- 1CEINGE Biotecnologie Avanzate “Franco Salvatore”, Naples, Italy
- 2Department of Environmental, Biological and Pharmaceutical Sciences and Technologies, Università degli Studi della Campania “Luigi Vanvitelli”, Caserta, Italy
- 3Section of Psychiatry, Laboratory of Translational and Molecular Psychiatry and Unit of Treatment-Resistant Psychosis, Department of Neuroscience, Reproductive Sciences and Odontostomatology, University Medical School of Naples “Federico II”, Naples, Italy
- 4”The Protein Factory 2.0”, Dipartimento di Biotecnologie e Scienze della Vita, Università degli Studi dell’Insubria, Varese, Italy
- 5Department of Neuroscience, Rehabilitation, Ophthalmology, Genetics, Maternal, and Child Health - DINOGMI, University of Genoa, Genoa, Italy
- 6Center of Translational and Experimental Myology, Istituto di Ricovero e Cura a Carattere Scientifico (IRCCS) Istituto Giannina Gaslini, Genoa, Italy
- 7Department of Maternal and Child Health, Unità Operativa semplice di Dipartimento (UOSD) of Child and Adolescent Psychiatry, Azienda Ospedaliera Universitaria (AOU) Federico II, Naples, Italy
- 8Dipartimento di Medicina Molecolare e Biotecnologie Mediche, Università degli Studi di Napoli “Federico II”, Naples, Italy
- 9Department of Medical and Translational Sciences, Child Neuropsychiatry, Federico II University, Napoli, Italy
- 10Centro Interuniversitario per Malattie Multigeniche e Multifattoriali e loro Modelli Animali (Federico II, Naples; Tor Vergata, Rome and “G. D’Annunzio”, Chieti-Pescara), Naples, Italy
- 11Dipartimento di Agraria, Università degli Studi di Napoli “Federico II”, Portici, Italy
Introduction: Schizophrenia (SCZ) and autism spectrum disorder (ASD) are neurodevelopmental diseases characterized by different psychopathological manifestations and divergent clinical trajectories. Various alterations at glutamatergic synapses have been reported in both disorders, including abnormal NMDA and metabotropic receptor signaling.
Methods: We conducted a bicentric study to assess the blood serum levels of NMDA receptors-related glutamatergic amino acids and their precursors, including L-glutamate, L-glutamine, D-aspartate, L-aspartate, L-asparagine, D-serine, L-serine and glycine, in ASD, SCZ patients and their respective control subjects. Specifically, the SCZ patients were subdivided into treatment-resistant and non-treatment-resistant SCZ patients, based on their responsivity to conventional antipsychotics.
Results: D-serine and D-aspartate serum reductions were found in SCZ patients compared to controls. Conversely, no significant differences between cases and controls were found in amino acid concentrations in the two ASD cohorts analyzed.
Discussion: This result further encourages future research to evaluate the predictive role of selected D-amino acids as peripheral markers for SCZ pathophysiology and diagnosis.
1 Introduction
Several lines of evidence suggest a neurodevelopmental origin of neuropsychiatric disorders such as schizophrenia (SCZ) (1, 2) and autistic spectrum disorder (ASD) (3), both considered polygenic and multifactorial in origin (4, 5). Compelling evidence from neurochemical and genetic investigations points to abnormal glutamate synaptic features as a major underpinning of SCZ and ASD pathophysiology (6–10). Accordingly, SCZ and ASD show pronounced synapse alterations (11, 12) and aberrant cortical-subcortical brain connectivity (13, 14). Specifically, glutamatergic dysfunction has been reported both at the level of transporters (15) and receptors (16, 17) as well as at glutamatergic postsynaptic density (PSD) macromolecular protein assembly, also coherent with the evidence of abnormal Shank, Homer, and PSD95 expression (12, 18–21).
The relevance of glutamatergic system dysfunction in SCZ pathophysiology has been further expanded by the discovery of altered metabolism of two free amino acids in the atypical D-configuration, D-serine (D-Ser) and D-aspartate (D-Asp), in SCZ (22–26). Both D-amino acids modulate ionotropic NMDA receptor (NMDAR)-dependent transmission by acting as endogenous co-agonist (D-Ser) and agonist (D-Asp) at the glycine site of GluN1 (D-Ser) and the glutamate site of GluN2 (D-Asp) subunits of NMDARs (27–29). Furthermore, free D-Asp stimulates metabotropic Glu5 receptors (mGluR5) coupled to polyphosphoinositide hydrolysis in neonate rat brain slices, thus suggesting a functional involvement for this D-amino acid also on mGluR5 signaling during early postnatal life (30). Dedicated enzymatic systems regulate the endogenous levels of D-Ser and D-Asp. D-Ser is synthesized from L-Ser by serine racemase (SR) (31) and degraded by D-amino acid oxidase (DAAO) (32). Conversely, the enzymatic machinery responsible for D-Asp biosynthesis has not yet been fully identified even though SR can produce to some extent D-Asp in the forebrain (33, 34), while its degradation is catalyzed by D-aspartate oxidase (DASPO or DDO) activity (32, 35). Besides endogenous biosynthesis, recent studies highlighted that both dietary intake and gut microbiota contribute to the endogenous pool of D-amino acids (36–38). Interestingly, D-Ser and D-Asp display distinct ontogenetic profiles in the mammalian brain. Cerebral D-Ser levels are constantly elevated during lifetime and decrease in the elderly stage, due to reduced SR expression (39). On the other hand, D-Asp levels are substantially high in the developing brain and drastically decrease in adulthood (27, 29, 40). In agreement with the hypothesis of NMDAR hypofunction in SCZ (41, 42), previous evidence indicates lower D-Ser levels in the serum and cerebrospinal fluid of patients with SCZ (43–56). Furthermore, genetic studies revealed an association between SCZ and serine racemase (SR), and D-amino acid oxidase (DAAO) genes (57–61), as well as G72 gene, encoding the main DAAO modulator, pLG72 (62–64). Moreover, both D-Ser supplementation and DAAO inhibition have shown beneficial effects in modulating mismatch negativity response, cognition and extrapyramidal side-effects linked to antipsychotic treatment in SCZ patients (65–71). Also, preclinical studies revealed that reduced D-Ser levels in Sr knockout mice, a model of NMDAR hypofunction, show different phenotypes relevant to SCZ (72–74).
Despite the role of D-Asp in the mammalian central nervous system (CNS) has been so far much less detailed than that of D-Ser, preclinical and post-mortem findings in the last decade have suggested an involvement of this endogenous NMDAR agonist in SCZ pathophysiology (25, 26). In this regard, neurochemical analyses performed in two different post-mortem brain cohorts have shown that D-Asp content (detected in the order of tens of nmol/g tissue) decreases by about 30–40% in the prefrontal cortex (PFC) of SCZ patients, compared to non-psychiatric subjects (75, 76), accompanied by a concomitant reduction of D-Asp/total Asp ratio (76). Alteration of the latter parameter, representing an index of the metabolic conversion rate of L-Asp into its derivative, D-Asp, suggests the existence of a homeostatic cerebral dysregulation in the D-enantiomer metabolism, as also indicated by the increases in either enzymatic DDO activity (76) or DDO gene expression (75) in SCZ post-mortem PFC. Additionally, a recent study based on a machine learning hypothesis-free algorithm identified in the post-mortem dorsolateral PFC (DLPFC) a stable cluster of molecules of the glutamatergic synapse, including D-Asp/total Asp ratio and D-Ser, that discriminate SCZ patients from non-psychiatric controls (77). In line with the involvement of D-Asp metabolism deregulation as a potential vulnerability factor in the onset of neurodevelopmental disorders, we have recently identified a duplication of a chromosome 6 region, including the entire DDO gene, in a young patient with severe intellectual disability, thought disorders and behavioural abnormalities reminiscent of ASD and SCZ symptomatology (78). Consistent with this clinical evidence, we have also found that Ddo gene duplication and the consequent constitutive depletion of cerebral D-Asp levels in mice (79) produce abnormal corticogenesis, decrease cortico-striatal gray matter volume and induce social recognition memory deficit in adulthood (78).
Based on D-Ser and D-Asp involvement in NMDAR and mGluR5 signaling, in the present work, we analyzed the levels of these atypical molecules and other main neuroactive amino acids acting on glutamatergic neurotransmission in the serum of ASD and SCZ patients and their respective control groups.
2 Methods
2.1 Demographic and clinical characteristics of patients with schizophrenia
Blood serum samples were obtained from SCZ patients (n = 26) and non-psychiatric controls (n = 13). Patients with schizophrenia were recruited at the A.O.U. “Federico II” hospital of Naples over 6 months and diagnosed according to the Diagnostic and Statistical Manual of mental disorders, Fifth Edition (DSM-5) (80). Inclusion criteria for patients were: age 18–60 years; no evidence of worsening psychotic symptoms in the previous 6 months; absence of other major systemic, psychiatric (e.g., addictive disorders, frequent substance use in the 6 months prior to enrollment, etc.), or neurological disorders. Healthy controls were sex-matched individuals with no history of neurological, psychiatric, or systemic conditions or family psychiatric history. SCZ patients were divided into two groups according to treatment resistance: non-treatment-resistant schizophrenia (nTRS; n = 13) and treatment-resistant schizophrenia (TRS; n = 13). The treatment resistance condition was defined as a failure of at least two different antipsychotic regimens, each administered for > 6 weeks and at an optimal dose, according to the modified Treatment Response and Resistance in Psychosis Working Group Consensus criteria (81). All TRS patients were under treatment with clozapine while nTRS patients were treated with different conventional antipsychotics, such as olanzapine, risperidone, haloperidol, amisulpride, promazine, paliperidone and aripiprazole. Clinical data were collected within 1 month from the blood sample and included the severity of psychotic symptoms measured by the Positive and Negative Syndrome Scale (PANSS) (82) and cognitive performances assessed by the Brief Assessment of Cognition in Schizophrenia (BACS) (83). Demographic characteristics are reported in Table 1. Phlebotomy was conducted by a psychiatric nurse; collection was performed in fasting status, in the morning before breakfast. Serum was separated by centrifugation and stored at −80°C until analysis. Written informed consent was obtained from all subjects, according to the Declaration of Helsinki. The study was approved by the Ethics Committee of the University “Federico II” of Naples (protocol number: 195/19).

Table 1 Demographic characteristics of schizophrenia and control patients enrolled in the blood serum collection.
2.2 Demographic and clinical characteristics of patients with autism spectrum disorder
Blood serum samples were obtained from two different Italian hospitals: A.O.U. “Federico II”, Naples, Italy (ASD, n = 33; Control, n = 6) and Istituto Giannina Gaslini, Genoa, Italy (ASD, n = 20; Control, n = 24). Participants from A.O.U. “Federico II” were consecutive samples of children and adolescents, along 6 months, referred to the Department of Pediatrics — Unit of Child and Adolescent Neuropsychiatry, for an evaluation in a clinical hypothesis or revaluation of ASD. All the subjects received a full assessment, including a complete history (pregnancy, childbirth, psychomotor development), structured clinical interviews and validated observations [Autism Diagnostic Observation Schedule-2 (84), Griffiths Mental Development Scale (85) or Leiter International Performance Test-Revised (86), Vineland Adaptive Behavior Scales—II edition (87)]. Diagnosis of ASD was formulated according to DSM-5 (80).
About 60 subjects were evaluated; study participants included 33/60 ASD subjects, whose parents signed an informed consent form to participate in the study. Subjects were aged between 18 and 189 months, both males (n = 28) and females (n = 5). Inclusion criteria were a clinical diagnosis of ASD, less than 18 years of age; exclusion criteria included: epilepsy diagnosis or other neurological disorders; psychiatric comorbidity (e.g. obsessive-compulsive disorder, psychosis, etc.), other chronic diseases (e.g. chronic intestinal diseases, malabsorption, etc.).
Six healthy typically developed subjects were recruited as a control group; inclusion criteria were the absence of psychiatric diagnosis, less than 18 years of age. For the control group, the same exclusion criteria were used.
The enrolled subjects followed routine clinical procedures for outpatients, from which data were collected. Each patient was also investigated by blood samples, as per routine procedures during clinical evaluation. Blood samples were collected in the hospital for both ASD and control children. Phlebotomy was conducted by a pediatric nurse, collection was made in fasting status, in the morning before breakfast. Serum was separated by centrifugation and stored at −80°C until analysis. The study was conducted according to the principles of the Declaration of Helsinki; ethical approval was obtained by the Ethics Committee of the University Federico II of Naples (220/18). Written informed consent was collected from parents or legal guardians of enrolled children for both clinical information collection and data acquisition and treatment.
Participants from Istituto Giannina Gaslini were consecutive samples of children and adolescents, referred to the Child Neuropsychiatry Unit Day Hospital for a third-level neuroradiological, biochemical, metabolic and genetic evaluation in a clinical diagnosis of ASD. All the subjects received a full assessment, including a complete history (pregnancy, childbirth, psychomotor development), structured clinical interviews and validated observations [Autism Diagnostic Observation Schedule-2 (84), Autism Diagnostic Interview (Lord et al., 1994), Griffiths Mental Development Scale (85), Wechsler Intelligence Scale for Children – fourth edition (Wechsler D., 2003) or the Wechsler Preschool and Primary Scale of Intelligence - III edition - WPPSI-III (Wechsler D., 2002), Vineland Adaptive Behavior Scales—II edition (87)]. Diagnosis of ASD was formulated according to DSM-5 (80).
Study participants included 20 ASD subjects aged between 3 years and 6 months and 11 years and 4 months, 19 males and 1 female. Inclusion criteria were a clinical diagnosis of ASD, less than 18 years of age; while exclusion criteria were the presence of other psychiatric diagnosis, epilepsy or other chronic diseases. Twenty-four developing normal children were recruited as a control group; inclusion criteria were the absence of psychiatric diagnosis, less than 18 years of age. For the control group, the same exclusion criteria were used.
Blood collection was made in fasting status, in the morning. Serum was separated by centrifugation and stored at −80°C until analysis. The study was conducted according to the principles of the Declaration of Helsinki; ethical approval was obtained by the Ethics Committee of the Liguria Region (N. CET - Liguria: 437/2023 - DB id 13411). Demographic characteristics of ASD and control individuals from both hospitals’ cohorts, such as age and sex distribution, are reported in Table 2. The hospitals involved in the study were chosen for the presence of SCZ outpatient clinics including a referral center for treatment-resistant psychosis (adult patients at University Federico II - Psychiatry Section) and for being regional centers of child psychiatry referral (Child Neuropsychiatry at Istituto Giannina Gaslini of Genoa and A.O.U. Federico II Neuropsychiatry Section of Naples).
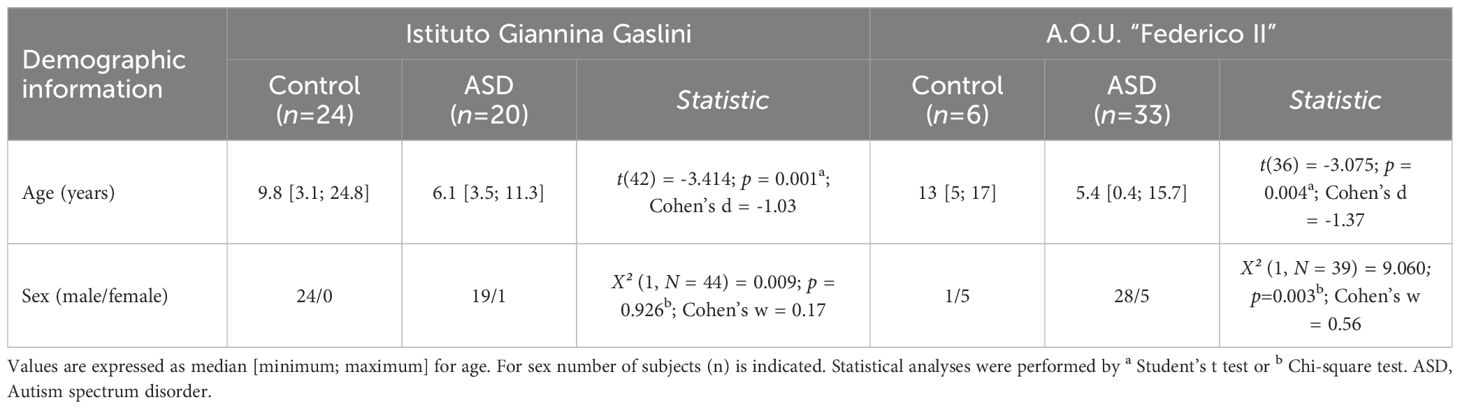
Table 2 Demographic characteristics of ASD and control patients enrolled by two different Italian hospitals.
2.3 HPLC analysis
Serum samples were mixed in a 1:10 dilution with HPLC-grade methanol (900 µL) and centrifuged at 13,000 x g for 10 min. Supernatants were dried at 45°C and suspended in 0.2 M trichloroacetic acid (TCA). Samples were then neutralized with 0.2 M NaOH and subjected to pre-column derivatization with o-phthaldialdehyde/N-acetyl-L-cysteine in 50% methanol. To resolve diastereoisomer derivatives, two types of columns were used: a ZORBAX Eclipse Plus C8 5-μm reversed-phase column (Agilent, 4.6x250 mm) and a Symmetry C8 5 μm reversed-phase column (Waters, 4.6x250mm); the separation was performed under isocratic conditions (0.1 M sodium acetate buffer, pH 6.2, 1% tetrahydrofuran, 1 mL/min flow rate). A washing step in 0.1 M sodium acetate buffer, 3% tetrahydrofuran and 47% acetonitrile, was performed after every single run. Identification and quantification of D-Asp, L-aspartate (L-Asp), L-glutamate (L-Glu), L-asparagine (L-Asn), D-Ser, L-serine (L-Ser), L-glutamine (L-Gln) and glycine (Gly) were based on retention times and peak areas and then compared with those associated with external standards (Figure 1A). Peak’s identity was confirmed by the selective degradation of the D-enantiomers by RgDAAO M213R variant (Figure 1A) (88). Ten μg of the enzyme was added to the samples, incubated at 30°C for at least 3 h, and subsequently derivatized. Amino acids concentration in the serum were expressed as µM. D-amino acid/total amino acid ratio was expressed as a percentage (%). Quantification of enantiomers was based on peak areas using calibration curves for each enantiomer.
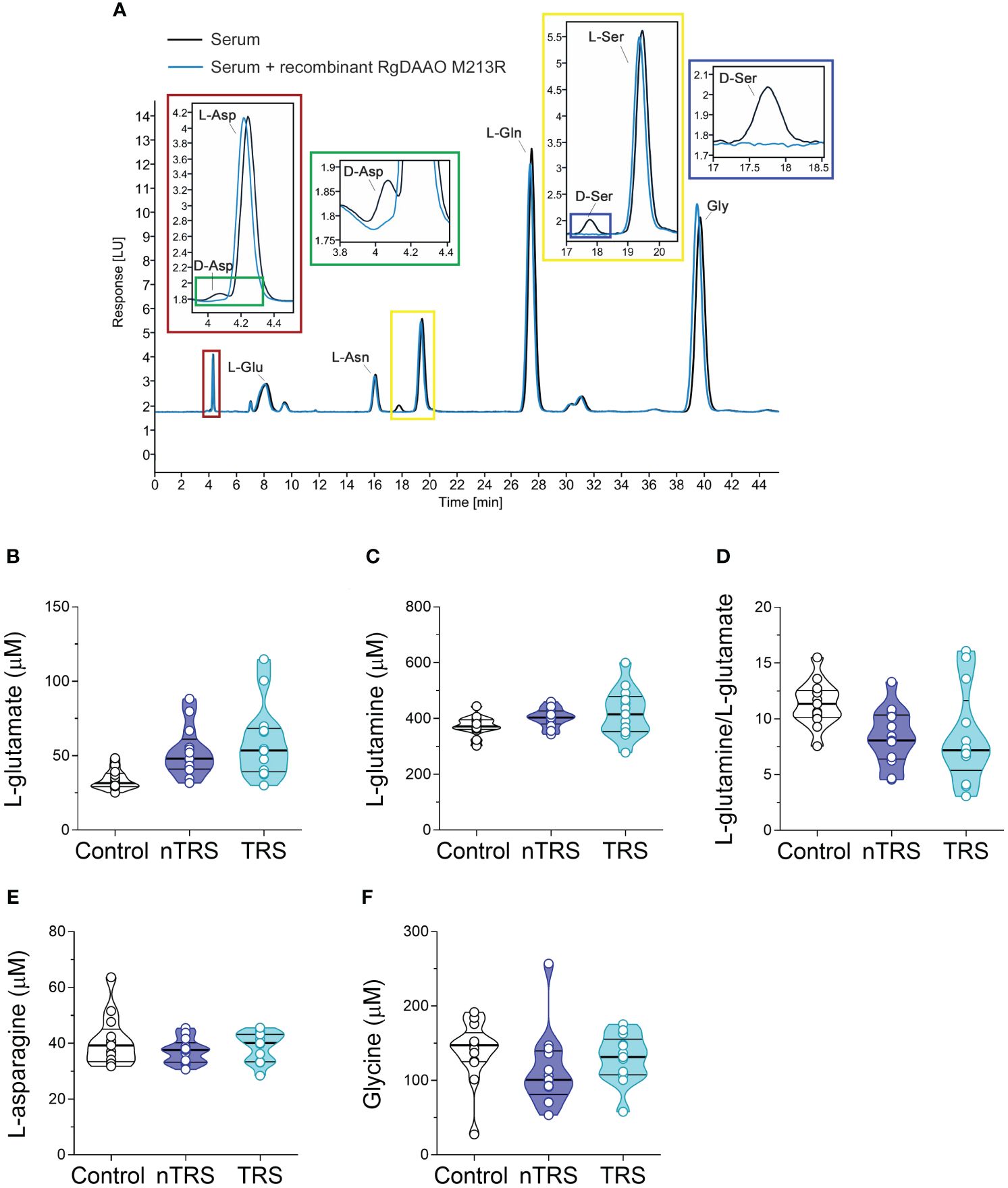
Figure 1 L-glutamate, L-glutamine, L-asparagine and glycine levels in the serum of schizophrenia patients and control subjects. (A) Overlaid HPLC chromatograms illustrating the specificity of D-aspartate (D-Asp) and D-serine (D-Ser) peaks obtained from blood serum samples. The identity of the peaks corresponding to D-aspartate and D-serine were verified by treating serum sample with RgDAAO M213R variant (a, inset, blu line). Analysis of (B) L-glutamate and (C) L-glutamine levels, (D) L-glutamine/L-glutamate ratio, (E) L-asparagine and (F) glycine levels in the serum of non-treatment-resistant schizophrenia (nTRS, n = 13) and treatment-resistant schizophrenia (TRS, n = 13) compared to control subjects (n = 13). The amino acid content was expressed as μM. In each sample, free amino acids were detected in a single run. Dots represent the single subjects’ values, while bars illustrate the median with interquartile range.
2.4 Statistical analysis
Data for clinical characteristics are reported as medians with the respective interquartile range (first-third quartile). The hypothesis of normality was assessed with the Shapiro-Wilk test. For variables not normally distributed, statistical analyses were performed using their log-transformed values. To identify potential confounders, we compared the demographic characteristics between patients and controls using two-sample (two-tailed) t-tests for age and Chi-square tests with Yates’ correction for sex. ANCOVA models (controlling for statistically different covariates between groups) were adopted to assess significant differences in amino acid concentrations between cases and controls. The p-values from ANCOVA models were corrected for multiple testing, following Bonferroni’s method. ANCOVAs were followed by Tukey post-hoc comparisons. To estimate the effect magnitude of significant outcomes, we computed the partial eta square (η²p), which provides a quantifiable measure of the proportion of variance in the dependent variable that is associated with an independent variable, while controlling for other variables. For Chi-square and t-test statistics, the Cohen’s w and d were computed as effect sizes, respectively. All statistical analyses were conducted with RStudio R version 4.1.2.
3 Results
3.1 L-glutamate serum levels show a trend toward an increase in schizophrenia patients
We recruited SCZ patients (n = 26) and non-psychiatric control subjects (n = 13) in A.O.U. “Federico II” Hospital (Figure 1; Table 1) to measure by HPLC the serum levels of L-Glu and Gly, which in the brain represent, respectively, the main excitatory amino acid and a major NMDAR co-agonist (together with D-Ser), as well as L-Gln and L-Asn, which participate to L-Glu and L-Asp biosynthesis, respectively. Specifically, SCZ patients were subdivided into nTRS and TRS groups (n = 13/condition) based on the assessment of symptoms persistence after at least two different conventional antipsychotic regimens (see Materials and Methods). No statistically significant differences were found in sex [χ2 (2, N = 39) = 5.0556, p = 0.0798, Cohen’s w = 0.36], while significant age-dependent variations were observed [median (min; max) of years: Ctrl = 28 (21; 40), nTRS = 47 (22; 60), TRS = 34 (25; 57), F(2, 36) = 10.688, p = 0.0002, η²p = 0.37, one-way ANOVA; Table 1] among groups. Based on this, to assess the changes in amino acid levels, we used an ANCOVA model considering the effect of age as a confounding factor.
We found significant differences in L-Glu serum content among groups [F(2, 35) = 5.552, p = 0.0082, η²p = 0.24], evidencing an increase of this amino acid in both nTRS and TRS patients, compared to controls (Figures 1A, B; Supplementary Table 1). However, such L-Glu serum variation did not survive after correction with the Bonferroni multiple comparisons method (Supplementary Table 1). Similarly, no alterations among groups were found for L-Gln levels, L-Gln/L-Glu ratio, L-Asn and Gly levels (Figures 1A, C–F; Supplementary Table 1). Overall, our analysis revealed no significant changes in L-Glu, L-Gln, L-Asn and Gly serum levels, as well as L-Gln/L-Glu serum ratio, in both nTRS and TRS patients, compared to their non-psychiatric controls.
3.2 Reduced D-serine and D-aspartate levels in the serum of schizophrenia patients
After we measured the levels of D-Ser, D-Asp and their respective precursors, L-Ser and L-Asp, the latter being also one of the major NMDAR agonists in the CNS. Statistical analysis revealed significant alteration in D-Asp levels in nTRS and TRS patients, compared to control individuals [F(2, 35) = 8.397, p = 0.001, η²p = 0.324], which survived also after correction with Bonferroni multiple comparisons (Figures 2A; Supplementary Table 1). The following Tukey post-hoc test highlighted that both nTRS and TRS patients displayed significantly reduced D-Asp levels, compared to controls (Ctrl vs nTRS, p = 0.0104; Ctrl vs TRS, p = 0.0177), while no significant alteration was observed between nTRS and TRS groups (TRS vs nTRS, p = 0.976) (Figure 2A; Supplementary Table 1). Conversely, we found comparable levels of L-Asp [F(2, 35) = 2.794, p = 0.0749] and D-Asp/total Asp ratio [F(2, 35) = 0.476, p = 0.6255] among nTRS, TRS and control subjects (Figures 2B, C; Supplementary Table 1).
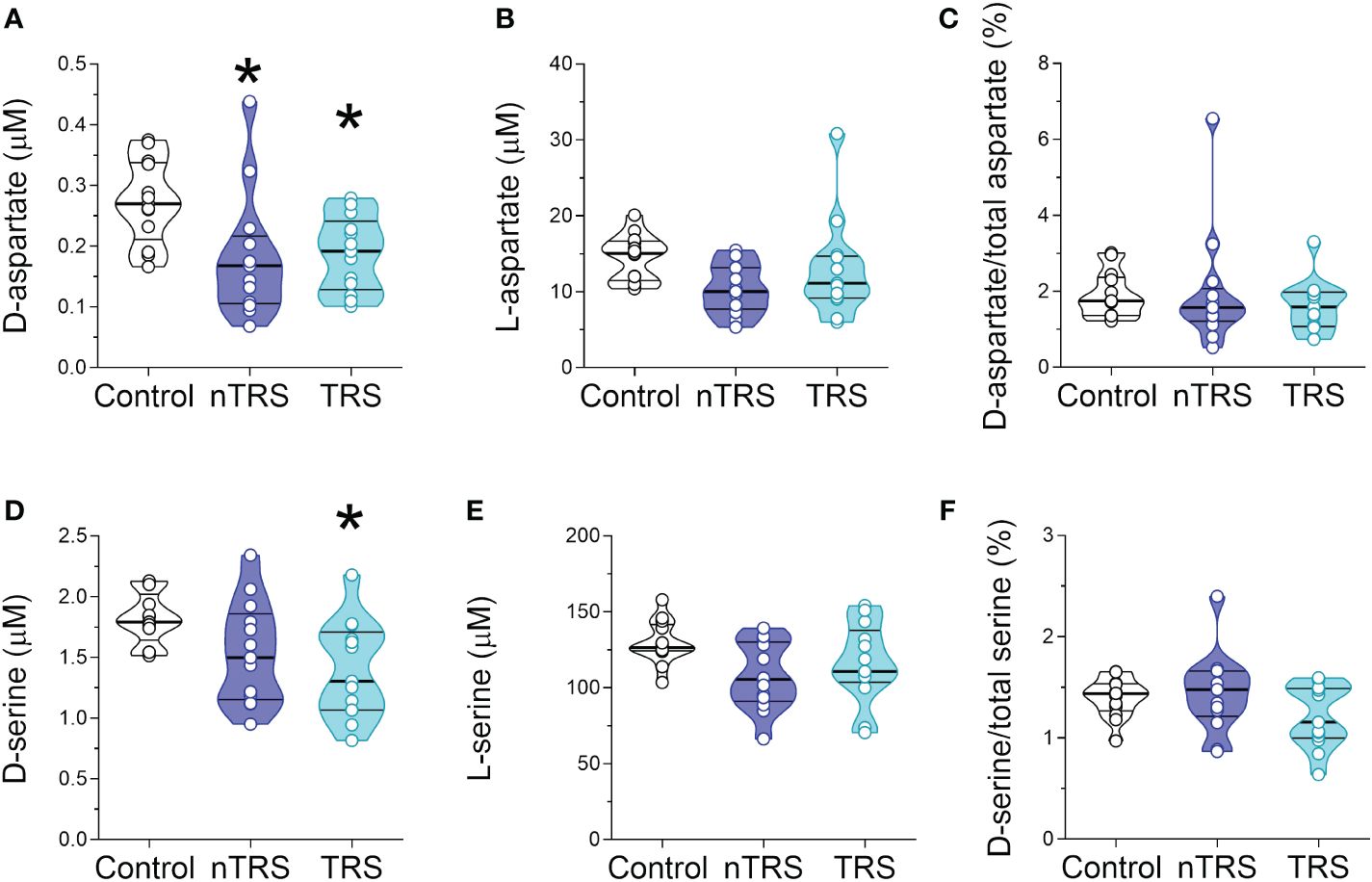
Figure 2 D-aspartate, L-aspartate, D-serine and L-serine levels in the serum of schizophrenia patients and control subjects. Analysis of (A) D-aspartate and (B) L-aspartate levels, (C) D-aspartate/total aspartate ratio, (D) D-serine and (E) L-serine levels, and (F) D-serine/total serine ratio in the serum of non-treatment-resistant schizophrenia (nTRS, n = 13) and treatment-resistant schizophrenia (TRS, n = 13) compared to control subjects (n = 13). The amino acid content was expressed as μM, while the ratios were expressed as percentages (%). In each sample, free amino acids were detected in a single run. Dots represent the single subjects’ values, while bars illustrate the median with interquartile range. *p <0.05, compared to the control group (Tukey post-hoc comparisons).
Statistical analysis also revealed significant differences in D-Ser serum levels among nTRS, TRS patients and control subjects [F(2, 35) = 6.322, p = 0.0045, η²p = 0.265; Figure 2D; Supplementary Table 1), which was confirmed after correction with Bonferroni multiple comparisons (Supplementary Table 1). The following Tukey post-hoc analysis evidenced a significant decrease of D-Ser in TRS but not in nTRS patients, compared to controls (Ctrl vs nTRS, p = 0.1078; Ctrl vs TRS, p = 0.0103; Figure 2D; Supplementary Table 1). However, no D-Ser changes were found between TRS and nTRS patients (TRS vs nTRS, p = 0.569; Figure 2D; Supplementary Table 1). Also in this case, the deregulation was confined to the D-enantiomer levels, as L-Ser levels did not significantly change among groups [F(2, 35) = 2.900, p = 0.0683; Figure 2E; Supplementary Table 1]. Despite the decrease being specific for the D-Ser, we found an unaltered D-Ser/total Ser ratio [F(2, 35) = 1.757, p = 0.1875; Figure 2F; Supplementary Table 1].
Altogether, our analyses showed selective reductions in D-Asp serum levels in both nTRS and TRS patients, and in D-Ser serum levels only in TRS group, compared to non-psychiatric control subjects. Conversely, no alterations were found in their respective L-enantiomers, L-Asp and L-Ser, among nTRS, TRS and control individuals.
3.3 Unaltered L-glutamate, L-glutamine, L-asparagine and glycine levels in the serum of ASD patients
Then we measured the levels of the same neuroactive amino acids and their precursors in the serum of pediatric ASD patients and control subjects recruited in two different Italian Hospitals (Istituto Giannina Gaslini: ASD, n = 20, Ctrl, n = 24; A.O.U. “Federico II”: ASD, n = 33; Ctrl, n = 6). First, we analyzed the cohort of ASD patients and control subjects from Istituto Giannina Gaslini. Before proceeding with statistical comparisons, we assessed potential imbalance in clinical variables, such as sex and age, between ASD patients and control individuals. No statistically significant differences were found in sex (χ2 (1, N = 44) = 0.009, p = 0.926), while significant variations between groups were observed in age [median (min; max) of years: Ctrl = 9.8 (3.1; 24.8) vs ASD = 6.1 (3.5; 11.3), t(42) = -3.414, p = 0.001, Cohen’s d = -1.03; Student’s t test] (Table 2). Based on this, to assess amino acid variations between ASD and control subjects, we used ANCOVA model, including age as a confounder. Statistical analysis revealed no significant alterations in L-Glu [F(1, 41) = 0.769, p = 0.3855] and L-Gln [F(1, 41) = 2.127, p = 0.1524] levels, L-Gln/L-Glu ratio [F(1, 41) = 0.285, p = 0.5961], as well as L-Asn [F(1, 41) = 1.785; p = 0.1889] and Gly [F(1, 41) = 2.143; p = 0.1508] levels between the two diagnosis groups (Figures 3A–E, Supplementary Table 2).
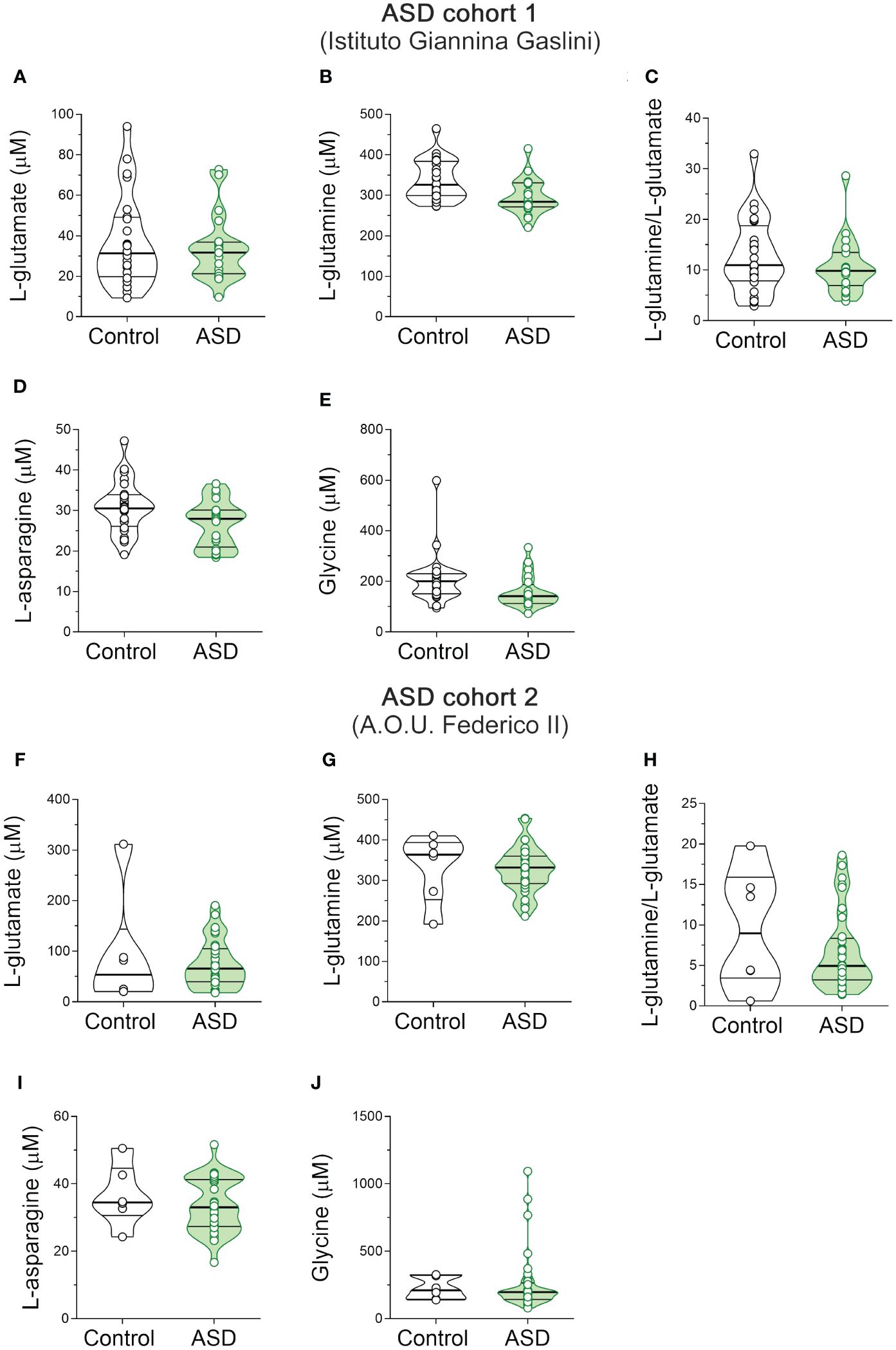
Figure 3 L-glutamate, L-glutamine, L-asparagine and glycine content in the serum of autism spectrum disorder patients. Analysis of (A, F) L-glutamate and (B, G) L-glutamine levels, (C, H) L-glutamine/L-glutamate ratio, (D, I) L-asparagine and (E, J) glycine levels in the serum of autism spectrum disorder (ASD) patients compared to control subjects enrolled in (A–E) Istituto Giannina Gaslini (Control, n = 24; ASD, n = 20) and (F–J) A.O.U. Federico II (Control, n = 6; ASD, n = 33) hospitals. The amino acid content was expressed as μM. In each sample, free amino acids were detected in a single run. Dots represent the single subjects’ values, while bars illustrate the median with interquartile range.
Then, we analyzed the cohort of ASD patients and control subjects recruited from A.O.U. “Federico II” hospital. We observed significant differences in both sex (χ2 (1, N = 39) = 9.060; p = 0.003, Cohen’s w = 0.56) and age [median (min; max) of years: Ctrl = 13 (5; 17) vs ASD = 5.4 (0.4; 15.7); t(36) = -3.075, p = 0.004, Cohen’s d = -1.37; Student’s t test] (Table 2). For this reason, we evaluated the differences between groups by ANCOVA models, including both age and sex as confounders. HPLC analysis revealed unaltered levels of each of the analyzed amino acids [L-Glu: F(1, 34) = 3.307, p = 0.0778; L-Gln: F(1, 34) = 1.504, p = 0.2285; L-Gln/L-Glu ratio: F(1, 34) = 0.170, p = 0.6825; L-Asn F(1, 34) = 1.188, p = 0.2834; Gly: F(1, 34) = 0.044, p = 0.8346] (Figures 3F–J, Supplementary Table 2) in ASD patients, compared to control subjects. Collectively, our results show no significant alterations in L-Glu, L-Gln, L-Asn and Gly serum levels, as well as L-Gln/L-Glu serum ratio, in both cohorts of ASD patients analyzed, compared to their respective control individuals.
3.4 Unaltered D-serine, D-aspartate and their L-enantiomers levels in the serum of ASD patients
Finally, we measured the serum content of D-Ser, L-Ser, D-Asp and L-Asp. Statistical analysis in ASD patients and control subjects from Istituto Giannina Gaslini revealed no significant differences in D-Asp (F(1, 41) = 0.046, p = 0.8317), L-Asp (F(1, 41) = 1.443, p = 0.2366), and D-Asp/total Asp ratio (F(1, 41) = 0.561, p = 0.4583) between ASD patients and control subjects (Figures 4A–C, Supplementary Table 2). Likewise, we found comparable levels between diagnoses also for D-Ser (F(1, 41) = 0.235, p = 0.6301), L-Ser (F(1, 41) = 0.659, p = 0.4218) and D-Ser/total Ser ratio (F(1, 41) = 0.058, p = 0.8113) (Figures 4D–F, Supplementary Table 2). Finally, we analyzed ASD patients and control subjects from A.O.U. “Federico II”. We found unaltered D-Asp levels between diagnoses (F(1, 41) = 0.036, p = 0.8507; Figure 4G, Supplementary Table 2). Conversely, we showed a slight but significant L-Asp levels increase in ASD patients, compared to controls (F(1, 34) = 4.449, p = 0.0424; η²p = 0.12; Figure 4H, Supplementary Table 2), which did not survive after correction with Bonferroni multiple comparisons method (Supplementary Table 2). In line with unaltered D-Asp and L-Asp levels, D-Asp/total Asp ratio did not significantly change between diagnosis groups (F(1, 34) = 0.0001 p = 0.9954; Figure 4I, Supplementary Table 2). Again, ANCOVA analysis revealed comparable D-Ser and L-Ser levels [F(1, 34) = 0.027, p = 0.8704], L-Ser [F(1, 34) = 3.132, p = 0.0858], and D-Ser/total Ser ratio [F(1, 34) = 1.347; p = 0.2539] (Figures 4J–L, Supplementary Table 2) between ASD patients and control individuals. Our overall HPLC analysis revealed no significant changes in D-Asp, L-Asp, D-Ser and L-Ser serum levels, as well as D-Asp/total Asp and D-Ser/total Ser serum ratios, in both cohorts of ASD patients analyzed, compared to their respective control individuals.
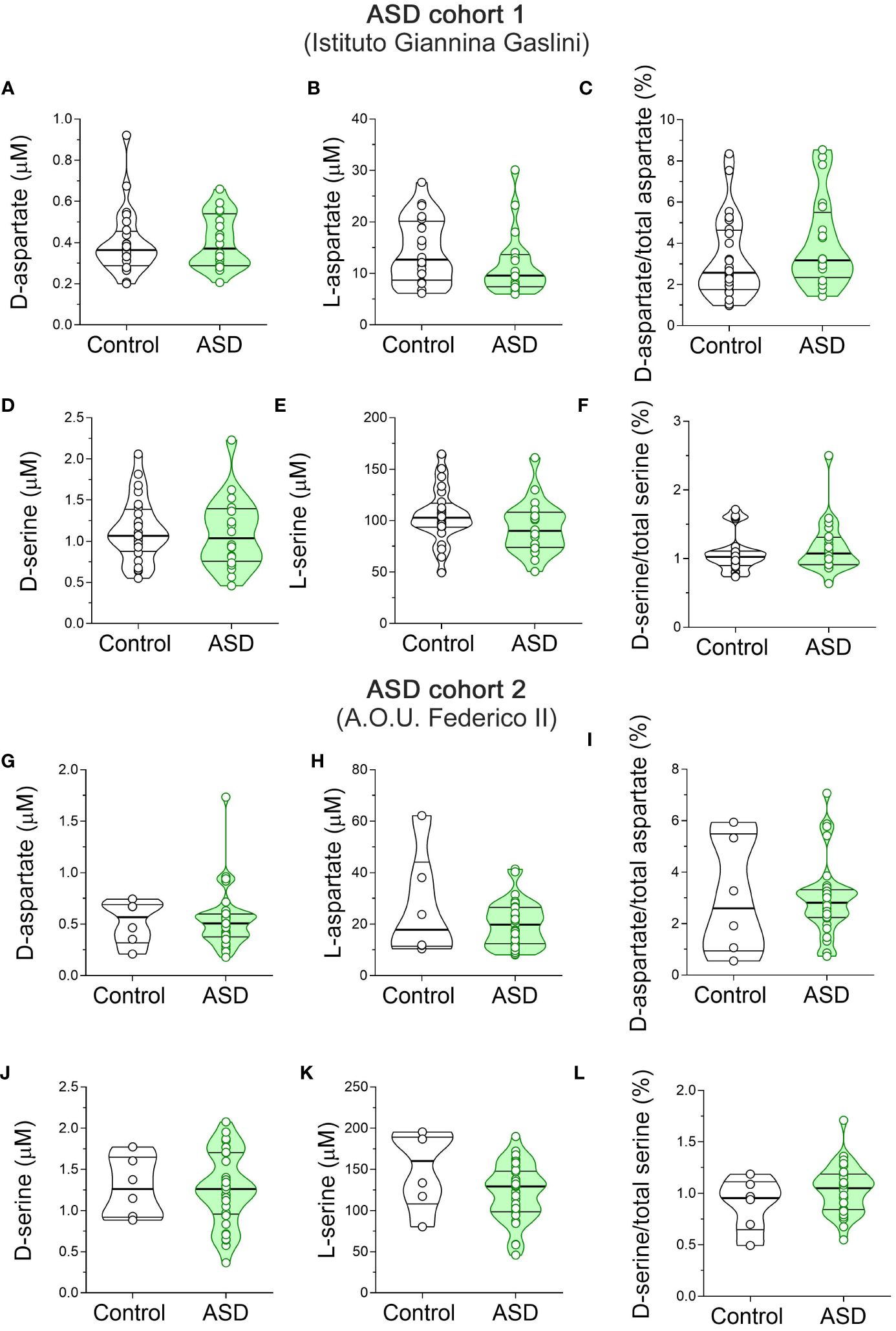
Figure 4 D-aspartate, L-aspartate, D-serine and L-serine levels in the serum of autism spectrum disorder patients and control subjects. Analysis of (A, G) D-aspartate and (B, H) L-aspartate levels, (C, I) D-aspartate/total aspartate ratio, (D, J) D-serine and (E, K) L-serine levels, and (F, L) D-serine/total serine ratio in the serum of autism spectrum disorder (ASD) patients compared to control subjects enrolled by (A–F) Istituto Giannina Gaslini (Control, n = 24; ASD, n = 20) and (G–I) A.O.U. Federico II (Control, n = 6; ASD, n = 33) hospitals. The amino acid content was expressed as μM, while the ratios were expressed as percentages (%). In each sample, free amino acids were detected in a single run. Dots represent the single subjects’ values, while bars illustrate the median with interquartile range. .
4 Discussion
In the present work, we aimed to measure the serum levels of the major neuroactive amino acids acting in glutamatergic system in patients with ASD and SCZ, compared to healthy controls. Overall, in ASD patients, recruited in two different Medical Centers, we failed to reveal significant variations in serum levels of all the amino acids detected. Previous findings have shown contrasting results on this subject (89). Indeed, some studies are in line with our data as they revealed unaltered Gln, Gly, Asp, L-Ser and D-Ser levels in the blood of ASD patients, compared to control subjects (90, 91). Conversely, increased Glu, Asp, Asn (90–100) or decreased Gln and Asn (90, 92–95, 98, 100, 101) levels have been reported in other investigations. Methodological issues and the sample size of the cohorts of patients included in the different studies may explain these discrepancies (89). Furthermore, divergent clinical phenotypes may also cause inconsistency, as ASD features are highly heterogeneous in terms of clinical presentation, genetic variability, and comorbidity (102).
Unlike ASD, HPLC analysis revealed variations in both D-Asp and D-Ser serum levels in SCZ patients, compared to non-psychiatric controls. In particular, we detected significantly lower D-Ser serum levels in TRS, and a trend toward reduction in the nTRS group, compared to controls. D-Ser levels were comparable between TRS and nTRS patients. No statistically significant changes in L-Ser levels occurred between clinical conditions. These results are in agreement with other studies revealing decreased peripheral blood levels of D-Ser in SCZ patients (43, 44, 48–55). Noteworthy, this is the first study providing peripheral blood levels of D-Asp and its ratio with total Asp in SCZ patients. Interestingly, we detected a significant downregulation in D-Asp serum levels in both TRS and nTRS patients relative to controls. In contrast, the serum concentration of the putative D-Asp precursor, L-Asp, and the D-Asp/total Asp ratio did not change among clinical conditions.
The decreased D-Ser and D-Asp serum levels reported in the current investigation may reflect previous evidence of altered levels of both D-amino acids in the brain and CSF of SCZ patients, compared to non-psychiatric subjects. Indeed, lower D-Ser levels and/or D-Ser/total Ser ratio were found in two independent CSF cohorts of SCZ patients (46, 47). Likewise, a significant downregulation of D-Asp levels and D-Asp/total Asp ratio was found in two independent cohorts of post-mortem PFC samples from SCZ patients, associated with either increased DDO gene expression (103) or enzymatic DDO activity (76). In line with a possible D-Asp metabolism dysfunction in neurodevelopmental processes, a recent study reported the first case of DDO gene duplication in a young patient with clinical manifestations resembling both ASD and SCZ symptoms (78).
Several studies support the hypothesis that NMDAR hypofunction in the SCZ brain accounts for cognitive and attentional deficits commonly reported in patients (104). Based on this assumption, abnormally lower cerebral D-Ser and D-Asp levels in the developing brain might produce NMDAR abnormalities and, in turn, contribute to SCZ pathophysiology (6, 26, 105). However, despite the existence of a correlation between blood and CSF amino acid levels, including glycine and serine (106), and the ability of D-Ser and D-Asp to cross the blood-brain barrier (107–109), it is yet unknown whether the circulating amounts of D-Ser and D-Asp may reflect the synaptic concentrations of these endogenous NMDAR signaling molecules in the SCZ brain.
We argue that lower serum levels of D-Ser and D-Asp, but not of their corresponding L-enantiomers, in SCZ patients may depend on the complex dynamics of D-amino acid metabolism in both brain and peripheral organs (38), thus possibly implying dysfunction of the catabolic enzymes, DDO and DAAO, the DAAO modulator pLG72, and the biosynthetic enzyme SR, the latter being also involved in D-Asp production, at least in the mouse forebrain regions (33, 34).
Besides canonical endogenous sources, recent work revealed that an important contribution to systemic D-amino acid level variations originates from the intestine (37), which regulates the absorption of diet- and microbial-derived exogenous amino acids. In this organ, the abundance of bacterial D-amino acids exerts a key role in modulating mammalian immune responses and symbiosis with bacteria (110). Therefore, any event producing dysbiosis, such as inflammation or pharmacological treatments, may alter peripheral and central D-Ser and D-Asp homeostasis as both D-amino acids cross the blood-brain barrier (108, 109). Since an increased prevalence of neuroinflammation and autoimmune disorders is well documented in SCZ (111), we hypothesize that these conditions may contribute to the altered D-Ser and D-Asp serum levels reported in patients.
Another important issue is whether D-Ser and D-Asp serum levels are linked to antipsychotic treatments. A longitudinal analysis of D-Ser plasma levels in TRS patients revealed a comparable reduction of this D-amino acid either before or after clozapine treatment, relative to baseline, despite the decrease being statistically significant only before clozapine administration (51). This evidence would suggest that clozapine administration does not contribute to the specific D-Ser serum level reduction observed in our TRS patients, as they were all under treatment with this antipsychotic. Another study evidenced increased serum levels of different amino acids, among which Asp, Ser, Glu and Gly, in SCZ patients treated with clozapine, relative to those treated with conventional antipsychotics (112). However, a comparison with our study is challenging since the work of Melkersson et al. lacks a reference non-psychiatric control group to assess basal amino acid concentrations and does not discriminate between D- and L-enantiomers. Finally, although restricted to the CNS of preclinical models, our and other studies revealed an effect of clozapine and olanzapine on the brain availability of D-Ser and D-Asp, respectively. Indeed, it has been reported that clozapine modulates extracellular D-Ser release in the medial PFC of freely moving rats (113), while olanzapine affects D-Asp metabolism in the mouse brain by inhibiting enzymatic DDO activity (109).
A recent line of research is bringing attention to the influence of antipsychotic medications on the composition of gut microbiota (114), thus potentially linking their use to changes in systemic D-amino acids availability. In particular, a recent study reported differentially abundant bacterial species in the gut microbiota of individuals with SCZ responding or resistant to antipsychotic treatment compared to healthy controls suggesting a potential role of clozapine in TRS patients (115). Future studies on larger cohorts of nTRS and TRS patients extended to first-episode patients and at-risk mental state individuals, will be needed to help clarify the still unclear role of antipsychotics on D-amino acid metabolism. Additionally, blood D-Ser levels are strongly correlated with glomerular filtration rate, emerging as a potential biomarker of kidney functionality (116, 117). As SCZ patients are more likely to have comorbid chronic kidney diseases (118), the potential bias of kidney dysfunction should be taken into account when considering peripheral D-Ser changes in SCZ patients.
Here, we also found a remarkable trend towards L-Glu upregulation in the serum of both nTRS and TRS patients, compared to controls. In line with present data, previous findings revealed that peripheral blood variations of this excitatory amino acid may reflect antipsychotic therapy (112, 119–122).
The major limitation of this work is the relatively small sample size of the ASD and SCZ patient cohorts and their relative controls. In the ASD study, such constraint is partially attenuated by recruiting two independent cohorts of patients and respective control subjects, whose HPLC serum detections revealed comparable results for all the amino acids analyzed.
To our knowledge, the present work represents the first serum detection of D-Asp in ASD and SCZ patients, as prior studies did not differentiate the relative contribution of D- and L-stereoisomers. Another strength point of our study is the simultaneous analysis of all the main D- and L-excitatory amino acids implicated in NMDAR transmission and their precursors. However, as L-Glu is also the precursor of the principal inhibitory neurotransmitter of the CNS, the gamma amino-butyric acid (GABA), future studies are required to evaluate the contribution of inhibitory neurotransmission in developmental psychiatric disorders and understand whether D-Ser and D-Asp serum levels may change in SCZ patients as compensatory events for potential inhibitory system dysfunctions.
In conclusion, further studies on new and more numerous cohorts of patients are needed to confirm the unaltered amino acid levels found in the serum of ASD patients, compared to controls. Conversely, the detection of lower D-Ser and D-Asp serum levels in SCZ patients encourages future research aimed at evaluating the potential role of these atypical amino acids as in vivo biochemical markers for alteration of the glutamatergic system in SCZ and association of putative differential changes of D-Ser and D-Asp levels with different classes of antipsychotics and response to treatment.
Data availability statement
The raw data supporting the conclusions of this article will be made available by the authors, without undue reservation.
Ethics statement
The studies involving humans were approved by Ethics Committee of the University “Federico II” of Naples (protocol number: 195/19); Ethics Committee of the University Federico II of Naples (220/18); Ethics Committee of the Liguria Region (N. CET - Liguria: 437/2023 - DB id 13411). The studies were conducted in accordance with the local legislation and institutional requirements. Written informed consent for participation in this study was provided by the participants’ legal guardians/next of kin.
Author contributions
MG: Investigation, Writing – original draft. GD: Investigation, Writing – original draft. ZM: Investigation, Writing – original draft. TN: Investigation, Writing – original draft. ED: Resources, Writing – review & editing. CB: Resources, Writing – review & editing. SB: Resources, Writing – review & editing. MR: Resources, Writing – review & editing. LP: Writing – review & editing. CB: Resources, Writing – review & editing. FI: Resources, Writing – review & editing. FS: Conceptualization, Writing – review & editing. LP: Conceptualization, Funding acquisition, Supervision, Validation, Writing – review & editing. FE: Conceptualization, Funding acquisition, Supervision, Writing – review & editing. AD: Conceptualization, Funding acquisition, Resources, Supervision, Writing – review & editing. AU: Conceptualization, Funding acquisition, Project administration, Supervision, Writing – review & editing.
Funding
The author(s) declare financial support was received for the research, authorship, and/or publication of this article. The study was supported by the Italian Ministry of Universities and Research (Ministero dell’Università e della Ricerca, MUR) through PRIN 2017 -Project nr 2017M42834 (to FE, AB and AU), PRIN 2020 -Project nr 2020K53E57 (to AU and LP) and PRIN PNRR 2022 financed by the European Union -Next Generation EU -Project nr P2022ZEMZF (to FE and AU). The work of FI, GS, and TN was supported by #NEXTGENERATIONEU (NGEU) and funded by MUR, National Recovery and Resilience Plan (NRRP), project MNESYS (PE0000006) - A Multiscale integrated approach to the study of the nervous system in health and disease (DN. 1553 11.10.2022). ZM is a PhD student of the Life Sciences and Biotechnology course at the University of Insubria.
Conflict of interest
The authors declare that the research was conducted in the absence of any commercial or financial relationships that could be construed as a potential conflict of interest.
The author(s) declared that they were an editorial board member of Frontiers, at the time of submission. This had no impact on the peer review process and the final decision.
Publisher’s note
All claims expressed in this article are solely those of the authors and do not necessarily represent those of their affiliated organizations, or those of the publisher, the editors and the reviewers. Any product that may be evaluated in this article, or claim that may be made by its manufacturer, is not guaranteed or endorsed by the publisher.
Supplementary material
The Supplementary Material for this article can be found online at: https://www.frontiersin.org/articles/10.3389/fpsyt.2024.1408175/full#supplementary-material
References
1. Weinberger DR. The neurodevelopmental origins of schizophrenia in the penumbra of genomic medicine. World psychiatry: Off J World Psychiatr Assoc (WPA). (2017) 16:225–6. doi: 10.1002/wps.20474
2. Weinberger DR. From neuropathology to neurodevelopment. Lancet. (1995) 346:552–7. doi: 10.1016/S0140-6736(95)91386-6
3. Girault JB, Piven J. The neurodevelopment of autism from infancy through toddlerhood. Neuroimaging Clinics North America. (2020) 30:97–114. doi: 10.1016/j.nic.2019.09.009
4. Bayer TA, Falkai P, Maier W. Genetic and non-genetic vulnerability factors in schizophrenia: the basis of the “Two hit hypothesis. J Psychiatr Res. (1999) 33:543–8. doi: 10.1016/S0022-3956(99)00039-4
5. Grove J, Ripke S, Als TD, Mattheisen M, Walters RK, Won H, et al. Identification of common genetic risk variants for autism spectrum disorder. Nat Genet. (2019) 51:431–44. doi: 10.1038/s41588-019-0344-8
6. Uno Y, Coyle JT. Glutamate hypothesis in schizophrenia. Psychiatry Clin Neurosci. (2019) 73:204–15. doi: 10.1111/pcn.12823
7. Montanari M, Martella G, Bonsi P, Meringolo M. Autism spectrum disorder: focus on glutamatergic neurotransmission. Int J Mol Sci. (2022) 23:3861. doi: 10.3390/ijms23073861
8. Nisar S, Bhat AA, Masoodi T, Hashem S, Akhtar S, Ali TA, et al. Genetics of glutamate and its receptors in autism spectrum disorder. Mol Psychiatry. (2022) 27:2380–92. doi: 10.1038/s41380-022-01506-w
9. Eltokhi A, Santuy A, Merchan-Perez A, Sprengel R. Glutamatergic dysfunction and synaptic ultrastructural alterations in schizophrenia and autism spectrum disorder: evidence from human and rodent studies. Int J Mol Sci. (2020) 22:59. doi: 10.3390/ijms22010059
10. Singh T, Poterba T, Curtis D, Akil H, Al Eissa M, Barchas JD, et al. Rare coding variants in ten genes confer substantial risk for schizophrenia. Nature. (2022) 604:509–16. doi: 10.1038/s41586-022-04556-w
11. Zatkova M, Bakos J, Hodosy J, Ostatnikova D. Synapse alterations in autism: Review of animal model findings. Biomed papers Med Faculty Univ Palacky Olomouc CzechoSlovakia. (2016) 160:201–10. doi: 10.5507/bp.2015.066
12. de Bartolomeis A, Vellucci L, De Simone G, Mazza B, Barone A, Ciccarelli M. Dysregulated signaling at postsynaptic density: A systematic review and translational appraisal for the pathophysiology, clinics, and antipsychotics’ Treatment of schizophrenia. Cells. (2023) 12:574. doi: 10.3390/cells12040574
13. Friston KJ, Frith CD. Schizophrenia: a disconnection syndrome? Clin Neurosci (New York NY). (1995) 3:89–97.
14. O’Reilly C, Lewis JD, Elsabbagh M. Is functional brain connectivity atypical in autism? A systematic review of EEG and MEG studies. PloS One. (2017) 12:e0175870. doi: 10.1371/journal.pone.0175870
15. Parkin GM, Gibbons A, Udawela M, Dean B. Excitatory amino acid transporter (EAAT)1 and EAAT2 mRNA levels are altered in the prefrontal cortex of subjects with schizophrenia. J Psychiatr Res. (2020) 123:151–8. doi: 10.1016/j.jpsychires.2020.02.004
16. Nakazawa K, Sapkota K. The origin of NMDA receptor hypofunction in schizophrenia. Pharmacol Ther. (2020) 205:107426. doi: 10.1016/j.pharmthera.2019.107426
17. Won H, Lee H-R, Gee HY, Mah W, Kim J-I, Lee J, et al. Autistic-like social behaviour in Shank2-mutant mice improved by restoring NMDA receptor function. Nature. (2012) 486:261–5. doi: 10.1038/nature11208
18. Guo H, Bettella E, Marcogliese PC, Zhao R, Andrews JC, Nowakowski TJ, et al. Disruptive mutations in TANC2 define a neurodevelopmental syndrome associated with psychiatric disorders. Nat Commun. (2019) 10:4679. doi: 10.1038/s41467-019-12435-8
19. de Bartolomeis A, Barone A, Buonaguro EF, Tomasetti C, Vellucci L, Iasevoli F. The Homer1 family of proteins at the crossroad of dopamine-glutamate signaling: An emerging molecular “Lego” in the pathophysiology of psychiatric disorders. A systematic review and translational insight. Neurosci Biobehav Rev. (2022) 136:104596. doi: 10.1016/j.neubiorev.2022.104596
20. Coley AA, Gao WJ. PSD95: A synaptic protein implicated in schizophrenia or autism? Prog Neuropsychopharmacol Biol Psychiatry. (2018) 82:187–94. doi: 10.1016/j.pnpbp.2017.11.016
21. Sala C, Vicidomini C, Bigi I, Mossa A, Verpelli C. Shank synaptic scaffold proteins: keys to understanding the pathogenesis of autism and other synaptic disorders. J Neurochem. (2015) 135:849–58. doi: 10.1111/jnc.13232
22. Errico F, Mothet JP, Usiello A. D-Aspartate: An endogenous NMDA receptor agonist enriched in the developing brain with potential involvement in schizophrenia. J Pharm BioMed Anal. (2015) 116:7–17. doi: 10.1016/j.jpba.2015.03.024
23. Javitt DC, Zukin SR, Heresco-Levy U, Umbricht D. Has an angel shown the way? Etiological and therapeutic implications of the PCP/NMDA model of schizophrenia. Schizophr Bull. (2012) 38:958–66. doi: 10.1093/schbul/sbs069
24. Labrie V, Wong AH, Roder JC. Contributions of the D-serine pathway to schizophrenia. Neuropharmacology. (2012) 62:1484–503. doi: 10.1016/j.neuropharm.2011.01.030
25. de Bartolomeis A, Vellucci L, Austin MC, De Simone G, Barone A. Rational and translational implications of D-amino acids for treatment-resistant schizophrenia: from neurobiology to the clinics. Biomolecules. (2022) 12:909. doi: 10.3390/biom12070909
26. Errico F, Nuzzo T, Carella M, Bertolino A, Usiello A. The emerging role of altered d-aspartate metabolism in schizophrenia: New insights from preclinical models and human studies. Front Psychiatry. (2018) 9:559. doi: 10.3389/fpsyt.2018.00559
27. Souza INO, Roychaudhuri R, de Belleroche J, Mothet JP. d-Amino acids: new clinical pathways for brain diseases. Trends Mol Med. (2023) 29:1014–28. doi: 10.1016/j.molmed.2023.09.001
28. Coyle JT, Balu D, Wolosker H. D-serine, the shape-shifting NMDA receptor co-agonist. Neurochem Res. (2020) 45:1344–53. doi: 10.1007/s11064-020-03014-1
29. Errico F, Cuomo M, Canu N, Caputo V, Usiello A. New insights on the influence of free d-aspartate metabolism in the mammalian brain during prenatal and postnatal life. Biochim Biophys Acta Proteins Proteom. (2020) 1868:140471. doi: 10.1016/j.bbapap.2020.140471
30. Molinaro G, Pietracupa S, Di Menna L, Pescatori L, Usiello A, Battaglia G, et al. D-Aspartate activates mGlu receptors coupled to polyphosphoinositide hydrolysis in neonate rat brain slices. Neurosci Letters. (2010) 478:128–30. doi: 10.1016/j.neulet.2010.04.077
31. Wolosker H, Blackshaw S, Snyder SH. Serine racemase: a glial enzyme synthesizing D-serine to regulate glutamate-N-methyl-D-aspartate neurotransmission. Proc Natl Acad Sci U S A. (1999) 96:13409–14. doi: 10.1073/pnas.96.23.13409
32. Pollegioni L, Sacchi S, Murtas G. Human D-amino acid oxidase: structure, function, and regulation. Front Mol Biosci. (2018) 5:107. doi: 10.3389/fmolb.2018.00107
33. Horio M, Ishima T, Fujita Y, Inoue R, Mori H, Hashimoto K. Decreased levels of free D-aspartic acid in the forebrain of serine racemase (Srr) knock-out mice. Neurochem Int. (2013) 62:843–7. doi: 10.1016/j.neuint.2013.02.015
34. Ito T, Hayashida M, Kobayashi S, Muto N, Hayashi A, Yoshimura T, et al. Serine racemase is involved in d-aspartate biosynthesis. J Biochem. (2016) 160:345–53. doi: 10.1093/jb/mvw043
35. Pollegioni L, Molla G, Sacchi S, Murtas G. Human D-aspartate oxidase: A key player in D-aspartate metabolism. Front Mol Biosci. (2021) 8:689719. doi: 10.3389/fmolb.2021.689719
36. Sasabe J, Miyoshi Y, Rakoff-Nahoum S, Zhang T, Mita M, Davis BM, et al. Interplay between microbial d-amino acids and host d-amino acid oxidase modifies murine mucosal defence and gut microbiota. Nat Microbiol. (2016) 1:16125. doi: 10.1038/nmicrobiol.2016.125
37. Gonda Y, Matsuda A, Adachi K, Ishii C, Suzuki M, Osaki A, et al. Mammals sustain amino acid homochirality against chiral conversion by symbiotic microbes. Proc Natl Acad Sci U S A. (2023) 120:e2300817120. doi: 10.1073/pnas.2300817120
38. Suzuki M, Shimizu-Hirota R, Mita M, Hamase K, Sasabe J. Chiral resolution of plasma amino acids reveals enantiomer-selective associations with organ functions. Amino Acids. (2022) 54:421–32. doi: 10.1007/s00726-022-03140-w
39. Orzylowski M, Fujiwara E, Mousseau DD, Baker GB. An overview of the involvement of D-serine in cognitive impairment in normal aging and dementia. Front Psychiatry. (2021) 12:754032. doi: 10.3389/fpsyt.2021.754032
40. Hashimoto A, Oka T. Free D-aspartate and D-serine in the mammalian brain and periphery. Prog Neurobiol. (1997) 52:325–53. doi: 10.1016/S0301-0082(97)00019-1
41. Coyle JT. NMDA receptor and schizophrenia: a brief history. Schizophr Bull. (2012) 38:920–6. doi: 10.1093/schbul/sbs076
42. Moghaddam B, Javitt D. From revolution to evolution: the glutamate hypothesis of schizophrenia and its implication for treatment. Neuropsychopharmacology. (2012) 37:4–15. doi: 10.1038/npp.2011.181
43. Cho SE, Na KS, Cho SJ, Kang SG. Low d-serine levels in schizophrenia: A systematic review and meta-analysis. Neurosci Lett. (2016) 634:42–51. doi: 10.1016/j.neulet.2016.10.006
44. Calcia MA, Madeira C, Alheira FV, Silva TC, Tannos FM, Vargas-Lopes C, et al. Plasma levels of D-serine in Brazilian individuals with schizophrenia. Schizophr Res. (2012) 142:83–7. doi: 10.1016/j.schres.2012.09.014
45. Ohnuma T, Sakai Y, Maeshima H, Hatano T, Hanzawa R, Abe S, et al. Changes in plasma glycine, L-serine, and D-serine levels in patients with schizophrenia as their clinical symptoms improve: results from the Juntendo University Schizophrenia Projects (JUSP). Prog Neuropsychopharmacol Biol Psychiatry. (2008) 32:1905–12. doi: 10.1016/j.pnpbp.2008.07.022
46. Bendikov I, Nadri C, Amar S, Panizzutti R, De Miranda J, Wolosker H, et al. A CSF and postmortem brain study of D-serine metabolic parameters in schizophrenia. Schizophr Res. (2007) 90:41–51. doi: 10.1016/j.schres.2006.10.010
47. Hashimoto K, Engberg G, Shimizu E, Nordin C, Lindstrom LH, Iyo M. Reduced D-serine to total serine ratio in the cerebrospinal fluid of drug naive schizophrenic patients. Prog Neuropsychopharmacol Biol Psychiatry. (2005) 29:767–9. doi: 10.1016/j.pnpbp.2005.04.023
48. Hashimoto K, Fukushima T, Shimizu E, Komatsu N, Watanabe H, Shinoda N, et al. Decreased serum levels of D-serine in patients with schizophrenia: evidence in support of the N-methyl-D-aspartate receptor hypofunction hypothesis of schizophrenia. Arch Gen Psychiatry. (2003) 60:572–6. doi: 10.1001/archpsyc.60.6.572
49. Hons J, Zirko R, Vasatova M, Doubek P, Klimova B, Masopust J, et al. Impairment of executive functions associated with lower D-serine serum levels in patients with schizophrenia. Front Psychiatry. (2021) 12:514579. doi: 10.3389/fpsyt.2021.514579
50. Panizzutti R, Fisher M, Garrett C, Man WH, Sena W, Madeira C, et al. Association between increased serum d-serine and cognitive gains induced by intensive cognitive training in schizophrenia. Schizophr Res. (2019) 207:63–9. doi: 10.1016/j.schres.2018.04.011
51. Yamamori H, Hashimoto R, Fujita Y, Numata S, Yasuda Y, Fujimoto M, et al. Changes in plasma D-serine, L-serine, and glycine levels in treatment-resistant schizophrenia before and after clozapine treatment. Neurosci Lett. (2014) 582:93–8. doi: 10.1016/j.neulet.2014.08.052
52. El-Tallawy HN, Saleem TH, El-Ebidi AM, Hassan MH, Gabra RH, Farghaly WM, et al. Clinical and biochemical study of d-serine metabolism among schizophrenia patients. Neuropsychiatr Dis Treat. (2017) 13:1057–63. doi: 10.2147/NDT
53. Yamada K, Ohnishi T, Hashimoto K, Ohba H, Iwayama-Shigeno Y, Toyoshima M, et al. Identification of multiple serine racemase (SRR) mRNA isoforms and genetic analyses of SRR and DAO in schizophrenia and D-serine levels. Biol Psychiatry. (2005) 57:1493–503. doi: 10.1016/j.biopsych.2005.03.018
54. Fukushima T, Iizuka H, Yokota A, Suzuki T, Ohno C, Kono Y, et al. Quantitative analyses of schizophrenia-associated metabolites in serum: serum D-lactate levels are negatively correlated with gamma-glutamylcysteine in medicated schizophrenia patients. PloS One. (2014) 9:e101652. doi: 10.1371/journal.pone.0101652
55. Liu R, Dang W, Du Y, Zhou Q, Liu Z, Jiao K. Correlation of functional GRIN2A gene promoter polymorphisms with schizophrenia and serum D-serine levels. Gene. (2015) 568:25–30. doi: 10.1016/j.gene.2015.05.011
56. Uzun Uysal E, Tomruk NB, Cakir Sen C, Yildizhan E. D-serine and D-amino acid oxidase levels in patients with schizophrenia spectrum disorders in the first episode and 6-month follow-up. J Psychiatr Res. (2024) 175:123–30. doi: 10.1016/j.jpsychires.2024.05.004
57. Morita Y, Ujike H, Tanaka Y, Otani K, Kishimoto M, Morio A, et al. A genetic variant of the serine racemase gene is associated with schizophrenia. Biol Psychiatry. (2007) 61:1200–3. doi: 10.1016/j.biopsych.2006.07.025
58. Labrie V, Fukumura R, Rastogi A, Fick LJ, Wang W, Boutros PC, et al. Serine racemase is associated with schizophrenia susceptibility in humans and in a mouse model. Hum Mol Genet. (2009) 18:3227–43. doi: 10.1093/hmg/ddp261
59. Li Y, Chen YT, Hu J, Wei LM, Gao HL, Xu CT. [Correlation of D-amino acid-oxidase gene polymorphism to schizophrenia]. Nan fang yi ke da xue xue bao = J South Med University. (2010) 30:2142–4.
60. Liu YL, Wang SC, Hwu HG, Fann CS, Yang UC, Yang WC, et al. Haplotypes of the D-amino acid oxidase gene are significantly associated with schizophrenia and its neurocognitive deficits. PloS One. (2016) 11:e0150435. doi: 10.1371/journal.pone.0150435
61. Suliman H, Schumacher J, Becker T, Cichon S, Schulze TG, Propping P, et al. Association study of 20 genetic variants at the (D)-amino acid oxidase gene in schizophrenia. Psychiatr Genet. (2010) 20:82–3. doi: 10.1097/YPG.0b013e3283351244
62. Sacchi S, Bernasconi M, Martineau M, Mothet JP, Ruzzene M, Pilone MS, et al. pLG72 modulates intracellular D-serine levels through its interaction with D-amino acid oxidase: effect on schizophrenia susceptibility. J Biol Chem. (2008) 283:22244–56. doi: 10.1074/jbc.M709153200
63. Sacchi S, Cappelletti P, Giovannardi S, Pollegioni L. Evidence for the interaction of D-amino acid oxidase with pLG72 in a glial cell line. Mol Cell Neurosci. (2011) 48:20–8. doi: 10.1016/j.mcn.2011.06.001
64. Murtas G, Pollegioni L, Molla G, Sacchi S. Biochemical properties and physiological functions of pLG72: twenty years of investigations. Biomolecules. (2022) 12:858. doi: 10.3390/biom12060858
65. Lane HY, Lin CH, Green MF, Hellemann G, Huang CC, Chen PW, et al. Add-on treatment of benzoate for schizophrenia: a randomized, double-blind, placebo-controlled trial of D-amino acid oxidase inhibitor. JAMA Psychiatry. (2013) 70:1267–75. doi: 10.1001/jamapsychiatry.2013.2159
66. Lin CH, Lin CH, Chang YC, Huang YJ, Chen PW, Yang HT, et al. Sodium benzoate, a D-amino acid oxidase inhibitor, added to clozapine for the treatment of schizophrenia: A randomized, double-blind, placebo-controlled trial. Biol Psychiatry. (2018) 84:422–32. doi: 10.1016/j.biopsych.2017.12.006
67. Kantrowitz JT, Epstein ML, Lee M, Lehrfeld N, Nolan KA, Shope C, et al. Improvement in mismatch negativity generation during d-serine treatment in schizophrenia: Correlation with symptoms. Schizophr Res. (2018) 191:70–9. doi: 10.1016/j.schres.2017.02.027
68. Lane HY, Chang YC, Liu YC, Chiu CC, Tsai GE. Sarcosine or D-serine add-on treatment for acute exacerbation of schizophrenia: a randomized, double-blind, placebo-controlled study. Arch Gen Psychiatry. (2005) 62:1196–204. doi: 10.1001/archpsyc.62.11.1196
69. O’Donnell P, Dong C, Murthy V, Asgharnejad M, Du X, Summerfelt A, et al. The D-amino acid oxidase inhibitor luvadaxistat improves mismatch negativity in patients with schizophrenia in a randomized trial. Neuropsychopharmacology. (2023) 48:1–8. doi: 10.1038/s41386-023-01560-0
70. Sacchi S, Rosini E, Pollegioni L, Molla G. D-amino acid oxidase inhibitors as a novel class of drugs for schizophrenia therapy. Curr Pharm Des. (2013) 19:2499–511. doi: 10.2174/1381612811319140002
71. Heresco-Levy U, Javitt DC, Ebstein R, Vass A, Lichtenberg P, Bar G, et al. D-serine efficacy as add-on pharmacotherapy to risperidone and olanzapine for treatment-refractory schizophrenia. Biol Psychiatry. (2005) 57:577–85. doi: 10.1016/j.biopsych.2004.12.037
72. Matveeva TM, Pisansky MT, Young A, Miller RF, Gewirtz JC. Sociality deficits in serine racemase knockout mice. Brain behavior. (2019) 9:e01383. doi: 10.1002/brb3.1383
73. Balu DT, Li Y, Puhl MD, Benneyworth MA, Basu AC, Takagi S, et al. Multiple risk pathways for schizophrenia converge in serine racemase knockout mice, a mouse model of NMDA receptor hypofunction. Proc Natl Acad Sci U S A. (2013) 110:E2400–9. doi: 10.1073/pnas.1304308110
74. Hagiwara H, Iyo M, Hashimoto K. Neonatal disruption of serine racemase causes schizophrenia-like behavioral abnormalities in adulthood: clinical rescue by d-serine. PloS One. (2013) 8:e62438. doi: 10.1371/journal.pone.0062438
75. Errico F, Napolitano F, Squillace M, Vitucci D, Blasi G, de Bartolomeis A, et al. Decreased levels of D-aspartate and NMDA in the prefrontal cortex and striatum of patients with schizophrenia. J Psychiatr Res. (2013) 47:1432–7. doi: 10.1016/j.jpsychires.2013.06.013
76. Nuzzo T, Sacchi S, Errico F, Keller S, Palumbo O, Florio E, et al. Decreased free d-aspartate levels are linked to enhanced d-aspartate oxidase activity in the dorsolateral prefrontal cortex of schizophrenia patients. NPJ Schizophr. (2017) 3:16. doi: 10.1038/s41537-017-0015-7
77. De Rosa A, Fontana A, Nuzzo T, Garofalo M, Di Maio A, Punzo D, et al. Machine Learning algorithm unveils glutamatergic alterations in the post-mortem schizophrenia brain. Schizophr (Heidelb). (2022) 8:8. doi: 10.1038/s41537-022-00231-1
78. Lombardo B, Pagani M, De Rosa A, Nunziato M, Migliarini S, Garofalo M, et al. D-aspartate oxidase gene duplication induces social recognition memory deficit in mice and intellectual disabilities in humans. Transl Psychiatry. (2022) 12:305. doi: 10.1038/s41398-022-02088-5
79. De Rosa A, Mastrostefano F, Di Maio A, Nuzzo T, Saitoh Y, Katane M, et al. Prenatal expression of D-aspartate oxidase causes early cerebral D-aspartate depletion and influences brain morphology and cognitive functions at adulthood. Amino Acids. (2020) 52:597–617. doi: 10.1007/s00726-020-02839-y
80. American Psychiatric Association D-TF. Diagnostic and statistical manual of mental disorders: DSM-5™. 5th ed. Arlington, VA, US: American Psychiatric Publishing, Inc (2013) p. 947–xliv. doi: 10.1176/appi.books.9780890425596
81. Howes OD, McCutcheon R, Agid O, de Bartolomeis A, van Beveren NJ, Birnbaum ML, et al. Treatment-resistant schizophrenia: treatment response and resistance in psychosis (TRRIP) working group consensus guidelines on diagnosis and terminology. Am J Psychiatry. (2017) 174:216–29. doi: 10.1176/appi.ajp.2016.16050503
82. Kay SR, Fiszbein A, Opler LA. The positive and negative syndrome scale (PANSS) for schizophrenia. Schizophr Bull. (1987) 13:261–76. doi: 10.1093/schbul/13.2.261
83. Keefe RS, Goldberg TE, Harvey PD, Gold JM, Poe MP, Coughenour L. The Brief Assessment of Cognition in Schizophrenia: reliability, sensitivity, and comparison with a standard neurocognitive battery. Schizophr Res. (2004) 68:283–97. doi: 10.1016/j.schres.2003.09.011
84. Lord C, Risi S, Lambrecht L, Cook EH Jr., Leventhal BL, DiLavore PC, et al. The autism diagnostic observation schedule-generic: a standard measure of social and communication deficits associated with the spectrum of autism. J Autism Dev Disord. (2000) 30:205–23. doi: 10.1023/A:1005592401947
85. Luiz D, Bernard A, Knoesen N, Kotras H, McAlinden P, O’Connel R. Griffiths mental development scales-extended revised (GMDS-ER) administration manual. Amersham (Bucks, UK: Association for Research in Child Development (2004).
86. Roid GH, Miller LJ. Leiter International Performance Scale-Revised (Leiter-R) manual. Wood Dale, IL: Stoelting (1997). doi: 10.1037/t05120-000
87. Balboni G, Belacchi C, Bonichini S, Coscarelli A. Vineland-II. Vineland Adaptive Behavior Scales Second Edition. (2006), Survey Form-Standardizzazione italiana. Giunti Psychometrics.
88. Sacchi S, Lorenzi S, Molla G, Pilone MS, Rossetti C, Pollegioni L. Engineering the substrate specificity of D-amino-acid oxidase. J Biol Chem. (2002) 277:27510–6. doi: 10.1074/jbc.M203946200
89. Zheng H-F, Wang W-Q, Li X-M, Rauw G, Baker GB. Body fluid levels of neuroactive amino acids in autism spectrum disorders: a review of the literature. Amino Acids. (2017) 49:57–65. doi: 10.1007/s00726-016-2332-y
90. Tirouvanziam R, Obukhanych TV, Laval J, Aronov PA, Libove R, Banerjee AG, et al. Distinct plasma profile of polar neutral amino acids, leucine, and glutamate in children with Autism Spectrum Disorders. J Autism Dev Disord. (2012) 42:827–36. doi: 10.1007/s10803-011-1314-x
91. Shinohe A, Hashimoto K, Nakamura K, Tsujii M, Iwata Y, Tsuchiya KJ, et al. Increased serum levels of glutamate in adult patients with autism. Prog Neuropsychopharmacol Biol Psychiatry. (2006) 30:1472–7. doi: 10.1016/j.pnpbp.2006.06.013
92. Moreno-Fuenmayor H, Borjas L, Arrieta A, Valera V, Socorro-Candanoza L. Plasma excitatory amino acids in autism. Invest Clin. (1996) 37:113–28.
93. Aldred S, Moore KM, Fitzgerald M, Waring RH. Plasma amino acid levels in children with autism and their families. J Autism Dev Disord. (2003) 33:93–7. doi: 10.1023/A:1022238706604
94. Shimmura C, Suda S, Tsuchiya KJ, Hashimoto K, Ohno K, Matsuzaki H, et al. Alteration of plasma glutamate and glutamine levels in children with high-functioning autism. PloS One. (2011) 6:e25340. doi: 10.1371/journal.pone.0025340
95. Naushad SM, Jain JM, Prasad CK, Naik U, Akella RR. Autistic children exhibit distinct plasma amino acid profile. Indian J Biochem Biophys. (2013) 50:474–8.
96. El-Ansary A, Al-Ayadhi L. GABAergic/glutamatergic imbalance relative to excessive neuroinflammation in autism spectrum disorders. J Neuroinflammation. (2014) 11:189. doi: 10.1186/s12974-014-0189-0
97. Cai J, Ding L, Zhang JS, Xue J, Wang LZ. Elevated plasma levels of glutamate in children with autism spectrum disorders. Neuroreport. (2016) 27:272–6. doi: 10.1097/WNR.0000000000000532
98. El-Ansary A. Data of multiple regressions analysis between selected biomarkers related to glutamate excitotoxicity and oxidative stress in Saudi autistic patients. Data Brief. (2016) 7:111–6. doi: 10.1016/j.dib.2016.02.025
99. Moreno H, Borjas L, Arrieta A, Saez L, Prassad A, Estevez J, et al. [Clinical heterogeneity of the autistic syndrome: a study of 60 families]. Invest Clin. (1992) 33:13–31.
100. Tu WJ, Chen H, He J. Application of LC-MS/MS analysis of plasma amino acids profiles in children with autism. J Clin Biochem Nutr. (2012) 51:248–9. doi: 10.3164/jcbn.12-45
101. Good P. Do salt cravings in children with autistic disorders reveal low blood sodium depleting brain taurine and glutamine? Med Hypotheses. (2011) 77:1015–21.
102. Masi A, DeMayo MM, Glozier N, Guastella AJ. An overview of autism spectrum disorder, heterogeneity and treatment options. Neurosci Bull. (2017) 33:183–93. doi: 10.1007/s12264-017-0100-y
103. Errico F, D’Argenio V, Sforazzini F, Iasevoli F, Squillace M, Guerri G, et al. A role for D-aspartate oxidase in schizophrenia and in schizophrenia-related symptoms induced by phencyclidine in mice. Transl Psychiatry. (2015) 5:e512. doi: 10.1038/tp.2015.2
104. Coyle JT, Ruzicka WB, Balu DT. Fifty years of research on schizophrenia: the ascendance of the glutamatergic synapse. Am J Psychiatry. (2020) 177:1119–28. doi: 10.1176/appi.ajp.2020.20101481
105. Hashimoto K. Recent advances in the early intervention in schizophrenia: future direction from preclinical findings. Curr Psychiatry Rep. (2019) 21:75. doi: 10.1007/s11920-019-1063-7
106. D’Souza DC, Gil R, Cassello K, Morrissey K, Abi-Saab D, White J, et al. IV glycine and oral D-cycloserine effects on plasma and CSF amino acids in healthy humans. Biol Psychiatry. (2000) 47:450–62. doi: 10.1016/S0006-3223(99)00133-X
107. Hashimoto A, Chiba S. Effect of systemic administration of D-serine on the levels of D- and L-serine in several brain areas and periphery of rat. Eur J Pharmacol. (2004) 495:153–8. doi: 10.1016/j.ejphar.2004.05.036
108. Bauer D, Hamacher K, Broer S, Pauleit D, Palm C, Zilles K, et al. Preferred stereoselective brain uptake of d-serine–a modulator of glutamatergic neurotransmission. Nucl Med Biol. (2005) 32:793–7. doi: 10.1016/j.nucmedbio.2005.07.004
109. Sacchi S, Novellis V, Paolone G, Nuzzo T, Iannotta M, Belardo C, et al. Olanzapine, but not clozapine, increases glutamate release in the prefrontal cortex of freely moving mice by inhibiting D-aspartate oxidase activity. Sci Rep. (2017) 7:46288. doi: 10.1038/srep46288
110. Suzuki M, Sujino T, Chiba S, Harada Y, Goto M, Takahashi R, et al. Host-microbe cross-talk governs amino acid chirality to regulate survival and differentiation of B cells. Sci Adv. (2021) 7:eabd6480. doi: 10.1126/sciadv.abd6480
111. Murphy CE, Walker AK, Weickert CS. Neuroinflammation in schizophrenia: the role of nuclear factor kappa B. Transl Psychiatry. (2021) 11:528. doi: 10.1038/s41398-021-01607-0
112. Melkersson K, Lewitt M, Hall K. Higher serum concentrations of tyrosine and glutamate in schizophrenia patients treated with clozapine, compared to in those treated with conventional antipsychotics. Neuro Endocrinol Lett. (2015) 36:465–80.
113. Tanahashi S, Yamamura S, Nakagawa M, Motomura E, Okada M. Clozapine, but not haloperidol, enhances glial D-serine and L-glutamate release in rat frontal cortex and primary cultured astrocytes. Br J Pharmacol. (2012) 165:1543–55. doi: 10.1111/j.1476-5381.2011.01638.x
114. Seeman MV. The gut microbiome and antipsychotic treatment response. Behav Brain Res. (2021) 396:112886. doi: 10.1016/j.bbr.2020.112886
115. Vasileva SS, Yang Y, Baker A, Siskind D, Gratten J, Eyles D. Associations of the gut microbiome with treatment resistance in schizophrenia. JAMA Psychiatry. (2024) 81:292–302. doi: 10.1001/jamapsychiatry.2023.5371
116. Hesaka A, Sakai S, Hamase K, Ikeda T, Matsui R, Mita M, et al. (D)-Serine reflects kidney function and diseases. Sci Rep. (2019) 9:5104. doi: 10.1038/s41598-019-41608-0
117. Kimura T, Hesaka A, Isaka Y. D-Amino acids and kidney diseases. Clin Exp Nephrol. (2020) 24:404–10. doi: 10.1007/s10157-020-01862-3
118. Carswell C, Cogley C, Bramham K, Chilcot J, Noble H, Siddiqi N. Chronic kidney disease and severe mental illness: a scoping review. J Nephrol. (2023) 36:1519–47. doi: 10.1007/s40620-023-01599-8
119. Loureiro CM, da Roza DL, Corsi-Zuelli F, Shuhama R, Fachim HA, Simoes-Ambrosio LMC, et al. Plasma amino acids profile in first-episode psychosis, unaffected siblings and community-based controls. Sci Rep. (2020) 10:21423. doi: 10.1038/s41598-020-78559-w
120. Palomino A, Gonzalez-Pinto A, Aldama A, Gonzalez-Gomez C, Mosquera F, Gonzalez-Garcia G, et al. Decreased levels of plasma glutamate in patients with first-episode schizophrenia and bipolar disorder. Schizophr Res. (2007) 95:174–8. doi: 10.1016/j.schres.2007.06.012
121. Krivoy A, Hochman E, Sendt KV, Hollander S, Vilner Y, Selakovic M, et al. Association between serum levels of glutamate and neurotrophic factors and response to clozapine treatment. Schizophr Res. (2018) 192:226–31. doi: 10.1016/j.schres.2017.05.040
Keywords: D-serine, D-aspartate, treatment-resistant, antipsychotics, schizophrenia, autism spectrum disorder
Citation: Garofalo M, De Simone G, Motta Z, Nuzzo T, De Grandis E, Bruno C, Boeri S, Riccio MP, Pastore L, Bravaccio C, Iasevoli F, Salvatore F, Pollegioni L, Errico F, de Bartolomeis A and Usiello A (2024) Decreased free D-aspartate levels in the blood serum of patients with schizophrenia. Front. Psychiatry 15:1408175. doi: 10.3389/fpsyt.2024.1408175
Received: 27 March 2024; Accepted: 17 June 2024;
Published: 10 July 2024.
Edited by:
Aye-Mu Myint, Maastricht University, NetherlandsReviewed by:
Lorenz S. Neuwirth, State University of New York at Old Westbury, United StatesHsien-Yuan Lane, China Medical University, Taiwan
Jean-Pierre Mothet, Université Paris-Saclay, France
Copyright © 2024 Garofalo, De Simone, Motta, Nuzzo, De Grandis, Bruno, Boeri, Riccio, Pastore, Bravaccio, Iasevoli, Salvatore, Pollegioni, Errico, de Bartolomeis and Usiello. This is an open-access article distributed under the terms of the Creative Commons Attribution License (CC BY). The use, distribution or reproduction in other forums is permitted, provided the original author(s) and the copyright owner(s) are credited and that the original publication in this journal is cited, in accordance with accepted academic practice. No use, distribution or reproduction is permitted which does not comply with these terms.
*Correspondence: Francesco Errico, ZnJhbmNlc2NvLmVycmljb0B1bmluYS5pdA==; Andrea de Bartolomeis, YWRlYmFydG9AdW5pbmEuaXQ=
†These authors have contributed equally to this work