- 1Department of Oncology, Hengshui Hospital of Traditional Chinese Medicine, Hengshui, Hebei, China
- 2Department of Psychiatry, Xijing Hospital, Air Force Medical University, Xi’an, Shaanxi, China
- 3Department of Psychiatry, The 907th Hospital of the PLA Joint Logistics Support Force, Nanping, Fujian, China
- 4Institute for Hospital Management Research, Chinese PLA General Hospital, Beijing, China
Background: Cuprizone (CPZ)-treated mice show significant demyelination, altered gut microbiome, and depressive-like behaviors. However, the effects of venlafaxine (Ven) on the gut microbiome and depressive-like behavior of CPZ-treated mice are largely unclear.
Methods: Male C57BL/6J mice were fed a chow containing 0.2% cuprizone (w/w) for 5 weeks to induce a model of demyelination. Meanwhile, the gut microbiota and depressive-like behaviors were assessed after the mice were fed with Ven (20 mg/kg/day) or equal volumes of distilled water for 2 weeks by oral gavage from the third week onward during CPZ treatment.
Results: CPZ treatment decreased the sucrose preference rate in the sucrose preference test and increased the immobility time in the tail-suspension test, and it also induced an abnormality in β-diversity and changes in microbial composition. Ven alleviated the depressive-like behavior and regulated the composition of the gut microbiota, such as the increase of Lactobacillus and Bifidobacterium in CPZ-treated mice.
Conclusion: The anti-depressant effects of Ven might be related to the regulation of gut microbiota in the CPZ-treated mice.
1 Introduction
Major depressive disorder (MDD) is the most prevalent mental illness that affects millions of individuals and constitutes a substantial share of the disease burden globally (1). The lifetime prevalence of depression ranges from 20% to 25% in women and from 7% to 12% in men (2, 3). Besides the persistent loss of interest and sadness, MDD is always accompanied by appetite, disturbed sleep, low self-worth, tiredness, and hopelessness. MDD also leads to nearly 800,000 people dying of suicide every year (4). The causative factors of MDD are complex and may be related to multiple factors such as genetic, hormonal, neuroimmune, environmental, and psychological stress (5). Compared with healthy subjects, patients with MDD showed reductions in myelin content, axon numbers, and myelin-related genes and proteins in various brain areas (6, 7). Consistently, decreased white matter (WM) volume and total length of myelinated fibers in WM were observed in a chronic unpredictable stress (CUS) model of depression in rats (8), and a reduction in oligodendrocyte-related proteins and myelin were also shown in a mice model of unpredictable chronic mild stress (UCMS) (9). Moreover, impaired WM integrity is more common in other neurological disorders that are accompanied by depressive symptoms, such as multiple sclerosis (MS) (10) and Parkinson’s disease (11). Myelin damage might participate in the pathogenesis of MDD, and the disruption of proliferation and differentiation of oligodendrocytes (OL) lineage cell may contribute to the depression phenotype (12–14).
There is growing evidence that MDD may be closely related to gut microbiota abnormalities and that the gut microbiota can affect the central nervous system through the gut–brain axis, altering gut–brain signaling in patients, which, in turn, plays a crucial role in host physiology, homeostasis, development and metabolism (15, 16). Patients with MDD exhibit disruptions in the gut microbiota and its metabolites (17, 18), and alterations in gut microbiota composition are also a hallmark of depression (19, 20). Meanwhile, prebiotics and probiotics showed anti-depressive effects (21, 22). In turn, dysfunction in the gut microbiota induces depressive-like behaviors and reinforces the risk of depression (23–26)—for example, when the gut microbiota of a depressed-like mice is transplanted into a normal mouse gavaged with antibiotics, that mouse exhibits a depression-like behavior (23, 27, 28). In contrast, transplantation of healthy population microbiota into mice with depressive-like behavior attenuates depressive-like symptoms (29, 30). Recently, studies further showed that Dialister and Coprococcus were found to be depleted in MDD patients (31, 32) and indicated a potential role of microbial γ-aminobutyric acid (GABA) production in depression (33). Intriguingly, gut microbiota has also been associated with processes such as myelin formation and development. Studies have shown that gut microbiota-derived metabolites and cellular components are important to improve brain homeostasis and the progression of neuropsychological problems—for example, tryptophan precursors and metabolites, 5-hydroxytryptamine (5-HT), GABA, glutamine, histamine, branched-chain amino acids (BCAAs), LPS, and catecholamines are important host/microbial-derived metabolites or components that occur in the regulation of neurogenesis, glial cell function, and myelin formation (34, 35). In the experimental autoimmune encephalomyelitis (EAE) mouse model, altered gut microbes also affect myelin integrity, with mice treated with broad-spectrum antibiotics preventing motor dysfunction and myelin damage, while bacterial recolonization impairs motor function and axonal integrity (36, 37). In short, there is a potential link between myelin integrity and specific alterations in the gut microbiota.
Venlafaxine (Ven) is a 5-HT and norepinephrine reuptake inhibitor (SNRI) antidepressant that has been widely used in MDD, anxiety disorders, and neuropathy. Among them, 5-HT plays a neurotransmitter role in the brain and enteric nervous system, participates in mood and cognitive regulation, and also regulates gastrointestinal secretion and motility in the enteric nervous system (38, 39). Treatment with Ven at a dosage of 10 mg/kg/day has been demonstrated to effectively reverse the reductions in body weight, motor activity, and sucrose consumption induced by chronic unpredictable mild stress (CUMS) and ameliorate depressive-like behaviors (40). Additionally, Ven has been found to attenuate myelin loss in the corpus callosum and prefrontal cortex, leading to an increase in the total number of oligodendrocyte lineage (OL) cells, including non-maturing oligodendrocytes (41). These findings highlight the significant role of venlafaxine in protecting the myelin, although the precise mechanism underlying this protective effect remains unclear. Meanwhile, a recent work found that Ven decreases the abundance but not the homogeneity of the microbial community and regulates the composition of the microorganisms, with a significant decrease in the relative abundance of Ruminococcus and Adlercreutzia, which are associated with its antidepressant effects (42). However, the effects of Ven on depressive-like behavior and regulation of gut microbial structure and function in a mouse model of demyelination have not been fully characterized.
Bis-cyclohexanone-oxaldihydrazone (cuprizone, CPZ) is a copper chelator that induces loss of myelin in specific brain regions when fed to rodents (43). The widely used CPZ model was established by Hiremath and colleagues in 1998, which used lower-dose CPZ (0.2% w/w) administration in C57BL/6 mice (44). CPZ is commonly administered for 4–6 weeks to study acute demyelination and to study remyelination after CPZ withdrawal. Although it was the most commonly used MS model, previous studies have confirmed that CPZ-treated mice exhibit significant depressive-like behaviors (41, 45). Moreover, CPZ administration also leads to an abnormal composition of the gut microbiota (37, 46). Considering the above-mentioned details, the purpose of this study was to explore the effects of Ven on the intestinal microbiome and depressive-like effects of CPZ model mice. The data could shed more light on the effects of antidepressants on gut microbes.
2 Materials and methods
2.1 Experimental design
To investigate the impact of venlafaxine (Ven) on the gut microbiome of CPZ-treated mice, 30 mice were randomly divided into three groups (10 per group)—control, CPZ, and CPZ + Ven—after 1 week of acclimatization (Figure 1A). The mice in the control group received regular chows plus daily treatment for 5 weeks. The mice in CPZ and CPZ + Ven groups were administered with CPZ (Sigma-Aldrich, St. Louis, USA), 0.2% by weight, in standard powdered rodent chow for 5 weeks (47, 48). From the third week onward, the CPZ + Ven group was fed with venlafaxine at 20 mg/kg/day for 2 weeks by oral gavage, whereas the control and CPZ groups were fed with equal volumes of distilled water. Body weight was measured at each weekend. Behavioral tests were performed to evaluate depressive-like behaviors during the sixth week. Finally, the mice were sacrificed, and the brains were obtained for fast blue staining. Data analysis was conducted by experimenters who were blind to the experimental grouping.
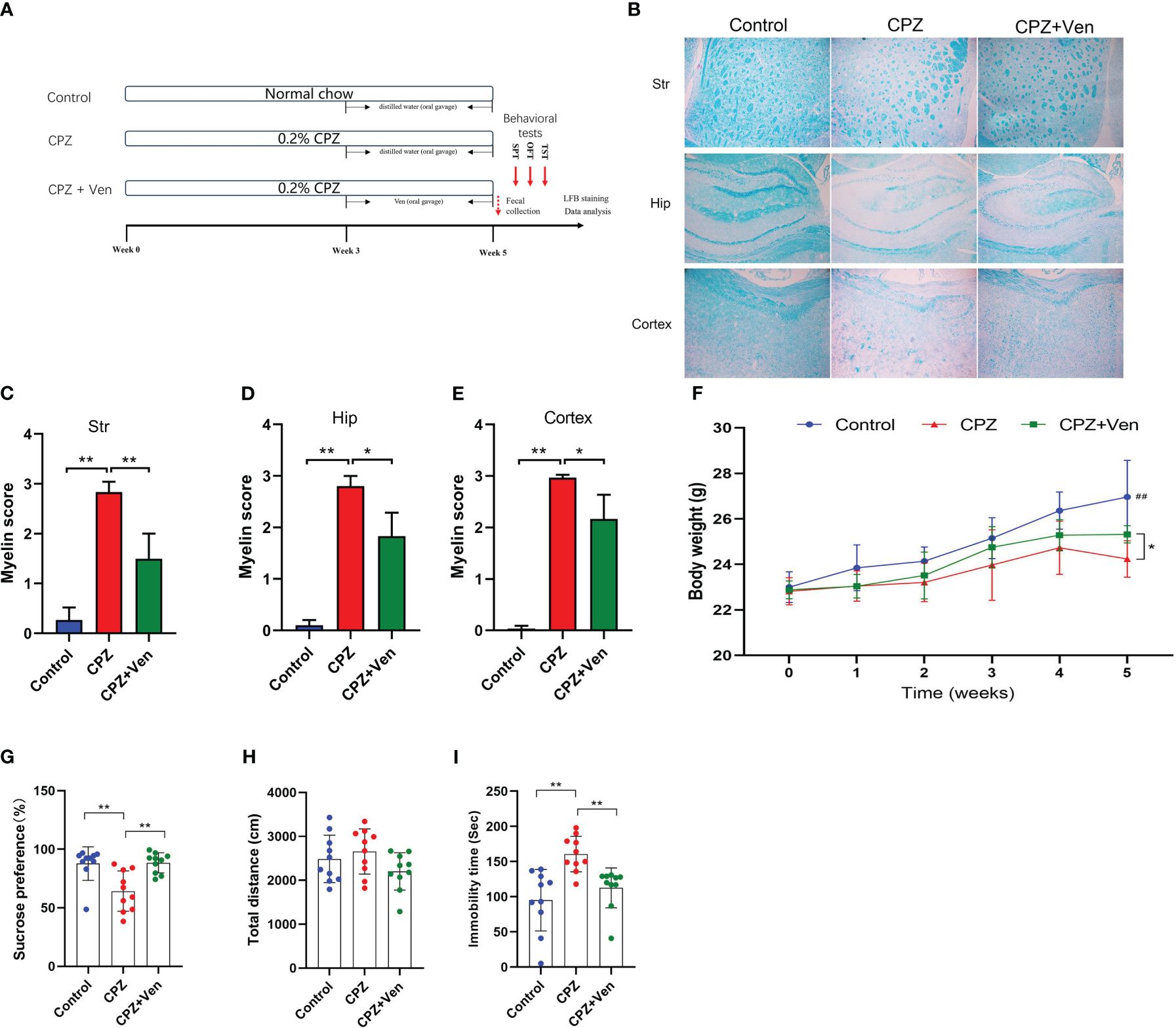
Figure 1 Ven improves cuprizone (CPZ)-induced depressive-like behaviors. (A) Experimental timeline. (B) FBL staining showed the myelin staining in the stritum (Str), hippocampus (Hip) and cerebral cortex in the three groups. (C–E) Quantitative analysis of demyelination was performed by measuring the percentage of immunostained area using ImageJ in the (C) stritum (Str), (D) hippocampus (Hip), and (E) cerebral cortex in the three groups. Data are presented as mean ± SD. (F) Changes in body weight in control, CPZ, and CPZ + Ven groups. ##P < 0.01 vs. CPZ or CPZ + Ven group. (G) Sucrose preference rate in SPT test, (H) total distance traveled in the OPT, and (I) immobility time in the TST test. The circle represents one value from individual mice in control, CPZ, and CPZ + Ven groups, respectively. *P < 0.05; **P < 0.01.
2.2 Animals
Adult male C57BL/6 mice (8 weeks old, 18–22 g in weight) were group-housed (n = 5) in cages (310 × 205 × 180 mm) at 20°C–25°C and maintained on a 12-h light/dark daily cycle (lights on from 8 a.m. to 8 p.m.) with unrestricted access to food and water. All animal experiments in this study were approved by the Animal Experimental Welfare & Ethical Inspection of Air Force Medical University (20230452) and carried out in accordance with the National Institutes of Health Guide for the Care and Use of Laboratory Animals.
2.3 Fecal sample
Fecal samples were collected when the mice were placed in a metabolic cage between 7:00 a.m. and 11:00 a.m. on the first day after the completion of the modeling. Defecation in undefecated mice was promoted by lifting their tails, ensuring that at least one fecal sample was collected from each mouse at each collection time point. The fecal samples were collected in sterile cryotubes and immediately frozen in liquid nitrogen before further analysis.
2.4 Behavioral tests
Before the formal test of all behavioral experiments, the mice were put into the experimental environment for half an hour in advance. The tests were videotaped and scored by a trained observer. The sucrose preference test (SPT) was conducted prior to the open-field test (OFT), and the tail suspension test (TST) was performed 24 h after the OFT. The test area was cleaned with 75% ethanol between tests to prevent odor interference and cross-infection.
2.4.1 SPT
Prior to the SPT test, the mice were group-housed in cages and underwent a 1% sucrose water adaptive training for 48 h. First, the mice were adapted to two bottles of 1% sucrose solution for 24 h, and then a bottle of 1% sucrose solution was replaced with pure water for another 24 h. After the adaptive training, the mice were deprived of water and food for 24 h. At the beginning of the formal experiment, all mice were individually placed in cages, and each mouse was given two bottles of quantitative liquid: a bottle of 1% sucrose solution and a bottle of pure water. The positions of the bottles were switched every 1 h. The consumption of sucrose solution and pure water was weighed 2 h later, and the sucrose preference rate was calculated: sucrose preference rate (%) = sucrose consumption/(sucrose consumption + water consumption).
2.4.2 OFT
The OFT was performed according to a recent study (49). The mice were placed in the center of an open-field box (40 cm × 40 cm × 40 cm), and then the activity was recorded for a period of 5 min. The total distance traveled was measured by using the activity software (Top Scan, Clever Sys Inc., USA).
2.4.3 TST
After having the mouse adapted to the environment, the tail of the mouse was fixed with adhesive tape and hung on the test rack (40 cm above the ground) for 6 min. Then, the mouse was returned to the cage, and the tape was gently removed to prevent additional pain. Their activity for the duration of the last 5 min was analyzed, and immobility was defined as lack of skeletal movement for at least 1 s (analyzed by Top Scan behavioral analysis software from side view).
2.5 Histopathological examination
The mice were anesthetized (sodium pentobarbital, i.p. 50 mg/kg) and then perfused with 4% paraformaldehyde in phosphate-buffered saline (PBS). The brains were removed and transferred to 30% sucrose in PBS for 1 week to dehydrate and then sectioned (15-μm brain coronal sections) with a cryostat and mounted on gelatinized slides. Myelination was examined by Luxol Fast blue (LFB, Sigma-Aldrich) staining. After the slices were cleaned with distilled water, they were immersed in 0.1% LFB solution, sealed at 60°C for 8–16 h, and continued to be separated by 0.05% lithium carbonate solution for more than 10 s. Then, the color separation was continued with 70% alcohol until the gray and white matter were clearly distinguished under the microscope. The neutral resin seal was photographed for microscopic observations, and the myelin sheath was bright blue.
Demyelination was measured using an improved semi-quantitative scale system (0–3), with a score of 0 indicating normal myelin status, 1 indicating only one-third of the myelin bundle fibers equivalent to demyelination, 2 indicating two-thirds demyelination, and 3 indicating complete myelin degradation (50). The scores of the different parts were added together to find the average score for each group.
2.6 Gut microbiota profiling by 16S rRNA gene sequencing
To profile the microbial composition, fecal samples were subjected to total genome DNA extraction using the HiPure Stool DNA Kit following the manufacturer’s instruction (D3141, Guang Zhou Genedenovo, China). The V3–V4 region of 16S rRNA genes of the samples was amplified by polymerase chain reaction (PCR) (98°C for 60 s, followed by 30 cycles at 98°C for 10 s, 50°C for 30 s, 72°C for 60 s, and 72°C for 5 min) using primers 341F 5′-CCTACGGGNGGCWGCAG-3′ and 806R 5′-GGACTACHVGGGTATCTAAT907–3′. The sequencing libraries of the V3–V4 region of the 16 S rRNA genes were generated using the TruSeq® DNA PCR-Free Sample Preparation Kit (Illumina, San Diego, CA, USA) following the manufacturer’s instruction, and index codes were added. Libraries were sequenced using an Illumina Novaseq 6000 sequencing platform. Bioinformatic analysis was performed using Omicsmart, a real-time interactive online platform for data analysis (http://www.omicsmart.com).
2.7 Statistical analysis
Upon 16S rRNA gene sequencing, 9,680,987 sequences were generated for high-quality filtering and chimera examination, with an average length of 456.38 bp for tags across all samples. The average number of reads per sample was 122,047, ranging from 36,252 to 137,595 clean reads. Based on relative abundances, the taxonomic analysis suggested 12 bacterial phyla, 19 classes, 37 orders, 55 families, and 120 genera [based on species abundances >0.1% count, in which low abundance species/operational taxonomic units (OTUs) have been filtered out]. Body weight, behavioral outcomes (total distance traveled, sucrose preference index, and immobility time), and the quantification of demyelination were assessed and compared between the groups using one-way analysis of variance (ANOVA). The results are expressed as mean ± SEM. p < 0.05 was considered statistically significant. OTUs with 97% similarity were used for α and β diversity statistics. The PCoA analysis was based on the Bray–Curtis index, and weighted and unweighted UniFrac metrics were used to assess changes in bacterial composition between groups and stages. LEfSe analysis, combined with Kruskal–Wallis test and linear discriminant analysis, was used to identify differences between the three groups of fecal microbiome (linear discriminant analysis, LDA >3.0]. The data were tested for normality, potentially followed by an independent sample t-test or a non-parametric test depending on the test results. SPSS 22.0 (IBM, Armonk, USA) and GraphPad Prism software (version 8.0) were used for statistical analysis.
3 Results
3.1 Ven improves CPZ-induced myelin injury and depressive-like behaviors
The effect of Ven on myelin injury induced by CPZ was studied by LFB staining of brain sections. As shown in Figures 1B–E, 5 weeks of CPZ treatment caused a significant myelin damage in the striatum, hippocampus, and cerebral cortex of the mice. The results of LFB staining showed that, compared with the control group, the staining of striatum (F2, 6 = 41.58, P < 0.001), hippocampus (F2, 6 = 66.49, P < 0.001), and cerebral cortex (F 2, 6 = 89.97, P < 0.001) decreased. The demyelination score was significantly higher (Figures 1C–E), and demyelination was partially alleviated by Ven intervention (CPZ vs. CPZ + Ven, P < 0.05). Meanwhile, the body weight of mice in the CPZ group and CPZ + Ven group was lower than that in the control group after 5 weeks of CPZ feeding (P < 0.01), and Ven treatment increased the body weight of CPZ-treated mice (CPZ vs. CPZ + Ven, P < 0.05, Figure 1F). There were also significant differences in the sucrose preference rate (F2, 27 = 9.948, P < 0.01, Figure 1G) and immobility time in TST (F2, 27 = 10.35, P < 0.01, Figure 1I). However, there was no significant difference in the total distance traveled in the OPT (F2, 27 = 2.108, P = 0.141, Figure 1H) and total liquid intake (F2, 27 = 0.495, P = 0.617) among the three group. Post hoc comparisons further showed that CPZ reduced the sucrose preference rate but increased the immobility time (CPZ vs. Control, P < 0.01). Ven ameliorated the depressive-like behavior induced by CPZ, as evidenced by the increasing sucrose preference rate and decreasing immobility time observed in the Ven + CPZ group (CPZ vs. CPZ + Ven, P < 0.01).
3.2 Ven influences β-diversity and the composition of gut microbiota in CPZ-treated mice
To assess the overall differences in microbial community structure between the control, CPZ, and CPZ + Ven groups, we calculated α- and β-diversity measures for ecological diversity within and between the given samples. There were no significant differences in comparative α-diversity among the three groups, including Sobs, ACE, Chao1, Simpson, Shannon, and PD tree (P > 0.05, Figures 2A–F). However, fecal microbiomes can be well separated into three groups according to Bray–Curtis (r2 = 0.2240, P = 0.001; Figure 3A), weighted UniFrac (r2 = 0.159, P = 0.001; Figure 3B), and unweighted UniFrac (r2 = 0.118, P = 0.001; Figure 3C) analyses. Moreover, a Venn diagram comparison showed that the number of OTUs in the control, CPZ, and CPZ + Ven groups was 690, 665, and 714, respectively (Figure 3D). The number of shared OTUs among the three groups was 458, while 147, 85, and 113 OTUs were unique to the control, CPZ, and CPZ + Ven groups, respectively.
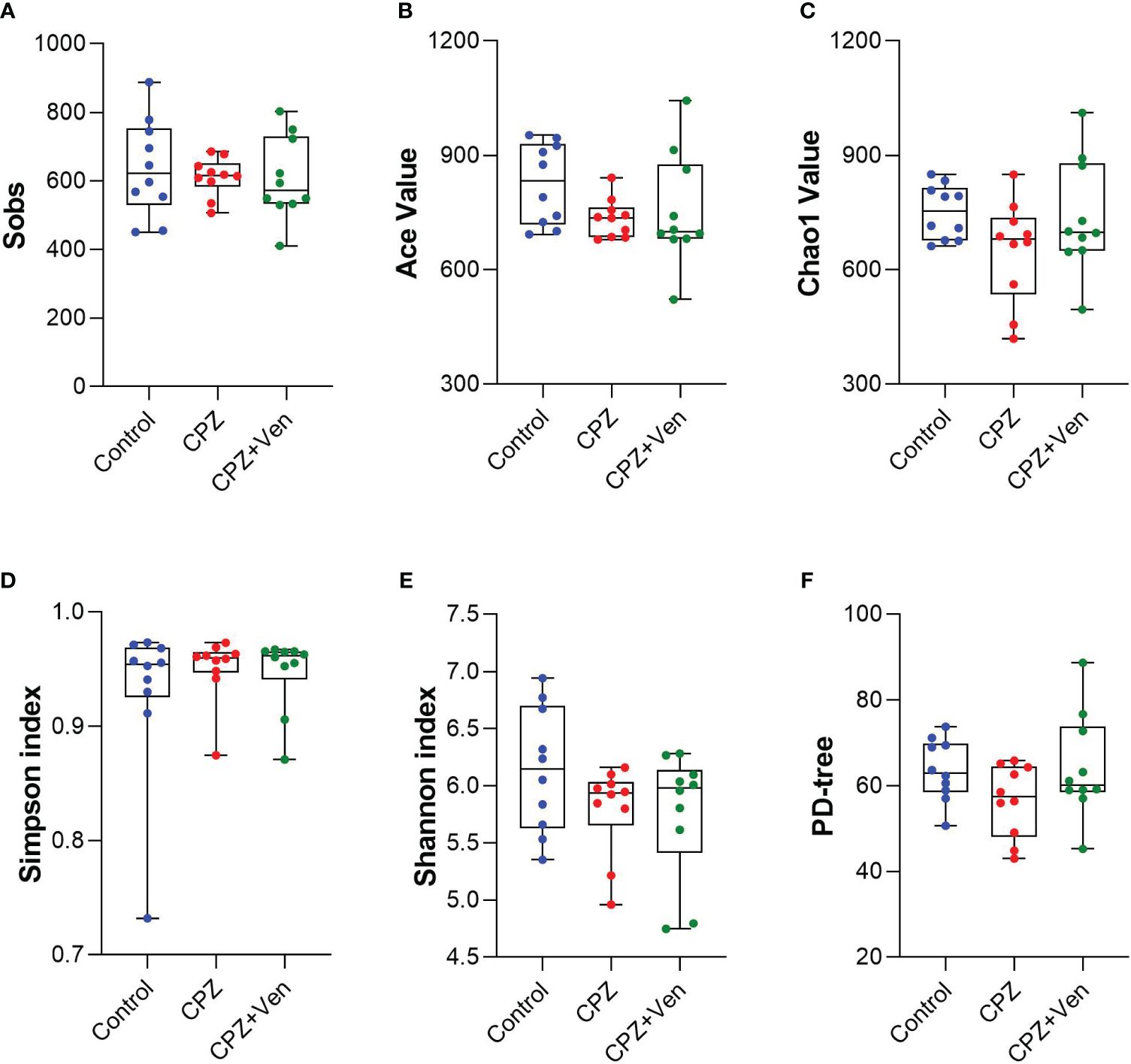
Figure 2 Comparison of α-diversity of gut microbiota among control, cuprizone (CPZ), and CPZ + Ven groups. The box plots depicted the indices of (A) Sobs, (B) Ace value, (C) Chao1 value, (D) Simpson and (E) Shannon index, and (F) PD tree of the operational taxonomic unit level. The horizontal lines in the box plots represent median values; the upper and lower ranges of the box represent the 75% and 25% quartiles, respectively. The blue, red, and green circle represents one value from individual mice in each group, respectively.
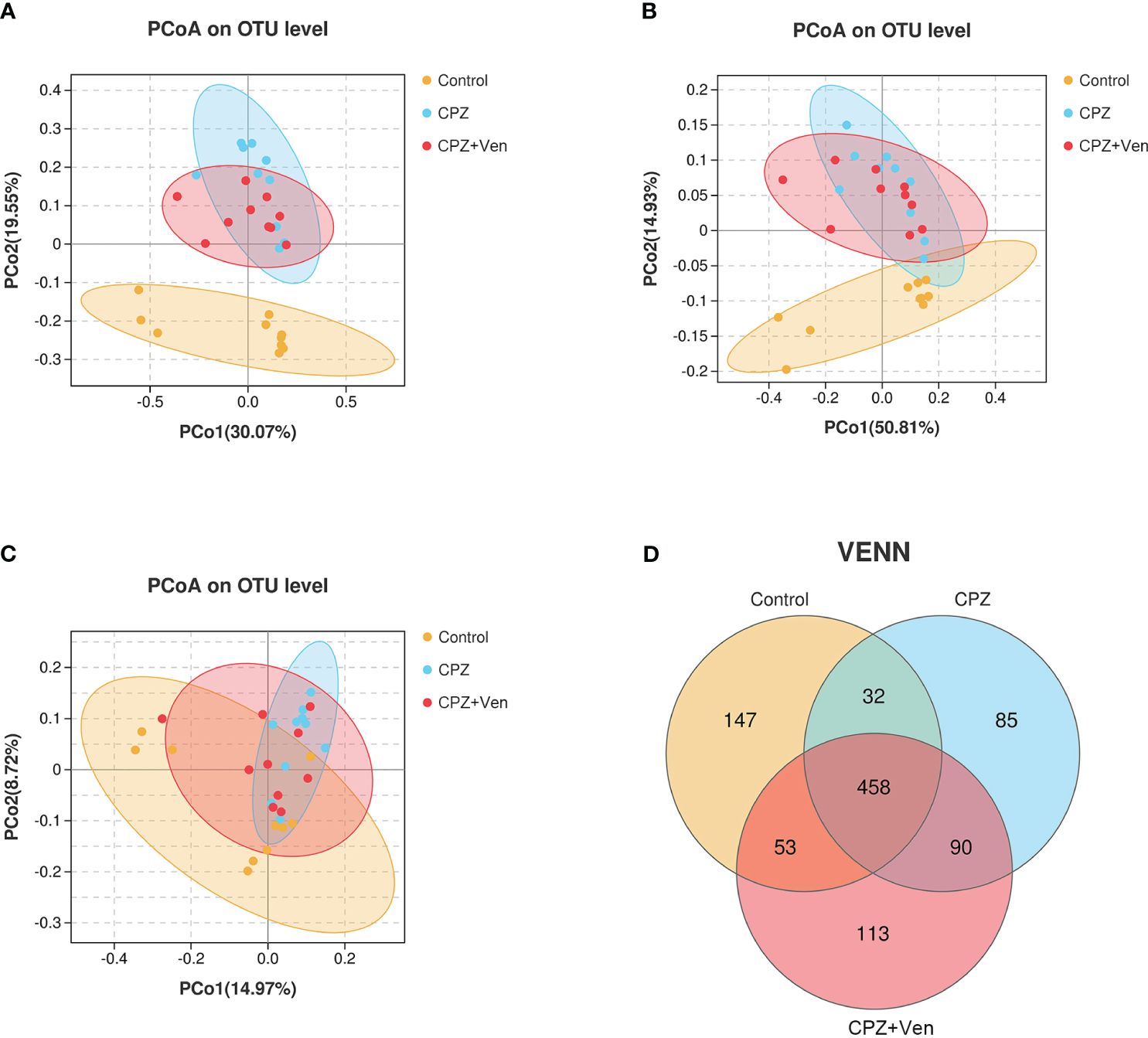
Figure 3 Comparison of β-diversity among control, cuprizone (CPZ), and CPZ + Ven groups. PCoA plots of bacterial beta-diversity based on (A) Bray–Curtis, (B) weighted UniFrac, and (C) unweighted UniFrac distance. (D) The number of common and unique operational taxonomic units between each group is displayed by the Venn diagram. The yellow, blue, or red circle represents one value from individual mice in control, CPZ, and CPZ + Ven group, respectively.
As shown in Figure 4A, Bacteroidetes, Firmicutes, Proteobacteria, and Actinobacteria were the most abundant bacterial phyla distributed in the three groups. At the family level, Muribaculaceae, Lactobacillaceae, Lachnospiraceae, Burkholderiaceae, Ruminococcaceae, Erysipelotrichaceae, Prevotellaceae, Saccharimonadaceae, and Rikenellaceae dominated in the three treatment groups of mice (Figure 4B). At the genus level, Lactobacillus, Ralstonia, Lachnospiraceae_NK4A136_group, Ruminococcaceae_UCG-014, Dubosiella, Candidatu_saccharimon, Alloprevotella, Bacteroides, Allobaculum, and Mucispirillum were mainly distributed in the three groups (Figure 4C).
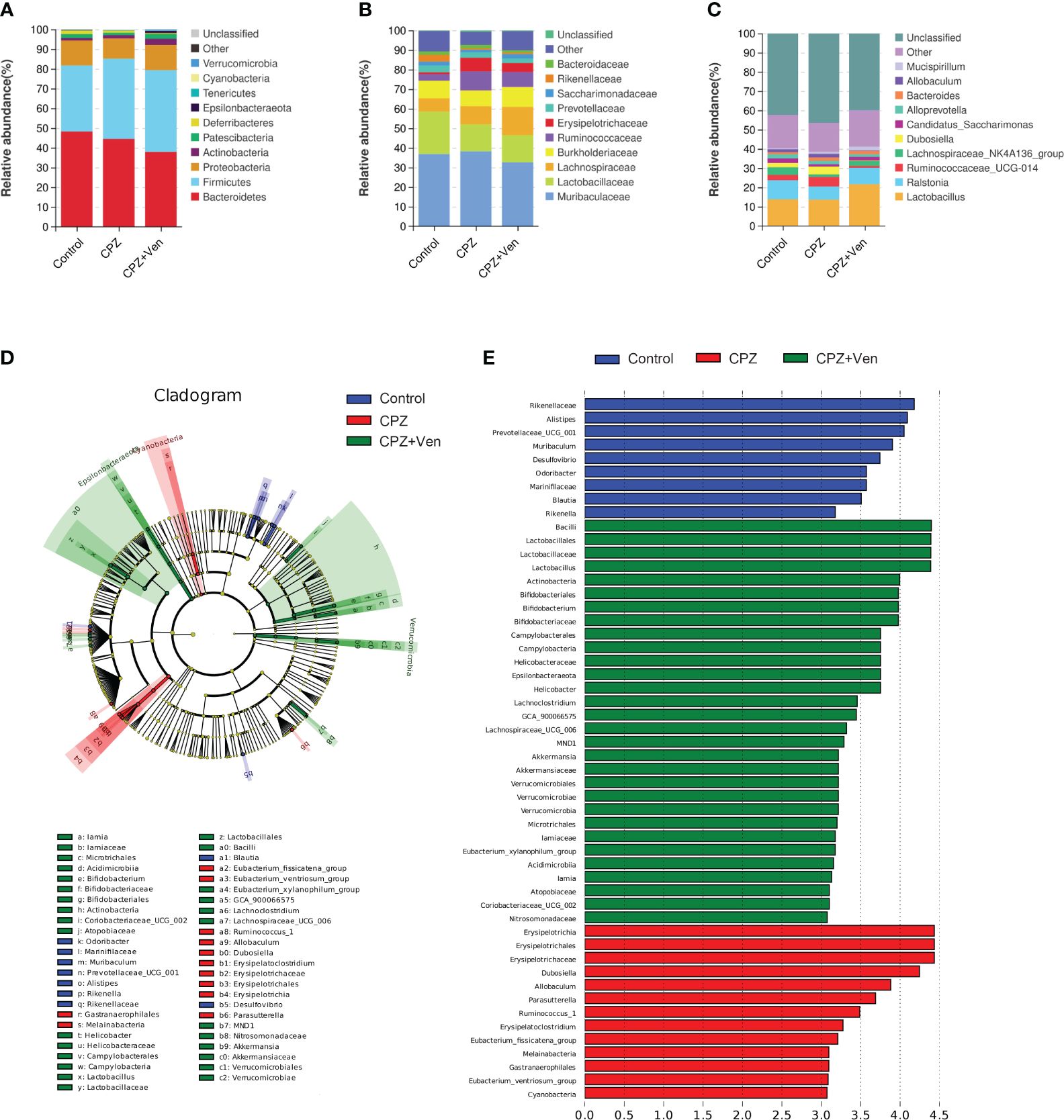
Figure 4 The comparison of taxonomic composition among control, cuprizone (CPZ), and CPZ + Ven groups. Community bar plot analysis showing the relative abundance of sequences at the (A) phylum, (B) family, and (C) genus levels. (D) The taxonomic cladogram shows the bacterial taxa enriched in control (blue dots), CPZ (red dots), and CPZ + Ven groups (green dots). (E) The LDA discriminant bar chart shows the microbial taxa with significant differences in the control (blue), CPZ (red), and CPZ + Ven groups (green). Larger LDA scores represent a greater effect of species abundance on the different effects. Only taxa with an LDA score >3.0 are shown in the figure.
To distinguish the major taxa and to reveal changes in specific bacteria and the whole gut microbial community among the three groups, LEfSe analysis was performed. As shown in Figures 4D, E; Supplementary Table S1, the relative abundance of six family (such as Leuconostocaceae, Rikenellaceae, and Clostridiaceae_1) and 11 genera (such as Prevotellaceae_UCG_001, Ileibacterium, and Blautia) was enriched in the control group; family Erysipelotrichaceae, Mycoplasmataceae, and Family_XIII and 17 genera (such as Ruminococcus_1, Candidatus_Stoquefichus, and Lachnospiraceae_FCS020_group) were enriched in the CPZ group; phylum Spirochaetes, Verrucomicrobia, and Epsilonbacteraeota, 11 families (such as Streptococcaceae, Akkermansiaceae, and Iamiaceae), and 15 genera (such as Streptococcus, Coriobacteriaceae_UCG_002, and UBA1819) were enriched in the CPZ + Ven group.
3.3 Correlation between the gut microbiome composition and depressive-like behaviors
Spearman correlation showed that the relative abundance of genus Dubosiella was negatively correlated, whereas those of Blautia and Parabacteroides were positively correlated with sucrose preference rate; the relative abundance of Dubosiella, Alistipes, and Ruminiclostridium_9 was negatively correlated with body weight; Dubosiella and Alistipes were positively correlated, whereas Bifidobacterium, Blautia, and Muribaculum were negatively correlated with immobility time (Figure 5).
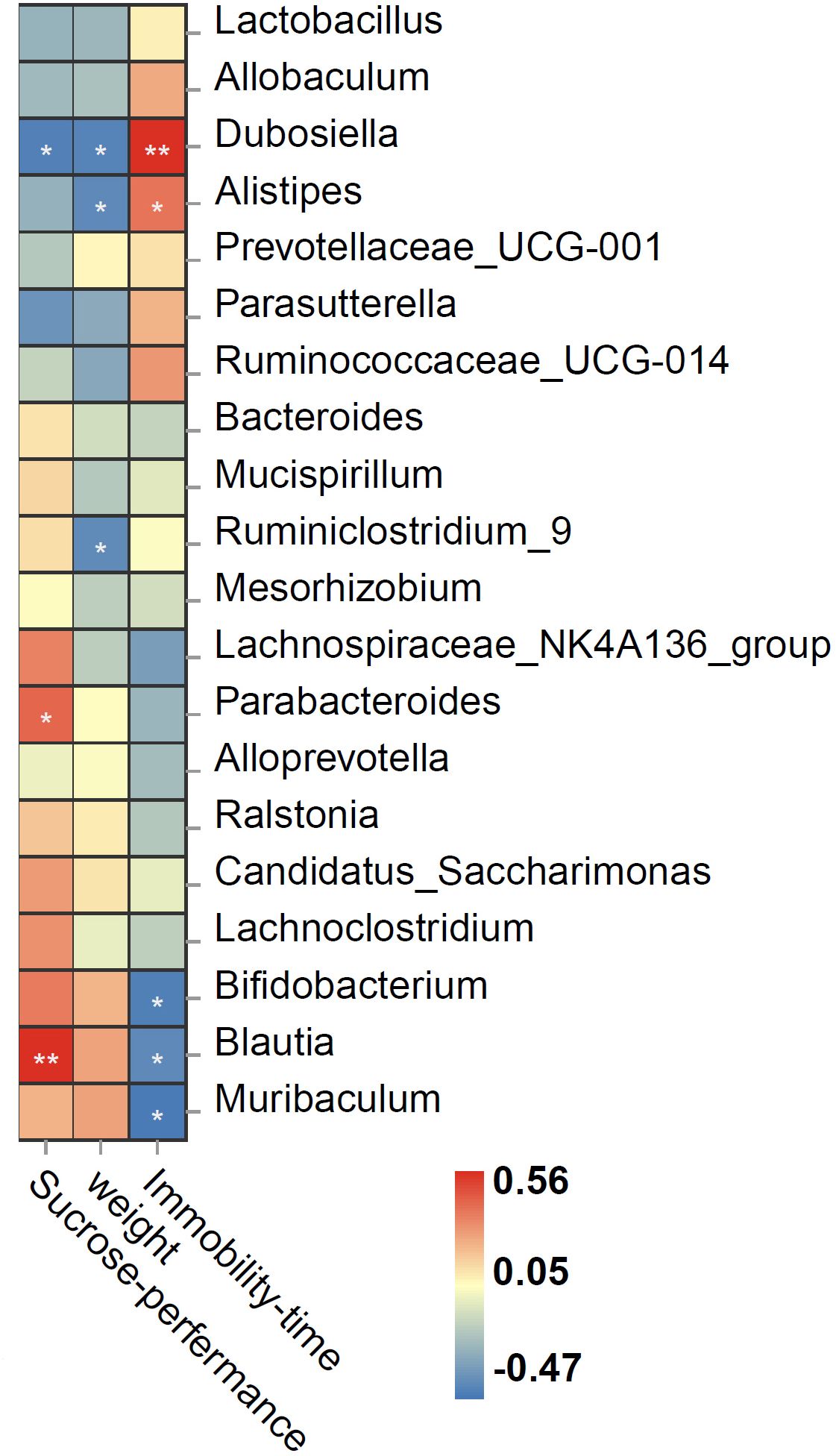
Figure 5 Spearman correlation heat map ranking the correlations between behavioral indices and bacterial abundance. The red and blue squares indicate positive and negative correlations, respectively, and the intensities of the colors are proportional to the degree of correlation. *P < 0.05; **P < 0.01.
4 Discussion
Depression poses a huge economic and medical burden to society and individuals due to its high mortality and disability rates, and investigation for the pathogenesis of depression is still urgently needed (51, 52). The gut microbiota, an integral part of the body, performs basic immune, metabolic, structural, and neurological functions and can even be called one of the “organs” of the body (53, 54). Multiple studies have shown that the gut flora can communicate with the brain and vice versa and is associated with the central nervous system through the microbiota–gut–brain axis (MGBA), thus affecting neurological and behavioral functions. Based on current data, depression is inextricably linked to altered gut microbiota, and regulation of the MGBA is emerging as a new target for the treatment of depression (55, 56).
CPZ acts specifically on oligodendrocytes in the central nervous system, causing cell death in the oligodendrocyte line and further leading to extensive demyelination of the corpus callosum, cortex, and hippocampus (57). This pattern of cell death is similar to the white matter abnormalities detected by neuroimaging in patients with demyelinating diseases (58). Although CPZ-induced demyelination is often used as a multiple sclerosis (MS) model, it also showed depressive-like behaviors, such as decreased autonomy and reduced spatial exploration (59). In fact, more than half of patients with MS exhibit depression comorbidities, indicating that both MDD and MS may have some common biological abnormalities simultaneously (e.g., deficits of myelin and excessive neuroinflammation) (60–62). Consistent with these results (47, 63), the present study found that 5 weeks of CPZ feeding induced a decrease of the sucrose preference rate and an increase in immobility time in TST but did not influence the locomotion in mice. These results indicate that the CPZ model might serve as a model that can simulate demyelination injury in MDD. Moreover, we found that Ven treatment ameliorated depressive-like behaviors induced by CPZ. Ven is one of the widely used antidepressants in clinical practices. Its neuroprotective and anti-inflammatory effects have been reported previously (64). A recent study found that Ven improves motor performance and sensitivity to a cold stimulus and ameliorates the deficit of superoxide dismutase activity in the mouse model of CPZ-induced demyelination. Ven alongside improves depressive-like behavior related to demyelination, indicating that Ven may have a potential improvement effect on MDD with abnormal white matter.
Multiple evidences elucidate that the abnormality in gut microbiota plays a role in the pathogenesis of MDD. The effect of CPZ on the gut microbiota of mice has been reported. Previous studies found that there was a significant difference in β-diversity, but not in α-diversity, after 6 weeks of CPZ treatment (46, 65). Similarly, the present study found that there was a significant difference in comparative β-diversity, but not in α-diversity, among the control, CPZ, and CPZ + Ven groups, indicating that Ven can restore the CPZ-induced abnormalities of β-diversity in the gut microbiota. Due to β-diversity reflecting the degree of change in community composition or the degree of community differentiation, we speculate that Ven has a more significant impact on the composition of microbial communities. Interestingly, a previous study found that Ven reduced the richness of microbial communities but did not affect their evenness in alpha diversity analyses, whereas the β-diversity of fecal microbial communities from mice receiving Ven was higher than that of the control samples (42), and this effect might be related to its regulation of the 5-HT, 5-hydroxyindoleacetic acid (5-HIAA), and glutamate (Glu) levels (66). The effect of Ven on 5-HT, 5-HIAA, and Glu of CPZ-treated mice still needs further observation. Furthermore, Moles et al. reported that the Shannon index was increased at the beginning and progressively decreased from week 2 during CPZ feeding, whereas microbial richness seems to increase during the CPZ intake (37). It is well known that C57BL/6 mouse fed with 0.2% (w/w) cuprizone induced the demyelination process, which can be detectable after 3 weeks of treatment and was complete at week 5. Then, the remyelinated axons appeared between weeks 5 and 6 (67). It suggests that the impact of CPZ on microbial α-diversity is inconsistent at different stages of demyelination/remyelination and the abnormal β-diversity persists during demyelination in CPZ-treated mice. Given that this study only observed diversity after 5 weeks of demyelination, the impact of different CPZ intervention durations on microbial diversity remains to be studied.
LEfSe analysis was performed to reveal changes in specific bacteria among the three groups. The results showed that 17 genera were enriched in the CPZ group, which were related to metabolism and inflammation—for example, Lachnospiraceae_FCS020_group was increased in patients with chronic kidney disease (68), Ruminococcus_1 was positively correlated with the expression levels of proinflammatory cytokines tumor necrosis factor α (TNF-α) and interleukin-1β (IL-1β) in the offspring brain of maternal sleep deprivation rats (69), whereas Candidatus_Stoquefichus was negatively correlated with both TNF-α and IL-4 concentration during postpartum uterine recovery in mice (70). Meanwhile, 15 genera (such as Lactobacillus and Bifidobacterium) were enriched in the CPZ + Ven group. Lactobacillus and Bifidobacterium are probiotics (71), indicating that the regulatory effect of Ven on the gut microbiota of CPZ-treated mice might be related to upregulating the relative abundance of probiotics. However, the relationship between these changed bacteria and the efficacy of Ven is still unclear, and its mechanism of action needs further clarification.
Furthermore, genera Dubosiella was enriched in the CPZ group, and its relative abundance was negatively correlated with sucrose preference rate and was positively correlated with immobility time. A previous study indicated that Dubosiella is related to SCFAs produced, and Dubosiella newyorkensis, a strain of Dubosiella, possessed a strong effect of increasing superoxide dismutase (SOD) in aged mice (72). Therefore, the effect of CPZ on demyelination and depressive-like behaviors may be related to the excessive oxidative stress and inflammation. Besides this, genus Bifidobacterium was enriched in the CPZ + Ven group, and its relative abundance was negatively correlated with immobility time. Genera Alistipes, Blautia, and Muribaculum were enriched in the control group, and their relative abundance was positively or negatively correlated with depressive-like behaviors. Given that Bifidobacterium and Blautia are well-known probiotics (73, 74) and the decrease of Muribaculum was related to acute sleep deprivation-induced psychological defects (75), these results indicate that CPZ may lead to a depressive-like behavior by reducing the beneficial bacteria, and Bifidobacterium might be the key bacteria involved in the antidepressant effect of Ven. Nevertheless, it is a limitation in such a way that we did not clarify which species are affected by CPZ or Ven—for example, there is contrasting evidence reported that Alistipes may have protective effects against cancer immunotherapy (76), liver fibrosis (77), and cardiovascular disease (78). However, Alistipes is also pathogenic in depression (79) and colorectal cancer (80). Since genus Alistipes consists of 13 species, the species that causes this functional difference may be more critical. Similarly, a previous study found that Lactobacillus rhamnosus reduces stress-induced anxiety- and depression-related behaviors by regulating the central GABA receptor expression (81). In contrast, another study found that Lactobacillus reuteri and Lactobacillus intestinalis induces depression phenotypes in antibiotic-treated mice (82). Therefore, different species of the same genus have inconsistent or even opposite functions, and future research needs to be precise to the level of species.
The individual differences in animal behavior are also worth exploring—for example, some mice were able to develop a sucrose preference in the CPZ group, while individual mice in the control group did not develop the sucrose preference, although there is a significant difference in sucrose preference between the two groups. These differences might be attributed to the plasticity or flexibility of individual behavior, which was related to a series of factors such as gene modification, neural circuit function, and stress regulation (83, 84). Meanwhile, behavioral detection methods, such as sucrose preference and tail suspension experiments, are both methods to detect a depressive-like behavior and forms to cope with stress in mice (85). Further research is needed to investigate the effects of these stresses on brain function and myelin integrity.
In conclusion, the present study suggests that CPZ induces disruption of the gut microbiota in mice, including characteristic changes in community diversity, taxon abundance, and depressive-like behaviors. Ven ameliorates CPZ-induced depressive-like behaviors and upregulates the relative abundance of probiotics, such as Bifidobacterium and Lactobacillus. However, the specific mechanism by which Ven affects the microbiota and the correlation between microbiota changes and myelin repair still needs to be elucidated. Further observation of the influence of Ven on peripheral and brain monoamine neurotransmitters, fatty acids, inflammatory response, and oxidative stress levels will help explain this effect. Nonetheless, the mice were group-housed in cages with unrestricted access to standard powdered rodent chow that contained CPZ (0.2% w/w) in the present study, which was performed according to previous studies. We cannot be sure that all the animals inside the cage have submitted to the same levels of CPZ. On the other hand, although single-cage feeding can accurately evaluate the intake of CPZ, long-term single-cage feeding is also a strong negative stress, and it is difficult to rule out the impact on mouse behavior. It is worth noting that the effects of single-cage and group-housed feeding of CPZ on mouse demyelination and behavior need to be further explored. Finally, several shortcomings of this study need to be pointed out. Firstly, the impact of different doses and duration of Ven on the microbiota of normal or CPZ-treated mice is not yet clear. Secondly, we did not observe whether the effect of Ven on the microbiota was related to its myelin-protective effect. Moreover, the molecular mechanisms by which Ven regulates the gut microbiota is still unclear. Further investigations need to observe the impact of Ven on microbial communities at the species level and further explore its potential mechanisms of action.
Data availability statement
The original contributions presented in the study are included in the article/Supplementary Material. Further inquiries can be directed to the corresponding authors.
Ethics statement
The animal study was approved by the Animal Experimental Welfare and Ethical Inspection of Air Force Medical University. The study was conducted in accordance with the local legislation and institutional requirements.
Author contributions
NT: Conceptualization, Formal Analysis, Funding acquisition, Project administration, Supervision, Writing – review & editing, Writing – original draft. CD: Conceptualization, Funding acquisition, Supervision, Writing – review & editing. TZ: Data curation, Formal Analysis, Methodology, Writing – original draft. CF: Data curation, Formal Analysis, Supervision, Writing – review & editing. QS: Data curation, Formal Analysis, Investigation, Writing – review & editing. ZC: Data curation, Formal Analysis, Writing – review & editing. XS: Data curation, Formal Analysis, Writing – review & editing. YL: Data curation, Formal Analysis, Writing – review & editing. GD: Data curation, Formal Analysis, Writing – review & editing. XZ: Conceptualization, Project administration, Supervision, Writing – review & editing.
Funding
The author(s) declare that financial support was received for the research, authorship, and/or publication of this article. This study was financially supported by Scientific Research Foundation of Administration of Traditional Chinese Medicine of Hebei (number 2021360) and the Natural Science Foundation of Nanping City (number N2021J037).
Conflict of interest
The authors declare that the research was conducted in the absence of any commercial or financial relationships that could be construed as a potential conflict of interest.
Publisher’s note
All claims expressed in this article are solely those of the authors and do not necessarily represent those of their affiliated organizations, or those of the publisher, the editors and the reviewers. Any product that may be evaluated in this article, or claim that may be made by its manufacturer, is not guaranteed or endorsed by the publisher.
Supplementary material
The Supplementary Material for this article can be found online at: https://www.frontiersin.org/articles/10.3389/fpsyt.2024.1347867/full#supplementary-material
Supplementary Table 1 | Comparison of differences in microbial communities at different levels among three groups.
References
1. Herrman H, Kieling C, McGorry P, Horton R, Sargent J, Patel V. Reducing the global burden of depression: a Lancet-World Psychiatric Association Commission. Lancet. (2019) 393:e42–43. doi: 10.1016/S0140–6736(18)32408–5
2. Silverstein B, Ajdacic-Gross V, Rossler W, Angst J. The gender difference in depressive prevalence is due to high prevalence of somatic depression among women who do not have depressed relatives. J Affect Disord. (2017) 210:269–72. doi: 10.1016/j.jad.2017.01.006
3. Wang J, Wu X, Lai W, Long E, Zhang X, Li W, et al. Prevalence of depression and depressive symptoms among outpatients: a systematic review and meta-analysis. BMJ Open. (2017) 7:e17173. doi: 10.1136/bmjopen-2017–017173
4. Ledford H. Medical research: if depression were cancer. Nature. (2014) 515:182–84. doi: 10.1038/515182a
5. Monroe SM, Harkness KL. Major depression and its recurrences: life course matters. Annu Rev Clin Psychol. (2022) 18:329–57. doi: 10.1146/annurev-clinpsy-072220–021440
6. Regenold WT, Phatak P, Marano CM, Gearhart L, Viens CH, Hisley KC. Myelin staining of deep white matter in the dorsolateral prefrontal cortex in schizophrenia, bipolar disorder, and unipolar major depression. Psychiatry Res. (2007) 151:179–88. doi: 10.1016/j.psychres.2006.12.019
7. Tham MW, Woon PS, Sum MY, Lee TS, Sim K. White matter abnormalities in major depression: evidence from post-mortem, neuroimaging and genetic studies. J Affect Disord. (2011) 132:26–36. doi: 10.1016/j.jad.2010.09.013
8. Gao Y, Ma J, Tang J, Liang X, Huang CX, Wang SR, et al. White matter atrophy and myelinated fiber disruption in a rat model of depression. J Comp Neurol. (2017) 525:1922–33. doi: 10.1002/cne.24178
9. Wang J, Qiao J, Zhang Y, Wang H, Zhu S, Zhang H, et al. Desvenlafaxine prevents white matter injury and improves the decreased phosphorylation of the rate-limiting enzyme of cholesterol synthesis in a chronic mouse model of depression. J Neurochem. (2014) 131:229–38. doi: 10.1111/jnc.12792
10. Rocca MA, Pravata E, Valsasina P, Radaelli M, Colombo B, Vacchi L, et al. Hippocampal-DMN disconnectivity in MS is related to WM lesions and depression. Hum Brain Mapp. (2015) 36:5051–63. doi: 10.1002/hbm.22992
11. Wu JY, Zhang Y, Wu WB, Hu G, Xu Y. Impaired long contact white matter fibers integrity is related to depression in Parkinson's disease. CNS Neurosci Ther. (2018) 24:108–14. doi: 10.1111/cns.12778
12. Zhou H, Zhong X, Chen B, Wang Q, Zhang M, Mai N, et al. Elevated homocysteine levels, white matter abnormalities and cognitive impairment in patients with late-life depression. Front Aging Neurosci. (2022) 14:931560. doi: 10.3389/fnagi.2022.931560
13. Greenberg T, Bertocci MA, Versace A, Lima SJ, Chase HW, Siffler R, et al. Depression and anxiety mediate the relationship between frontotemporal white matter integrity and quality of life in distressed young adults. J Psychiatr Res. (2021) 132:55–9. doi: 10.1016/j.jpsychires.2020.10.001
14. Zhou B, Zhu Z, Ransom BR, Tong X. Oligodendrocyte lineage cells and depression. Mol Psychiatry. (2021) 26:103–17. doi: 10.1038/s41380–020-00930–0
15. Liang S, Wu X, Hu X, Wang T, Jin F. Recognizing depression from the microbiota(-)Gut(-)Brain axis. Int J Mol Sci. (2018) 19:16. doi: 10.3390/ijms19061592
16. Suda K, Matsuda K. How microbes affect depression: underlying mechanisms via the gut-brain axis and the modulating role of probiotics. Int J Mol Sci. (2022) 23:1172. doi: 10.3390/ijms23031172
17. Zheng P, Yang J, Li Y, Wu J, Liang W, Yin B, et al. Gut microbial signatures can discriminate unipolar from bipolar depression. Adv Sci (Weinh). (2020) 7:1902862. doi: 10.1002/advs.201902862
18. Yang Z, Li J, Gui X, Shi X, Bao Z, Han H, et al. Updated review of research on the gut microbiota and their relation to depression in animals and human beings. Mol Psychiatry. (2020) 25:2759–72. doi: 10.1038/s41380–020-0729–1
19. Song J, Zhou B, Kan J, Liu G, Zhang S, Si L, et al. Gut microbiota: Linking nutrition and perinatal depression. Front Cell Infect Microbiol. (2022) 12:932309. doi: 10.3389/fcimb.2022.932309
20. Chen YH, Xue F, Yu SF, Li XS, Liu L, Jia YY, et al. Gut microbiota dysbiosis in depressed women: The association of symptom severity and microbiota function. J Affect Disord. (2021) 282:391–400. doi: 10.1016/j.jad.2020.12.143
21. Hung CC, Lee YH, Kuo YM, Hsu PC, Tsay HJ, Hsu YT, et al. Soluble epoxide hydrolase modulates immune responses in activated astrocytes involving regulation of STAT3 activity. J Neuroinflamm. (2019) 16:123. doi: 10.1186/s12974–019-1508–2
22. Tian P, Chen Y, Zhu H, Wang L, Qian X, Zou R, et al. Bifidobacterium breve CCFM1025 attenuates major depression disorder via regulating gut microbiome and tryptophan metabolism: A randomized clinical trial. Brain Behav Immun. (2022) 100:233–41. doi: 10.1016/j.bbi.2021.11.023
23. Kelly JR, Borre Y, O' BC, Patterson E, El AS, Deane J, et al. Transferring the blues: Depression-associated gut microbiota induces neurobehavioural changes in the rat. J Psychiatr Res. (2016) 82:109–18. doi: 10.1016/j.jpsychires.2016.07.019
24. Tillmann S, Abildgaard A, Winther G, Wegener G. Altered fecal microbiota composition in the Flinders sensitive line rat model of depression. Psychopharmacol (Berl). (2019) 236:1445–57. doi: 10.1007/s00213–018-5094–2
25. Zheng P, Zeng B, Zhou C, Liu M, Fang Z, Xu X, et al. Gut microbiome remodeling induces depressive-like behaviors through a pathway mediated by the host's metabolism. Mol Psychiatry. (2016) 21:786–96. doi: 10.1038/mp.2016.44
26. Zhang Y, Fan Q, Hou Y, Zhang X, Yin Z, Cai X, et al. Bacteroides species differentially modulate depression-like behavior via gut-brain metabolic signaling. Brain Behav Immun. (2022) 102:11–22. doi: 10.1016/j.bbi.2022.02.007
27. Tian P, O'Riordan KJ, Lee YK, Wang G, Zhao J, Zhang H, et al. Towards a psychobiotic therapy for depression: Bifidobacterium breve CCFM1025 reverses chronic stress-induced depressive symptoms and gut microbial abnormalities in mice. Neurobiol Stress. (2020) 12:100216. doi: 10.1016/j.ynstr.2020.100216
28. Li N, Wang Q, Wang Y, Sun A, Lin Y, Jin Y, et al. Fecal microbiota transplantation from chronic unpredictable mild stress mice donors affects anxiety-like and depression-like behavior in recipient mice via the gut microbiota-inflammation-brain axis. Stress. (2019) 22:592–602. doi: 10.1080/10253890.2019.1617267
29. Ding Y, Bu F, Chen T, Shi G, Yuan X, Feng Z, et al. A next-generation probiotic: Akkermansia muciniphila ameliorates chronic stress-induced depressive-like behavior in mice by regulating gut microbiota and metabolites. Appl Microbiol Biotechnol. (2021) 105:8411–26. doi: 10.1007/s00253–021-11622–2
30. Zhou M, Fan Y, Xu L, Yu Z, Wang S, Xu H, et al. Microbiome and tryptophan metabolomics analysis in adolescent depression: roles of the gut microbiota in the regulation of tryptophan-derived neurotransmitters and behaviors in human and mice. Microbiome. (2023) 11:145. doi: 10.1186/s40168–023-01589–9
31. Gao M, Tu H, Liu P, Zhang Y, Zhang R, Jing L, et al. Association analysis of gut microbiota and efficacy of SSRIs antidepressants in patients with major depressive disorder. J Affect Disord. (2023) 330:40–7. doi: 10.1016/j.jad.2023.02.143
32. Sanada K, Nakajima S, Kurokawa S, Barcelo-Soler A, Ikuse D, Hirata A, et al. Gut microbiota and major depressive disorder: A systematic review and meta-analysis. J Affect Disord. (2020) 266:1–13. doi: 10.1016/j.jad.2020.01.102
33. Valles-Colomer M, Falony G, Darzi Y, Tigchelaar EF, Wang J, Tito RY, et al. The neuroactive potential of the human gut microbiota in quality of life and depression. Nat Microbiol. (2019) 4:623–32. doi: 10.1038/s41564-018-0337-x
34. Chen T, Noto D, Hoshino Y, Mizuno M, Miyake S. Butyrate suppresses demyelination and enhances remyelination. J Neuroinflamm. (2019) 16:165. doi: 10.1186/s12974-019-1552-y
35. Anbalagan S. Endocrine cross-talk between the gut microbiome and glial cells in development and disease. J Neuroendocrinol. (2021) 33:e12924. doi: 10.1111/jne.12924
36. Stanisavljevic S, Lukic J, Momcilovic M, Miljkovic M, Jevtic B, Kojic M, et al. Gut-associated lymphoid tissue, gut microbes and susceptibility to experimental autoimmune encephalomyelitis. Benef Microbes. (2016) 7:363–73. doi: 10.3920/BM2015.0159
37. Moles L, Egimendia A, Osorio-Querejeta I, Iparraguirre L, Alberro A, Suarez J, et al. Gut microbiota changes in experimental autoimmune encephalomyelitis and cuprizone mice models. ACS Chem Neurosci. (2021) 12:893–905. doi: 10.1021/acschemneuro.0c00695
38. McLean PG, Borman RA, Lee K. 5-HT in the enteric nervous system: gut function and neuropharmacology. Trends Neurosci. (2007) 30:9–13. doi: 10.1016/j.tins.2006.11.002
39. Grimaldi B, Fillion G. 5-HT-moduline controls serotonergic activity: implication in neuroimmune reciprocal regulation mechanisms. Prog Neurobiol. (2000) 60:1–12. doi: 10.1016/s0301–0082(98)00074–4
40. Wang CH, Gu JY, Zhang XL, Dong J, Yang J, Zhang YL, et al. Venlafaxine ameliorates the depression-like behaviors and hippocampal S100B expression in a rat depression model. Behav Brain Funct. (2016) 12:34. doi: 10.1186/s12993-016-0116-x
41. Zhang Y, Bi X, Adebiyi O, Wang J, Mooshekhian A, Cohen J, et al. Venlafaxine improves the cognitive impairment and depression-like behaviors in a cuprizone mouse model by alleviating demyelination and neuroinflammation in the brain. Front Pharmacol. (2019) 10:332. doi: 10.3389/fphar.2019.00332
42. Lukic I, Getselter D, Ziv O, Oron O, Reuveni E, Koren O, et al. Antidepressants affect gut microbiota and Ruminococcus flavefaciens is able to abolish their effects on depressive-like behavior. Transl Psychiatry. (2019) 9:133. doi: 10.1038/s41398-019-0466-x
43. Zirngibl M, Assinck P, Sizov A, Caprariello AV, Plemel JR. Oligodendrocyte death and myelin loss in the cuprizone model: an updated overview of the intrinsic and extrinsic causes of cuprizone demyelination. Mol Neurodegener. (2022) 17:34. doi: 10.1186/s13024–022-00538–8
44. Hiremath MM, Saito Y, Knapp GW, Ting JP, Suzuki K, Matsushima GK. Microglial/macrophage accumulation during cuprizone-induced demyelination in C57BL/6 mice. J Neuroimmunol. (1998) 92:38–49. doi: 10.1016/s0165–5728(98)00168–4
45. Liu Q, Lv HW, Yang S, He YQ, Ma QR, Liu J. NEP1–40 alleviates behavioral phenotypes and promote oligodendrocyte progenitor cell differentiation in the hippocampus of cuprizone-induced demyelination mouse model. Neurosci Lett. (2020) 725:134872. doi: 10.1016/j.neulet.2020.134872
46. Wang X, Chang L, Wan X, Tan Y, Qu Y, Shan J, et al. (R)-ketamine ameliorates demyelination and facilitates remyelination in cuprizone-treated mice: A role of gut-microbiota-brain axis. Neurobiol Dis. (2022) 165:105635. doi: 10.1016/j.nbd.2022.105635
47. Sen MK, Almuslehi M, Coorssen JR, Mahns DA, Shortland PJ. Behavioural and histological changes in cuprizone-fed mice. Brain Behav Immun. (2020) 87:508–23. doi: 10.1016/j.bbi.2020.01.021
48. Wang HN, Liu GH, Zhang RG, Xue F, Wu D, Chen YC, et al. Quetiapine ameliorates schizophrenia-like behaviors and protects myelin integrity in cuprizone intoxicated mice: the involvement of notch signaling pathway. Int J Neuropsychopharmacol. (2015) 19:pyv088. doi: 10.1093/ijnp/pyv088
49. Zhou CH, Chen YH, Xue SS, Shi QQ, Guo L, Yu H, et al. rTMS ameliorates depressive-like behaviors and regulates the gut microbiome and medium- and long-chain fatty acids in mice exposed to chronic unpredictable mild stress. CNS Neurosci Ther. (2023) 29:3549–66. doi: 10.1111/cns.14287
50. Ghaiad HR, Nooh MM, El-Sawalhi MM, Shaheen AA. Resveratrol promotes remyelination in Cuprizone model of multiple sclerosis: biochemical and histological study. Mol Neurobiol. (2017) 54:3219–29. doi: 10.1007/s12035–016-9891–5
51. Greenberg P, Chitnis A, Louie D, Suthoff E, Chen SY, Maitland J, et al. The economic burden of adults with major depressive disorder in the United States (2019). Adv Ther. (2023) 40:4460–79. doi: 10.1007/s12325–023-02622-x
52. Johnston KM, Powell LC, Anderson IM, Szabo S, Cline S. The burden of treatment-resistant depression: A systematic review of the economic and quality of life literature. J Affect Disord. (2019) 242:195–210. doi: 10.1016/j.jad.2018.06.045
53. de Vos WM, Tilg H, Van Hul M, Cani PD. Gut microbiome and health: mechanistic insights. Gut. (2022) 71:1020–32. doi: 10.1136/gutjnl-2021–326789
54. Puschhof J, Pleguezuelos-Manzano C, Clevers H. Organoids and organs-on-chips: Insights into human gut-microbe interactions. Cell Host Microbe. (2021) 29:867–78. doi: 10.1016/j.chom.2021.04.002
55. Chang L, Wei Y, Hashimoto K. Brain-gut-microbiota axis in depression: A historical overview and future directions. Brain Res Bull. (2022) 182:44–56. doi: 10.1016/j.brainresbull.2022.02.004
56. Du Y, Gao XR, Peng L, Ge JF. Crosstalk between the microbiota-gut-brain axis and depression. Heliyon. (2020) 6:e4097. doi: 10.1016/j.heliyon.2020.e04097
57. Vega-Riquer JM, Mendez-Victoriano G, Morales-Luckie RA, Gonzalez-Perez O. Five decades of cuprizone, an updated model to replicate demyelinating diseases. Curr Neuropharmacol. (2019) 17:129–41. doi: 10.2174/1570159X15666170717120343
58. Paton KF, Hong S, Biggerstaff A, Kivell BM. Sex differences in the behavioural aspects of the cuprizone-induced demyelination model in mice. Brain Sci. (2022) 12:1678. doi: 10.3390/brainsci12121687
59. Mooshekhian A, Sandini T, Wei Z, Van Bruggen R, Li H, Li XM, et al. Low-field magnetic stimulation improved cuprizone-induced depression-like symptoms and demyelination in female mice. Exp Ther Med. (2022) 23:210. doi: 10.3892/etm.2022.11133
60. Solaro C, Gamberini G, Masuccio FG. Depression in multiple sclerosis: epidemiology, aetiology, diagnosis and treatment. CNS Drugs. (2018) 32:117–33. doi: 10.1007/s40263–018-0489–5
61. Morris G, Reiche E, Murru A, Carvalho AF, Maes M, Berk M, et al. Multiple immune-inflammatory and oxidative and nitrosative stress pathways explain the frequent presence of depression in multiple sclerosis. Mol Neurobiol. (2018) 55:6282–306. doi: 10.1007/s12035–017-0843–5
62. Wang H. MicroRNAs, multiple sclerosis, and depression. Int J Mol Sci. (2021) 22:7802. doi: 10.3390/ijms22157802
63. Sen MK, Mahns DA, Coorssen JR, Shortland PJ. The roles of microglia and astrocytes in phagocytosis and myelination: Insights from the cuprizone model of multiple sclerosis. Glia. (2022) 70:1215–50. doi: 10.1002/glia.24148
64. Galecki P, Mossakowska-Wojcik J, Talarowska M. The anti-inflammatory mechanism of antidepressants - SSRIs, SNRIs. Prog Neuropsychopharmacol Biol Psychiatry. (2018) 80:291–94. doi: 10.1016/j.pnpbp.2017.03.016
65. Wang X, Eguchi A, Yang Y, Chang L, Wan X, Shan J, et al. Key role of the gut-microbiota-brain axis via the subdiaphragmatic vagus nerve in demyelination of the cuprizone-treated mouse brain. Neurobiol Dis. (2023) 176:105951. doi: 10.1016/j.nbd.2022.105951
66. Shen W, Tao Y, Zheng F, Zhou H, Wu H, Shi H, et al. The alteration of gut microbiota in venlafaxine-ameliorated chronic unpredictable mild stress-induced depression in mice. Behav Brain Res. (2023) 446:114399. doi: 10.1016/j.bbr.2023.114399
67. Gudi V, Gingele S, Skripuletz T, Stangel M. Glial response during cuprizone-induced de- and remyelination in the CNS: lessons learned. Front Cell Neurosci. (2014) 8:73. doi: 10.3389/fncel.2014.00073
68. Chao YT, Lin YK, Chen LK, Huang P, Hsu YC. Role of the gut microbiota and their metabolites in hemodialysis patients. Int J Med Sci. (2023) 20:725–36. doi: 10.7150/ijms.82667
69. Yao ZY, Li XH, Zuo L, Xiong Q, He WT, Li DX, et al. Maternal sleep deprivation induces gut microbial dysbiosis and neuroinflammation in offspring rats. Zool Res. (2022) 43:380–90. doi: 10.24272/j.issn.2095–8137.2022.023
70. Yang X, He Z, Hu R, Yan J, Zhang Q, Li B, et al. Dietary beta-carotene on postpartum uterine recovery in mice: crosstalk between gut microbiota and inflammation. Front Immunol. (2021) 12:744425. doi: 10.3389/fimmu.2021.744425
71. McNamara MP, Singleton JM, Cadney MD, Ruegger PM, Borneman J, Garland T. Early-life effects of juvenile Western diet and exercise on adult gut microbiome composition in mice. J Exp Biol. (2021) 224:jeb239699. doi: 10.1242/jeb.239699
72. Liu TH, Wang J, Zhang CY, Zhao L, Sheng YY, Tao GS, et al. Gut microbial characteristical comparison reveals potential anti-aging function of Dubosiella newyorkensis in mice. Front Endocrinol (Lausanne). (2023) 14:1133167. doi: 10.3389/fendo.2023.1133167
73. Liu X, Mao B, Gu J, Wu J, Cui S, Wang G, et al. Blautia-a new functional genus with potential probiotic properties? Gut Microbes. (2021) 13:1–21. doi: 10.1080/19490976.2021.1875796
74. Wieers G, Verbelen V, Van Den Driessche M, Melnik E, Vanheule G, Marot JC, et al. Do probiotics during in-hospital antibiotic treatment prevent colonization of gut microbiota with multi-drug-resistant bacteria? A randomized placebo-controlled trial comparing saccharomyces to a mixture of lactobacillus, bifidobacterium, and saccharomyces. Front Public Health. (2020) 8:578089. doi: 10.3389/fpubh.2020.578089
75. Yang DF, Huang WC, Wu CW, Huang CY, Yang Y, Tung YT. Acute sleep deprivation exacerbates systemic inflammation and psychiatry disorders through gut microbiota dysbiosis and disruption of circadian rhythms. Microbiol Res. (2023) 268:127292. doi: 10.1016/j.micres.2022.127292
76. Iida N, Dzutsev A, Stewart CA, Smith L, Bouladoux N, Weingarten RA, et al. Commensal bacteria control cancer response to therapy by modulating the tumor microenvironment. Science. (2013) 342:967–70. doi: 10.1126/science.1240527
77. Rau M, Rehman A, Dittrich M, Groen AK, Hermanns HM, Seyfried F, et al. Fecal SCFAs and SCFA-producing bacteria in gut microbiome of human NAFLD as a putative link to systemic T-cell activation and advanced disease. United Eur Gastroenterol J. (2018) 6:1496–507. doi: 10.1177/2050640618804444
78. Zuo K, Li J, Li K, Hu C, Gao Y, Chen M, et al. Disordered gut microbiota and alterations in metabolic patterns are associated with atrial fibrillation. Gigascience. (2019) 8:giz058. doi: 10.1093/gigascience/giz058
79. Jiang H, Ling Z, Zhang Y, Mao H, Ma Z, Yin Y, et al. Altered fecal microbiota composition in patients with major depressive disorder. Brain Behav Immun. (2015) 48:186–94. doi: 10.1016/j.bbi.2015.03.016
80. Thanikachalam K, Khan G. Colorectal cancer and nutrition. Nutrients. (2019) 11:164. doi: 10.3390/nu11010164
81. Bravo JA, Forsythe P, Chew MV, Escaravage E, Savignac HM, Dinan TG, et al. Ingestion of Lactobacillus strain regulates emotional behavior and central GABA receptor expression in a mouse via the vagus nerve. Proc Natl Acad Sci U S A. (2011) 108:16050–55. doi: 10.1073/pnas.1102999108
82. Wang S, Ishima T, Zhang J, Qu Y, Chang L, Pu Y, et al. Ingestion of Lactobacillus intestinalis and Lactobacillus reuteri causes depression- and anhedonia-like phenotypes in antibiotic-treated mice via the vagus nerve. J Neuroinflamm. (2020) 17:241. doi: 10.1186/s12974-020-01916-z
83. Bialek W, Shaevitz JW. Long timescales, individual differences, and scale invariance in animal behavior. Phys Rev Lett. (2024) 132:48401. doi: 10.1103/PhysRevLett.132.048401
84. Stamps JA. Individual differences in behavioural plasticities. Biol Rev Camb Philos Soc. (2016) 91:534–67. doi: 10.1111/brv.12186
Keywords: depressive-like behavior, cuprizone, gut microbiota, venlafaxine, depression
Citation: Du C, Zhang T, Feng C, Sun Q, Chen Z, Shen X, Liu Y, Dai G, Zhang X and Tang N (2024) The effects of venlafaxine on depressive-like behaviors and gut microbiome in cuprizone-treated mice. Front. Psychiatry 15:1347867. doi: 10.3389/fpsyt.2024.1347867
Received: 01 December 2023; Accepted: 13 May 2024;
Published: 03 June 2024.
Edited by:
Elaine C. Gavioli, Federal University of Rio Grande do Norte, BrazilReviewed by:
Victor Duarte Holanda, Johns Hopkins University, United StatesLaila Asth, University of Ferrara, Italy
Copyright © 2024 Du, Zhang, Feng, Sun, Chen, Shen, Liu, Dai, Zhang and Tang. This is an open-access article distributed under the terms of the Creative Commons Attribution License (CC BY). The use, distribution or reproduction in other forums is permitted, provided the original author(s) and the copyright owner(s) are credited and that the original publication in this journal is cited, in accordance with accepted academic practice. No use, distribution or reproduction is permitted which does not comply with these terms.
*Correspondence: Nailong Tang, tangfmmu@163.com; Xuan Zhang, zhangxuan@301hospital.com.cn
†These authors have contributed equally to this work