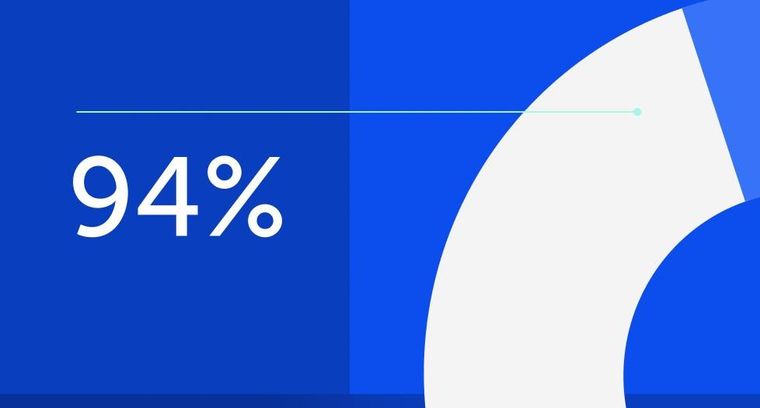
94% of researchers rate our articles as excellent or good
Learn more about the work of our research integrity team to safeguard the quality of each article we publish.
Find out more
ORIGINAL RESEARCH article
Front. Psychiatry, 06 June 2024
Sec. Autism
Volume 15 - 2024 | https://doi.org/10.3389/fpsyt.2024.1403476
This article is part of the Research TopicEffects of Autism Spectrum Disorder (ASD) Risk Genes on Phenotypes of Each HierarchyView all 5 articles
Background: Social isolation during critical periods of development is associated with alterations in behavior and neuronal circuitry. This study aimed to investigate the immediate and developmental effects of social isolation on firing properties, neuronal activity-regulated pentraxin (NARP) and parvalbumin (PV) expression in the prefrontal cortex (PFC), social behavior in juvenile socially isolated mice, and the biological relevance of NARP expression in autism spectrum disorder (ASD).
Methods: Mice were subjected to social isolation during postnatal days 21–35 (P21–P35) and were compared with group-housed control mice. Firing properties in the PFC pyramidal neurons were altered in P35 socially isolated mice, which might be associated with alterations in NARP and PV expression.
Results: In adulthood, mice that underwent juvenile social isolation exhibited difficulty distinguishing between novel and familiar mice during a social memory task, while maintaining similar levels of social interaction as the control mice. Furthermore, a marked decrease in NARP expression in lymphoblastoid cell lines derived from adolescent humans with ASD as compared to typically developing (TD) humans was found.
Conclusion: Our study highlights the role of electrophysiological properties, as well as NARP and PV expression in the PFC in mediating the developmental consequences of social isolation on behavior.
Neural networks exhibit heightened susceptibility to modulation by cellular activity patterns during the postnatal developmental stage, denoted as the critical period (1). This period, which represents a transient time window for the developing brain, is characterized by the rewiring and consolidation of neuronal networks in response to environmental stimuli (1). Indeed, early life experiences trigger intrinsic mechanisms within a critical period to enhance cortical synaptic plasticity, culminating in enduring large-scale alterations in adult brain systems (2–4). Prior research has demonstrated that aberrant juvenile social encounters, such as neglect or social rejection, exert persistent effects on the structure and function of the prefrontal cortex (PFC), a brain region that plays a central role in higher-level cognitive processes in both rodents (4–8) and humans (9, 10). Notably, these detrimental effects remain resistant to amelioration through subsequent human foster care (9) and rodent resocialization (5). Consequently, the impact of such stress on the PFC circuitry may hold significant relevance during the adolescent phase and could serve as a predisposing element for the emergence or amplification of psychiatric conditions, such as autism spectrum disorder (ASD) and schizophrenia (11–13).
There are two principal neuronal classifications within the cerebral cortex: glutamatergic excitatory pyramidal neurons and GABAergic inhibitory interneurons. Studies utilizing genetically or pharmacologically manipulated mice have primarily focused on the role of inhibitory interneurons during this critical period. In particular, parvalbumin-expressing (PV) neurons, which comprise the most abundant subclass of inhibitory interneurons (14, 15), appear to be intimately involved in critical period plasticity, as PV neurons act as powerful regulators of excitatory pyramidal neuron activity, maintaining an appropriate dynamic range of cortical excitation (16). Furthermore, multiple lines of evidence suggest that the dysfunction of PV neurons within the PFC is implicated in various psychiatric disorders (17–21). Collectively, elucidating the cellular mechanisms driving the developmental plasticity of the prefrontal excitatory and inhibitory circuitry during adolescence is essential for public mental health initiatives and facilitates the development of preventative strategies and novel therapeutic approaches.
We have previously demonstrated that a two-week period of social isolation following weaning induces alterations in the electrophysiological properties of excitatory and inhibitory neurons within the medial PFC of adult mice (7, 8). Furthermore, an immediate influence on specific subtypes of deep-layer excitatory pyramidal and inhibitory neurons at the end of the two-week juvenile social isolation phase was observed (6). However, the extent to which these initial effects on excitatory and inhibitory neurons are accompanied by physiological and molecular changes across the PFC remains poorly understood. Sensory experiences foster the growth and maturation of neuronal circuits, partly by stimulating activity-dependent gene transcription (22). Therefore, neuronal activity-regulated pentraxin (NARP), one of the immediate early gene products, may be one potential molecular factor that affects such alterations following exposure to social isolation during this critical period. NARP is prominently secreted from excitatory pyramidal neurons in an activity-dependent manner and helps in the development of excitatory synapses exclusively on PV neurons (23). Generally, excitatory inputs from pyramidal neurons stimulate PV neurons, which in turn provide feedback inhibition to the pyramidal neurons (24). Consequently, the function of NARP is believed to play a vital role in maintaining cortical excitatory and inhibitory circuitry during critical periods of developmental plasticity (25). Additionally, given the alterations in NARP expression within the PFC in schizophrenia (26), mood disorders (26), and Alzheimer’s disease (27), the NARP gene product may prove integral to the modified properties of excitatory and inhibitory neurons resulting from aberrant juvenile social experiences.
In the present study, using electrophysiological, gene, and protein expression, and behavioral analyses, we explored the timing and molecular processes through which the prefrontal excitatory and inhibitory circuitry is altered in socially isolated mice, with a focus on the expression and function of NARP. Finally, using human blood samples, potential alterations in NARP expression in lymphoblastoid cell line (LCL) samples from patients with ASD were assessed.
C57BL/6J mice were used for all experimental procedures, without duplications between experiments. The animals were housed in our animal facility under standard conditions with a 12-hour light-dark cycle and had libitum access to food and water. Following a previously established protocol (7), a cohort of four male littermates was randomly assigned to either one isolated mouse or three group-housed mice after weaning on postnatal day 21 (P21). The isolated mice were individually housed in cages from P21 to P35 (jSI). GH mice served as the control group for typically developing animals. During the re-socialization period, each isolated mouse was housed with its three littermates until experiments were conducted between P63 and P70. Consequently, the following experiments were conducted to evaluate the effect of social isolation at P35 and/or during adulthood (P63–70). All experiments were approved by the Animal Care and Use Committee of Nara Medical University and conducted according to their guidelines.
All study participants and their legal guardians provided written informed consent before enrollment. ASD was diagnosed by two experienced child psychiatrists using the criteria outlined in the Diagnostic and Statistical Manual of Mental Disorders 5th edition (DSM-5) and clinical interviews were conducted. The Structured Clinical Interview for DSM-5 was utilized to thoroughly assess any personal or family history of past or present mental illness.
Peripheral blood mononuclear cells (PBMCs) were isolated from venous whole blood collected from 29 TD subjects, averaging 12.0 (SD 2.3) years in age, and from 32 subjects diagnosed with ASD, averaging 11.7 (SD 2.7) years in age (Supplementary Table 1). All LCLs were developed by infecting PBMCs with Epstein-Barr virus produced in the supernatant of cultured B95–8 cells, as described previously (28). This study was approved by the Nara Medical University Ethics Committee and was conducted in accordance with the Declaration of Helsinki.
Brain slices, including the medial PFC, were prepared from P35 mice (n = 21 cells from 3 mice; GH, n = 24 cells from 3 mice; jSI). The brain was quickly removed under anesthesia with isoflurane and immersed in an ice-cold sucrose-based solution bubbled with a mixed gas of 95% O2/5% CO2 containing (in mM) 230 sucrose, 2.5 KCl, 25 NaHCO3, 1.25 NaH2PO4, 0.5 CaCl2, 10 MgSO4, and 10 D-glucose. The frontal cortex was sectioned into 300–330 μm-thick slices in the coronal plane by a vibrating tissue slicer (Linear Slicer Pro 7, Dosaka). Slices were incubated for at least 60 min in a chamber filled with a standard artificial cerebrospinal fluid (ACSF) continuously bubbled with mixed gas, containing (in mM) 125 NaCl, 2.5 KCl, 25 NaHCO3, 1.25 NaH2PO4, 2 CaCl2, 1 MgCl2, and 25 D-glucose at 32°C, and then maintained in the ACSF at 25°C. Pyramidal cells in the medial PFC layer 3 were current-clamped in the conventional whole-cell configuration using a Multiclamp 700A amplifier (Axon Instruments). Patch pipettes were pulled from the borosilicate glass and filled with an intracellular solution containing (in mM) 141 K-gluconate, 4 KCl, 2 MgCl2, 2 Mg-ATP, 0.3 Na-GTP, 0.2 EGTA, 10 HEPES, pH adjusted to 7.25 with KOH. All membrane potentials were corrected for a 13 mV liquid junction potential, as described previously (29). Data acquisition and stimulation were controlled using Signal 4 software with Power 1401 interface equipment (Cambridge Electronics Design).
For the current-clamp recordings, the series resistance was monitored and compensated using a bridge circuit, and the pipette capacitance was compensated. The voltage signals were low-pass filtered at 10 kHz and digitized at 20 kHz. The baseline membrane potential was maintained near -70 mV with current injection. To examine the action potential and subthreshold membrane properties, we recorded the membrane potential responses to depolarizing current pulses from 10–100 pA in 10 pA increments (500-ms duration). The spike threshold was calculated as the voltage at which the slope of the action potential trace reached 10 mV/ms at the rheobase, defined as the minimum current value that elicited at least one action potential. Spike amplitude and spike frequency were defined as the voltage and frequencies of the spikes in response to depolarizing 100 pA current injections from baseline, respectively.
Mouse brains were quickly removed from the skull, and medial PFC sections (Bregma +2.8 mm to +2.1 mm) were microdissected for sample preparations (n = 7; each group at P35, and n = 7; each group at adulthood). From samples of mouse tissues and human LCLs, total RNA was isolated from TRIzol homogenates and purified using the Direct-Zol RNA Miniprep kit (Zymo Research, Irvine, CA, USA) according to the manufacturer’s instructions. First-strand cDNA was synthesized using an iScript kit (Bio-Rad Laboratories, Hercules, CA, USA), and qPCR was performed using SYBR Premix Ex Taq II (Tli RNase H Plus, TAKARA BIO Inc., Otsu, Shiga, Japan) in a StepOne Plus real-time PCR system (Life Technologies, Carlsbad, CA). The specificity of amplification was confirmed by monitoring the dissociation curve at the end of each run. All primer sets (Supplementary Table 2) had amplification efficiency ≥ 96% confirmed by standard curve method. Normalization and relative quantification of the expression levels of the target genes were determined by the ΔCT method, using the constitutively expressed genes β-actin (ACTB), glyceraldehyde-3-phosphate dehydrogenase (GAPDH), and cyclophilin (Cyclo) in rodent brains and human LCLs (Supplementary Table 2). Furthermore, the levels of these three transcripts in the medial PFC were not affected by age or housing conditions.
The medial PFC sections (Bregma +2.8 mm to +2.1 mm) from mice (n = 7; each group at P35, and n = 7; each group at adulthood) were microdissected, and total protein was immediately isolated using ice-cold homogenizing buffer [50 mM Tris-HCl buffer (pH 7.5), 8 M urea, 0.1 M NaCl, 2 mM EDTA, 1 mM dithiothreitol (DTT)] with cOmplete™ protease inhibitor cocktail (Roche, Mannheim, Germany). The total protein concentration was determined in triplicate using a BCA assay kit (Thermo Fisher Scientific, Rockford, IL, USA), according to the manufacturer’s instructions. Western blotting was performed with the Simple Western™ system, employing capillary electrophoresis to automatically detect and quantify a protein of interest (ProteinSimple, San Jose, CA). In brief, according to the manufacturer’s instructions (ProteinSimple, San Jose, CA), lysates of medial PFC sections (1.5 mg/mL), primary antibodies to Cofilin (#5175; Cell Signaling Technology), NPTX2 (10889–1-AP; Proteintech), and PV (AF5058; R&D Systems) with secondary antibodies were loaded into either 12–230 kDa or 2–20 kDa Separation Module (ProteinSimple) depending on the protein’s molecular weight to run on the Simple Western machine, Wes™ (ProteinSimple). The digital image was analyzed using Compass software, where the quantified data of the detected protein were reported as molecular weight and signal/peak intensity. The expression level of each protein was calculated based on its abundance relative to that of cofilin.
The sociability and social recognition memory of test subjects were measured using a three-chamber test (n = 11; GH, n = 11; jSI). The apparatus consisted of an acrylic open-topped box (60 cm L × 40 cm W × 40 cm H) partitioned into three chambers with two acrylic walls. Each 10-minute phase was initiated by allowing the test mice to freely explore the 3-chamber apparatus for habituation. During the sociability phase, the test mouse was directed to the center chamber, while a wired cup containing a strange (stranger 1) mouse and an empty cup was introduced into the other two chambers. Subsequently, during the social recognition phase, another strange mouse (stranger 2) was introduced into the empty cup, and the movement of the subject mouse was tracked for another 10 min. To counterbalance any possible bias, the orientation of the wired cups containing strangers 1 or 2 (empty) was randomized for each set of experiments. The time spent in each chamber and the time spent exploring the enclosed novel mice or empty cups (novel objects) were recorded using an overhead-mounted camera and analyzed using an automated tracking program (TopScan, Clever Sys Inc.) during the first 4 min of each session (30), with comprehensive data encompassing the entire 10-minute sessions provided in the Supplementary Figure 1. To minimize individual differences, the sociability index was calculated as (time spent exploring the stranger 1)/(time spent exploring the stranger 1 + time spent exploring the empty cup), while the social recognition index was calculated as (time spent exploring the stranger 2)/(time spent exploring the stranger 2 + time spent exploring the familiar).
Statistical analyses were performed using the software Prism 8.20 (GraphPad Software Inc., CA, USA). For qPCR analyses, statistical significance was determined using two-way analysis of variance (ANOVA), followed by Tukey’s test. Otherwise, the Student’s t-test or Welch’s t-test was applied based on the outcomes of a test for the equality of variances. All data are presented as mean ± standard error of the mean (SEM), and differences between group means were considered significant if p values showed less than 0.05.
We previously reported that juvenile social isolation (jSI) from postnatal day 21 to 35 (P21–P35) induced long-lasting working memory deficits and altered the electrophysiological properties of excitatory and inhibitory neurons in the adult medial PFC (5, 7, 8). Therefore, we first explored the functional abnormalities that arise after exposure to social isolation (P35) in layer 3 pyramidal neurons, which are considered crucial neuronal substrates for working memory. As shown in Figures 1A, B, whole-cell current-clamp recordings were performed on medial PFC pyramidal cells in jSI mice at P35. jSI mice had significantly higher spike thresholds than group-housed (GH) mice of the same age (Figure 1C; p < 0.0001). Furthermore, the spike amplitude in jSI mice was significantly smaller than that in GH mice (Figure 1D; p = 0.010), although the spike frequency in excitatory pyramidal cells did not differ between the two groups (Figure 1E; p = 0.973). Recently, we uncovered reduced excitability of a specific subtype of pyramidal neurons in the deep layer of the medial PFC after jSI at P35 (6). Considering the differences in properties and connections to multiple intracortical and subcortical targets between pyramidal neurons in layers 3 and 5, electrophysiological alterations in medial PFC excitatory neurons might be one of the crucial contributors to the subsequent dysfunction of the entire medial PFC after exposure to social isolation.
Figure 1 Changes in the excitability of medial PFC pyramidal cells following juvenile social isolation. (A) Schematic diagram illustrating juvenile social isolation. Mice underwent social isolation between P21 and P35, with whole-cell patch-clamp recordings performed at P35. (B) Top: Current-clamp recordings from pyramidal neurons in the medial PFC layer 3 conducted at P35. Bottom: Representative action potential traces from GH and jSI mice. The top traces depict a spike at the rheobase, while the bottom traces show a spike with a 100 pA current injection. (C) jSI mice exhibited significantly higher spike thresholds compared to GH mice [two-tailed t-test, t43 = 4.571, p < 0.0001, n = 21 (GH), n = 24 (jSI)]. (D) The spike amplitude in jSI mice was significantly smaller than in GH mice [two-tailed t-test, t43 = 2.680, p = 0.0104, n = 21 (GH), n = 24 (jSI)]. (E) There was no significant difference in spike frequency between GH and jSI mice [two-tailed t-test, t43 = 0.03442, p = 0.9727, n = 21 (GH), n = 24 (jSI)]. PFC, Prefrontal Cortex; jSI, Juvenile Social Isolation; GH, Group-Housed; P21, Postnatal Day 21; P35, Postnatal Day 35. * 0.01 ≤ p < 0.05; **** p < 0.0001.
As previously mentioned, NARP secreted from pyramidal cells influences the activity of PV neurons, which are required for homeostatic synapse scaling (22). Considering the dampened firing properties observed in both layer 3 (Figure 1) and the specific subtype of layer 5 pyramidal neurons (6–8) within the medial PFC after jSI, we hypothesized that jSI influences the expressions of NARP and PV in the medial PFC during brain development (Figure 2A). Using quantitative PCR (qPCR), we found that the mRNA levels of NARP and PV in the medial PFC exhibited distinct expression patterns in jSI mice compared to GH mice (Figures 2B, E). Indeed, the developmental trajectories of NARP and PV mRNA expression in GH mice aligned with trends in prior studies (26, 31–33). However, NARP mRNA levels were significantly influenced by housing condition (2-way ANOVA: p = 0.04) and interaction effects (2-way ANOVA: p = 0.016), with a trend effect for age (2-way ANOVA: p = 0.07). Tukey’s post-hoc analyses revealed that jSI mice at P35 had lower NARP mRNA levels than GH mice of the same age (p = 0.013). In contrast, PV mRNA levels were significantly influenced by housing conditions (2-way ANOVA: p = 0.002) and showed a trend with age (2-way ANOVA: p = 0.06). Tukey’s post-hoc analyses showed that jSI mice had lower PV mRNA levels than GH mice in adulthood (p = 0.036). Western blot analysis confirmed that the protein levels of NARP, but not PV, were significantly lower at P35 in jSI mice than in GH mice (Figures 2C, D, p = 0.037). Moreover, the protein levels of PV, but not NARP, were significantly lower in jSI mice than in GH mice during adulthood (Figures 2F, G, p = 0.005). These data suggest that relatively lower levels of NARP during the critical period may subsequently affect PV expression in the adult medial PFC, which may contribute to the altered electrophysiological properties of prefrontal excitatory and inhibitory neurons in adult jSI mice.
Figure 2 Altered NARP expression impacts the expression of parvalbumin in inhibitory neurons in socially isolated mice. (A) Schematic diagram of molecular and behavioral analyses. Molecular analysis was conducted at P35 and adulthood, and behavioral analysis was conducted in adulthood. (B) NARP mRNA levels in the medial PFC of jSI mice were lower than those in GH mice at P35, but not at adult [2-way ANOVA, age (P35 and adult) x housing (GH and jSI) interaction F(1. 24) = 6.677 p = 0.0163, age F(1. 24) = 3.505 p = 0.0734, housing F(1. 24) = 4.600 p = 0.0423; Tukey test p = 0.0135 (GH vs jSI at P35), n = 7 (GH, P35), n = 7 (jSI, P35), n = 7 (GH, adult), n = 7 (jSI, adult)]. (C) NARP protein levels in the medial PFC of jSI mice were lower than those in GH mice at P35 (two-tailed t-test, t12 = 2.348, p = 0.0369). (D) Representative images of NARP protein expression in the medial PFC of GH and jSI mice. (E) PV mRNA levels in the medial PFC of jSI mice were lower than those in GH mice at adult, but not at P35 [2-way ANOVA, age (P35 and adult) x housing (GH and jSI) interaction F(1. 24) = 0.4791, p = 0.4955; age F(1. 24) = 3.821, p = 0.0624; housing F(1. 24) = 11.71, p = 0.0022; Tukey test p = 0.0361 (GH vs jSI at adult), n = 7 (GH, P35), n = 7 (jSI, P35), n = 7 (GH, adult), n = 7 (jSI, adult)]. (F) PV protein levels in the medial PFC of jSI mice were lower than those in GH mice at adult (two-tailed t-test, t12 = 3.440, p = 0.0049). (G) Representative images of PV protein expression in the medial PFC of GH and jSI mice. NARP, Neuronal Activity-Regulated Pentraxin; PV, Parvalbumin; PFC, Prefrontal Cortex; GH, Group-Housed; jSI, Juvenile Social Isolation; P35, Postnatal Day 35; Adult/Adulthood, Postnatal Day 63–70; ANOVA, Analysis of Variance. * 0.01 ≤ p < 0.05; ** 0.001 ≤ p < 0.01.
Numerous lines of evidence from human neuroimaging and rodent studies (34–38) have suggested that the evolutionarily conserved medial PFC is a component of a network governing social behavior. Therefore, we examined whether NARP-related functional alterations in the prefrontal excitatory and inhibitory circuitry in socially isolated mice could affect the social behavior of adult jSI mice. In a three-chamber social preference assay, jSI mice showed social interactions comparable to those of their GH littermates (Figures 3A–C, p = 0.19). During the social memory task, GH mice spent significantly more time exploring the novel stranger mice. However, jSI mice failed to differentiate between the novel stranger and familiar mice (Figures 3D–F, p = 0.0003). The behavioral deficits in adult jSI mice remained consistent throughout the entire 10-minute observation period (Supplementary Figure 1). This disparity in response to the novel stranger mouse cannot be attributed to motor or activity anomalies, as both GH and jSI mice exhibited similar locomotor activity during the first 10-minute phase in the three-chamber apparatus (Supplementary Figure 2, p = 0.36). These findings suggest that social isolation during the critical period influences certain aspects of social behavior in adult mice.
Figure 3 Social isolation during the critical period affects behavior in adult mice. (A) Schematic diagram of the social preference assay. (B) No significant differences in investigation time of social and object contacts were observed between GH and jSI mice [2-way ANOVA, contact (social and object) x housing (GH and jSI) interaction F(1. 40) = 3.050, p = 0.0884; contact F(1. 40) = 14.76, p = 0.0004; housing F(1. 40) = 0.00076, p = 0.9781, n = 11 (GH), n = 11 (jSI)]. (C) No significant difference in social preference was observed between GH and jSI mice [two-tailed t-test, t20 = 1.344, p = 0.1939, n = 11 (GH), n = 11 (jSI)]. (D) Schematic diagram of the social recognition assay. (E) Time spent in contact with a novel stranger by juvenile socially isolated (jSI) mice was significantly lower than that by group-housed (GH) mice [2-way ANOVA, contact (novelty and familiar) x housing (GH and jSI) interaction F(1. 40) = 13.20, p = 0.0008; contact F(1. 40) = 0.4613, p = 0.5009; housing F(1. 40) = 0.1073, p = 0.7449; Tukey test p = 0.0376 (GH vs jSI in familiar), n = 11 (GH), n = 11 (jSI)]. (F) Social recognition in jSI mice was significantly lower than in GH mice [two-tailed t-test, t20 = 3.390, p = 0.0029, n = 11 (GH), n = 11 (jSI)]. GH, Group-Housed; jSI, Juvenile Social Isolation; S, Social; O, Object; N, Novelty; F, Familiar; ANOVA, Analysis of Variance. * 0.01 ≤ p < 0.05; ** 0.001 ≤ p < 0.01.
These data underscore the significant involvement of the NARP gene in the neuronal and behavioral aspects of socially isolated mice. Obtaining neurons or neuronal cells from living human subjects is challenging. However, previous studies have suggested that LCLs can be used to identify biologically plausible links between candidate genes and various psychiatric disorders (39–41), including ASD (42). Therefore, we assessed the NARP mRNA levels in LCLs derived from adolescents with ASD and typically developing (TD) adolescents. As shown in Figure 4, a marked decrease in NARP expression in individuals with ASD compared to TD individuals (p = 0.047) was identified. Since social isolation is considered a critical factor in the development or exacerbation of ASD and is common among patients with ASD, these data suggest that alterations in NARP expression may contribute to the pathophysiology of ASD.
Figure 4 Lower NARP gene expression in ASD LCLs may relate to ASD pathophysiology. Top: Schematic diagram of NARP mRNA assay in LCLs from adolescents with Typically Developing (TD) individuals and those with ASD. Bottom: NARP mRNA expression was lower in ASD LCLs than in TD LCLs [two-tailed t-test, t59 = 2.172, p = 0.0339, n = 29 (TD), n = 32 (ASD)]. NARP, Neuronal Activity-Regulated Pentraxin; ASD, Autism Spectrum Disorder; LCLs, Lymphoblastoid Cell Lines; TD, Typically Developing. * 0.01 ≤ p < 0.05.
In the present study, using a comprehensive approach that integrates electrophysiological, gene and protein expression, and behavioral analyses, we investigated the timing and molecular mechanisms underlying the changes in prefrontal excitatory and inhibitory circuitry in socially isolated mice. After juvenile social isolation at P35, we found that it immediately affected the excitability of medial PFC layer 3 pyramidal cells, potentially contributing to subsequent dysfunction in the adult medial PFC. Furthermore, the altered expression of NARP, an immediate early gene product, appeared to influence the expression of PV neurons in socially isolated mice, which might affect the electrophysiological properties of prefrontal excitatory and inhibitory neurons in adult mice. We also observed that while socially isolated mice exhibited similar levels of social interaction as control mice, they had difficulty distinguishing between novel and familiar mice during the social memory task, indicating that social isolation during the critical period affects certain aspects of social behavior in adult mice. Finally, given the social behavioral deficits in individuals with ASD, decreased NARP expression in ASD LCLs might indicate its potential involvement in ASD pathophysiology. Collectively, we suggest the importance of the role of the NARP gene in the neuronal and behavioral features of socially isolated mice and its potential association with psychiatric disorders, such as ASD.
Our electrophysiological study revealed that social isolation during the critical period can immediately lead to changes in the firing properties of pyramidal neurons in layers 3 and 5 of the medial PFC, which in turn may affect the functional properties and communication within the cortical circuitry. Previous studies have shown that layer 3 pyramidal neurons, which are generally smaller than layer 5 pyramidal neurons (43), are involved in the integration and processing of information within the medial PFC. These neurons primarily project to other cortical areas, including the contralateral medial PFC and other association cortices, and thus play a crucial role in intracortical communication (44). In contrast, layer 5 pyramidal neurons, with their distinct electrophysiological properties, contribute to the output of the medial PFC to subcortical structures (45). This diverse innervation pattern allows layer 5 neurons to modulate various behavioral and cognitive processes. Furthermore, the reciprocal connections between layers 3 and 5 neurons play a crucial role in the encoding, integration, and processing of information within the medial PFC (46). Alterations in the intrinsic properties of neurons in these layers due to social isolation during the critical period may disrupt the functional balance and communication between these layers. Collectively, this disruption might impair the overall functionality of the medial PFC, which is essential for higher-level cognitive processes such as working memory and social behavior. However, our current study does not directly track the long-term persistence of these electrophysiological alterations. Several lines of evidence suggest that social isolation during a critical period could have long-lasting effects on several behavioral deficits (47, 48) and electrophysiological changes, particularly in layer 5 pyramidal neurons (6, 8, 49, 50). Therefore, further longitudinal studies are necessary to explicitly trace the electrophysiological changes in layer 3 pyramidal neurons from the juvenile period into adulthood.
Altered expression of NARP, an immediate early gene product that appears to influence the expression of PV in socially isolated mice at P35 was observed. This alteration may be associated with the electrophysiological properties of prefrontal excitatory and inhibitory neurons in socially isolated adult mice. NARP is an activity-dependent gene product that is predominantly secreted by excitatory pyramidal neurons, such as those found in layers 3 and 5 and is involved in the development of excitatory synapses exclusively in PV neurons (23). The significant effect of housing conditions on NARP mRNA expression suggests that social isolation during the critical period may disrupt the normal activity-dependent regulation of NARP expression in these pyramidal neurons. This disruption could potentially lead to altered development of excitatory synapses in PV neurons, which in turn might affect the balance between excitation and inhibition in the cortical circuitry during critical periods of developmental plasticity (32, 51). Furthermore, given the role of PV neurons in regulating the activity of excitatory pyramidal neurons, including those in layers 3 and 5, decreased PV expression in jSI mice may result in an imbalance between excitation and inhibition within the prefrontal circuitry, potentially contributing to medial PFC dysfunction during adulthood. However, the present study revealed that NARP and PV mRNA expression in the medial PFC of jSI exhibited distinctive patterns when compared to the GH. Although NARP has been implicated as a crucial regulator of excitatory and inhibitory synaptic scaling, particularly in PV neurons (23), the lack of a direct correlation between NARP and PV expression in our study suggests that additional molecular factors may be involved in regulating the balance between excitation and inhibition in the medial PFC of socially isolated mice. Alternatively, recent studies have identified various subtypes of inhibitory interneurons, including PV neurons, based on their morphoelectric and transcriptomic profiles (52, 53). Considering our previous finding that electrophysiological functions of a specific PV neuron subtype were immediately altered following social isolation at P35 (6), the observed PV expression levels at P35 might partially reflect a composite effect involving multiple PV neuron subtypes. Therefore, further investigations are required to elucidate the underlying molecular mechanisms and environmental factors that contribute to the observed changes in the excitatory and inhibitory circuits in the medial PFC of socially isolated mice.
In this study, we found that social isolation during the critical period affects certain aspects of social behavior in adult mice, specifically their ability to distinguish between novel and familiar mice during social memory tasks. Previous studies have demonstrated that the medial PFC plays a crucial role in social memory processing (54, 55). Therefore, the electrophysiological alterations observed in our study, particularly in medial PFC pyramidal neurons, could potentially contribute to the social memory deficits observed in socially isolated mice. However, research on the effects of social isolation during critical periods has produced mixed results regarding social behavior, including both reduced and intact social interaction (56–58). In our study, socially isolated mice exhibited comparable levels of social interaction to control mice. Given that perturbations in synaptic levels, such as spine density or gene expression, might also yield varied findings (48, 59), this discrepancy might be attributed to differences in experimental design, housing conditions, or the specific age at which isolation occurred. We suggest that the effects of social isolation on social behavior are complex and may vary depending on the framework or paradigm of social behavior examined. It would be interesting to investigate whether the mode of re-socialization, as studied by Makinodan et al. (58), influences the electrophysiological and molecular changes observed in socially isolated mice.
Given that excitatory and inhibitory synaptic dysfunction is considered a shared pathophysiological mechanism in psychiatric disorders, including ASD (60, 61), the significant reduction in NARP expression in LCLs from adolescents with ASD supports the hypothesis that NARP may play a role in the development or exacerbation of ASD symptoms. Although LCLs are not neuronal cells, several studies have demonstrated altered gene expression profiles in LCLs derived from individuals with ASD compared to TD (62–65). These findings suggest that LCLs may serve as a useful model for investigating molecular changes associated with ASD. Further investigation is required to confirm the role of NARP in the pathophysiology of ASD and to explore whether modulating NARP expression or function could be a potential therapeutic target for treating ASD and related conditions.
Our study has a few limitations. Firstly, our analysis focused solely on the medial PFC, an important brain region in the regulation of social behaviors (34); however, it is not the only brain region involved in these processes. Other regions, such as the amygdala (66), hippocampus (67, 68), and other cortical areas (69, 70), also play crucial roles in social behavior and cognition. Future studies employing optogenetic and chemogenetic approaches could explore the effects of social isolation on these other brain regions for a more comprehensive understanding of its impact on the brain. Secondly, we did not examine molecular changes at the cellular or laminar resolutions within the medial PFC. Investigating the effects of social isolation on specific cell types, such as different subpopulations of pyramidal neurons or interneurons, or the expression of molecular markers in distinct cortical layers, could provide further insights into the underlying mechanisms of the observed alterations in excitatory and inhibitory circuitry. Finally, although our study revealed significant alterations in NARP expression in LCLs generated from individuals with ASD, it is important to note that LCLs are not neuronal cells, and thus may not fully recapitulate the molecular and cellular processes occurring in the brain. Furthermore, the absence of clinical indices of sociability in our dataset precludes a direct correlation between NARP mRNA expression and sociability scores in ASD, which would have provided deeper insights into the biological underpinnings of social behavior in ASD. Future studies should incorporate these measures to elucidate the role of NARP in individuals with ASD. However, the significant reduction in NARP expression in ASD LCLs, combined with our findings of altered NARP and PV expression, electrophysiological properties, and social behavior in socially isolated mice, offers valuable insights into its role in the pathophysiology of ASD.
In conclusion, the present study provides novel insights into the impact of juvenile social isolation on the excitability of medial PFC pyramidal cells and expression of NARP, with subsequent effects on parvalbumin inhibitory neurons. Furthermore, we suggest a potential link between NARP expression, social behavior, and ASD pathophysiology. A deeper understanding of the molecular mechanisms underlying the effects of social experiences during critical periods may inform the development of early intervention strategies to mitigate the long-term consequences of aberrant juvenile social interactions on brain development and function.
The raw data supporting the conclusions of article will be made available by the authors, without undue reservation.
The studies involving humans were approved by the Nara Medical University Ethics Committee. The studies were conducted in accordance with the local legislation and institutional requirements. Written informed consent for participation in this study was provided by the participants’ legal guardians/next of kin. The animal study was approved by the Animal Care and Use Committee of Nara Medical University. The study was conducted in accordance with the local legislation and institutional requirements.
YY: Formal analysis, Investigation, Writing – original draft, Writing – review & editing, Data curation. KOka: Formal analysis, Funding acquisition, Investigation, Writing – original draft, Writing – review & editing, Data curation. KY: Writing – review & editing, Investigation. KOku: Writing – review & editing, Investigation. TKo: Writing – review & editing, Investigation. MT: Writing – review & editing, Investigation, Resources. RT: Writing – review & editing, Investigation. YN: Writing – review & editing, Investigation. DI: Writing – review & editing, Investigation. TY: Writing – review & editing, Investigation. MM: Writing – review & editing, Investigation, Supervision. HY: Writing – review & editing, Investigation, Supervision. YS: Writing – review & editing, Supervision. HM: Writing – review & editing, Resources, Supervision. TKi: Supervision, Writing – review & editing. SK: Formal analysis, Funding acquisition, Investigation, Methodology, Supervision, Writing – original draft, Writing – review & editing, Data curation.
The author(s) declare financial support was received for the research, authorship, and/or publication of this article. This study was supported by Grants-in-Aid for Scientific Research from the Japan Society for the Promotion of Science (15K19742 to SK and 19K17117 to KOka) and the Takeda Scientific Foundation (to SK).
We would like to thank Editage (www.editage.cn) for English language editing.
The authors declare that the research was conducted in the absence of any commercial or financial relationships that could be construed as a potential conflict of interest.
All claims expressed in this article are solely those of the authors and do not necessarily represent those of their affiliated organizations, or those of the publisher, the editors and the reviewers. Any product that may be evaluated in this article, or claim that may be made by its manufacturer, is not guaranteed or endorsed by the publisher.
The Supplementary Material for this article can be found online at: https://www.frontiersin.org/articles/10.3389/fpsyt.2024.1403476/full#supplementary-material
1. Knudsen EI. Sensitive periods in the development of the brain and behavior. J Cognit Neurosci. (2004) 16:1412–25. doi: 10.1162/0898929042304796
2. Maffei A, Lambo ME, Turrigiano GG. Critical period for inhibitory plasticity in rodent binocular V1. J Neurosci. (2010) 30:3304–9. doi: 10.1523/JNEUROSCI.5340-09.2010
3. Maffei A, Turrigiano G. The age of plasticity: developmental regulation of synaptic plasticity in neocortical microcircuits. Prog Brain Res. (2008) 169:211–23. doi: 10.1016/S0079-6123(07)00012-X
4. Whitaker LR, Degoulet M, Morikawa H. Social deprivation enhances VTA synaptic plasticity and drug-induced contextual learning. Neuron. (2013) 77:335–45. doi: 10.1016/j.neuron.2012.11.022
5. Makinodan M, Rosen KM, Ito S, Corfas G. A critical period for social experience-dependent oligodendrocyte maturation and myelination. Science. (2012) 337:1357–60. doi: 10.1126/science.1220845
6. Okamura K, Yoshino H, Ogawa Y, Yamamuro K, Kimoto S, Yamaguchi Y, et al. Juvenile social isolation immediately affects the synaptic activity and firing property of fast-spiking parvalbumin-expressing interneuron subtype in mouse medial prefrontal cortex. Cereb Cortex. (2023) 33:3591–606. doi: 10.1093/cercor/bhac294
7. Yamamuro K, Yoshino H, Ogawa Y, Makinodan M, Toritsuka M, Yamashita M, et al. Social isolation during the critical period reduces synaptic and intrinsic excitability of a subtype of pyramidal cell in mouse prefrontal cortex. Cereb Cortex. (2018) 28:998–1010. doi: 10.1093/cercor/bhx010
8. Yamamuro K, Yoshino H, Ogawa Y, Okamura K, Nishihata Y, Makinodan M, et al. Juvenile social isolation enhances the activity of inhibitory neuronal circuits in the medial prefrontal cortex. Front Cell Neurosci. (2020) 14:105. doi: 10.3389/fncel.2020.00105
9. Chugani HT, Behen ME, Muzik O, Juhász C, Nagy F, Chugani DC. Local brain functional activity following early deprivation: a study of postinstitutionalized Romanian orphans. Neuroimage. (2001) 14:1290–301. doi: 10.1006/nimg.2001.0917
10. Eluvathingal TJ, Chugani HT, Behen ME, Juhász C, Muzik O, Maqbool M, et al. Abnormal brain connectivity in children after early severe socioemotional deprivation: a diffusion tensor imaging study. Pediatrics. (2006) 117:2093–100. doi: 10.1542/peds.2005-1727
11. Arnsten AF, Rubia K. Neurobiological circuits regulating attention, cognitive control, motivation, and emotion: disruptions in neurodevelopmental psychiatric disorders. J Am Acad Child Adolesc Psychiatry. (2012) 51:356–67. doi: 10.1016/j.jaac.2012.01.008
12. Dienel SJ, Lewis DA. Alterations in cortical interneurons and cognitive function in schizophrenia. Neurobiol Dis. (2019) 131:104208. doi: 10.1016/j.nbd.2018.06.020
13. Xu P, Chen A, Li Y, Xing X, Lu H. Medial prefrontal cortex in neurological diseases. Physiol Genomics. (2019) 51:432–42. doi: 10.1152/physiolgenomics.00006.2019
14. Gonchar Y, Wang Q, Burkhalter A. Multiple distinct subtypes of GABAergic neurons in mouse visual cortex identified by triple immunostaining. Front Neuroanat. (2007) 1:3. doi: 10.3389/neuro.05.003.2007
15. Xu X, Roby KD, Callaway EM. Immunochemical characterization of inhibitory mouse cortical neurons: three chemically distinct classes of inhibitory cells. J Comp Neurol. (2010) 518:389–404. doi: 10.1002/cne.22229
16. Fagiolini M, Fritschy JM, Löw K, Möhler H, Rudolph U, Hensch TK. Specific GABAA circuits for visual cortical plasticity. Science. (2004) 303:1681–3. doi: 10.1126/science.1091032
17. Gonzalez-Burgos G, Cho RY, Lewis DA. Alterations in cortical network oscillations and parvalbumin neurons in schizophrenia. Biol Psychiatry. (2015) 77:1031–40. doi: 10.1016/j.biopsych.2015.03.010
18. Hashemi E, Ariza J, Rogers H, Noctor SC, Martínez-Cerdeño V. The number of parvalbumin-expressing interneurons is decreased in the prefrontal cortex in autism. Cereb Cortex. (2017) 27:1931–43. doi: 10.1093/cercor/bhw021
19. Iaccarino HF, Singer AC, Martorell AJ, Rudenko A, Gao F, Gillingham TZ, et al. Gamma frequency entrainment attenuates amyloid load and modifies microglia. Nature. (2016) 540:230–5. doi: 10.1038/nature20587
20. Lewis DA, Curley AA, Glausier JR, Volk DW. Cortical parvalbumin interneurons and cognitive dysfunction in schizophrenia. Trends Neurosci. (2012) 35:57–67. doi: 10.1016/j.tins.2011.10.004
21. Ruden JB, Dugan LL, Konradi C. Parvalbumin interneuron vulnerability and brain disorders. Neuropsychopharmacology. (2021) 46:279–87. doi: 10.1038/s41386-020-0778-9
22. West AE, Greenberg ME. Neuronal activity-regulated gene transcription in synapse development and cognitive function. Cold Spring Harb Perspect Biol. (2011) 3(6):a005744. doi: 10.1101/cshperspect.a005744
23. Chang MC, Park JM, Pelkey KA, Grabenstatter HL, Xu D, Linden DJ, et al. Narp regulates homeostatic scaling of excitatory synapses on parvalbumin-expressing interneurons. Nat Neurosci. (2010) 13:1090–7. doi: 10.1038/nn.2621
24. Lawrence JJ. Subcortical neuromodulation of feedforward and feedback inhibitory microcircuits by the reticular activating system. In: Monti JM, Pandi-Perumal SR, Möhler H, editors. GABA and Sleep: Molecular, Functional and Clinical Aspects. Springer Basel, Basel (2010). p. 147–68
25. Gu Y, Huang S, Chang MC, Worley P, Kirkwood A, Quinlan EM. Obligatory role for the immediate early gene NARP in critical period plasticity. Neuron. (2013) 79:335–46. doi: 10.1016/j.neuron.2013.05.016
26. Kimoto S, Zaki MM, Bazmi HH, Lewis DA. Altered markers of cortical γ-aminobutyric acid neuronal activity in schizophrenia: role of the NARP gene. JAMA Psychiatry. (2015) 72:747–56. doi: 10.1001/jamapsychiatry.2015.0533
27. Xiao MF, Xu D, Craig MT, Pelkey KA, Chien CC, Shi Y, et al. NPTX2 and cognitive dysfunction in Alzheimer’s Disease. Elife. (2017) 6:e23798. doi: 10.7554/eLife.23798
28. Yamashita Y, Makinodan M, Toritsuka M, Yamauchi T, Ikawa D, Kimoto S, et al. Anti-inflammatory effect of ghrelin in lymphoblastoid cell lines from children with autism spectrum disorder. Front Psychiatry. (2019) 10:152. doi: 10.3389/fpsyt.2019.00152
29. Neher E. Correction for liquid junction potentials in patch clamp experiments. Methods Enzymol. (1992) 207:123–31. doi: 10.1016/0076-6879(92)07008-C
30. Lo SC, Scearce-Levie K, Sheng M. Characterization of social behaviors in caspase-3 deficient mice. Sci Rep. (2016) 6:18335. doi: 10.1038/srep18335
31. del Río JA, de Lecea L, Ferrer I, Soriano E. The development of parvalbumin-immunoreactivity in the neocortex of the mouse. Brain Res Dev Brain Res. (1994) 81:247–59. doi: 10.1016/0165-3806(94)90311-5
32. Tsui CC, Copeland NG, Gilbert DJ, Jenkins NA, Barnes C, Worley PF. Narp, a novel member of the pentraxin family, promotes neurite outgrowth and is dynamically regulated by neuronal activity. J Neurosci. (1996) 16:2463–78. doi: 10.1523/JNEUROSCI.16-08-02463.1996
33. Ueno H, Suemitsu S, Okamoto M, Matsumoto Y, Ishihara T. Parvalbumin neurons and perineuronal nets in the mouse prefrontal cortex. Neuroscience. (2017) 343:115–27. doi: 10.1016/j.neuroscience.2016.11.035
34. Bicks LK, Koike H, Akbarian S, Morishita H. Prefrontal cortex and social cognition in mouse and man. Front Psychol. (2015) 6:1805. doi: 10.3389/fpsyg.2015.01805
35. Grossmann T. The role of medial prefrontal cortex in early social cognition. Front Hum Neurosci. (2013) 7:340. doi: 10.3389/fnhum.2013.00340
36. Ko J. Neuroanatomical substrates of rodent social behavior: the medial prefrontal cortex and its projection patterns. Front Neural Circuits. (2017) 11:41. doi: 10.3389/fncir.2017.00041
37. Krueger F, Barbey AK, Grafman J. The medial prefrontal cortex mediates social event knowledge. Trends Cognit Sci. (2009) 13:103–9. doi: 10.1016/j.tics.2008.12.005
38. Saxe R. Uniquely human social cognition. Curr Opin Neurobiol. (2006) 16:235–9. doi: 10.1016/j.conb.2006.03.001
39. Iwamoto K, Kakiuchi C, Bundo M, Ikeda K, Kato T. Molecular characterization of bipolar disorder by comparing gene expression profiles of postmortem brains of major mental disorders. Mol Psychiatry. (2004) 9:406–16. doi: 10.1038/sj.mp.4001437
40. Lai CY, Scarr E, Udawela M, Everall I, Chen WJ, Dean B. Biomarkers in schizophrenia: A focus on blood based diagnostics and theranostics. World J Psychiatry. (2016) 6:102–17. doi: 10.5498/wjp.v6.i1.102
41. Michaelovsky E, Carmel M, Gothelf D, Weizman A. Lymphoblast transcriptome analysis in 22q11.2 deletion syndrome individuals with schizophrenia-spectrum disorder. World J Biol Psychiatry. (2024) 25:242–54. doi: 10.1080/15622975.2024.2327030
42. Yasuda Y, Hashimoto R, Yamamori H, Ohi K, Fukumoto M, Umeda-Yano S, et al. Gene expression analysis in lymphoblasts derived from patients with autism spectrum disorder. Mol Autism. (2011) 2:9. doi: 10.1186/2040-2392-2-9
43. Wang Y, Ye M, Kuang X, Li Y, Hu S. A simplified morphological classification scheme for pyramidal cells in six layers of primary somatosensory cortex of juvenile rats. IBRO Rep. (2018) 5:74–90. doi: 10.1016/j.ibror.2018.10.001
44. Dembrow N, Johnston D. Subcircuit-specific neuromodulation in the prefrontal cortex. Front Neural Circuits. (2014) 8:54. doi: 10.3389/fncir.2014.00054
45. Gabbott PL, Warner TA, Jays PR, Salway P, Busby SJ. Prefrontal cortex in the rat: projections to subcortical autonomic, motor, and limbic centers. J Comp Neurol. (2005) 492:145–77. doi: 10.1002/cne.20738
46. Harris KD, Shepherd GM. The neocortical circuit: themes and variations. Nat Neurosci. (2015) 18:170–81. doi: 10.1038/nn.3917
47. Fone KC, Porkess MV. Behavioural and neurochemical effects of post-weaning social isolation in rodents-relevance to developmental neuropsychiatric disorders. Neurosci Biobehav Rev. (2008) 32:1087–102. doi: 10.1016/j.neubiorev.2008.03.003
48. Li DC, Hinton EA, Gourley SL. Persistent behavioral and neurobiological consequences of social isolation during adolescence. Semin Cell Dev Biol. (2021) 118:73–82. doi: 10.1016/j.semcdb.2021.05.017
49. Li DC, Hinton EA, Guo J, Knight KA, Sequeira MK, Wynne ME, et al. Social experience in adolescence shapes prefrontal cortex structure and function in adulthood. Mol Psychiatry. (2024). doi: 10.1038/s41380-024-02540-6
50. Yamamuro K, Bicks LK, Leventhal MB, Kato D, Im S, Flanigan ME, et al. A prefrontal-paraventricular thalamus circuit requires juvenile social experience to regulate adult sociability in mice. Nat Neurosci. (2020) 23:1240–52. doi: 10.1038/s41593-020-0695-6
51. Spiegel I, Mardinly AR, Gabel HW, Bazinet JE, Couch CH, Tzeng CP, et al. Npas4 regulates excitatory-inhibitory balance within neural circuits through cell-type-specific gene programs. Cell. (2014) 157:1216–29. doi: 10.1016/j.cell.2014.03.058
52. Miyamae T, Chen K, Lewis DA, Gonzalez-Burgos G. Distinct physiological maturation of parvalbumin-positive neuron subtypes in mouse prefrontal cortex. J Neurosci. (2017) 37:4883–902. doi: 10.1523/JNEUROSCI.3325-16.2017
53. Gouwens NW, Sorensen SA, Baftizadeh F, Budzillo A, Lee BR, Jarsky T, et al. Integrated morphoelectric and transcriptomic classification of cortical GABAergic cells. Cell. (2020) 183:935–953.e919. doi: 10.1016/j.cell.2020.09.057
54. Dere E, Huston JP, De Souza Silva MA. The pharmacology, neuroanatomy and neurogenetics of one-trial object recognition in rodents. Neurosci Biobehav Rev. (2007) 31:673–704. doi: 10.1016/j.neubiorev.2007.01.005
55. Felix-Ortiz AC, Burgos-Robles A, Bhagat ND, Leppla CA, Tye KM. Bidirectional modulation of anxiety-related and social behaviors by amygdala projections to the medial prefrontal cortex. Neuroscience. (2016) 321:197–209. doi: 10.1016/j.neuroscience.2015.07.041
56. Ieraci A, Mallei A, Popoli M. Social isolation stress induces anxious-depressive-like behavior and alterations of neuroplasticity-related genes in adult male mice. Neural Plast. (2016) 2016:6212983. doi: 10.1155/2016/6212983
57. Lukkes JL, Watt MJ, Lowry CA, Forster GL. Consequences of post-weaning social isolation on anxiety behavior and related neural circuits in rodents. Front Behav Neurosci. (2009) 3:18. doi: 10.3389/neuro.08.018.2009
58. Makinodan M, Ikawa D, Yamamuro K, Yamashita Y, Toritsuka M, Kimoto S, et al. Effects of the mode of re-socialization after juvenile social isolation on medial prefrontal cortex myelination and function. Sci Rep. (2017) 7:5481. doi: 10.1038/s41598-017-05632-2
59. Lander SS, Linder-Shacham D, Gaisler-Salomon I. Differential effects of social isolation in adolescent and adult mice on behavior and cortical gene expression. Behav Brain Res. (2017) 316:245–54. doi: 10.1016/j.bbr.2016.09.005
60. Rubenstein JL, Merzenich MM. Model of autism: increased ratio of excitation/inhibition in key neural systems. Genes Brain Behav. (2003) 2:255–67. doi: 10.1034/j.1601-183X.2003.00037.x
61. Sohal VS, Rubenstein JLR. Excitation-inhibition balance as a framework for investigating mechanisms in neuropsychiatric disorders. Mol Psychiatry. (2019) 24:1248–57. doi: 10.1038/s41380-019-0426-0
62. Gregg JP, Lit L, Baron CA, Hertz-Picciotto I, Walker W, Davis RA, et al. Gene expression changes in children with autism. Genomics. (2008) 91:22–9. doi: 10.1016/j.ygeno.2007.09.003
63. Nishimura Y, Martin CL, Vazquez-Lopez A, Spence SJ, Alvarez-Retuerto AI, Sigman M, et al. Genome-wide expression profiling of lymphoblastoid cell lines distinguishes different forms of autism and reveals shared pathways. Hum Mol Genet. (2007) 16:1682–98. doi: 10.1093/hmg/ddm116
64. Talebizadeh Z, Butler MG, Theodoro MF. Feasibility and relevance of examining lymphoblastoid cell lines to study role of microRNAs in autism. Autism Res. (2008) 1:240–50. doi: 10.1002/aur.33
65. Talebizadeh Z, Lam DY, Theodoro MF, Bittel DC, Lushington GH, Butler MG. Novel splice isoforms for NLGN3 and NLGN4 with possible implications in autism. J Med Genet. (2006) 43:e21. doi: 10.1136/jmg.2005.036897
66. Gangopadhyay P, Chawla M, Dal Monte O, Chang SWC. Prefrontal-amygdala circuits in social decision-making. Nat Neurosci. (2021) 24:5–18. doi: 10.1038/s41593-020-00738-9
67. Tao K, Chung M, Watarai A, Huang Z, Wang MY, Okuyama T. Disrupted social memory ensembles in the ventral hippocampus underlie social amnesia in autism-associated Shank3 mutant mice. Mol Psychiatry. (2022) 27:2095–105. doi: 10.1038/s41380-021-01430-5
68. Tzakis N, Holahan MR. Social memory and the role of the hippocampal CA2 region. Front Behav Neurosci. (2019) 13:233. doi: 10.3389/fnbeh.2019.00233
69. Campbell-Meiklejohn DK, Kanai R, Bahrami B, Bach DR, Dolan RJ, Roepstorff A, et al. Structure of orbitofrontal cortex predicts social influence. Curr Biol. (2012) 22:R123–124. doi: 10.1016/j.cub.2012.01.012
Keywords: social isolation, brain development, prefrontal cortex (PFC), neuronal activity-regulated pentraxin (NARP), parvalbumin (PV), social behavior, autism spectrum disorder (ASD)
Citation: Yamaguchi Y, Okamura K, Yamamuro K, Okumura K, Komori T, Toritsuka M, Takada R, Nishihata Y, Ikawa D, Yamauchi T, Makinodan M, Yoshino H, Saito Y, Matsuzaki H, Kishimoto T and Kimoto S (2024) NARP-related alterations in the excitatory and inhibitory circuitry of socially isolated mice: developmental insights and implications for autism spectrum disorder. Front. Psychiatry 15:1403476. doi: 10.3389/fpsyt.2024.1403476
Received: 19 March 2024; Accepted: 17 May 2024;
Published: 06 June 2024.
Edited by:
Jun Egawa, Niigata University, JapanReviewed by:
Laurence Coutellier, The Ohio State University, United StatesCopyright © 2024 Yamaguchi, Okamura, Yamamuro, Okumura, Komori, Toritsuka, Takada, Nishihata, Ikawa, Yamauchi, Makinodan, Yoshino, Saito, Matsuzaki, Kishimoto and Kimoto. This is an open-access article distributed under the terms of the Creative Commons Attribution License (CC BY). The use, distribution or reproduction in other forums is permitted, provided the original author(s) and the copyright owner(s) are credited and that the original publication in this journal is cited, in accordance with accepted academic practice. No use, distribution or reproduction is permitted which does not comply with these terms.
*Correspondence: Sohei Kimoto, c2tpbW90b0B3YWtheWFtYS1tZWQuYWMuanA=
†These authors have contributed equally to this work and share first authorship
Disclaimer: All claims expressed in this article are solely those of the authors and do not necessarily represent those of their affiliated organizations, or those of the publisher, the editors and the reviewers. Any product that may be evaluated in this article or claim that may be made by its manufacturer is not guaranteed or endorsed by the publisher.
Research integrity at Frontiers
Learn more about the work of our research integrity team to safeguard the quality of each article we publish.