gray matter volumes: a voxel-based morphometry study. Addict Biol. (2012) 17:977–80. doi: 10.1111/j.1369-1600.2010.00250.x
130. Blaser HC, Dostert TW, Mak DB. NF and ROS crosstalk in inflammation. Trends Cell Biol. (2016) 26:249–61. doi: 10.1016/j.tcb.2015.12.002
131. Orient A, Donkó A, Szabó A, Leto TL, Geiszt M. Novel sources of reactive oxygen species in the human body. Nephrol Dial Transplant. (2007) 22:1281–8. doi: 10.1093/ndt/gfm077
132. Rastogi R, Geng X, Li F, Ding Y. NOX activation by subunit interaction and underlying mechanisms in disease. Front Cell Neurosci. (2016) 10:301. doi: 10.3389/fncel.2016.00301
133. Miyamoto A, Wake H, Ishikawa AW, Eto K, Shibata K, Murakoshi H, et al. Microglia contact induces synapse formation in developing somatosensory cortex. Nat Commun. (2016) 7:12540. doi: 10.1038/ncomms12540
134. Torres L, Danver J, Ji K, Miyauchi JT, Chen D, Anderson ME, et al. (2016). Dynamic microglial modulation of spatial learning and social behavior. Brain Behav. Immun. 55:6–16. doi: 10.1016/j.bbi.2015.09.001
135. Dani JA, Balfour DJ. Historical and current perspective on tobacco use and nicotine addiction. Trends Neurosci. (2011) 34:383–92. doi: 10.1016/j.tins.2011.05.001
136. Leslie FM, Mojica CY, Reynaga DD. Nicotinic receptors in addiction pathways. Mol Pharmacol. (2013) 83:753–8. doi: 10.1124/mol.112.083659
137. Egea J, Buendia I, Parada E, Navarro E, León R, Lopez MG. Anti-inflammatory role of microglial alpha7 nAChRs and its role in neuroprotection. Biochem. Pharmacol. (2015) 97:463–72. doi: 10.1016/j.bcp.2015.07.032
138. De Simone R, Ajmone-Cat MA, Carnevale D, Minghetti L. Activation of alpha7 nicotinic acetylcholine receptor by nicotine selectively up-regulates cyclooxygenase-2 and prostaglandin E2 in rat microglial cultures. J Neuroinflammat. (2005) 2:4. doi: 10.1186/1742-2094-2-4
139. King JR, Gillevet TC, Kabbani N. AG protein-coupled alpha7 nicotinic receptor regulates signaling and TNF-alpha release in microglia. FEBS Open Bio. (2017) 7:1350–61. doi: 10.1002/2211-5463.12270
140. Mencel M, Nash M, Jacobson C. Neuregulin upregulates microglial alpha7 nicotinic acetylcholine receptor expression in immortalized cell lines: implications for regulating neuroinflammation. PLoS ONE. (2013) 8:e70338. doi: 10.1371/journal.pone.0070338
141. Shytle RD, Mori T, Townsend K, Vendrame M, Sun N, Zeng J, et al. Cholinergic modulation of microglial activation by alpha 7 nicotinic receptors. J Neurochem. (2004) 89:337–43. doi: 10.1046/j.1471-4159.2004.02347.x
142. Suzuki T, Hide I, Matsubara A, Hama C, Harada K, Miyano K, et al. Microglial alpha7 nicotinic acetylcholine receptors drive a phospholipase C/IP3 pathway and modulate the cell activation toward a neuroprotective role. J Neurosci Res. (2006) 83:1461–70. doi: 10.1002/jnr.20850
143. Sofroniew MV, Vinters HV. Astrocytes: biology and pathology. ActaNeuropathol. (2010) 119:7–35. doi: 10.1007/s00401-009-0619-8
144. Alfonso-Loeches S, Urena-Peralta J, Morillo-Bargues MJ, Gomez-Pinedo U, Guerri C. Ethanol-induced TLR4/NLRP3 neuroinflammatory response in microglial cells promotes leukocyte infiltration across the BBB. Neurochem Res. (2016) 41:193–209. doi: 10.1007/s11064-015-1760-5
145. Blanco AM, Valles SL, Pascual M, Guerri C. Involvement of TLR4/type I IL-1 receptor signaling in the induction of inflammatory mediators and cell death induced by ethanol in cultured astrocytes. J Immunol. (2005) 175:6893–9. doi: 10.4049/jimmunol.175.10.6893
146. Montesinos J, Pascual M, Rodriguez-Arias M, Minarro J, Guerri C. Involvement of TLR4 in the long-term epigenetic changes, rewarding and anxiety effects induced by intermittent ethanol treatment in adolescence. Brain Behav Immun. (2016) 53:159–71. doi: 10.1016/j.bbi.2015.12.006
147. Zhu R, Bu Q, Fu D, Shao X, Jiang L, Guo W, et al. Toll-like receptor 3 modulates the behavioral effects of cocaine in mice. J Neuroinflamm. (2018) 15:93. doi: 10.1186/s12974-018-1130-8
148. Sherafat Y, Bautista M, Fowler CD. Multidimensional intersection of nicotine, gene expression, and behavior. Front Behav Neurosci. (2021) 15:39. doi: 10.3389/fnbeh.2021.649129
149. Catale C, Gironda S, Lo Iacono L, Carola V. Microglial function in the effects of early-life stress on brain and behavioral development. J Clin Med. (2020) 9:468. doi: 10.3390/jcm9020468
150. Marinelli S, Basilico B, Marrone MC, Ragozzino D. Microglia-neuron crosstalk: Signaling mechanism and control of synaptic transmission. Semin Cell Dev Biol. (2019) 94:138–51. doi: 10.1016/j.semcdb.2019.05.017
151. Guan YZ, Jin XD, Guan LX, Yan HC, Wang P, Gong Z, et al. Nicotine inhibits microglial proliferation and is neuroprotective in global ischemia rats. Mol Neurobiol. (2015) 51:1480–8. doi: 10.1007/s12035-014-8825-3
152. Aldana BI. Microglia-specific metabolic changes in neurodegeneration. J Mol Biol. (2019) 431:1830–42. doi: 10.1016/j.jmb.2019.03.006
153. Saravia R, Ten-Blanco M, Grande MT, Maldonado R, Berrendero F. Anti-inflammatory agents for smoking cessation? Focus on cognitive deficits associated with nicotine withdrawal in male mice. Brain Behav Immun. (2019) 75:228–39. doi: 10.1016/j.bbi.2018.11.003
154. Liddelow SA, Guttenplan KA, Clarke LE, Bennett FC, Bohlen CJ, Schirmer L, et al. Neurotoxic reactive astrocytes are induced by activated microglia. Nature. (2017) 541:481–7. doi: 10.1038/nature21029
155. Rodriguez MJ, Martinez-Moreno M, Ortega FJ, Mahy N. Targeting Microglial K(ATP) channels to treat neurodegenerative diseases: a mitochondrial issue. Oxidative Med Cell Longev. (2013) 2013:194546. doi: 10.1155/2013/194546
156. Schiavone S, Sorce S, Dubois-Dauphin M, Jaquet VC, Marilena Z, Margherita C, et al. Involvement of NOX2 in the development of behavioral and pathologic alterations in isolated rats. Biol Psychiatr. (2009) 66:384–92. doi: 10.1016/j.biopsych.2009.04.033
157. Hu Y, Fang Z, Yang Y, Dekai Rohlsen N, Cheng F, Wang J. Analyzing the genes related to nicotine addiction or schizophrenia via a pathway and network based approach. Sci Rep. (2018) 8:2894. doi: 10.1038/s41598-018-21297-x
158. Roden DM, Altman RB, Benowitz NL, Flockhart DA, Giacomini KM, Johnson JA, et al. Pharmacogenetics research network. Ann Intern Med. (2006) 145:749–57. doi: 10.1038/sj.clpt.6100087
159. DiFranza JR, Wellman RJ. A sensitization-homeostasis model of nicotine craving, withdrawal, and tolerance: integrating the clinical and basic science literature. Nicotine Tob Res. (2005) 7:9–26. doi: 10.1080/14622200412331328538
160. DiFranza JR, Huang W, King J. Neuroadaptation in nicotine addiction: update on the sensitization-homeostasis model. Brain Sci. (2012) 2:523–52. doi: 10.3390/brainsci2040523
161. Difranza J, Ursprung W. Sanouri W. The latency to the onset of nicotine withdrawal: a test of the sensitization-homeostasis theory. Addictive Behav. (2008) 33:1148–53. doi: 10.1016/j.addbeh.2008.04.011
162. Bechara A, Berridge KC, Bickel WK, Morón JA, Williams SB, Stein JS. A neurobehavioral approach to addiction: implications for the opioid epidemic and the psychology of addiction. Psychol Sci Public Interest. (2019) 20:96–127. doi: 10.1177/1529100619860513
163. Farber HJ, Walley SC, Groner JA, Nelson KE. Section on tobacco control. Clinical practice policy to protect children from tobacco, nicotine, tobacco smoke. Pediatrics. (2015) 136:1008–17. doi: 10.1542/peds.2015-3108
164. Lessov-Schlaggar CN, Pergadia ML, Khroyan TV, Swan GE. Genetics of nicotine dependence and pharmacotherapy. Biochem Pharmacol. (2008) 75:178–95. doi: 10.1016/j.bcp.2007.08.018
165. Gomez-Nicola D, Perry VH. Microglial dynamics and role in the healthy and diseased brain: a paradigm of functional plasticity. Neuroscientist. (2015) 21:169–84. doi: 10.1177/1073858414530512
166. Kohno M, Link J, Dennis LE, McCready H, Huckans M, Hoffman WF, et al. Neuroinflammation in addiction: a review of neuroimaging studies and potential immunotherapies. Pharmacol Biochem Behav. (2019) 179:34–42. doi: 10.1016/j.pbb.2019.01.007
167. DiFranza JR. Implications of the autonomy theory of nicotine dependence. MedGenMed. (2002) 4:8. doi: 10.1001/archpedi.156.4.397
168. Nunes SO, Vargas HO, Prado E, Barbosa DS, de Melo LP, Moylan S, et al. The shared role of oxidative stress and inflammation in major depressive disorder and nicotine dependence. Neurosci Biobehav Rev. (2013) 37:1336–45. doi: 10.1016/j.neubiorev.2013.04.014
169. Johnston LD, Miech RA, O'Malley PM, Bachman JG, Schulenberg JE, Patrick ME. Monitoring the future national survey results on drug use, 1975–2017: overview, key findings on adolescent drug use (2018). Available online at: www.monitoringthefuture.org/pubs/monographs/mtf-overview2018.pdf
170. Beckers L, Ory D, Geric I, Declercq L, Koole M, Kassiou M, et al. Increased expression of translocator protein (TSPO) marks pro-inflammatory microglia but does not predict neurodegeneration. Mol Imaging Biol. (2018) 20:94–102. doi: 10.1007/s11307-017-1099-1
171. Blanco AM, Perez-Arago A, Fernandez-Lizarbe S, Guerri C. Ethanol mimics ligand-mediated activation and endocytosis of IL-1RI/TLR4 receptors via lipid rafts caveolae in astroglial cells. J. Neurochem. (2008) 106:625–39. doi: 10.1111/j.1471-4159.2008.05425.x
Keywords: nicotine, neuroscience, microglia, etiology, addiction, adolescent
Citation: Mahajan SD, Homish GG and Quisenberry A (2021) Multifactorial Etiology of Adolescent Nicotine Addiction: A Review of the Neurobiology of Nicotine Addiction and Its Implications for Smoking Cessation Pharmacotherapy. Front. Public Health 9:664748. doi: 10.3389/fpubh.2021.664748
Received: 05 February 2021; Accepted: 24 May 2021;
Published: 05 July 2021.
Edited by:
Jochen Mutschler, Private Clinic Meiringen, SwitzerlandReviewed by:
Manoranjan DSouza, Ohio Northern University, United StatesCopyright © 2021 Mahajan, Homish and Quisenberry. This is an open-access article distributed under the terms of the Creative Commons Attribution License (CC BY). The use, distribution or reproduction in other forums is permitted, provided the original author(s) and the copyright owner(s) are credited and that the original publication in this journal is cited, in accordance with accepted academic practice. No use, distribution or reproduction is permitted which does not comply with these terms.
*Correspondence: Supriya D. Mahajan, c21haGFqYW5AYnVmZmFsby5lZHU=
Disclaimer: All claims expressed in this article are solely those of the authors and do not necessarily represent those of their affiliated organizations, or those of the publisher, the editors and the reviewers. Any product that may be evaluated in this article or claim that may be made by its manufacturer is not guaranteed or endorsed by the publisher.
Research integrity at Frontiers
Learn more about the work of our research integrity team to safeguard the quality of each article we publish.