- 1Red Sea Research Centre and Computational Bioscience Research Center, King Abdullah University of Science and Technology, Thuwal, Saudi Arabia
- 2Bioscience and Environmental Science and Technology Division, King Abdullah University of Science and Technology, Thuwal, Saudi Arabia
Uniform CO2 during human evolution (180 to 280 ppm) resulted, because of the role of the CO2-bicarbonate buffer in regulating pH, in rather constant pH (7.35 to 7.45) in human fluids, cells and tissues, determining, in turn, the narrow pH range for optimal functioning of the human proteome. Herein, we hypothesize that chronic exposure to elevated pCO2 with increasing atmospheric CO2 (>400 ppm), and extended time spent in confined, crowded indoor atmospheres (pCO2 up to 5,000 ppm) with urban lifestyles, may be an important, largely overlooked driver of change in human proteome performance. The reduced pH (downregulated from 0.1 to 0.4 units below the optimum pH) of extant humans chronically exposed to elevated CO2 is likely to lead to proteome malfunction. This malfunction is due to protein misfolding, aggregation, charge distribution, and altered interaction with other molecules (e.g., nucleic acids, metals, proteins, and drugs). Such alterations would have systemic effects that help explain the prevalence of syndromes (obesity, diabetes, respiratory diseases, osteoporosis, cancer, and neurological disorders) characteristic of the modern lifestyle. Chronic exposure to elevated CO2 poses risks to human health that are too serious to be ignored and require testing with fit-for-purpose equipment and protocols along with indoor carbon capture technologies to bring CO2 levels down to approach levels (180–280 ppm) under which the human proteome evolved.
Introduction
pH is a key factor that determines the performance of the human proteome, as the chemical reactions involving proteins occur mainly in aqueous phases or at the interface between aqueous phases and biological membranes (1). pH ranges narrowly around 7.35, typically from 7.25 to 7.44, in human and mammalian blood and well-irrigated tissues (2) such as the brain (3) and the lungs (4). The narrow pH range in human blood and tissues is consistent with the optimal pH human proteome performance, which has been experimentally demonstrated to be narrowly constrained around 7.35 (Table 1). Deviations in pH from this optimal value may, thus, affect the performance of the human proteome and, more broadly, a range of additional biomolecules.
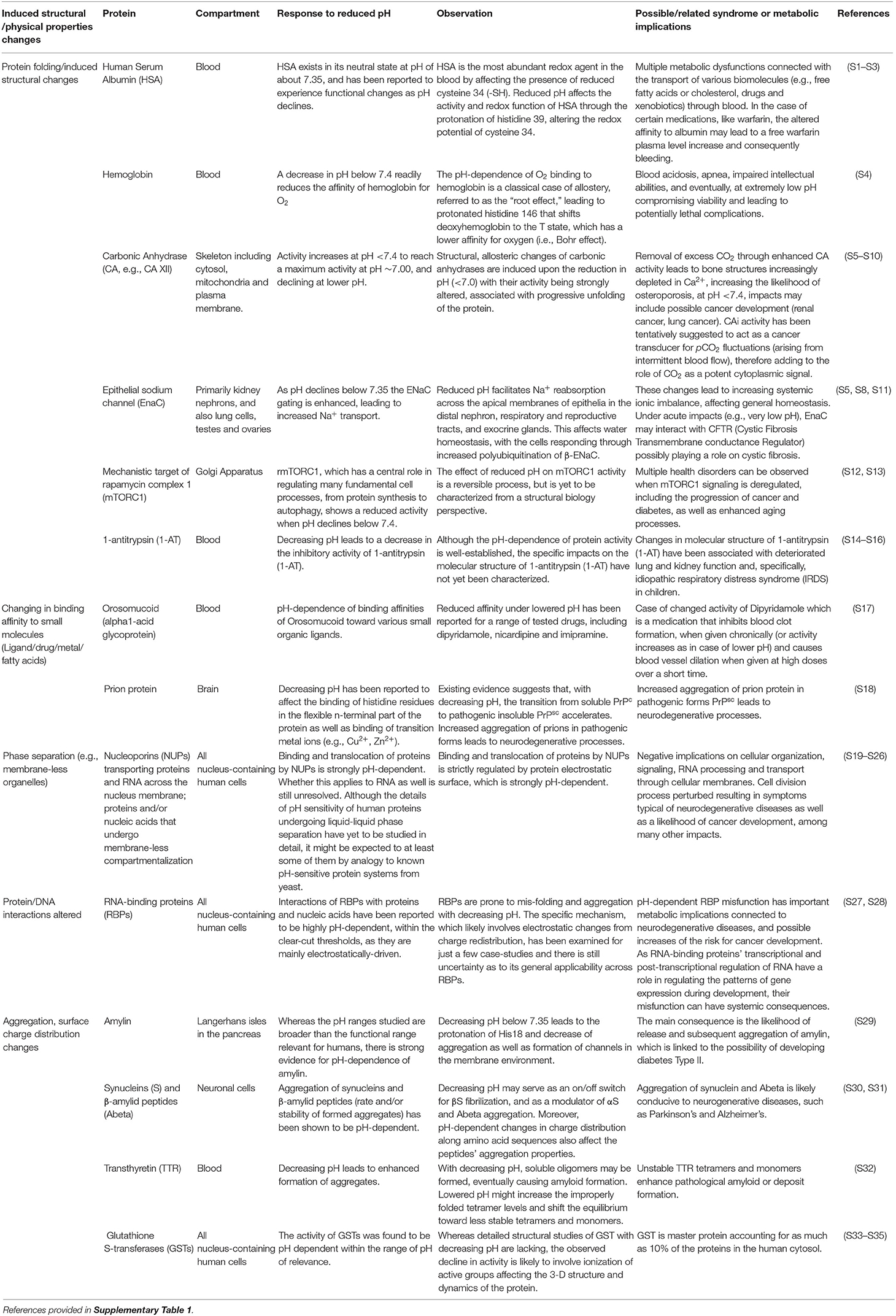
Table 1. Examples of different structural and physical changes resulting in the pH sensitive functioning of the human proteome and the associated health syndromes.
pH buffering in human blood is mainly controlled by the CO2-bicarbonate buffer system (1). In a reversible reaction catalyzed by carbonic anhydrase, gaseous CO2 reacts with H2O to form carbonic acid, which rapidly dissociates into bicarbonate and hydrogen ions. pH homeostasis by the CO2-bicarbonate buffer system is aided by additional buffering elements, including partially protonated histidine or cysteine side chains, the N-terminal α-amino groups of proteins, and organic/inorganic phosphate groups (5). Indeed, the marine CO2-bicarbonate system was preserved during the evolution of metazoans, initiated in the ocean about 700 Myrs ago (6), as the abundant buffer primarily responsible for pH homeostasis in metazoan fluids and tissue compartments (7). Indeed, CO2-bicarbonate is also the main buffer responsible for pH homeostasis in human fluids (2, 5, 8, 9).
However, the CO2-bicarbonate buffering system also links the pH in animal fluids, cells, and tissues to the pCO2 (partial pressure of carbon dioxide) in the ambient atmosphere [(2, 5, 10–12), Figure 1]. This resembles the link between ocean surface pH and atmospheric CO2, which is also mediated by the oceanic CO2-bicarbonate buffering system (13). As a result, changes in pCO2 in air results in a change of the blood pH in a matter of minutes (14, 15). The control ambient CO2 exerts on the pH of human blood (2, 16, 17) is then transferred to the pH regime of tissues irrigated by blood, which reflect changes in ambient CO2 (2).
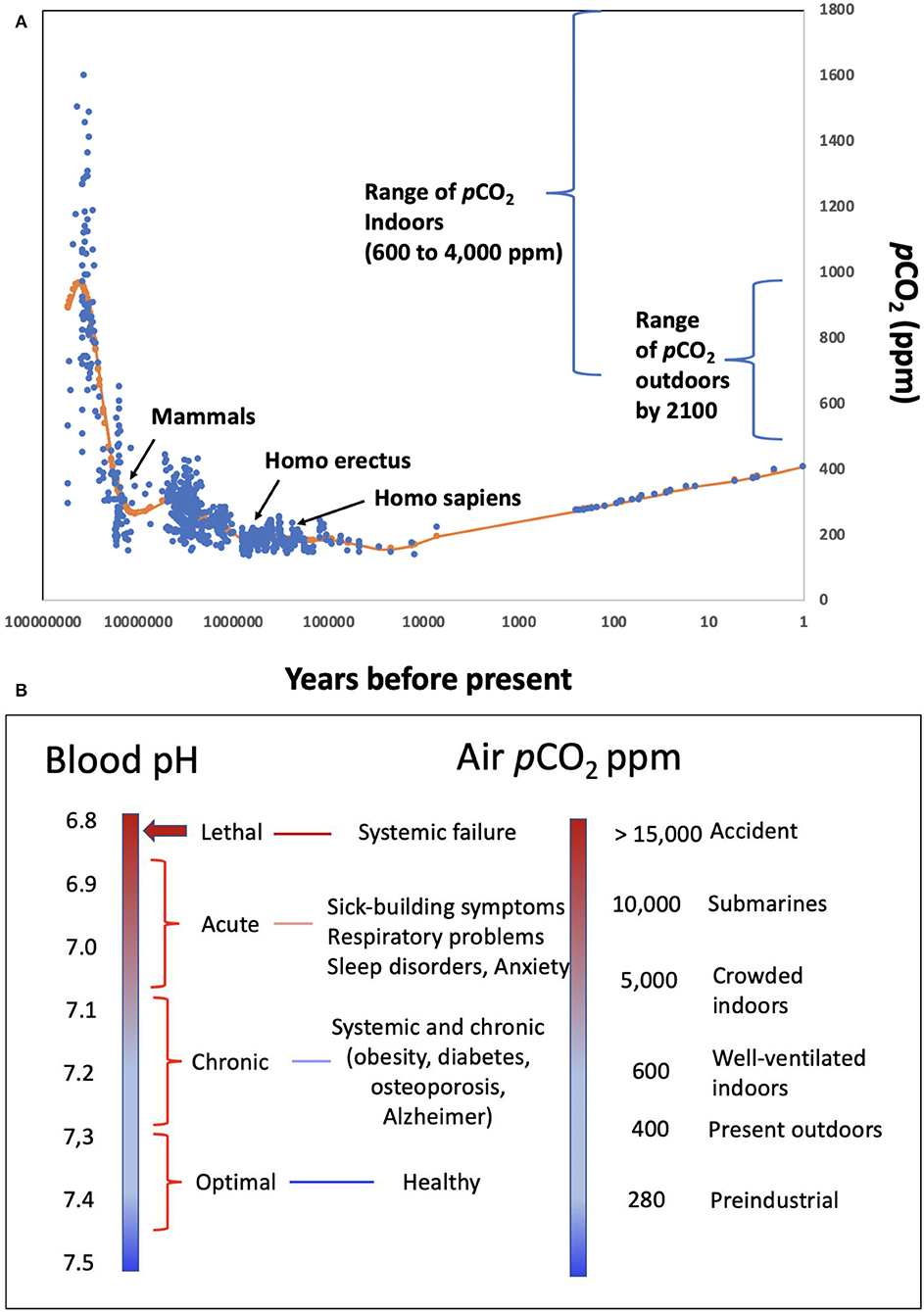
Figure 1. (A) pCO2 levels in the atmosphere experienced throughout the evolution of mammals and humans, as well as the range of pCO2 characteristic of indoors environments and the range expected by 2,100 under different emission scenario pathways (19); (B) correspondence between different levels of ambient pCO2, and the environments where these may be encountered, and expected blood pH values and human condition.
Atmospheric pCO2 has narrowly fluctuated between 135 and 280 ppm along glacial-interglacial cycles between the appearance of humans, about 1 million years, and the onset of the industrial revolution (Figure 1). During this period, the pH in human blood would have ranged narrowly between 7.35 and 7.45 for venous and arterial blood, respectively (18) (Figure 1), encompassing the optimal buffering capacity of the carbonate buffer.
The recent rise in atmospheric pCO2 that currently exceeds 400 ppm has exposed humans to atmospheric pCO2 levels unprecedented through the evolution of humans and mammals (Figure 1). This implies a slight decline of blood pH below the 7.35–7.45 optimal performance range for the human proteome, similar to the decline in ocean pH that has occurred since the industrial revolution (13, 20). The major change in human exposure to elevated pCO2, however, has arisen because of a shift in lifestyles. Until the 1950s, most people spent significant time outdoors. Now, people are living in increasingly confined and crowded spaces, such as those in heated and air-conditioned buildings and vehicles. Europeans, for example, currently spend over 90% of their time indoors [> 21 h per day; (21)]. pCO2 typically ranges from 600 to 800 ppm in well-ventilated indoor areas to 4,000 ppm or above in crowded, poorly ventilated environments (12, 22–25), such as school classrooms (26, 27) and conference rooms (10) (Figure 1). Most humans are therefore chronically exposed to elevated pCO2 levels comparable to or higher than the highest pCO2 experienced in the Earth's atmosphere over the past 400 Myr (28), which is unprecedented since the appearance of mammals (Figure 1). Accordingly, the pH of human blood and tissues is likely shifted to values significantly below (<-0.1 pH units) the 7.35 pH optimum throughout significant spans of our lives.
The important role of elevated CO2, and the associated decrease in blood pH in affecting human respiration, e.g., Haldane effect (29), and reducing hemoglobin affinity for O2 with increasing CO2, i.e., Bohr effect (30), have been known for at least one century, which therefore leads to the expectation that the elevated CO2 humans now experience may affect human performance (1, 12, 25, 31). Lethal levels of CO2 are on the order of 10% in air (32), corresponding to a lethal threshold blood pH of about 6.8 (Figure 1), and the recommended occupational threshold level for 8-h time-weighted average exposures to CO2 is 5,000 ppm (33). Experimental assessments show that exposure of pigs to air highly enriched in CO2 leads to rapid brain pH decline, declining to lethal levels of 6.7 within 1 min (34). Hence, exposure to high CO2 concentration in ambient air is used to promote pre-slaughter anesthesia in swine (34).
Exposure to pCO2 levels > 600 ppm has been shown to lead to the “sick-building syndrome,” resulting in irritation, fatigue, anxiety, headaches, and poor cognitive performance (22, 24–26, 35) and sleep apnea (36), linked to elevated pCO2 in blood (10). Exposure to elevated CO2 also leads to the emotional responses of fear and panic in humans, a behavior that has also been experimentally confirmed in rats (37). Elevated indoor CO2 concentrations, well in excess of 1,000 ppm CO2, are also characteristic of farm animal houses [e.g., (38, 39)]. Yet, there is a surprising lack of assessments in the veterinary literature of the effects of chronic exposure to elevated CO2 levels consistent with indoor concentrations, resulting in potential effects on animal health being ignored in reviews on the effects of climate change on farm animals (40).
The lowest ambient CO2 values that have been tested in chronic exposure studies with animal models are 2,000 and 3,000 ppm, including experiments on dogs, rats, and guinea pigs, as well as observations on humans in military submarine patrols (23, 41). These studies show that chronic exposure to elevated CO2 leads to increased gastric-acid secretion, increased calcification in kidneys, and increased CO2 uptake, but reduced Ca concentration in bones across mammal species tested, including humans, rats, guinea-pigs, pigs, and dogs (23, 41).
Existing arguments on the effect of elevated CO2 on human health focus on the effects of exposure to acute CO2 levels and/or cognitive responses to elevated indoor CO2 levels (24, 25). We submit, however, that broader, more profound but subtle changes may operate through disruptions of human proteome functions under chronic exposure to elevated CO2 levels, provided the associated reduction in blood pH below the 7.35 optimum. Yet, the effects of human exposure to elevated CO2 levels have been mostly assessed in terms of physiological and cognitive levels (23). The effects of human exposure to elevated CO2 on the proteome, which can elicit broad systemic impacts, have been poorly explored.
Here, we put forth the hypothesis that chronic exposure to elevated pCO2 with increasing atmospheric CO2 and extended time spent in confined, crowded indoor atmospheres is a major, largely overlooked, driver of change in the performance of the human proteome. Specifically, we describe the multiple ways in which lowered pH resulting from elevated pCO2 levels may affect the performance of the human proteome, leading to metabolic changes favoring multiple health syndromes characterizing modern, urban societies.
Discussion
A wealth of evidence for the effects of pH on the performance of proteins and, more broadly, biomolecules is available (Table 1). Unfortunately, many experiments assess pH ranges that are too broad (typically 2 pH units, Table 1), often using buffers (e.g., PBS—Phosphate-Buffered Saline) and acids (e.g., HCl—hydrochloric acid) to manipulate pH rather than the CO2-bicarbonate system operating in mammals. The 280 ppm pCO2 under which human proteins evolved, and that dictated the standard blood pH of 7.35 (Figure 1) at which the performance of most human proteins is optimized, is no longer experienced by humans, since atmospheric pCO2 has already exceeded 400 ppm and is expected to rise further (Figure 1). The shift in pH expected from the seven-fold increase in pCO2 experienced regularly by humans (from “standard” 280 ppm to the upper level of about 5,000 ppm in moderately crowded indoor environments, Figure 1), is calculated to involve a 0.3–0.4 pH unit reduction in blood (10, 42), cascading through all tissues and organs (Figure 1). Intracellular pH responds to changes in extracellular pH, with this coupling arising because the proteins that regulate intracellular pH are also sensitive to extracellular pH. This leads to a re-balancing of transmembrane acid–base fluxes that alter the steady-state intracellular pH, as recently demonstrated using high-throughput intracellular pH techniques (9). The rising pCO2 in the environment is, therefore, expected to lead to chronic acidosis, resulting in pH in human fluids chronically below the optimal pH of activity for the majority of human proteins (Table 1). Humans, therefore, regularly experience a dynamic pH range [[pH]pCO2] ranging from 7.1 to 7.45 (Figure 1), with the lower lethal pH threshold for humans being about 6.8 (43–45).
The pH-dependence of protein performance has been known for decades, but detailed understanding of the processes involved continues to grow (Table 1), providing evidence for a narrowly defined pH range for optimal performance of the human proteome [e.g., (46); Table 1, Figure 1], with the exception of proteins operating along the digestive tract, which perform at much lower pH values. Lowered pH affects protein folding, thereby affecting their ability to function properly (Table 1), through redistribution of surface charges that govern molecule-molecule interactions. For instance, when pH in the blood is reduced, the fraction of protonated histidine (His39) imidazole side chains in human serum albumin increases. This is important because human serum albumin is a major component of human blood. This affects the His39 role in preserving the required fraction of free thiols (-SH) in the blood, for which albumin (Cys34 residue existing in a reduced state) is the main provider [(47); Table 1]. pH changes within pCO2 also affect the aggregation of proteins, such as transthyretin in blood, synucleins and amyloid β-peptides in neuronal axons, and the human hormone amylin (Table 1), present in pancreas. Amylin misfolds into amyloid plaques with reduced pH, as a result of the protonation of the His18 residue that acts as an electrostatic switch to inhibit aggregation (48). Interactions between proteins and other proteins and/or nucleic acids are also likely to be pH dependent, affecting the formation of active complexes involved in gene regulation and repair mechanisms (Table 1). Changes in pH are likely to affect charge distribution on RNA-binding proteins (RBPs), thereby modulating protein-RNA interactions (Table 1). Moreover, RBPs are prone to misfolding as well as aggregation processes with shifting pH values (49, 50). Changes in the interactome with changes in pH also apply to their affinities for exogenous molecules such as drugs (51). Allosteric changes driven by molecular interactions are also pH dependent, as exemplified by the classic case of competition between O2 and CO2 binding sites in hemoglobin (52) (Figure 1).
Therefore, a reduction in pH with increased ambient pCO2 elicits a broad range of changes in the human proteome and the associated interactome (Table 1). Whereas these changes need to be better assessed within the relevant pH range (7.1–7.45) imposed by changes in CO2 (53), we submit that changes in the performance of the proteome with increasing pCO2 in the environment are very likely to contribute to a number of health syndromes affecting modern, urban societies (31). Brain performance is believed to be particularly sensitive to shifting pH (54). This is consistent with extensive epidemiological evidence that humans spending significant times in environments with elevated pCO2 suffer from attention deficit and learning disorders [(26, 55); e.g., Figure 1]. Moreover, the aggregation of proteins into insoluble amyloid fibrils, which could be achieved with a reduction in pH, is the hallmark of many highly debilitating brain pathologies such as Alzheimer's or Parkinson's disease.
The range of syndromes that may be rooted in chronic exposure to elevated pCO2 extend broadly to include diabetes (e.g., through pH-dependent changes in aggregation, Table 1), neurodegeneration (e.g., pH-dependent changes in synuclein and amyloid β-peptide aggregation, Table 1), schizophrenia and bipolar disorder (54), respiratory syndromes (e.g., pH-dependent changes in hemoglobin O2 affinity, i.e., the the Bohr effect, and α-trombin inhibitor activity, Table 1), osteoporosis (pH-dependent changes in carbonic anhydrase, Table 1), inflammation (23), and cancer (e.g., pH-dependent changes in GST, Table 1).
The role of elevated pCO2 is not limited, however, to indirect effects driven by reduced pH, but may also derive directly from the role of CO2 as a signaling molecule, broadly interpreted as corresponding to elevated metabolic demand. Exposure to elevated CO2 leading to a rise in arterial pCO2 and decrease in pH are detected by peripheral and central chemoreceptor cells and elicit a number of physiological and emotional responses. Emotional responses in humans exposed to elevated pCO2 result in distress behaviors, including fear, anxiety, and escape reactions. Similar responses have been confirmed experimentally on rat models exposed to very high CO2 levels (37), and involve the amygdala acting as a chemoreceptor for changes in pCO2/H+ and releasing adaptive responses. For instance, specific brainstem neurons (e.g., retrotrapezoid nucleus) are activated by elevated pCO2 and stimulate breathing (14, 36). The list of human cell types that functionally sense CO2, typically involving soluble adenylyl cyclase acting in tandem with carbonic anhydrase, is constantly expanding (56). Human preadipocytes were recently shown to sense elevated CO2, along the pH range of 7.1–7.4, triggering a cascade of responses that eventually lead to adipogenesis and obesity (53), a major problem of modern, sedentary societies exposed to chronically elevated pCO2 indoors. However, experiments testing such responses to chronic exposure at indoor (e.g., 2,000 and 3,000 ppm) CO2 levels are missing.
Consideration of the anomalous proteome of the naked mole rat (NMR, Heterocephalus glaber) provides further indirect evidence for the role of exposure to stable ambient CO2 on the evolution of a pH optimum for the performance of the mammalian proteome. NMR, a rodent from the African Horn, has received considerable attention because of their remarkable longevity and lack of cancer [over two decades compared to <3 years of other rodents; (57)]. NMR inhabits underground galleries under extreme crowding and, as a consequence, is adapted to extraordinarily high pCO2 levels in their burrows (58, 59). Remarkably, the only difference found thus far between NMR and its closest rodent relatives inhabiting outdoor environments is the substantially enhanced proteome fold stability, the resistance to reactive oxygen species (60), and/thus efficient DNA repair mechanisms in their acidized blood (60, 61). However, their remarkable properties vanish when NMRs are forced to live at atmospheres with ambient pCO2 (62). Thus, the adaptation of NMRs to life under high pCO2 levels is dependent, critically, on the adaptation toward increased protein stability at their low blood pH, suggesting their proteome has adapted to optimal performance at elevated pCO2 (therefore reduced pH) in their habitat. Hence, the proteome may evolve to perform at different optimal pCO2 conditions reflecting those prevailing in the habitat, but the pCO2 range for optimal performance remains narrow.
The link between elevated pCO2 and proteome stability and associated human health syndromes proposed here remains hypothetical and needs additional experimentation. This requires, however, that experiments with human cell lines be conducted under carefully controlled and monitored pH conditions (9). Unfortunately, most experiments using human cell lines neglect to control and monitor pH (9), and often use buffers absent from human fluids in the formulation of the culture medium (9). Equipment and standards to perform experiments with human cell lines controlling pH with CO2 additions (within the range 6.8–7.4), and to monitor pH and CO2 levels through the experiments are urgently needed. Protocols to examine the effects of pH changes within the range 6.8–7.4 on the structure and function of biomolecules are also required, as most assessments have been conducted, for technical convenience, across very large pH ranges of 2 pH units and greater (Table 1), which are beyond the physiological range for humans and mammals (Table 1).
In conclusion, we hypothesize that the five-fold rise in pCO2 levels that extant humans experience in their increasingly urban and sedentary lives relative to those they experienced during 1 million years of evolution may have systemic but yet overlooked impacts on the performance of the human proteome. Whereas, most research has focused on the consequences of acute exposure to extreme pCO2 levels, widespread impacts on the performance of the human proteome are also evident under the narrower pH range (7.10–7.45) corresponding to the chronically elevated CO2 levels most humans experience. Existing evidence suggests that these impacts are consistent with the diseases characteristic of modern, urban, and crowded lifestyles, such as diabetes, obesity, attention disorder, osteoporosis, cancer, anxiety, and prevalence of mental and respiratory disorders. The role of components of this lifestyle, such as unhealthy diets and sedentarism, on health has been extensively examined. However, the role of chronic exposure to elevated CO2 has only been addressed in relation to respiratory performance under acute exposure, while the more systemic effects operating through pH regulation of proteome performance have been largely overlooked. Yet, the link between elevated CO2 and modern lifestyle health syndromes proposed here provides a non-exclusive but parsimonious explanation for these disorders that has yet to be addressed. Ignoring the hypothesis formulated here may result in shifting health baselines of human populations that are continuously exposed to rising CO2 in the environment as humans adhere to increasingly crowded indoor, urban lifestyles. The suite of health syndromes associated with chronic exposure to elevated CO2 in indoor environments is not unavoidable (55), as solutions may be relatively simple. For instance, efficient ventilation systems in intelligent buildings combined with carbon capture technologies may be deployed in buildings to ensure CO2 levels indoors are conducive to healthier lifestyles (63, 64). Addressing the systemic effects that elevated CO2 may have on the human proteome requires the development of new approaches, standards, and equipment to examine its performance under narrow pH ranges. These efforts need progress beyond the very rough control of pH (typically 6.0–7.4) and the use of buffering systems absent from the human system, rather than the CO2-bicarbonate buffer, that characterize the majority of current research with human cell lines.
Author Contributions
All authors listed have made a substantial, direct and intellectual contribution to the work, and approved it for publication.
Funding
This work was supported by King Abdullah University of Science and Technology through baseline funding to CD, LJ, and MJ.
Conflict of Interest
The authors declare that the research was conducted in the absence of any commercial or financial relationships that could be construed as a potential conflict of interest.
Acknowledgments
We thank Mawadda Alghrably for help and Benjamin Gabriel Poulson for English editing.
Supplementary Material
The Supplementary Material for this article can be found online at: https://www.frontiersin.org/articles/10.3389/fpubh.2020.543322/full#supplementary-material
References
1. Casey JR, Grinstein S, Orlowski J. Sensors and regulators of intracellular pH. Nat Rev Mol Cell Biol. (2010) 11:50. doi: 10.1038/nrm2820
2. Robson J, Bone J, Lambie AT. Intracellular pH. Adv Clin Chem. (1969) 11:213–75. doi: 10.1016/S0065-2423(08)60060-8
3. Magnotta VA, Heo H-Y, Dlouhy BJ, Dahdaleh NS, Follmer RL, Thedens DR, et al. Detecting activity-evoked pH changes in human brain. Proc Nat Acad Sci. (2012) 109:8270–3. doi: 10.1073/pnas.1205902109
4. Effros RM, Chinard FP. The in vivo pH of the extravascular space of the lung. J Clin Invest. (1969) 48:1983–96. doi: 10.1172/JCI106164
5. Melzner F, Gutowska M, Langenbuch M, Dupont S, Lucassen M, Thorndyke M, et al. Physiological basis for high CO2 tolerance in marine ectothermic animals: pre-adaptation through lifestyle and ontogeny? Biogeosciences. (2009) 6:2313–31. doi: 10.5194/bg-6-2313-2009
6. Christen R, Ratto A, Baroin A, Perasso R, Grell K, Adoutte A. An analysis of the origin of metazoans, using comparisons of partial sequences of the 28S RNA, reveals an early emergence of triploblasts. EMBO J. (1991) 10:499–503. doi: 10.1002/j.1460-2075.1991.tb07975.x
7. Banfalvi G. Evolution of osmolyte Systems. Biochem Edu. (1991) 19:136–9. doi: 10.1016/0307-4412(91)90055-D
8. Hulikova A, Aveyard N, Harris AL, Vaughan-Jones RD, Swietach P. Intracellular carbonic anhydrase activity sensitizes cancer cell pH signaling to dynamic changes in CO2 partial pressure. J Biol Chem. (2014) 289:25418–30. doi: 10.1074/jbc.M114.547844
9. Michl J, Park KC, Swietach P. Evidence-based guidelines for controlling pH in mammalian live-cell culture systems. Commun Biol. (2019) 2:1–12. doi: 10.1038/s42003-019-0393-7
10. Vehviläinen T, Lindholm H, Rintamäki H, Pääkkönen R, Hirvonen A, Niemi O, et al. High indoor CO2 concentrations in an office environment increases the transcutaneous CO2 level and sleepiness during cognitive work. J Occup Environ Hyg. (2016) 13:19–29. doi: 10.1080/15459624.2015.1076160
11. Robertson D. The rise in the atmospheric concentration of carbon dioxide and the effects on human health. Med Hypotheses. (2001) 56:513–8. doi: 10.1054/mehy.2000.1256
12. Robertson D. Health effects of increase in concentration of carbon dioxide in the atmosphere. Curr Sci. (2006) 90:1607–1609.
13. Caldeira K, Jain AK, Hoffert MI. Climate sensitivity uncertainty and the need for energy without CO2 emission. Science. (2003) 299:2052–4. doi: 10.1126/science.1078938
14. Battisti-Charbonney A, Fisher J, Duffin J. The cerebrovascular response to carbon dioxide in humans. J Physiol. (2011) 589:3039–48. doi: 10.1113/jphysiol.2011.206052
15. Kolkman J, Steverink P, Groeneveld A, Meuwissen S. Characteristics of time-dependent PCO2 tonometry in the normal human stomach. Br J Anaest. (1998) 81:669–75. doi: 10.1093/bja/81.5.669
16. Jacobs M. Some aspects of cell permeability to weak electrolytes. In: Paper Presented at: Cold Spring Harbor Symposia on Quantitative Biology. (1940).
17. Caldwell PC. Intracellular pH. Int Re Cytol. (1956) 5:229–77. doi: 10.1016/S0074-7696(08)62572-3
18. Arthurs G, Sudhakar M. Carbon dioxide transport. Contin Edu Anaest Crit Care Pain. (2005) 5:207–10. doi: 10.1093/bjaceaccp/mki050
19. Geraldi NR, Klein SG, Anton A, Duarte CM. A framework for experimental scenarios of global change in marine systems using coral reefs as a case study. R Soc Open Sci. (2020) 7:191118. doi: 10.1098/rsos.191118
20. Doney SC. The growing human footprint on coastal and open-ocean biogeochemistry. Science. (2010) 328:1512–6. doi: 10.1126/science.1185198
21. Schweizer C, Edwards RD, Bayer-Oglesby L, Gauderman WJ, Ilacqua V, Jantunen MJ, et al. Indoor time–microenvironment–activity patterns in seven regions of Europe. J Expos Sci Environ Epidemiol. (2007) 17:170. doi: 10.1038/sj.jes.7500490
22. Seppänen O, Fisk W, Mendell M. Association of ventilation rates and CO2 concentrations with health andother responses in commercial and institutional buildings. Indoor Air. (1999) 9:226–52. doi: 10.1111/j.1600-0668.1999.00003.x
23. Jacobson TA, Kler JS, Hernke MT, Braun RK, Meyer KC, Funk WE. Direct human health risks of increased atmospheric carbon dioxide. Nat Sustain. (2019) 2:691–701. doi: 10.1038/s41893-019-0323-1
24. Du B, Tandoc MC, Mack ML, Siegel JA. Indoor CO2 concentrations and cognitive function: a critical review. Indoor Air. (2020). doi: 10.1111/ina.12706
25. Karnauskas KB, Miller SL, Schapiro AC. Fossil fuel combustion is driving indoor CO2 toward levels harmful to human cognition. GeoHealth. (2020) 4:e2019GH000237. doi: 10.1029/2019GH000237
26. Petersen S, Jensen K, Pedersen A, Rasmussen HS.The effect of increased classroom ventilation rate indicated by reduced CO2 concentration on the performance of schoolwork by children. Indoor Air. (2016) 26:366–79. doi: 10.1111/ina.12210
27. Sidorin D. Dynamics of carbon dioxide concentrations in the air and its effect on the cognitive ability of school students. Izv Atmosp Ocean Phys. (2015) 51:871–9. doi: 10.1134/S000143381508006X
28. Foster GL, Royer DL, Lunt DJ. Future climate forcing potentially without precedent in the last 420 million years. Nat Commun. (2017) 8:14845. doi: 10.1038/ncomms14845
29. Haldane J, Priestley JG. The regulation of the lung-ventilation. J Physiol. (1905) 32:225–66. doi: 10.1113/jphysiol.1905.sp001081
30. Bohr C, Hasselbalch K, Krogh A. Ueber einen in biologischer Beziehung wichtigen Einfluss, den die Kohlensäurespannung des Blutes auf dessen Sauerstoffbindung übt 1. Skandinavisches Archiv für Physiologie. (1904) 16:402–12. doi: 10.1111/j.1748-1716.1904.tb01382.x
31. Sethi R. Air Pollution: Sources, Prevention and Health Effects. Hauppauge, NY: Nova Science Publishers (2013).
32. Permentier K, Vercammen S, Soetaert S, Schellemans C. Carbon dioxide poisoning: a literature review of an often forgotten cause of intoxication in the emergency department. Int J Emerg Med. (2017) 10:14. doi: 10.1186/s12245-017-0142-y
33. ACGIH Co. TLVs and BEIs based on the documentation of the threshold limit values for chemical substances and physical agents, and biological exposure indices. In: Paper Presented at: American Conference of Governmental Industrial Hygienists Cincinnati. Cincinnati, OH (2008).
34. Martoft L, Stødkilde-Jørgensen H, Forslid A, Pedersen H, Jørgensen P. CO2 induced acute respiratory acidosis and brain tissue intracellular pH: a 31P NMR study in swine. Lab Anim. (2003) 37:241–8. doi: 10.1258/002367703766453092
35. Dai-Hua T, Jia-Shiang L, Chang-Chuan C. Office workers' sick building syndrome and indoor carbon dioxide concentrations. J Occup Environ Hyg. (2012) 9:345–51. doi: 10.1080/15459624.2012.675291
36. Guyenet PG, Bayliss DA. Neural control of breathing and CO2 homeostasis. Neuron. (2015) 87:946–61. doi: 10.1016/j.neuron.2015.08.001
37. Améndola L, Weary DM. Understanding rat emotional responses to CO2. Transl Psychiatry. (2020) 10:1–12. doi: 10.1038/s41398-020-00936-w
38. Clark P, McQuitty J. Air quality in six Alberta commercial free-stall dairy barns. Can Agric Eng. (1987) 29:77–80.
39. Dong H, Kang G, Zhu Z, Tao X, Chen Y, Xin H, et al. Ammonia, methane, and carbon dioxide concentrations and emissions of a hoop grower-finisher swine barn. Trans ASABE. (2009) 52:1741–7. doi: 10.13031/2013.29136
40. Rojas-Downing MM, Nejadhashemi AP, Harrigan T, Woznicki SA. Climate change and livestock: impacts, adaptation, and mitigation. Clim Risk Manag. (2017) 16:145–63. doi: 10.1016/j.crm.2017.02.001
41. Schaefer K. Effect of increased ambient CO2 levels on human and animals. Experientia. (1982) 38:1163–7.
42. Schaefer KE. Blood pH and pCO2 homeostasis in chronic respiratory acidosis related to the use of amine and other buffers. Ann N Y Acad Sci. (1961) 92: 401–13.
43. Kostek H, Kujawa A, Szponar J, Danielewicz P, Majewska M, Drelich G. Is it possible to survive metabolic acidosis with pH measure below 6.8? A study of two cases of inedible alcohol intoxication. Przeglad lekarski. (2011) 68:518–20.
44. Mackey D, Wasnic J. Morgan and Mikhail's Clinical Anesthesiology. 5th ed. New York, NY: McGraw-Hill Education (2013).
45. Betts JG, Young KA, Wise JA, Johnson E, Poe B, Kruse DH, et al. Anatomy and Physiology. Houston, TX: OpenStax College and Rice University (2013).
46. Talley K, Alexov E. On the pH-optimum of activity and stability of proteins. Proteins Struct Funct Bioinform. (2010) 78:2699–706. doi: 10.1002/prot.22786
47. Stewart AJ, Blindauer CA, Berezenko S, Sleep D, Tooth D, Sadler PJ. Role of Tyr84 in controlling the reactivity of Cys34 of human albumin. FEBS J. (2005) 272:353–62. doi: 10.1111/j.1742-4658.2004.04474.x
48. Jha S, Snell JM, Sheftic SR, Patil S, Daniels SB, Kolling F, et al. pH dependence of amylin fibrillization. Biochemistry. (2014) 53:300–10. doi: 10.1021/bi401164k
49. Harrison AF, Shorter J. RNA-binding proteins with prion-like domains in health and disease. Biochem J. (2017) 474:1417–38. doi: 10.1042/BCJ20160499
50. Bley N, Lederer M, Pfalz B, Reinke C, Fuchs T, Glaß M, et al. Stress granules are dispensable for mRNA stabilization during cellular stress. Nucleic Acids Res. (2014) 43:e26. doi: 10.1093/nar/gku1275
51. Urien S, Bree F, Testa B, Tillement J. pH-dependency of basic ligand binding to α1-acid glycoprotein (orosomucoid). Biochem J. (1991) 280:277–80. doi: 10.1042/bj2800277
52. Kelman GR. Digital computer subroutine for the conversion of oxygen tension into saturation. J Appl Physiol. (1966) 21:1375–6. doi: 10.1152/jappl.1966.21.4.1375
53. Kikuchi R, Tsuji T, Watanabe O, Yamaguchi K, Furukawa K, Nakamura H, et al. Hypercapnia accelerates adipogenesis: a novel role of high CO2 in exacerbating obesity. Am J Respir Cell Mol Biol. (2017) 57:570–80. doi: 10.1165/rcmb.2016-0278OC
54. Hagihara H, Catts VS, Katayama Y, Shoji H, Takagi T, Huang FL, et al. Decreased brain pH as a shared endophenotype of psychiatric disorders. Neuropsychopharmacology. (2018) 43:459. doi: 10.1038/npp.2017.167
55. Lowe RJ, Huebner GM, Oreszczyn T. Possible future impacts of elevated levels of atmospheric CO2 on human cognitive performance and on the design and operation of ventilation systems in buildings. Build Serv Eng Res Technol. (2018) 39:698–711. doi: 10.1177/0143624418790129
56. Fessler MB. CO2 as a Potential Obesogen: A Gas That Will Stick to Your Ribs. New York, NY: American Thoracic Society (2017).
57. Gorbunova V, Seluanov A, Zhang Z, Gladyshev VN, Vijg J. Comparative genetics of longevity and cancer: insights from long-lived rodents. Nat Rev Genet. (2014) 15:531. doi: 10.1038/nrg3728
58. Weber RE, Jarvis JU, Fago A, Bennett NC. O2 binding and CO2 sensitivity in haemoglobins of subterranean African mole rats. J Exp Biol. (2017) 220:3939–48. doi: 10.1242/jeb.160457
59. Holtze S, Braude S, Lemma A, Koch R, Morhart M, Szafranski K, et al. The microenvironment of naked mole-rat burrows in East Africa. Afr J Ecol. (2018) 56:279–89. doi: 10.1111/aje.12448
60. Pérez VI, Buffenstein R, Masamsetti V, Leonard S, Sakmon AB, Mele J, et al. Protein stability and resistance to oxidative stress are determinants of longevity in the longest-living rodent, the naked mole-rat. Proc Nat Acad Sci. (2009) 106:3059–64. doi: 10.1073/pnas.0809620106
61. Petruseva I, Evdokimov A, Lavrik O. Genome stability maintenance in naked mole-rat. Acta Naturae (англоязычная версия). (2017) 9:31–41. doi: 10.32607/20758251-2017-9-4-31-41
62. Welsh J, Traum T. Regarding mole rats and cancer. Vet Pathol. (2016) 53:1264–5. doi: 10.1177/0300985816646434
63. Gall ET, Nazaroff WW. New directions: potential climate and productivity benefits from CO2 capture in commercial buildings. Atoms Environ. (2015) 103:378–80. doi: 10.1016/j.atmosenv.2015.01.004
Keywords: CO2, pH, climate-change, human, health
Citation: Duarte CM, Jaremko Ł and Jaremko M (2020) Hypothesis: Potentially Systemic Impacts of Elevated CO2 on the Human Proteome and Health. Front. Public Health 8:543322. doi: 10.3389/fpubh.2020.543322
Received: 16 March 2020; Accepted: 21 September 2020;
Published: 16 November 2020.
Edited by:
Pierre Echaubard, SOAS University of London, United KingdomReviewed by:
Simon Rodrigo Rüegg, University of Zurich, SwitzerlandSandeep K. Sharma, Indian Institute of Toxicology Research (CSIR), India
Copyright © 2020 Duarte, Jaremko and Jaremko. This is an open-access article distributed under the terms of the Creative Commons Attribution License (CC BY). The use, distribution or reproduction in other forums is permitted, provided the original author(s) and the copyright owner(s) are credited and that the original publication in this journal is cited, in accordance with accepted academic practice. No use, distribution or reproduction is permitted which does not comply with these terms.
*Correspondence: Carlos M. Duarte, Y2FybG9zLmR1YXJ0ZUBrYXVzdC5lZHUuc2E=
†All authors have contributed equally to this work