- 1Mental Health Center and Psychiatric Laboratory, the State Key Laboratory of Biotherapy, West China Hospital, Sichuan University, Chengdu, China
- 2West China Biomedical Big Data Center, West China Hospital, Sichuan University, Chengdu, China
- 3Big Data Center, Sichuan University, Chengdu, China
Mood disorders, including major depressive disorder and bipolar disorder, have a profound impact on more than 300 million people worldwide. It has been demonstrated mood disorders were closely associated with deviations in biogenic amine metabolites, which are involved in numerous critical physiological processes. The peripheral and central alteration of biogenic amine metabolites in patients may be one of the potential pathogeneses of mood disorders. This review provides a concise overview of the latest research on biogenic amine metabolites in mood disorders, such as histamine, kynurenine, and creatine. Further studies need larger sample sizes and multi-center collaboration. Investigating the changes of biogenic amine metabolites in mood disorders can provide biological foundation for diagnosis, offer guidance for more potent treatments, and aid in elucidating the biological mechanisms underlying mood disorders.
1 Introduction
Mood disorder is a kind of chronic mental disorder, which has a great impact on people’s physical and mental health. The broadly defined mood disorders include major depressive disorder, bipolar disorder, dysthymia and other diseases (1, 2), while major depressive disorder and bipolar disorder, known as narrowly defined mood disorders, impact approximately 20% of population worldwide, placing a huge burden on the patients and society (3–6). Mood disorders, characterized by significant fluctuations in mood and sleep disturbances, can lead to suicidal tendencies. It is estimated that up to 50% of the 800,000 suicides worldwide each year occur during periods of depressive episodes (7, 8). The possible pathophysiology of major depressive illness has been attributed to neuroinflammation, hormones, and hereditary factors (9, 10). On the other hand, bipolar disorder seems to be caused by internal clock anomalies driven by low-grade inflammation (11). Studying the pathophysiology of mood disorders not only leads to more promising treatments, but also offers proof that the illnesses can be prevented altogether.
Prior research has demonstrated an extensive connection between mental health and physical fitness, with the biochemical alterations in the peripheral system potentially contributing to the development of mood disorders (12–14). The metabolites were reported to supply the substrates for biological processes and transmit vital signals via regulating other regulatory proteins (15), indicating whether or not the body’s physiological functions are proper. Biogenic amines, including catecholamines, indole amines and histamines, are nitrogen-containing compounds produced by α-decarboxylation of amino acids (16). Biogenic amines are highly prevalent in human tissues and are crucial for maintaining DNA replication, hormone synthesis, protein synthesis, cell activity, and other regular physiological processes (17). In the human body, biogenic amine metabolites, such as taurine, aniline, betaine, choline, histamine, kynurenine, and creatine, can respond to changes in cellular metabolism and provide insights into the normalcy of the physiological processes (18). In recent years, it has been discovered that these metabolites have an intimate connection with mental illnesses, particularly mood disorders (14, 19). Exploring the alterations in biogenic amine metabolites in the peripheral and central nervous systems of individuals suffering from mood disorders may not only offer an innovative approach to identify the conditions, but also shed light on the biological mechanisms underlying mood disorders and pave the way for the discovery of novel therapeutic targets in the future.
2 Histamine and mood disorders
Histamine, a metabolite of histidine, is expelled from mast cells in response to tissue damage or allergic reactions (20). As a transmitter in the nervous system and a signaling molecule in the gut, skin and immune system (21), histamine mainly exists in neuroepithelial cells and hematopoietic cells, and plays a great role in gastric acid secretion, immune regulation, bronchus smooth muscle contraction, and blood vessel dilation (21). The axons of histaminergic neurons predominantly reside in the posterior tuberomammillary nucleus of the hypothalamus (22). Histamine binds to the brain primarily through G-protein-coupled receptors, of which the brain contains four types: H1, H2, H3, and H4. (Table 1).
2.1 Major depressive disorder
As a neurotransmitter, histamine regulates several important brain functions, including cognition and mood (23). A clinical study showed that adolescents diagnosed with major depressive disorder exhibited notably elevated levels of peripheral histamine compared to healthy individuals (24). Not only peripheral histamine levels are different, but also histamine receptor levels in the brain of patients with depressive disorder are differentially expressed. The degree of the reduction in H1 receptor binding was found to be linked with the intensity of depression symptoms in the prefrontal cortex, frontal cortex, and cingulate gyrus (25), indicating that H1 receptors could be potential targets for treatment of major depressive disorder (26).
Histamine receptor inhibitors and inverse agonists have been demonstrated in numerous animal experiments to have antidepressant effects in mice (27–31) (Table 2). Previous studies found that adult mice lacking brain histamine exhibited indications of depression and reduced levels of activity (32). Selective serotonin reuptake inhibitors (SSRIs) such as citalopram have limited efficacy in treating depressive symptoms in mice with histamine synthesis disorders (33), suggesting that SSRIs antidepressants require a complete histamine system to be clinically effective. In addition, compared to normal mice, the mast cell-free mice reported elevated levels of anxiety and despair (34). Experiments revealed that inhibiting the activity of the H3 receptor using H3 receptor antagonists resulted in elevated levels of histamine, which in turn led to an upregulation of BDNF expression in the prefrontal and hippocampal regions of depressed mice via the H4 receptor. Additionally, this intervention normalized the overactive HPA axis, demonstrating an antidepressant effect (30).
However, early animal studies have shown that acute and chronic restraint stress elevate histamine turnover in the nucleus accumbens and striatum in rats (35, 36). After forced swimming experiment, there was a notable rise in the concentration of the histamine metabolite tele-methylhistamine in the cerebral cortex of mice. Antagonizing the H1 receptor may have an antidepressant effect since the rats’ forced swimming experiment’s rest period decreased after the H1 receptor antagonist was administered (37). In addition, the H3 receptor antagonist thioperamide can trigger the release of histamine in neurons, and the increased histamine stimulates the H1 receptor to mediate the anxiety effect, causing the mice exhibiting overt anxiety symptoms in the light-dark box experiment (38). H1 receptor antagonists injected into the lateral interventricular septum (39) and basolateral amygdala (40) significantly reversed anxiety-like behavior in rats. The aforementioned research demonstrates that both acute and long-term stress raise histamine turnover in the brain, which may be connected to the etiology of anxiety. It also reveals that anxiety symptoms may be mediated by the activation of the H1 receptor.
2.2 Bipolar disorder
The histaminergic system is involved in many basic physiological functions, including the sleep-wake cycle, which is strongly associated with bipolar disorder (41). Sectional analysis of the brains of bipolar disorder patients found that histamine H3 receptors in the prefrontal cortex may be involved in the regulation of cognitive impairment by regulating the connections between the hippocampus and various cortical and subcortical regions (22).
3 Kynurenine pathway and mood disorders
Tryptophan, an essential amino acid, forms a vary of neuroactive compounds with physiologically significant roles. Apart from the process of serotonin production, the kynurenine pathway is responsible for the metabolism of approximately 90% (42) of tryptophan. The kynurenine route commences with the transformation of tryptophan into kynurenine, which is the initial and rate-limiting step, facilitated by indoleamine 2, 3-dioxygenase (IDO) 1, IDO2, and tryptophan 2, 3-dioxygenase (TDO) (43). Numerous immune system cells express the IDO1 enzyme, such as macrophages, astrocytes, and microglia (44). Compared to IDO1 enzyme, IDO2 enzyme is mainly located in the liver and kidney with a reduced catalytic activity (45). Additionally, TDO is mostly found in the liver and can be preferentially triggered by glucocorticoids as well as low concentrations of reactive oxygen species (46). 60% of kynurenic acid in the central nervous system originates from the periphery and is able to cross the blood-brain barrier by utilizing neutral amino acid transporters (47). Kynurenine aminotransferase (KAT) I, II, III, and IV catalyzes the conversion of kynurenine in astrocytes to kynurenic acid, which is believed to have a neuroprotective impact against the excitotoxic and apoptotic effects of NMDA receptors (48, 49). In microglia and macrophages, kynurenine is progressively degraded to 3-hydroxykynurenine (3-HK), 3-hydroxycyanuric acid (3-HA), and ultimately converted to neurotoxic quinolinic acid (48)(Figure 1).
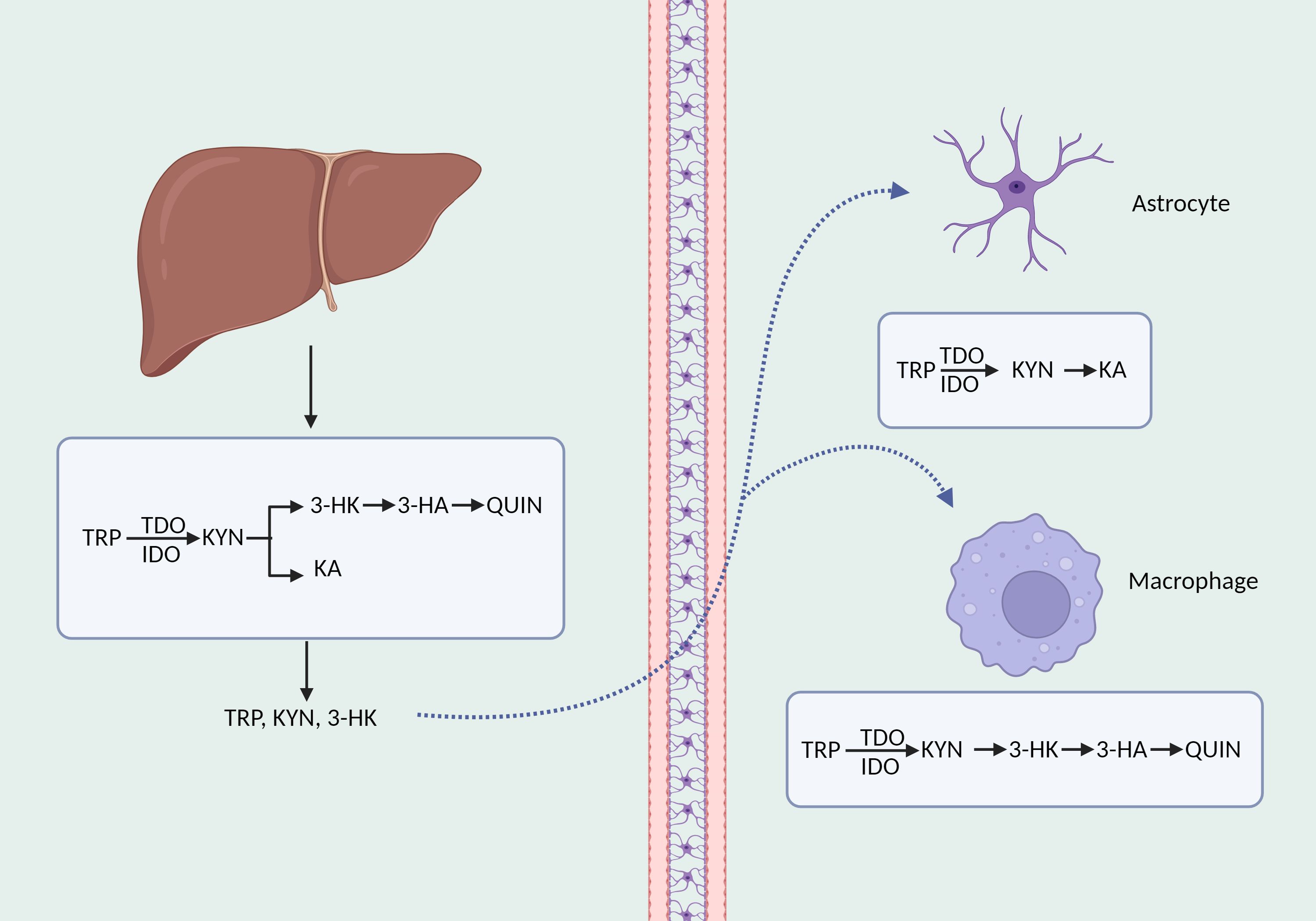
Figure 1. The synthesis and decomposition of kynurenine. TRP, tryptophan; KYN, kynurenine; QUIN, quinolinic acid; KA, kynurenic acid.
3.1 Major depressive disorder
Kynurenine pathway metabolites are intimately related to inflammatory pathways, and recent studies indicated that disruption of the kynurenine pathway could potentially contribute to the etiology of depression (50, 51). Numerous studies have examined the concentrations of metabolites in the peripheral kynurenine pathway in patients diagnosed with depression, and found that in comparison to healthy controls, patients with depression had lower levels of peripheral tryptophan, kynurenine, and kynurenine quinolinic acid (52–55), a lower ratio of kynurenine to kynurenine (56), and a higher ratio of kynurenine to tryptophan (57, 58). These findings suggest that major depressive disorder could be caused by an imbalance between neuroprotective and neurotoxic metabolites in the kynurenine pathway (54, 59). Previous study found that individuals with endogenous anxiety had higher plasma kynurenine concentrations than patients with endogenous depression, which suggests that plasma kynurenine can be used as an additional standard to discriminate between the two conditions (60). The severity of depression in individuals with postpartum depression was found to be positively correlated with elevated levels of plasma IL-6 and IL-8, as well as reduced levels of quinolinic acid (61).
Since kynurenine pathway metabolites can penetrate the blood-brain barrier, alterations in metabolite levels can affect central neurotransmitter signaling. A favorable association was seen between the concentration of plasma kynurenine, the 3-HAA content in the right caudate nucleus, and the total choline in the left putamina striatum in an adolescent depressive disorder research (62). In patients with depressive disorder, the ratio of serum kynurenic acid to 3-hydroxykynurenine was reported to be inversely linked with left hippocampus activity during autobiographical memory recall (63). Studies have shown that the ratio of serum kynurequinolinic acid to quinolinic acid in patients with depressive disorder is negatively connected with the severity of depressive symptoms (64, 65), but positively correlated with hippocampus volume (66). Following the administration of interferon-gamma, the concentrations of kynurenine, kynurenquinolinic acid, and quinolinic acid in the cerebral fluid of patients with hepatitis C were elevated, and were substantially linked to depressed symptoms (50).
At present, studies on the kynurenine pathway metabolites in the brain tissue of patients with depressive disorders are very limited. Patients with depressive disorders exhibited a notable reduction in the activation of the kynurenine pathway, as well as decreased levels of quinolinic acid and expression of the IDO1 and IDO2 enzymes in the ventral prefrontal cortex (67). Furthermore, studies have demonstrated that patients with major depressive disorder had higher densities of quinoline-positive microglia in both the anterior and premedia cingulate cortex (68), which further suggests certain regional specificity (69).
3.2 Bipolar disorder
In recent years, the equilibrium of the kynurenine pathway’s neuroprotective and neurotoxic effects has been proposed as one of the potential physiological underpinnings of bipolar disorder. Compared with healthy people, the levels of peripheral tryptophan, kynurenine and kynurenquinolinic acid decreased in BD patients, and the levels of tryptophan decreased the most in individuals with manic episodes (70). Peripheral 3-HK levels were associated with the severity of depressive episodes in patients with bipolar disorder (71), while peripheral kynurequinolinic acid concentrations had a weakly negative correlation with depressed episodes (72). A study conducted on adolescent patients revealed a favorable association between depression symptoms and the kynurenic acid/kynurenine ratio, as well as a negative correlation between depression symptoms with the kynurenine and kynurenine/tryptophan ratio (73). Peripheral kynurenine and kynurenic acid levels rose dramatically following ketamine administration, whereas the quinolinic acid to kynurenine ratio fell (74).
Studies have indicated that in bipolar disorder patients, there is a correlation between the integrity of white matter in multiple brain regions, including the corpus callosum, and the decreased level of peripheral kynurenic acid (75), the increased level of kynurenic acid, and the increased kynurenic acid/tryptophan ratio (76). Decreased levels of tryptophan are associated with depressive symptoms, but increased amounts of kynurenic acid may safeguard the structure of white matter in the brain. Activation of the kynurenine pathway may be cohort-specific in bipolar disorder patients, though, as evidenced by the lack of a statistically significant correlation between various metabolites of the kynurenine pathway in cerebrospinal fluid and depressive symptoms in bipolar disorder patients (77)(Table 3).
4 Creatine/creatine kinase pathway and mood disorders
Creatine kinase serves as the central regulating enzyme in cellular energy metabolism and is extensively present in muscle, brain, renal tubules, and other high-energy-demanding tissues and organs (78). Within the cell, creatine and creatine phosphate undergo reciprocal conversion through the control of creatine kinase, prompting the shuttle of high-energy phosphates at the site of ATP production, and providing support for mitochondrial and cellular energy turnover (79). Creatine, as a substrate for the reaction catalyzed by creatine kinase, is mostly present in muscle tissue and synthesized by the renal-liver axis. In the kidneys, arginine and glycine are synthesized into guanidine acetate, a precursor of creatine, under the action of glycinamide transferase (GATM) (80). After entering the peripheral circulation, guanidine acetate is absorbed by hepatocytes that produce guanidine acetate methyltransferase (GAMT), which then catalyzes the conversion of guanidine acetate to creatine. Following its export from the liver, creatine is taken up by cells that express creatine transporters and transformed by creatine kinases into creatine phosphate (81). Furthermore, creatine can be synthesized in other cell types, including fat, skeletal muscle, and pancreatic acinar cells, as well as absorbed by nutrition (82–84) (Figure 2).
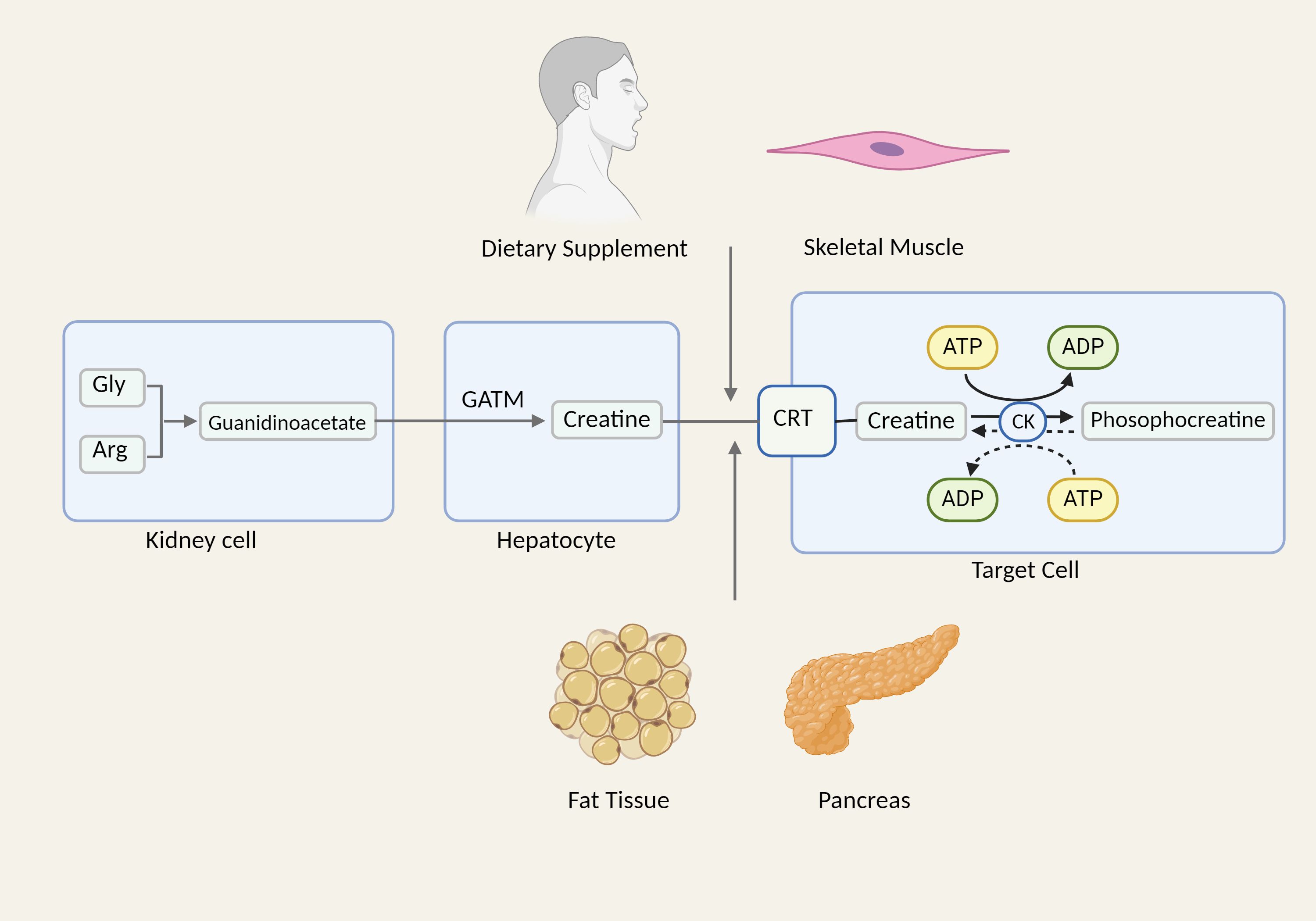
Figure 2. The synthesis and biological effects of creatine. Gly, glycine; Arg, arginine; CRT, creatine transporter.
4.1 Major depressive disorder
Studies have demonstrated a robust association between depression symptoms and fluctuations in levels of creatine and creatine kinase. Research findings indicated a correlation between the concentration of serum total creatine kinase and depressive symptoms in healthy women who are under stress (85). The volume of gray matter in the right medial superior frontal gyrus and the concentration of creatine in the prefrontal cortex were negatively correlated with the severity of depression, whereas the volume of gray matter in the right medial upper frontal cortex and the concentration of creatine in the medial prefrontal cortex were positively correlated (86), suggesting that increasing creatine concentration in the prefrontal cortex may improve the depressive symptoms of patients.
Numerous investigations on the amelioration of depression symptoms through creatine supplementation are already available, which amply supports the use of creatine in animal studies for the treatment of depression symptoms (87–92). One study showed that the administration of creatine helped improve depression-like behavior triggered by long-term corticosterone use (93). Systematic reviews have been conducted with an emphasis on creatine’s application in depression treatment (94). At present, many of the participants in the studies are women (95); In animal experiments, female rats exhibited a more favorable response to dietary creatine supplementation compared to male rats (96, 97), indicating that there might be a sex-dependent relationship between creatine and the treatment of depression symptoms. Studies of animal estrus cycle data revealed that the antidepressant effect of creatine on females mainly occurred in the proestrus and estrus, suggesting that ovarian hormones may have an impact on creatine’s therapeutic effects (96).
Previous review suggested that oligodendrocyte maturation and myelogenesis in the prefrontal cortex (PFC) were extremely responsive to stress experiences, and myelin-related transcriptional genes (MOG, MOBP, PLLP, PLP1, etc.) were significantly down-regulated when symptoms deteriorated. Exposing the mice to unpredictable chronic mild stress resulted in alterations in the transcription of approximately 30-40 genes, including genes linked with oligodendrocytes such as MBP, MOB, and CNP (98). Creatine can directly increase the production of mitochondrial ATP in the culture of oligodendrocyte line and protect caspase-dependent apoptosis of oligodendrocytes during myelin regeneration in the central nervous system (99), while the homozygous loss of GAMT, the rate-limiting enzyme of creatine production, leads to a significant reduction of mature oligodendrocytes in the corpus callosum and delays myelin formation (100). These findings suggested that creatine therapy may be related to the protection of nervous system oligodendrocytes and myelination.
4.2 Bipolar disorder
Current studies mostly have shown that patients with bipolar disorder exhibit notably elevated serum creatine kinase levels than healthy controls during the manic phase (101, 102). Hillbrand et al. investigated the relationship between creatine kinase and manic-like aggressive behavior and found that creatine kinase levels were strongly associated with aggressive behavior in patients treated with antipsychotics (103). This suggests that creatine kinase could serve as a valuable indicator for predicting violent behavior. Additionally, Meltzer et al. found that increased muscle-type creatine kinase levels precede aggression and may persist throughout the course of the disease (104).
In recent years, studies on the structural changes of brain tissue in patients with bipolar disorder have received extensive attention. Research has revealed that individuals with bipolar disorder have significantly reduced amounts of creatine kinase mRNA in hippocampal and prefrontal cortical regions compared to healthy individuals (105, 106). Du et al. used 31P magnetization transfer magnetic resonance spectroscopy to measure the forward response rate constant of creatine kinase. They discovered a noteworthy reduction in the forward response rate constant of creatine kinase in the bipolar disorder group when compared to the healthy control group. Furthermore, they pinpointed the precise molecular mechanism underlying bipolar disorder patients’ incapacity to refuel ATP in the brain during times of high energy demand (107).
A study involving phosphocreatine shuttling in the brain in bipolar disorder showed that in the frontal lobe and corpus callosum, high-energy phosphates are reduced in the form of phosphocreatine (108). Phosphocreatine levels in the frontal and occipital lobes, as well as the overall brain, are lower in adolescents and adults with bipolar disorder (109). This lends further credence to the hypothesis that bipolar disorder is associated with impaired mitochondrial activity or creatine metabolism. Nonetheless, certain research has demonstrated that there is no discernible variation in brain creatine levels between bipolar disorder patients and healthy individuals (110). The degree of physical and sexual abuse throughout childhood was linked to higher levels of creatine phosphate and lower creatine/creatine phosphate ratios in the anterior cingulate cortex in bipolar disorder patients (111).
Previous studies have showed that in addition to promoting and treating muscles, creatine supplementation may also promote brain health, such as cognitive processing, brain function, and repair after injury (112, 113). As a mitochondrial regulator, creatine monohydrate has been utilized as adjuvant therapy for individuals experiencing the depressive phase of bipolar disorder and has been reported to enhance cognitive function (114, 115). However, research has indicated that creatine can improve symptoms in patients with unipolar depression, while its use in bipolar depression may cause patients to transition to a manic phase (116). Bipolar disorder patients have been found to have lower levels of hippocampal myelination (117) and down-regulated brain oligodendrocytes as well as essential myelination-related genes (118) when compared to the control group.
5 Other biogenic amine metabolites and mood disorders
5.1 Taurine
Taurine, a sulfur-containing amino acid, is abundant in skeletal muscle (119–121) and plays a role in critical physiological processes including energy metabolism, lipid and carbohydrate metabolism and anti-inflammatory effects (122–124). As one of the essential amino acids for brain development, taurine has been found to be associated with the occurrence and development of depressive disorders. Previous studies showed that in depressed mice induced by chronic social failure stress, the level of taurine in extracellular fluid of the medial prefrontal cortex of the brain was significantly lessened. Additionally, the depression symptoms of the mice were significantly alleviated following taurine supplementation (125). In addition, taurine can lessen anxiety and enhance spatial memory in depressed rats, achieving the role of preventing depression-like behavior (126). The results suggested that the antidepressant effect of taurine may be related to the regulation of the hypothalamic-pituitary-adrenal (HPA) axis and the promotion of hippocampal neurogenesis, neuron survival and proliferation (126).
5.2 Betaine
Betaine, also known as trimethyl glycine, is mainly distributed in liver, kidney and brain (127). It can regulate human energy metabolism, relieve chronic inflammation (128), and help treat a range of illnesses, including obesity (129), diabetes (130, 131), Alzheimer’s disease (132) and other diseases. Furthermore, the research verified that betaine could ameliorate depression-like behavior in mice and has a neuroprotective effect on depressed mice generated by exposure to zinc oxide nanoparticles (133). Combining betaine with S-adenosine-methionine (SAMe) is more effective than SAMe alone in patients with mild to moderate depressive disorder (134, 135).
5.3 Choline
Choline is the hydrophilic head group of phosphatidylcholine and is involved in several physiological processes: the structural integrity of cell membranes, the transmission of lipid-derived signals and cholinergic neurotransmission and methylation (136). Choline supplementation might provide several beneficial outcomes, including diminishing the likelihood of cardiovascular events and breast cancer, as well as decreasing levels of inflammatory markers (137). In recent years, the connection between choline level and mood disorders has drawn a lot of attention. Dietary choline consumption was found to be inversely associated with the likelihood of experiencing depression symptoms in a cross-sectional study (138). Patients with refractory depressive disorder exhibited significantly elevated levels of choline in both the left and right hippocampus regions of the central nervous system, in comparison to first-episode patients and healthy individuals (139). Furthermore, a notable and favorable connection was discovered between the Hamilton Depression (HAMD) score and the choline/creatine (Cho/Cr) ratio in both the bilateral hippocampus areas and the left thalamus of patients with poststroke depression (140). After treatment, a significant decrease was detected in the Cho/Cr ratio of both the left thalamus and the bilateral hippocampal regions, raising the possibility that the aberrant choline metabolism in these regions is connected to post-stroke depression.
Manic individuals with greater average levels of choline in red blood cells are more likely to be unwell, have a worse prognosis, and a limited response to lithium treatment (141). Patients who do not respond to lithium demonstrate higher amounts of choline in the dorsolateral prefrontal lobe in comparison to patients who do respond positively to lithium (142). In addition, in contrast to controls, bipolar disorder patients had a considerably greater Cho/Cr-PCr ratio in the right cingulate cortex (143). The choline/creatine ratio in anterior cingulate cortex in adolescents with depressive disorder is significantly higher than that in patients with bipolar disorder (144), indicating that the total choline in anterior cingulate cortex may serve as a biomarker for the diagnosis of mood disorders in adolescents.
6 Associations between biogenic amine metabolites
The relationship between different biogenic amine metabolites remains uncertain. Taurine and amino acid metabolites such as creatine and histamine may work together through the same solute carrier superfamily. The SLC6 family, consisting of taurine and creatine transporters, is expressed in an array of tissues and is capable of actively transporting substrates on biofilms via gradients in sodium ion concentration (145). According to the study of Kurtz, J. A. et al., taurine supplementation can promote muscle regeneration and increase strength in male athletes (146), suggesting that exogenous taurine supplementation may affect creatine metabolism. Moreover, taurine and creatine have been found to present a time - and space-dependent imbalance in the brains of Alzheimer’s disease rats (147). Taurine supplementation throughout gestation can increase the N-acetyl aspartic acid (NAA)/creatine (Cr) ratio related to the number of neurons. It can also decrease the ratio of choline (Cho) to creatine (Cr), which is related to the number of glial cells. This improvement in hippocampal neuronal metabolism suggests that the balance between taurine and creatine may be linked to cognitive function (148). A previous study found that gut microbiota derived metabolites taurine, histamine, and spermine maintain the balance between host and gut microbiota by co-regulating NLRP6 inflammasome signaling, epithelial IL-18 secretion, and downstream antimicrobial peptide (AMP) profiles (149).
Choline, which is an organic penetrant and methyl donor in carbon metabolism (127), is converted to betaine, an oxidized byproduct. Betaine is a crucial neurotransmitter in a number of physiological functions, including memory retention and muscular control (150). A study in obese patients showed that choline and betaine supplements specifically raised histidine levels while simultaneously lowering taurine, acetic acid, and beta-hydroxybutyric acid levels, suggesting that choline and betaine supplements may have a beneficial effect on the citric acid cycle and can assist in the treatment of obesity-related diseases (151).
7 Other biological mechanisms of mood disorders interacting with biogenic amine metabolites
7.1 Inflammation
It has been discovered that inflammation is linked to stress-related mental diseases, such as major depressive disorder and bipolar disorder (152). Previous studies have shown that proinflammatory cytokines and acute phase proteins in blood were increased in depressive patients compared to healthy controls (153), and antidepressant treatment can considerably decrease the levels of IL-6, TNF, IL-10 and CCL2 in peripheral blood (154, 155). Furthermore, bipolar disorder patients also showed elevated serum levels of IL-2, IL-4, and IL-6 (156).
Inflammatory factors can act on the kynurenine pathway, thus affecting the occurrence of depression. Excessive activation of the kynurenine pathway results in disruptions in the levels of neurotoxic/neuroprotective compounds, particularly 3-HK/QA/Kyna. Studies have shown that patients treated with IFN-alpha have reduced serum tryptophan levels, increased Kyn levels and Kyn/Trp ratio activity, and increased depressive symptoms (157). Besides, antidepressants with anti-inflammatory properties suppress the activation of IDO by reducing levels of pro-inflammatory cytokines in immune-activated individuals, thereby aiding in the alleviation of depressive symptoms (158). The application of saffron extract can reduce depression-like behavior in mice through modulating the expression of KYN-related neurotoxicity (159).
Studies have shown that acute inflammation induced by lipopolysaccharides may lead to increased histamine and decreased serotonin in the central nervous system, leading to depression-like behaviors (160, 161). In addition, the utilization of creatine supplements has been shown to effectively alleviate many inflammation-related conditions, such as cancer (162) and osteoarthritis (163). Dietary creatine supplementation can delay the emergence of tumors in mice by regulating CD8+ T cell immunity (164). Zhang et al. demonstrated that betaine could facilitate the conversion of microglia from the M1 phenotype to the M2 phenotype by suppressing the activation of NLRP3 inflammasome, thus reducing the depressed-like behavior induced by lipopolysaccharide in mice (165).
7.2 Gut microbiota
A growing number of studies has demonstrated the link between mood disorders and alterations in the gut microbiome. It was widely acknowledged that proinflammatory bacteria were enriched and anti-inflammatory bacteria decreased in the gut of depressive patients, further strengthening the inflammatory hypothesis of depression (166). Metabolites derived from gut microbes are also strongly connected to mood disorders (167–170).
Increasing evidence suggests that kynurenine and its downstream metabolites, occurring in the gut, play an important role in regulating immune cell function, intestinal inflammation, and altering the production and inhibition of inflammatory cytokines (171, 172). Rats that received fecal microbiota transplants from depressive patients showed more pronounced depressive symptoms, associated with higher circulating kynurenine and kynurenine/tryptophan ratios (173). Probiotics, including L. helveticus R0052 and B. longum R0175, have been shown to have anxiety-reducing benefits in rats and positive psychological effects in healthy human volunteers (174).
Symbiotic microbes in the gut can produce histamine and related compounds under physiological conditions, suggesting that intestinal histamine might play a regulatory role in maintaining intestinal barrier function (149, 175, 176). Diets rich in polyunsaturated fatty acids, short-chain fatty acid and vitamin A have a protective effect against chronic stress in mice, while histamine deficiency can block this effect (177, 178).
The gut microbiome converts choline into several compounds, including trimethyl glycine (betaine) and trimethylamine (179). In addition, choline can undergo metabolism to produce hepatotoxic methylamines, such as dimethylamine, trimethylamine (TMA), and trimethylamine N-oxide (TMAO) (180). A high-fat diet alters the way choline is processed, leading to an increase in methylamine production (181). Furthermore, patients with major depressive disorder had higher levels of TMAO compared to healthy individuals, indicating that gut microorganisms can influence choline metabolism and are suspected of depressive symptoms in patients (182).
Multiple studies have shown dysregulation of intestinal taurine metabolism is significantly associated with anxiety and depression symptoms (183), and remedies using medication or modifications to the gut microbiota can ameliorate these symptoms by enhancing taurine metabolism (184, 185).
7.3 Brain-derived neurotrophic factor
Brain-derived neurotrophic factor (BDNF) binds to the tyrosine kinase receptor B (TrkB) receptor, which is directly involved in neurite growth, neuroplasticity and phenotypic maturation, and is crucial for the development and plasticity of synapses (186). The change of BDNF expression is closely related to normal and pathological aging (187), and has been verified to have a close correlation with mood disorders (188).
It is reported that the histamine H3R antagonist thioperamide hinders microglia activity and inflammatory response, and improves neurogenic injury and cognitive dysfunction by enhancing histamine release (189, 190). Besides, H3R antagonist s38093 was found to reverse the age-related effects of BDNF in the hippocampus of aging mice and increased hippocampal neurogenesis in adults, providing an innovative strategy to address cognitive deficits (191).
The mixture of creatine and taurine can trigger a notable rise in the expression of BDNF in stressed rats, and its antidepressant effect is related to the increase of behavior pattern mediated by extracellular regulated protein kinases (ERK)/BDNF pathway and mTORC1 signal pathway (192, 193). The presence of a specific genetic variation (BDNF rs6265) in the BDNF gene has a notable two-way interaction with creatine in bipolar disorder patients, suggesting that the pathogenesis of bipolar disorder may be related to the metabolism of the anterior cingulate cortex (194).
Taurine restores cognitive function and memory in rats exposed to toxic chemicals by stimulating N-methyl-D-aspartate (NMDA) receptors and elevating the expression of BDNF (195, 196), and counteracts the negative effects of cAMP-PKA-CREB signaling pathway on behavior and cognitive function of rats (197). Furthermore, taurine efficiently suppresses oxidative stress, neuroinflammation, and apoptosis in stressed rats by restoring BDNF expression and blocking the NF-κB signaling pathway (198).
7.4 Circadian rhythm
The disruption of rhythm is firmly connected with mood, sleep and physiological activities, and is one of the core symptoms of mood disorders, indicating that circadian rhythm disturbance is an important factor in the pathogenesis of mood disorders (199). The circadian system helps regulate a variety of vital functions, such as memory formation and maintenance, emotional regulation, cognitive function, and neuroplasticity (199, 200), via controlling the production of the CLOCK protein. Clock protein resides in various brain regions such as the hippocampus and amygdala (201), and can regulate the monoaminergic system in each region, thus regulating emotion-related behaviors (202, 203).
Tryptophan and kynurenine, as substrates for melatonin generation, could affect the synthesis of melatonin and the circadian rhythm, thereby impacting cognition and mood (204). In bipolar disorder, overactivation of the kynurenine pathway result in reduced tryptophan-produced melatonin, which leads to emotional symptoms and disruption of circadian rhythms (205). In addition, the kynurenine pathway and melatonin production may also be related to BDNF production, suggesting that the emergence of bipolar disorder may include a more intricate process.
Melatonin can interact with histamine signaling pathways and ultimately affect circadian rhythms (206). Previous studies have shown that histamine is associated with behavior and sleep-wake control (207), and is considered to be a downstream target of circadian action (208). Organic cation transporter 3 (OCT3) facilitates the transmission of histamine in mast cells and is linked to the regulation of the CLOCK gene, indicating that OCT3 plays a role in controlling the body’s internal clock (209). Changes in circadian rhythms can lead to modifications in the synthesis and transport of histamine, hence causing alterations in mood and behaviors.
8 Conclusion
Studies have shown that compared with healthy people, patients with mood disorders present notable variations in the biogenic amine metabolites in the peripheral and central nervous system. Future investigations should concentrate on the molecular pathway that links biogenic amine metabolites to mood disorders.
The two branches of kynurenine metabolism in the brain exhibit neuroprotective and neurotoxic effects, respectively, and the underlying mechanism of disease may be the equilibrium between the two branches, rather than the absolute levels of their metabolites. The disruption of circadian rhythm and melatonin synthesis may be attributed to the imbalance between the neurotoxic and neuroprotective pathways of kynurenine. Anti-inflammatory antidepressants may help to improve this imbalance.
Creatine, as a mitochondrial regulator, has been used to treat depressive disorders and bipolar depression, but there is a risk of transforming bipolar depression into bipolar mania. Previous studies have shown that patients with bipolar disorder and depressive disorder exhibit a decrease in the expression of genes associated with the development of oligodendrocytes and myelin. Additionally, creatine supplementation has been shown to protect oligodendrocytes and enhance myelin formation, raising the possibility that mood disorders may be caused by the creatine-related oligodendrocyte myelin pathway. The study on the mechanism of creatine as an adjunct therapy for depression can not only offer a fresh perspective on the treatment of depression, but also serve as a guide for managing depressive phase of bipolar disorder. In addition, peripheral creatine kinase levels also differed in patients with unipolar depression and bipolar disorder. Exploring the pathogenesis of peripheral creatine and creatine kinase pathway in mood disorders is helpful to identify new targets for the treatment of mood disorders.
Furthermore, biogenic amine metabolites are generated in peripheral tissues, such as liver and kidney, and it has been established that biogenic amine metabolites interact peripherally with the molecular processes of mood disorders, including gut microbiota and inflammation. In the future, studying the connections between peripheral signaling molecules and different brain regions—such as blood-brain barrier crossing and hormone axis changes—may be crucial to understanding the molecular foundations of mood disorders.
Author contributions
JY: Investigation, Visualization, Writing – original draft. MY: Funding acquisition, Writing – review & editing. WZ: Funding acquisition, Project administration, Supervision, Writing – review & editing.
Funding
The author(s) declare financial support was received for the research, authorship, and/or publication of this article. This work was supported by 1·3·5 Project for Disciplines of excellence, West China Hospital, Sichuan University (Grant number ZYJC21004) and Department of Science and Technology of Sichuan Province (Grant numbers 2023YFS0417).
Acknowledgments
The authors wish to express gratitude for the drawing materials provided by BioRender.
Conflict of interest
The authors declare that the research was conducted in the absence of any commercial or financial relationships that could be construed as a potential conflict of interest.
Publisher’s note
All claims expressed in this article are solely those of the authors and do not necessarily represent those of their affiliated organizations, or those of the publisher, the editors and the reviewers. Any product that may be evaluated in this article, or claim that may be made by its manufacturer, is not guaranteed or endorsed by the publisher.
References
1. Wilson JE, Blizzard L, Gall SL, Magnussen CG, Oddy WH, Dwyer T, et al. Youth diet quality and hazard of mood disorder in adolescence and adulthood among an Australian cohort. J Affect Disord. (2020) 276:511–8. doi: 10.1016/j.jad.2020.07.048
2. Simanek AM, Parry A, Dowd JB. Differences in the association between persistent pathogens and mood disorders among young- to middle-aged women and men in the U.S. Brain Behav Immun. (2018) 68:56–65. doi: 10.1016/j.bbi.2017.09.017
3. Kessler RC, Berglund P, Demler O, Jin R, Koretz D, Merikangas KR, et al. The epidemiology of major depressive disorder: results from the National Comorbidity Survey Replication (NCS-R). Jama. (2003) 289:3095–105. doi: 10.1001/jama.289.23.3095
4. Carvalho AF, Firth J, Vieta E. Bipolar disorder. N Engl J Med. (2020) 383:58–66. doi: 10.1056/NEJMra1906193
5. Coleman JRI, Gaspar HA, Bryois J, Breen G. The genetics of the mood disorder spectrum: genome-wide association analyses of more than 185,000 cases and 439,000 controls. Biol Psychiatry. (2020) 88:169–84. doi: 10.1016/j.biopsych.2019.10.015
6. Taylor CL. Creativity and mood disorder: A systematic review and meta-analysis. Perspect Psychol Sci. (2017) 12:1040–76. doi: 10.1177/1745691617699653
7. Otte C, Gold SM, Penninx BW, Pariante CM, Etkin A, Fava M, et al. Major depressive disorder. Nat Rev Dis Primers. (2016) 2:16065. doi: 10.1038/nrdp.2016.65
8. Miller JN, Black DW. Bipolar disorder and suicide: a review. Curr Psychiatry Rep. (2020) 22:6. doi: 10.1007/s11920-020-1130-0
9. Cui L, Li S, Wang S, Wu X, Liu Y, Yu W, et al. Major depressive disorder: hypothesis, mechanism, prevention and treatment. Signal Transduct Target Ther. (2024) 9:30. doi: 10.1038/s41392-024-01738-y
10. Yuan M, Yang B, Rothschild G, Mann JJ, Sanford LD, Tang X, et al. Epigenetic regulation in major depression and other stress-related disorders: molecular mechanisms, clinical relevance and therapeutic potential. Signal Transduct Target Ther. (2023) 8:309. doi: 10.1038/s41392-023-01519-z
11. Rantala MJ, Luoto S, Borráz-León JI, Krams I. Bipolar disorder: An evolutionary psychoneuroimmunological approach. Neurosci Biobehav Rev. (2021) 122:28–37. doi: 10.1016/j.neubiorev.2020.12.031
12. Zhu T, Liu X, Wang J, Kou R, Hu Y, Yuan M, et al. Explainable machine-learning algorithms to differentiate bipolar disorder from major depressive disorder using self-reported symptoms, vital signs, and blood-based markers. Comput Methods Programs BioMed. (2023) 240:107723. doi: 10.1016/j.cmpb.2023.107723
13. Zhang T, Guo L, Li R, Wang F, Yang WM, Yang JB, et al. Alterations of plasma lipids in adult women with major depressive disorder and bipolar depression. Front Psychiatry. (2022) 13:927817. doi: 10.3389/fpsyt.2022.927817
14. Yu M, Jia H, Zhou C, Yang Y, Zhao Y, Yang M, et al. Variations in gut microbiota and fecal metabolic phenotype associated with depression by 16S rRNA gene sequencing and LC/MS-based metabolomics. J Pharm BioMed Anal. (2017) 138:231–9. doi: 10.1016/j.jpba.2017.02.008
15. Baker SA, Rutter J. Metabolites as signalling molecules. Nat Rev Mol Cell Biol. (2023) 24:355–74. doi: 10.1038/s41580-022-00572-w
16. Frederick AL, Stanwood GD. Drugs, biogenic amine targets and the developing brain. Dev Neurosci. (2009) 31:7–22. doi: 10.1159/000207490
17. Zhang Z, Peng Y, Zheng J. Amino acid and biogenic amine adductions derived from reactive metabolites. Curr Drug Metab. (2021) 22:1076–86. doi: 10.2174/1389200223666211216143617
18. Schranner D, Kastenmüller G, Schönfelder M, Römisch-Margl W, Wackerhage H. Metabolite concentration changes in humans after a bout of exercise: a systematic review of exercise metabolomics studies. Sports Med Open. (2020) 6:11. doi: 10.1186/s40798-020-0238-4
19. Sun XL, Ma LN, Chen ZZ, Xiong YB, Jia J, Wang Y, et al. Search for serum biomarkers in patients with bipolar disorder and major depressive disorder using metabolome analysis. Front Psychiatry. (2023) 14:1251955. doi: 10.3389/fpsyt.2023.1251955
20. Li H, Xiao Y, Li Q, Yao J, Yuan X, Zhang Y, et al. The allergy mediator histamine confers resistance to immunotherapy in cancer patients via activation of the macrophage histamine receptor H1. Cancer Cell. (2022) 40:36–52.e9. doi: 10.1016/j.ccell.2021.11.002
21. Haas HL, Sergeeva OA, Selbach O. Histamine in the nervous system. Physiol Rev. (2008) 88:1183–241. doi: 10.1152/physrev.00043.2007
22. Jin CY, Anichtchik O, Panula P. Altered histamine H3 receptor radioligand binding in post-mortem brain samples from subjects with psychiatric diseases. Br J Pharmacol. (2009) 157:118–29. doi: 10.1111/j.1476-5381.2009.00149.x
23. Haas H, Panula P. The role of histamine and the tuberomamillary nucleus in the nervous system. Nat Rev Neurosci. (2003) 4:121–30. doi: 10.1038/nrn1034
24. Tao R, Fu Z, Xiao L. Chronic food antigen-specific igG-mediated hypersensitivity reaction as A risk factor for adolescent depressive disorder. Genomics Proteomics Bioinf. (2019) 17:183–9. doi: 10.1016/j.gpb.2019.05.002
25. Kano M, Fukudo S, Tashiro A, Utsumi A, Tamura D, Itoh M, et al. Decreased histamine H1 receptor binding in the brain of depressed patients. Eur J Neurosci. (2004) 20:803–10. doi: 10.1111/j.1460-9568.2004.03540.x
26. Ionov ID, Pushinskaya II, Gorev NP, Shpilevaya LA, Frenkel DD, Severtsev NN. Histamine H(1) receptors regulate anhedonic-like behavior in rats: Involvement of the anterior cingulate and lateral entorhinal cortices. Behav Brain Res. (2021) 412:113445. doi: 10.1016/j.bbr.2021.113445
27. Iida T, Yoshikawa T, Kárpáti A, Matsuzawa T, Kitano H, Mogi A, et al. JNJ10181457, a histamine H3 receptor inverse agonist, regulates in vivo microglial functions and improves depression-like behaviours in mice. Biochem Biophys Res Commun. (2017) 488:534–40. doi: 10.1016/j.bbrc.2017.05.081
28. Bahi A, Schwed JS, Walter M, Stark H, Sadek B. Anxiolytic and antidepressant-like activities of the novel and potent non-imidazole histamine H₃ receptor antagonist ST-1283. Drug Des Devel Ther. (2014) 8:627–37. doi: 10.2147/dddt.S63088
29. Femenía T, Magara S, DuPont CM, Lindskog M. Hippocampal-dependent antidepressant action of the H3 receptor antagonist clobenpropit in a rat model of depression. Int J Neuropsychopharmacol. (2015) 18:pyv032. doi: 10.1093/ijnp/pyv032
30. Kumar A, Dogra S, Sona C, Umrao D, Rashid M, Singh SK, et al. Chronic histamine 3 receptor antagonism alleviates depression like conditions in mice via modulation of brain-derived neurotrophic factor and hypothalamus-pituitary adrenal axis. Psychoneuroendocrinology. (2019) 101:128–37. doi: 10.1016/j.psyneuen.2018.11.007
31. Sanna MD, Ghelardini C, Thurmond RL, Masini E, Galeotti N. Behavioural phenotype of histamine H(4) receptor knockout mice: Focus on central neuronal functions. Neuropharmacology. (2017) 114:48–57. doi: 10.1016/j.neuropharm.2016.11.023
32. Yamada Y, Yoshikawa T, Naganuma F, Kikkawa T, Osumi N, Yanai K. Chronic brain histamine depletion in adult mice induced depression-like behaviours and impaired sleep-wake cycle. Neuropharmacology. (2020) 175:108179. doi: 10.1016/j.neuropharm.2020.108179
33. Munari L, Provensi G, Passani MB, Galeotti N, Cassano T, Benetti F, et al. Brain histamine is crucial for selective serotonin reuptake inhibitors' Behavioral and neurochemical effects. Int J Neuropsychopharmacol. (2015) 18:pyv045. doi: 10.1093/ijnp/pyv045
34. Chikahisa S, Kodama T, Soya A, Sagawa Y, Ishimaru Y, Séi H, et al. Histamine from brain resident MAST cells promotes wakefulness and modulates behavioral states. PLoS One. (2013) 8:e78434. doi: 10.1371/journal.pone.0078434
35. Ito C. The role of brain histamine in acute and chronic stresses. BioMed Pharmacother. (2000) 54:263–7. doi: 10.1016/s0753-3322(00)80069-4
36. Ito C, Shen H, Toyota H, Kubota Y, Sakurai E, Watanabe T, et al. Effects of the acute and chronic restraint stresses on the central histaminergic neuron system of Fischer rat. Neurosci Lett. (1999) 262:143–5. doi: 10.1016/s0304-3940(99)00052-x
37. Noguchi S, Fukuda Y, Inukai T. Possible contributory role of the central histaminergic system in the forced swimming model. Arzneimittelforschung. (1992) 42:611–3.
38. Imaizumi M, Onodera K. The behavioral and biochemical effects of thioperamide, a histamine H3-receptor antagonist, in a light/dark test measuring anxiety in mice. Life Sci. (1993) 53:1675–83. doi: 10.1016/0024-3205(93)90204-g
39. Zarrindast MR, Valizadegan F, Rostami P, Rezayof A. Histaminergic system of the lateral septum in the modulation of anxiety-like behaviour in rats. Eur J Pharmacol. (2008) 583:108–14. doi: 10.1016/j.ejphar.2008.01.003
40. Hajizadeh Moghaddam A, Roohbakhsh A, Rostami P, Heidary-Davishani A, Zarrindast MR. GABA and histamine interaction in the basolateral amygdala of rats in the plus-maze test of anxiety-like behaviors. Pharmacology. (2008) 82:59–66. doi: 10.1159/000131110
41. Shan L, Bao AM, Swaab DF. The human histaminergic system in neuropsychiatric disorders. Trends Neurosci. (2015) 38:167–77. doi: 10.1016/j.tins.2014.12.008
42. Schwarcz R, Bruno JP, Muchowski PJ, Wu HQ. Kynurenines in the mammalian brain: when physiology meets pathology. Nat Rev Neurosci. (2012) 13:465–77. doi: 10.1038/nrn3257
43. Brown SJ, Huang XF, Newell KA. The kynurenine pathway in major depression: What we know and where to next. Neurosci Biobehav Rev. (2021) 127:917–27. doi: 10.1016/j.neubiorev.2021.05.018
44. Yamazaki F, Kuroiwa T, Takikawa O, Kido R. Human indolylamine 2,3-dioxygenase. Its tissue distribution, and characterization of the placental enzyme. Biochem J. (1985) 230:635–8. doi: 10.1042/bj2300635
45. Meininger D, Zalameda L, Liu Y, Stepan LP, Borges L, McCarter JD, et al. Purification and kinetic characterization of human indoleamine 2,3-dioxygenases 1 and 2 (IDO1 and IDO2) and discovery of selective IDO1 inhibitors. Biochim Biophys Acta. (2011) 1814:1947–54. doi: 10.1016/j.bbapap.2011.07.023
46. Li JS, Han Q, Fang J, Rizzi M, James AA, Li J. Biochemical mechanisms leading to tryptophan 2,3-dioxygenase activation. Arch Insect Biochem Physiol. (2007) 64:74–87. doi: 10.1002/arch.20159
47. Gál EM, Sherman AD. L-kynurenine: its synthesis and possible regulatory function in brain. Neurochem Res. (1980) 5:223–39. doi: 10.1007/bf00964611
48. Perkins MN, Stone TW. An iontophoretic investigation of the actions of convulsant kynurenines and their interaction with the endogenous excitant quinolinic acid. Brain Res. (1982) 247:184–7. doi: 10.1016/0006-8993(82)91048-4
49. Han Q, Cai T, Tagle DA, Li J. Structure, expression, and function of kynurenine aminotransferases in human and rodent brains. Cell Mol Life Sci. (2010) 67:353–68. doi: 10.1007/s00018-009-0166-4
50. Raison CL, Dantzer R, Kelley KW, Lawson MA, Woolwine BJ, Vogt G, et al. CSF concentrations of brain tryptophan and kynurenines during immune stimulation with IFN-alpha: relationship to CNS immune responses and depression. Mol Psychiatry. (2010) 15:393–403. doi: 10.1038/mp.2009.116
51. Wichers MC, Koek GH, Robaeys G, Verkerk R, Scharpé S, Maes M. IDO and interferon-alpha-induced depressive symptoms: a shift in hypothesis from tryptophan depletion to neurotoxicity. Mol Psychiatry. (2005) 10:538–44. doi: 10.1038/sj.mp.4001600
52. Arnone D, Saraykar S, Salem H, Teixeira AL, Dantzer R, Selvaraj S. Role of Kynurenine pathway and its metabolites in mood disorders: A systematic review and meta-analysis of clinical studies. Neurosci Biobehav Rev. (2018) 92:477–85. doi: 10.1016/j.neubiorev.2018.05.031
53. Ogyu K, Kubo K, Noda Y, Iwata Y, Tsugawa S, Omura Y, et al. Kynurenine pathway in depression: A systematic review and meta-analysis. Neurosci Biobehav Rev. (2018) 90:16–25. doi: 10.1016/j.neubiorev.2018.03.023
54. Marx W, McGuinness AJ, Rocks T, Ruusunen A, Cleminson J, Walker AJ, et al. The kynurenine pathway in major depressive disorder, bipolar disorder, and schizophrenia: a meta-analysis of 101 studies. Mol Psychiatry. (2021) 26:4158–78. doi: 10.1038/s41380-020-00951-9
55. Colle R, Masson P, Verstuyft C, Fève B, Werner E, Boursier-Neyret C, et al. Peripheral tryptophan, serotonin, kynurenine, and their metabolites in major depression: A case-control study. Psychiatry Clin Neurosci. (2020) 74:112–7. doi: 10.1111/pcn.12944
56. Wu Y, Zhong X, Mai N, Wen Y, Shang D, Hu L, et al. Kynurenine pathway changes in late-life depression. J Affect Disord. (2018) 235:76–81. doi: 10.1016/j.jad.2018.04.007
57. Gabbay V, Klein RG, Katz Y, Mendoza S, Guttman LE, Alonso CM, et al. The possible role of the kynurenine pathway in adolescent depression with melancholic features. J Child Psychol Psychiatry. (2010) 51:935–43. doi: 10.1111/j.1469-7610.2010.02245.x
58. Messaoud A, Rym M, Wahiba D, Neffati F, Najjar MF, Gobbi G, et al. Investigation of the relationship among cortisol, pro-inflammatory cytokines, and the degradation of tryptophan into kynurenine in patients with major depression and suicidal behavior. Curr Top Med Chem. (2021) 22(25):2119–25. doi: 10.2174/1568026621666210909160210
59. Myint AM, Kim YK, Verkerk R, Scharpé S, Steinbusch H, Leonard B. Kynurenine pathway in major depression: evidence of impaired neuroprotection. J Affect Disord. (2007) 98:143–51. doi: 10.1016/j.jad.2006.07.013
60. Orlikov AB, Prakhye IB, Ryzov IV. Kynurenine in blood plasma and DST in patients with endogenous anxiety and endogenous depression. Biol Psychiatry. (1994) 36:97–102. doi: 10.1016/0006-3223(94)91189-4
61. Achtyes E, Keaton SA, Smart L, Burmeister AR, Heilman PL, Krzyzanowski S, et al. Inflammation and kynurenine pathway dysregulation in post-partum women with severe and suicidal depression. Brain Behav Immun. (2020) 83:239–47. doi: 10.1016/j.bbi.2019.10.017
62. Gabbay V, Liebes L, Katz Y, Liu S, Mendoza S, Babb JS, et al. The kynurenine pathway in adolescent depression: preliminary findings from a proton MR spectroscopy study. Prog Neuropsychopharmacol Biol Psychiatry. (2010) 34:37–44. doi: 10.1016/j.pnpbp.2009.09.015
63. Young KD, Drevets WC, Dantzer R, Teague TK, Bodurka J, Savitz J. Kynurenine pathway metabolites are associated with hippocampal activity during autobiographical memory recall in patients with depression. Brain Behav Immun. (2016) 56:335–42. doi: 10.1016/j.bbi.2016.04.007
64. Schwieler L, Samuelsson M, Frye MA, Bhat M, Schuppe-Koistinen I, Jungholm O, et al. Electroconvulsive therapy suppresses the neurotoxic branch of the kynurenine pathway in treatment-resistant depressed patients. J Neuroinflamm. (2016) 13:51. doi: 10.1186/s12974-016-0517-7
65. Meier TB, Drevets WC, Wurfel BE, Ford BN, Morris HM, Victor TA, et al. Relationship between neurotoxic kynurenine metabolites and reductions in right medial prefrontal cortical thickness in major depressive disorder. Brain Behav Immun. (2016) 53:39–48. doi: 10.1016/j.bbi.2015.11.003
66. Savitz J, Drevets WC, Smith CM, Victor TA, Wurfel BE, Bellgowan PS, et al. Putative neuroprotective and neurotoxic kynurenine pathway metabolites are associated with hippocampal and amygdalar volumes in subjects with major depressive disorder. Neuropsychopharmacology. (2015) 40:463–71. doi: 10.1038/npp.2014.194
67. Clark SM, Pocivavsek A, Nicholson JD, Notarangelo FM, Langenberg P, McMahon RP, et al. Reduced kynurenine pathway metabolism and cytokine expression in the prefrontal cortex of depressed individuals. J Psychiatry Neurosci. (2016) 41:386–94. doi: 10.1503/jpn.150226
68. Steiner J, Walter M, Gos T, Guillemin GJ, Bernstein HG, Sarnyai Z, et al. Severe depression is associated with increased microglial quinolinic acid in subregions of the anterior cingulate gyrus: evidence for an immune-modulated glutamatergic neurotransmission? J Neuroinflamm. (2011) 8:94. doi: 10.1186/1742-2094-8-94
69. Busse M, Busse S, Myint AM, Gos T, Dobrowolny H, Müller UJ, et al. Decreased quinolinic acid in the hippocampus of depressive patients: evidence for local anti-inflammatory and neuroprotective responses? Eur Arch Psychiatry Clin Neurosci. (2015) 265:321–9. doi: 10.1007/s00406-014-0562-0
70. Bartoli F, Misiak B, Callovini T, Cavaleri D, Cioni RM, Crocamo C, et al. The kynurenine pathway in bipolar disorder: a meta-analysis on the peripheral blood levels of tryptophan and related metabolites. Mol Psychiatry. (2021) 26:3419–29. doi: 10.1038/s41380-020-00913-1
71. Savitz J, Dantzer R, Wurfel BE, Victor TA, Ford BN, Bodurka J, et al. Neuroprotective kynurenine metabolite indices are abnormally reduced and positively associated with hippocampal and amygdalar volume in bipolar disorder. Psychoneuroendocrinology. (2015) 52:200–11. doi: 10.1016/j.psyneuen.2014.11.015
72. van den Ameele S, van Nuijs AL, Lai FY, Schuermans J, Verkerk R, van Diermen L, et al. A mood state-specific interaction between kynurenine metabolism and inflammation is present in bipolar disorder. Bipolar Disord. (2020) 22:59–69. doi: 10.1111/bdi.12814
73. Benevenuto D, Saxena K, Fries GR, Valvassori SS, Kahlon R, Saxena J, et al. Alterations in plasma kynurenine pathway metabolites in children and adolescents with bipolar disorder and unaffected offspring of bipolar parents: A preliminary study. Bipolar Disord. (2021) 23:689–96. doi: 10.1111/bdi.13027
74. Kadriu B, Farmer CA, Yuan P, Park LT, Deng ZD, Moaddel R, et al. The kynurenine pathway and bipolar disorder: intersection of the monoaminergic and glutamatergic systems and immune response. Mol Psychiatry. (2021) 26:4085–95. doi: 10.1038/s41380-019-0589-8
75. Poletti S, Myint AM, Schüetze G, Bollettini I, Mazza E, Grillitsch D, et al. Kynurenine pathway and white matter microstructure in bipolar disorder. Eur Arch Psychiatry Clin Neurosci. (2018) 268:157–68. doi: 10.1007/s00406-016-0731-4
76. Comai S, Melloni E, Lorenzi C, Bollettini I, Vai B, Zanardi R, et al. Selective association of cytokine levels and kynurenine/tryptophan ratio with alterations in white matter microstructure in bipolar but not in unipolar depression. Eur Neuropsychopharmacol. (2022) 55:96–109. doi: 10.1016/j.euroneuro.2021.11.003
77. Trepci A, Sellgren CM, Pålsson E, Brundin L, Khanlarkhani N, Schwieler L, et al. Central levels of tryptophan metabolites in subjects with bipolar disorder. Eur Neuropsychopharmacol. (2021) 43:52–62. doi: 10.1016/j.euroneuro.2020.11.018
78. Brewster LM, Clark JF, van Montfrans GA. Is greater tissue activity of creatine kinase the genetic factor increasing hypertension risk in black people of sub-Saharan African descent? J Hypertens. (2000) 18:1537–44. doi: 10.1097/00004872-200018110-00002
79. Ellington WR. Phosphocreatine represents a thermodynamic and functional improvement over other muscle phosphagens. J Exp Biol. (1989) 143:177–94. doi: 10.1242/jeb.143.1.177
80. Wyss M, Kaddurah-Daouk R. Creatine and creatinine metabolism. Physiol Rev. (2000) 80:1107–213. doi: 10.1152/physrev.2000.80.3.1107
81. Kazak L, Cohen P. Creatine metabolism: energy homeostasis, immunity and cancer biology. Nat Rev Endocrinol. (2020) 16:421–36. doi: 10.1038/s41574-020-0365-5
82. Russell AP, Ghobrial L, Wright CR, Lamon S, Brown EL, Kon M, et al. Creatine transporter (SLC6A8) knockout mice display an increased capacity for in vitro creatine biosynthesis in skeletal muscle. Front Physiol. (2014) 5:314. doi: 10.3389/fphys.2014.00314
83. da Silva RP, Clow K, Brosnan JT, Brosnan ME. Synthesis of guanidinoacetate and creatine from amino acids by rat pancreas. Br J Nutr. (2014) 111:571–7. doi: 10.1017/s0007114513003012
84. Kazak L, Chouchani ET, Lu GZ, Jedrychowski MP, Bare CJ, Mina AI, et al. Genetic depletion of adipocyte creatine metabolism inhibits diet-induced thermogenesis and drives obesity. Cell Metab. (2017) 26:660–671.e3. doi: 10.1016/j.cmet.2017.08.009
85. Kato A, Sakakibara H, Tsuboi H, Tatsumi A, Akimoto M, Shimoi K, et al. Depressive symptoms of female nursing staff working in stressful environments and their association with serum creatine kinase and lactate dehydrogenase - a preliminary study. Biopsychosoc Med. (2014) 8:21. doi: 10.1186/1751-0759-8-21
86. Faulkner P, Paioni SL, Kozhuharova P, Orlov N, Lythgoe DJ, Daniju Y, et al. Relationship between depression, prefrontal creatine and grey matter volume. J Psychopharmacol. (2021) 35:1464–72. doi: 10.1177/02698811211050550
87. Okwuofu EO, Ogundepo GE, Akhigbemen AM, Abiola AL, Ozolua RI, Igbe I, et al. Creatine attenuates seizure severity, anxiety and depressive-like behaviors in pentylenetetrazole kindled mice. Metab Brain Dis. (2021) 36:571–9. doi: 10.1007/s11011-021-00684-w
88. Kanekar S, Ettaro R, Hoffman MD, Ombach HJ, Brown J, Lynch C, et al. Sex-based impact of creatine supplementation on depressive symptoms, brain serotonin and SSRI efficacy in an animal model of treatment-resistant depression. Int J Mol Sci. (2021) 22:8195. doi: 10.3390/ijms22158195
89. Rosa JM, Pazini FL, Cunha MP, Colla ARS, Manosso LM, Mancini G, et al. Antidepressant effects of creatine on amyloid β(1-40)-treated mice: The role of GSK-3β/Nrf(2) pathway. Prog Neuropsychopharmacol Biol Psychiatry. (2018) 86:270–8. doi: 10.1016/j.pnpbp.2018.05.001
90. Bakian AV, Huber RS, Scholl L, Renshaw PF, Kondo D. Dietary creatine intake and depression risk among U.S. adults. Transl Psychiatry. (2020) 10:52. doi: 10.1038/s41398-020-0741-x
91. Yoon S, Kim JE, Hwang J, Kim TS, Kang HJ, Namgung E, et al. Effects of creatine monohydrate augmentation on brain metabolic and network outcome measures in women with major depressive disorder. Biol Psychiatry. (2016) 80:439–47. doi: 10.1016/j.biopsych.2015.11.027
92. Kondo DG, Sung YH, Hellem TL, Fiedler KK, Shi X, Jeong EK, et al. Open-label adjunctive creatine for female adolescents with SSRI-resistant major depressive disorder: a 31-phosphorus magnetic resonance spectroscopy study. J Affect Disord. (2011) 135:354–61. doi: 10.1016/j.jad.2011.07.010
93. Pazini FL, Cunha MP, Azevedo D, Rosa JM, Colla A, de Oliveira J, et al. Creatine prevents corticosterone-induced reduction in hippocampal proliferation and differentiation: possible implication for its antidepressant effect. Mol Neurobiol. (2017) 54:6245–60. doi: 10.1007/s12035-016-0148-0
94. Kious BM, Kondo DG, Renshaw PF. Creatine for the treatment of depression. Biomolecules. (2019) 9:406. doi: 10.3390/biom9090406
95. Lyoo IK, Yoon S, Kim TS, Hwang J, Kim JE, Won W, et al. A randomized, double-blind placebo-controlled trial of oral creatine monohydrate augmentation for enhanced response to a selective serotonin reuptake inhibitor in women with major depressive disorder. Am J Psychiatry. (2012) 169:937–45. doi: 10.1176/appi.ajp.2012.12010009
96. Allen PJ, D'Anci KE, Kanarek RB, Renshaw PF. Sex-specific antidepressant effects of dietary creatine with and without sub-acute fluoxetine in rats. Pharmacol Biochem Behav. (2012) 101:588–601. doi: 10.1016/j.pbb.2012.03.005
97. Allen PJ, D'Anci KE, Kanarek RB, Renshaw PF. Chronic creatine supplementation alters depression-like behavior in rodents in a sex-dependent manner. Neuropsychopharmacology. (2010) 35:534–46. doi: 10.1038/npp.2009.160
98. Zhou B, Zhu Z, Ransom BR, Tong X. Oligodendrocyte lineage cells and depression. Mol Psychiatry. (2021) 26:103–17. doi: 10.1038/s41380-020-00930-0
99. Chamberlain KA, Chapey KS, Nanescu SE, Huang JK. Creatine enhances mitochondrial-mediated oligodendrocyte survival after demyelinating injury. J Neurosci. (2017) 37:1479–92. doi: 10.1523/jneurosci.1941-16.2016
100. Rosko LM, Gentile T, Smith VN, Manavi Z, Melchor GS, Hu J, et al. Cerebral creatine deficiency affects the timing of oligodendrocyte myelination. J Neurosci. (2023) 43:1143–53. doi: 10.1523/jneurosci.2120-21.2022
101. Feier G, Valvassori SS, Rezin GT, Búrigo M, Streck EL, Kapczinski F, et al. Creatine kinase levels in patients with bipolar disorder: depressive, manic, and euthymic phases. Braz J Psychiatry. (2011) 33:171–5. doi: 10.1590/s1516-44462011005000005
102. Hollander S, Hochman E, Shoval G, Taler M, Trommer S, Hermesh H, et al. The association between serum creatine kinase, mood and psychosis in inpatients with schizophrenia, bipolar and schizoaffective disorders. Psychiatry Res. (2016) 238:333–7. doi: 10.1016/j.psychres.2016.01.058
103. Hillbrand M, Spitz RT, Foster HG, Krystal JH, Young JL. Creatine kinase elevations and aggressive behavior in hospitalized forensic patients. Psychiatr Q. (1998) 69:69–82. doi: 10.1023/a:1022137524475
104. Meltzer H. Creatine kinase and aldolase in serum: abnormality common to acute psychoses. Science. (1968) 159:1368–70. doi: 10.1126/science.159.3821.1368
105. MacDonald ML, Naydenov A, Chu M, Matzilevich D, Konradi C. Decrease in creatine kinase messenger RNA expression in the hippocampus and dorsolateral prefrontal cortex in bipolar disorder. Bipolar Disord. (2006) 8:255–64. doi: 10.1111/j.1399-5618.2006.00302.x
106. Shao L, Martin MV, Watson SJ, Schatzberg A, Akil H, Myers RM, et al. Mitochondrial involvement in psychiatric disorders. Ann Med. (2008) 40:281–95. doi: 10.1080/07853890801923753
107. Du F, Yuksel C, Chouinard VA, Huynh P, Ryan K, Cohen BM, et al. Abnormalities in high-energy phosphate metabolism in first-episode bipolar disorder measured using (31)P-magnetic resonance spectroscopy. Biol Psychiatry. (2018) 84:797–802. doi: 10.1016/j.biopsych.2017.03.025
108. Stork C, Renshaw PF. Mitochondrial dysfunction in bipolar disorder: evidence from magnetic resonance spectroscopy research. Mol Psychiatry. (2005) 10:900–19. doi: 10.1038/sj.mp.4001711
109. Kato T. Neurobiological basis of bipolar disorder: Mitochondrial dysfunction hypothesis and beyond. Schizophr Res. (2017) 187:62–6. doi: 10.1016/j.schres.2016.10.037
110. Ongür D, Prescot AP, Jensen JE, Cohen BM, Renshaw PF. Creatine abnormalities in schizophrenia and bipolar disorder. Psychiatry Res. (2009) 172:44–8. doi: 10.1016/j.pscychresns.2008.06.002
111. Bio DS, Moreno RA, Garcia-Otaduy MC, Nery F, Lafer B, Soeiro-de-Souza MG. Altered brain creatine cycle metabolites in bipolar I disorder with childhood abuse: A (1)H magnetic resonance spectroscopy study. Prog Neuropsychopharmacol Biol Psychiatry. (2021) 109:110233. doi: 10.1016/j.pnpbp.2020.110233
112. Roschel H, Gualano B, Ostojic SM, Rawson ES. Creatine supplementation and brain health. Nutrients. (2021) 13:586. doi: 10.3390/nu13020586
113. Forbes SC, Cordingley DM, Cornish SM, Gualano B, Roschel H, Ostojic SM, et al. Effects of creatine supplementation on brain function and health. Nutrients. (2022) 14:921. doi: 10.3390/nu14050921
114. Toniolo RA, Fernandes FBF, Silva M, Dias RDS, Lafer B. Cognitive effects of creatine monohydrate adjunctive therapy in patients with bipolar depression: Results from a randomized, double-blind, placebo-controlled trial. J Affect Disord. (2017) 224:69–75. doi: 10.1016/j.jad.2016.11.029
115. Toniolo RA, Silva M, Fernandes FBF, Amaral JAMS, Dias RDS, Lafer B. A randomized, double-blind, placebo-controlled, proof-of-concept trial of creatine monohydrate as adjunctive treatment for bipolar depression. J Neural Transm (Vienna). (2018) 125:247–57. doi: 10.1007/s00702-017-1817-5
116. Roitman S, Green T, Osher Y, Karni N, Levine J. Creatine monohydrate in resistant depression: a preliminary study. Bipolar Disord. (2007) 9:754–8. doi: 10.1111/j.1399-5618.2007.00532.x
117. Valdés-Tovar M, Rodríguez-Ramírez AM, Rodríguez-Cárdenas L, Sotelo-Ramírez CE, Camarena B, Sanabrais-Jiménez MA, et al. Insights into myelin dysfunction in schizophrenia and bipolar disorder. World J Psychiatry. (2022) 12:264–85. doi: 10.5498/wjp.v12.i2.264
118. Tkachev D, Mimmack ML, Ryan MM, Wayland M, Freeman T, Jones PB, et al. Oligodendrocyte dysfunction in schizophrenia and bipolar disorder. Lancet. (2003) 362:798–805. doi: 10.1016/s0140-6736(03)14289-4
119. Balshaw TG, Bampouras TM, Barry TJ, Sparks SA. The effect of acute taurine ingestion on 3-km running performance in trained middle-distance runners. Amino Acids. (2013) 44:555–61. doi: 10.1007/s00726-012-1372-1
120. Seidel U, Huebbe P, Rimbach G. Taurine: A regulator of cellular redox homeostasis and skeletal muscle function. Mol Nutr Food Res. (2019) 63:e1800569. doi: 10.1002/mnfr.201800569
121. Wu G. Important roles of dietary taurine, creatine, carnosine, anserine and 4-hydroxyproline in human nutrition and health. Amino Acids. (2020) 52:329–60. doi: 10.1007/s00726-020-02823-6
122. Carvalho MB, Brandao CFC, Fassini PG, Bianco TM, Batitucci G, Galan BSM, et al. Taurine supplementation increases post-exercise lipid oxidation at moderate intensity in fasted healthy males. Nutrients. (2020) 12:1540. doi: 10.3390/nu12051540
123. Haidari F, Asadi M, Mohammadi-Asl J, Ahmadi-Angali K. Evaluation of the effect of oral taurine supplementation on fasting levels of fibroblast growth factors, β-Klotho co-receptor, some biochemical indices and body composition in obese women on a weight-loss diet: a study protocol for a double-blind, randomized controlled trial. Trials. (2019) 20:315. doi: 10.1186/s13063-019-3421-5
124. Murakami S. Role of taurine in the pathogenesis of obesity. Mol Nutr Food Res. (2015) 59:1353–63. doi: 10.1002/mnfr.201500067
125. Zhu Y, Wang R, Fan Z, Luo D, Cai G, Li X, et al. Taurine alleviates chronic social defeat stress-induced depression by protecting cortical neurons from dendritic spine loss. Cell Mol Neurobiol. (2023) 43:827–40. doi: 10.1007/s10571-022-01218-3
126. Wu GF, Ren S, Tang RY, Xu C, Zhou JQ, Lin SM, et al. Antidepressant effect of taurine in chronic unpredictable mild stress-induced depressive rats. Sci Rep. (2017) 7:4989. doi: 10.1038/s41598-017-05051-3
127. Craig SA. Betaine in human nutrition. Am J Clin Nutr. (2004) 80:539–49. doi: 10.1093/ajcn/80.3.539
128. Zhao G, He F, Wu C, Li P, Li N, Deng J, et al. Betaine in inflammation: mechanistic aspects and applications. Front Immunol. (2018) 9:1070. doi: 10.3389/fimmu.2018.01070
129. Chen YM, Liu Y, Liu YH, Wang X, Guan K, Zhu HL. Higher serum concentrations of betaine rather than choline is associated with better profiles of DXA-derived body fat and fat distribution in Chinese adults. Int J Obes (Lond). (2015) 39:465–71. doi: 10.1038/ijo.2014.158
130. Schartum-Hansen H, Ueland PM, Pedersen ER, Meyer K, Ebbing M, Bleie Ø, et al. Assessment of urinary betaine as a marker of diabetes mellitus in cardiovascular patients. PLoS One. (2013) 8:e69454. doi: 10.1371/journal.pone.0069454
131. Lever M, Slow S, McGregor DO, Dellow WJ, George PM, Chambers ST. Variability of plasma and urine betaine in diabetes mellitus and its relationship to methionine load test responses: an observational study. Cardiovasc Diabetol. (2012) 11:34. doi: 10.1186/1475-2840-11-34
132. Madsen SK, Rajagopalan P, Joshi SH, Toga AW, Thompson PM. Higher homocysteine associated with thinner cortical gray matter in 803 participants from the Alzheimer's Disease Neuroimaging Initiative. Neurobiol Aging. (2015) 36 Suppl 1:S203–10. doi: 10.1016/j.neurobiolaging.2014.01.154
133. Jeyhoonabadi M, Alimoahmmadi S, Hassanpour S, Hashemnia M. Betaine ameliorates depressive-like behaviors in zinc oxide nanoparticles exposed mice. Biol Trace Elem Res. (2022) 200:4771–81. doi: 10.1007/s12011-021-03068-4
134. Di Pierro F, Orsi R, Settembre R. Role of betaine in improving the antidepressant effect of S-adenosyl-methionine in patients with mild-to-moderate depression. J Multidiscip Healthc. (2015) 8:39–45. doi: 10.2147/jmdh.S77766
135. Di Pierro F, Settembre R. Preliminary results of a randomized controlled trial carried out with a fixed combination of S-adenosyl-L-methionine and betaine versus amitriptyline in patients with mild depression. Int J Gen Med. (2015) 8:73–8. doi: 10.2147/ijgm.S79518
136. Wortmann SB, Mayr JA. Choline-related-inherited metabolic diseases-A mini review. J Inherit Metab Dis. (2019) 42:237–42. doi: 10.1002/jimd.12011
137. Zeisel SH, da Costa KA. Choline: an essential nutrient for public health. Nutr Rev. (2009) 67:615–23. doi: 10.1111/j.1753-4887.2009.00246.x
138. Li J, Kang X, Zhang L, Luo J, Zhang D. Dietary choline is inversely associated with depressive symptoms: A cross-sectional study of the National Health and Nutrition Examination Survey (NHANES) 2011 to 2018. J Affect Disord. (2022) 301:23–9. doi: 10.1016/j.jad.2022.01.013
139. de Diego-Adeliño J, Portella MJ, Gómez-Ansón B, López-Moruelo O, Serra-Blasco M, Vives Y, et al. Hippocampal abnormalities of glutamate/glutamine, N-acetylaspartate and choline in patients with depression are related to past illness burden. J Psychiatry Neurosci. (2013) 38:107–16. doi: 10.1503/jpn.110185
140. Huang Y, Chen W, Li Y, Wu X, Shi X, Geng D. Effects of antidepressant treatment on N-acetyl aspartate and choline levels in the hippocampus and thalami of post-stroke depression patients: a study using (1)H magnetic resonance spectroscopy. Psychiatry Res. (2010) 182:48–52. doi: 10.1016/j.pscychresns.2009.11.009
141. Stoll AL, Cohen BM, Snyder MB, Hanin I. Erythrocyte choline concentration in bipolar disorder: a predictor of clinical course and medication response. Biol Psychiatry. (1991) 29:1171–80. doi: 10.1016/0006-3223(91)90325-g
142. Mahal P, Deep R, Kumaran SS, Khandelwal SK. Elevated choline in dorsolateral prefrontal cortex of lithium responders with bipolar I disorder. Asian J Psychiatr. (2023) 79:103318. doi: 10.1016/j.ajp.2022.103318
143. Moore CM, Breeze JL, Gruber SA, Babb SM, Frederick BB, Villafuerte RA, et al. Choline, myo-inositol and mood in bipolar disorder: a proton magnetic resonance spectroscopic imaging study of the anterior cingulate cortex. Bipolar Disord. (2000) 2:207–16. doi: 10.1034/j.1399-5618.2000.20302.x
144. Shi XF, Forrest LN, Kuykendall MD, Prescot AP, Sung YH, Huber RS, et al. Anterior cingulate cortex choline levels in female adolescents with unipolar versus bipolar depression: a potential new tool for diagnosis. J Affect Disord. (2014) 167:25–9. doi: 10.1016/j.jad.2014.05.051
145. Stary D, Bajda M. Taurine and creatine transporters as potential drug targets in cancer therapy. Int J Mol Sci. (2023) 24:3788. doi: 10.3390/ijms24043788
146. Kurtz JA, VanDusseldorp TA, Doyle JA, Otis JS. Taurine in sports and exercise. J Int Soc Sports Nutr. (2021) 18:39. doi: 10.1186/s12970-021-00438-0
147. Muñoz-Moreno E, Simões RV, Tudela R, López-Gil X, Soria G. Spatio-temporal metabolic rewiring in the brain of TgF344-AD rat model of Alzheimer's disease. Sci Rep. (2022) 12:16958. doi: 10.1038/s41598-022-20962-6
148. Fang Q, Liu J, Chen L, Chen Q, Wang Y, Li Z, et al. Taurine supplementation improves hippocampal metabolism in immature rats with intrauterine growth restriction (IUGR) through protecting neurons and reducing gliosis. Metab Brain Dis. (2022) 37:2077–88. doi: 10.1007/s11011-021-00896-0
149. Levy M, Thaiss CA, Zeevi D, Dohnalová L, Zilberman-Schapira G, Mahdi JA, et al. Microbiota-modulated metabolites shape the intestinal microenvironment by regulating NLRP6 inflammasome signaling. Cell. (2015) 163:1428–43. doi: 10.1016/j.cell.2015.10.048
150. Youn J, Cho E, Lee JE. Association of choline and betaine levels with cancer incidence and survival: A meta-analysis. Clin Nutr. (2019) 38:100–9. doi: 10.1016/j.clnu.2018.01.042
151. Sivanesan S, Taylor A, Zhang J, Bakovic M. Betaine and choline improve lipid homeostasis in obesity by participation in mitochondrial oxidative demethylation. Front Nutr. (2018) 5:61. doi: 10.3389/fnut.2018.00061
152. Chan KL, Poller WC, Swirski FK, Russo SJ. Central regulation of stress-evoked peripheral immune responses. Nat Rev Neurosci. (2023) 24:591–604. doi: 10.1038/s41583-023-00729-2
153. Stewart JC, Rand KL, Muldoon MF, Kamarck TW. A prospective evaluation of the directionality of the depression-inflammation relationship. Brain Behav Immun. (2009) 23:936–44. doi: 10.1016/j.bbi.2009.04.011
154. Köhler CA, Freitas TH, Stubbs B, Maes M, Solmi M, Veronese N, et al. Peripheral alterations in cytokine and chemokine levels after antidepressant drug treatment for major depressive disorder: systematic review and meta-analysis. Mol Neurobiol. (2018) 55:4195–206. doi: 10.1007/s12035-017-0632-1
155. Wang L, Wang R, Liu L, Qiao D, Baldwin DS, Hou R. Effects of SSRIs on peripheral inflammatory markers in patients with major depressive disorder: A systematic review and meta-analysis. Brain Behav Immun. (2019) 79:24–38. doi: 10.1016/j.bbi.2019.02.021
156. Brietzke E, Stertz L, Fernandes BS, Kauer-Sant'anna M, Mascarenhas M, Escosteguy Vargas A, et al. Comparison of cytokine levels in depressed, manic and euthymic patients with bipolar disorder. J Affect Disord. (2009) 116:214–7. doi: 10.1016/j.jad.2008.12.001
157. Hunt C, Macedo E, Cordeiro T, Suchting R, de Dios C, Cuellar Leal VA, et al. Effect of immune activation on the kynurenine pathway and depression symptoms - A systematic review and meta-analysis. Neurosci Biobehav Rev. (2020) 118:514–23. doi: 10.1016/j.neubiorev.2020.08.010
158. Dawood S, Bano S, Badawy AA. Inflammation and serotonin deficiency in major depressive disorder: molecular docking of antidepressant and anti-inflammatory drugs to tryptophan and indoleamine 2,3-dioxygenases. Biosci Rep. (2022) 42:BSR20220426. doi: 10.1042/bsr20220426
159. Monchaux De Oliveira C, De Smedt-Peyrusse V, Morael J, Vancassel S, Capuron L, Gaudout D, et al. Prevention of stress-induced depressive-like behavior by saffron extract is associated with modulation of kynurenine pathway and monoamine neurotransmission. Pharmaceutics. (2021) 13:2155. doi: 10.3390/pharmaceutics13122155
160. Hersey M, Samaranayake S, Berger SN, Tavakoli N, Mena S, Nijhout HF, et al. Inflammation-induced histamine impairs the capacity of escitalopram to increase hippocampal extracellular serotonin. J Neurosci. (2021) 41:6564–77. doi: 10.1523/jneurosci.2618-20.2021
161. Hersey M, Reneaux M, Berger SN, Mena S, Buchanan AM, Ou Y, et al. A tale of two transmitters: serotonin and histamine as in vivo biomarkers of chronic stress in mice. J Neuroinflamm. (2022) 19:167. doi: 10.1186/s12974-022-02508-9
162. Coussens LM, Werb Z. Inflammation and cancer. Nature. (2002) 420:860–7. doi: 10.1038/nature01322
163. Goldring MB, Otero M. Inflammation in osteoarthritis. Curr Opin Rheumatol. (2011) 23:471–8. doi: 10.1097/BOR.0b013e328349c2b1
164. Di Biase S, Ma X, Wang X, Yu J, Wang YC, Smith DJ, et al. Creatine uptake regulates CD8 T cell antitumor immunity. J Exp Med. (2019) 216:2869–82. doi: 10.1084/jem.20182044
165. Zhang M, Wang XL, Shi H, Meng LQ, Quan HF, Yan L, et al. Betaine inhibits NLRP3 inflammasome hyperactivation and regulates microglial M1/M2 phenotypic differentiation, thereby attenuating lipopolysaccharide-induced depression-like behavior. J Immunol Res 2022. (2022) p:9313436. doi: 10.1155/2022/9313436
166. Liu L, Wang H, Zhang H, Chen X, Zhang Y, Wu J, et al. Toward a deeper understanding of gut microbiome in depression: the promise of clinical applicability. Adv Sci (Weinh). (2022) 9:e2203707. doi: 10.1002/advs.202203707
167. Zheng P, Zeng B, Zhou C, Liu M, Fang Z, Xu X, et al. Gut microbiome remodeling induces depressive-like behaviors through a pathway mediated by the host's metabolism. Mol Psychiatry. (2016) 21:786–96. doi: 10.1038/mp.2016.44
168. Mörkl S, Butler MI, Lackner S. Advances in the gut microbiome and mood disorders. Curr Opin Psychiatry. (2023) 36:1–7. doi: 10.1097/yco.0000000000000829
169. Liu L, Wang H, Chen X, Zhang Y, Zhang H, Xie P. Gut microbiota and its metabolites in depression: from pathogenesis to treatment. EBioMedicine. (2023) 90:104527. doi: 10.1016/j.ebiom.2023.104527
170. Begum N, Mandhare A, Tryphena KP, Srivastava S, Shaikh MF, Singh SB, et al. Epigenetics in depression and gut-brain axis: A molecular crosstalk. Front Aging Neurosci. (2022) 14:1048333. doi: 10.3389/fnagi.2022.1048333
171. Wang D, Wu J, Zhu P, Xie H, Lu L, Bai W, et al. Tryptophan-rich diet ameliorates chronic unpredictable mild stress induced depression- and anxiety-like behavior in mice: The potential involvement of gut-brain axis. Food Res Int. (2022) 157:111289. doi: 10.1016/j.foodres.2022.111289
172. Haq S, Grondin JA, Khan WI. Tryptophan-derived serotonin-kynurenine balance in immune activation and intestinal inflammation. FASEB J. (2021) 35:e21888. doi: 10.1096/fj.202100702R
173. Kelly JR, Borre Y, O'Brien C, Patterson E, El Aidy S, Deane J, et al. Transferring the blues: Depression-associated gut microbiota induces neurobehavioural changes in the rat. J Psychiatr Res. (2016) 82:109–18. doi: 10.1016/j.jpsychires.2016.07.019
174. Messaoudi M, Lalonde R, Violle N, Javelot H, Desor D, Nejdi A, et al. Assessment of psychotropic-like properties of a probiotic formulation (Lactobacillus helveticus R0052 and Bifidobacterium longum R0175) in rats and human subjects. Br J Nutr. (2011) 105:755–64. doi: 10.1017/s0007114510004319
175. Ishioh M, Nozu T, Miyagishi S, Igarashi S, Funayama T, Ueno N, et al. Brain histamine improves colonic hyperpermeability through the basal forebrain cholinergic neurons, adenosine A2B receptors and vagus nerve in rats. Biochem Pharmacol. (2024) 224:116201. doi: 10.1016/j.bcp.2024.116201
176. Barcik W, Pugin B, Westermann P, Perez NR, Ferstl R, Wawrzyniak M, et al. Histamine-secreting microbes are increased in the gut of adult asthma patients. J Allergy Clin Immunol. (2016) 138:1491–1494.e7. doi: 10.1016/j.jaci.2016.05.049
177. Costa A, Rani B, Bastiaanssen TFS, Bonfiglio F, Gunnigle E, Provensi G, et al. Diet prevents social stress-induced maladaptive neurobehavioural and gut microbiota changes in a histamine-dependent manner. Int J Mol Sci. (2022) 23:862. doi: 10.3390/ijms23020862
178. Li JM, Yu R, Zhang LP, Wen SY, Wang SJ, Zhang XY, et al. Dietary fructose-induced gut dysbiosis promotes mouse hippocampal neuroinflammation: a benefit of short-chain fatty acids. Microbiome. (2019) 7:98. doi: 10.1186/s40168-019-0713-7
179. Caspani G, Kennedy S, Foster JA, Swann J. Gut microbial metabolites in depression: understanding the biochemical mechanisms. Microb Cell. (2019) 6:454–81. doi: 10.15698/mic2019.10.693
180. Rudzki L, Stone TW, Maes M, Misiak B, Samochowiec J, Szulc A. Gut microbiota-derived vitamins - underrated powers of a multipotent ally in psychiatric health and disease. Prog Neuropsychopharmacol Biol Psychiatry. (2021) 107:110240. doi: 10.1016/j.pnpbp.2020.110240
181. Dumas ME, Barton RH, Toye A, Cloarec O, Blancher C, Rothwell A, et al. Metabolic profiling reveals a contribution of gut microbiota to fatty liver phenotype in insulin-resistant mice. Proc Natl Acad Sci U.S.A. (2006) 103:12511–6. doi: 10.1073/pnas.0601056103
182. Liu CC, Wu YF, Feng GM, Gao XX, Zhou YZ, Hou WJ, et al. Plasma-metabolite-biomarkers for the therapeutic response in depressed patients by the traditional Chinese medicine formula Xiaoyaosan: A (1)H NMR-based metabolomics approach. J Affect Disord. (2015) 185:156–63. doi: 10.1016/j.jad.2015.05.005
183. Hao Z, Meng C, Li L, Feng S, Zhu Y, Yang J, et al. Positive mood-related gut microbiota in a long-term closed environment: a multiomics study based on the "Lunar Palace 365" experiment. Microbiome. (2023) 11:88. doi: 10.1186/s40168-023-01506-0
184. Liang XQ, Mai PY, Qin H, Li S, Ou WJ, Liang J, et al. Integrated 16S rRNA sequencing and metabolomics analysis to investigate the antidepressant role of Yang-Xin-Jie-Yu decoction on microbe-gut-metabolite in chronic unpredictable mild stress-induced depression rat model. Front Pharmacol. (2022) 13:972351. doi: 10.3389/fphar.2022.972351
185. Ichikawa S, Abe R, Fujimoto H, Higashi K, Zang L, Nakayama H, et al. Paraburkholderia sabiae administration alters zebrafish anxiety-like behavior via gut microbial taurine metabolism. Front Microbiol. (2023) 14:1079187. doi: 10.3389/fmicb.2023.1079187
186. Mlyniec K. Interaction between zinc, GPR39, BDNF and neuropeptides in depression. Curr Neuropharmacol. (2021) 19:2012–9. doi: 10.2174/1570159x19666210225153404
187. Miranda M, Morici JF, Zanoni MB, Bekinschtein P. Brain-derived neurotrophic factor: A key molecule for memory in the healthy and the pathological brain. Front Cell Neurosci. (2019) 13:363. doi: 10.3389/fncel.2019.00363
188. Lin CC, Huang TL. Brain-derived neurotrophic factor and mental disorders. BioMed J. (2020) 43:134–42. doi: 10.1016/j.bj.2020.01.001
189. Wang J, Liu B, Sun F, Xu Y, Luan H, Yang M, et al. Histamine H3R antagonist counteracts the impaired hippocampal neurogenesis in Lipopolysaccharide-induced neuroinflammation. Int Immunopharmacol. (2022) 110:109045. doi: 10.1016/j.intimp.2022.109045
190. Wang N, Ma J, Liu J, Wang J, Liu C, Wang H, et al. Histamine H3 receptor antagonist enhances neurogenesis and improves chronic cerebral hypoperfusion-induced cognitive impairments. Front Pharmacol. (2019) 10:1583. doi: 10.3389/fphar.2019.01583
191. Guilloux JP, Samuels BA, Mendez-David I, Hu A, Levinstein M, Faye C, et al. S 38093, a histamine H(3) antagonist/inverse agonist, promotes hippocampal neurogenesis and improves context discrimination task in aged mice. Sci Rep. (2017) 7:42946. doi: 10.1038/srep42946
192. Kim S, Hong KB, Kim S, Suh HJ, Jo K. Creatine and taurine mixtures alleviate depressive-like behaviour in Drosophila melanogaster and mice via regulating Akt and ERK/BDNF pathways. Sci Rep. (2020) 10:11370. doi: 10.1038/s41598-020-68424-1
193. Pazini FL, Rosa JM, Camargo A, Fraga DB, Moretti M, Siteneski A, et al. mTORC1-dependent signaling pathway underlies the rapid effect of creatine and ketamine in the novelty-suppressed feeding test. Chem Biol Interact. (2020) 332:109281. doi: 10.1016/j.cbi.2020.109281
194. Scotti-Muzzi E, Chile T, Vallada H, Otaduy MCG, Soeiro-de-Souza MG. BDNF rs6265 differentially influences neurometabolites in the anterior cingulate of healthy and bipolar disorder subjects. Brain Imaging Behav. (2023) 17:282–93. doi: 10.1007/s11682-023-00757-7
195. Gao Y, Sun C, Gao T, Liu Z, Yang Z, Deng H, et al. Taurine ameliorates volatile organic compounds-induced cognitive impairment in young rats via suppressing oxidative stress, regulating neurotransmitter and activating NMDA receptor. Front Vet Sci. (2022) 9:999040. doi: 10.3389/fvets.2022.999040
196. Lee WJ, Lee GH, Hur J, Lee HG, Kim E, Won JP, et al. Taurine and ginsenoside rf induce BDNF expression in SH-SY5Y cells: A potential role of BDNF in corticosterone-triggered cellular damage. Molecules. (2020) 25:2819. doi: 10.3390/molecules25122819
197. Liu F, Yuan M, Li C, Guan X, Li B. The protective function of taurine on pesticide-induced permanent neurodevelopmental toxicity in juvenile rats. FASEB J. (2021) 35:e21273. doi: 10.1096/fj.202001290R
198. Jangra A, Rajput P, Dwivedi DK, Lahkar M. Amelioration of repeated restraint stress-induced behavioral deficits and hippocampal anomalies with taurine treatment in mice. Neurochem Res. (2020) 45:731–40. doi: 10.1007/s11064-019-02945-8
199. Logan RW, McClung CA. Rhythms of life: circadian disruption and brain disorders across the lifespan. Nat Rev Neurosci. (2019) 20:49–65. doi: 10.1038/s41583-018-0088-y
200. Zhang SL, Lahens NF, Yue Z, Arnold DM, Pakstis PP, Schwarz JE, et al. A circadian clock regulates efflux by the blood-brain barrier in mice and human cells. Nat Commun. (2021) 12:617. doi: 10.1038/s41467-020-20795-9
201. Hartsock MJ, Spencer RL. Memory and the circadian system: Identifying candidate mechanisms by which local clocks in the brain may regulate synaptic plasticity. Neurosci Biobehav Rev. (2020) 118:134–62. doi: 10.1016/j.neubiorev.2020.07.023
202. Olejniczak I, Begemann K, Wilhelm I, Oster H. The circadian neurobiology of reward. Acta Physiol (Oxf). (2023) 237:e13928. doi: 10.1111/apha.13928
203. Bhatnagar A, Murray G, Ray S. Circadian biology to advance therapeutics for mood disorders. Trends Pharmacol Sci. (2023) 44:689–704. doi: 10.1016/j.tips.2023.07.008
204. Kanova M, Kohout P. Tryptophan: A unique role in the critically ill. Int J Mol Sci. (2021) 22:11714. doi: 10.3390/ijms222111714
205. Bortolato B, Berk M, Maes M, McIntyre RS, Carvalho AF. Fibromyalgia and bipolar disorder: emerging epidemiological associations and shared pathophysiology. Curr Mol Med. (2016) 16:119–36. doi: 10.2174/1566524016666160126144027
206. Pham L, Baiocchi L, Kennedy L, Sato K, Meadows V, Meng F, et al. The interplay between mast cells, pineal gland, and circadian rhythm: Links between histamine, melatonin, and inflammatory mediators. J Pineal Res. (2021) 70:e12699. doi: 10.1111/jpi.12699
207. Tuomisto L, Lozeva V, Valjakka A, Lecklin A. Modifying effects of histamine on circadian rhythms and neuronal excitability. Behav Brain Res. (2001) 124:129–35. doi: 10.1016/s0166-4328(01)00222-4
208. Christ P, Sowa AS, Froy O, Lorentz A. The circadian clock drives mast cell functions in allergic reactions. Front Immunol. (2018) 9:1526. doi: 10.3389/fimmu.2018.01526
209. Nakamura Y, Ishimaru K, Shibata S, Nakao A. Regulation of plasma histamine levels by the mast cell clock and its modulation by stress. Sci Rep. (2017) 7:39934. doi: 10.1038/srep39934
210. Hu W, Chen Z. The roles of histamine and its receptor ligands in central nervous system disorders: An update. Pharmacol Ther. (2017) 175:116–32. doi: 10.1016/j.pharmthera.2017.02.039
211. Kárpáti A, Yoshikawa T, Naganuma F, Matsuzawa T, Kitano H, Yamada Y, et al. Histamine H(1) receptor on astrocytes and neurons controls distinct aspects of mouse behaviour. Sci Rep. (2019) 9:16451. doi: 10.1038/s41598-019-52623-6
212. Karlstedt K, Senkas A, Ahman M, Panula P. Regional expression of the histamine H(2) receptor in adult and developing rat brain. Neuroscience. (2001) 102:201–8. doi: 10.1016/s0306-4522(00)00464-4
213. Pillot C, Heron A, Cochois V, Tardivel-Lacombe J, Ligneau X, Schwartz JC, et al. A detailed mapping of the histamine H(3) receptor and its gene transcripts in rat brain. Neuroscience. (2002) 114:173–93. doi: 10.1016/s0306-4522(02)00135-5
214. Sallmen T, Lozada AF, Anichtchik OV, Beckman AL, Panula P. Increased brain histamine H3 receptor expression during hibernation in golden-mantled ground squirrels. BMC Neurosci. (2003) 4:24. doi: 10.1186/1471-2202-4-24
Keywords: mood disorder, major depressive disorder, bipolar disorder, metabolite, biogenic amine
Citation: Yang J, Yuan M and Zhang W (2024) The major biogenic amine metabolites in mood disorders. Front. Psychiatry 15:1460631. doi: 10.3389/fpsyt.2024.1460631
Received: 06 July 2024; Accepted: 04 September 2024;
Published: 24 September 2024.
Edited by:
Shaohua Hu, Zhejiang University, ChinaReviewed by:
Yiru Fang, Shanghai Jiao Tong University, ChinaXiaoping Wang, Central South University, China
Copyright © 2024 Yang, Yuan and Zhang. This is an open-access article distributed under the terms of the Creative Commons Attribution License (CC BY). The use, distribution or reproduction in other forums is permitted, provided the original author(s) and the copyright owner(s) are credited and that the original publication in this journal is cited, in accordance with accepted academic practice. No use, distribution or reproduction is permitted which does not comply with these terms.
*Correspondence: Wei Zhang, d2VpemhhbmdoeEAxNjMuY29t