- 1Research and Development Service, Veterans Affairs Portland Health Care System, Portland, OR, United States
- 2Department of Psychiatry, Oregon Health & Science University, Portland, OR, United States
- 3Department of Behavioral Neuroscience, Oregon Health & Science University, Portland, OR, United States
- 4Methamphetamine Research Center, Oregon Health & Science University, Portland, OR, United States
- 5Department of Pharmacology, Toxicology and Neuroscience, Louisiana State University Health Sciences Center at Shreveport, Shreveport, LA, United States
- 6Louisiana Addiction Research Center, Louisiana State University Health Sciences Center at Shreveport, Shreveport, LA, United States
- 7Department of Psychiatry and Behavioral Medicine, Louisiana State University Health Sciences Center at Shreveport, Shreveport, LA, United States
- 8Department of Neurology, Oregon Health & Science University, Portland, OR, United States
- 9Department of Molecular Microbiology and Immunology, Oregon Health & Science University, Portland, OR, United States
- 10Virogenomics BioDevelopment, Inc., Portland, OR, United States
Introduction: Currently, there are no FDA-approved medications to treat methamphetamine addiction, including the inflammatory, neurotoxic, and adverse neuropsychiatric effects. We have shown that partial (p)MHC class II constructs (i.e., Recombinant T-cell receptor Ligand – RTL1000), comprised of the extracellular α1 and β1 domains of MHC class II molecules linked covalently to myelin oligodendrocyte glycoprotein (MOG)-35-55 peptide, can address the neuroimmune effects of methamphetamine addiction through its ability to bind to and down-regulate CD74 expression, block macrophage migration inhibitory factor (MIF) signaling, and reduce levels of pro-inflammatory chemokine ligand 2 (CCL2). The present study evaluated the effects of our third-generation pMHC II construct, DRmQ, on cognitive function and concentration of inflammatory cytokines in the frontal cortex, a region critical for cognitive functions such as memory, impulse control, and problem solving.
Methods: Female and male C57BL/6J mice were exposed to methamphetamine (or saline) via subcutaneous (s.c.) injections administered four times per day every other day for 14 days. Following methamphetamine exposure, mice received immunotherapy (DRmQ or ibudilast) or vehicle s.c. injections daily for five days. Cognitive function was assessed using the novel object recognition test (NORT). To evaluate the effects of immunotherapy on inflammation in the frontal cortex, multiplex immunoassays were conducted. ANOVA was used to compare exploration times on the NORT and immune factor concentrations.
Results: Post hoc analysis revealed increased novel object exploration time in MA-DRmQ treated mice, as compared to MA-VEH treated mice (non-significant trend). One-way ANOVA detected a significant difference across the groups in the concentration of macrophage inflammatory protein-2 (MIP-2) (p = 0.03). Post hoc tests indicated that mice treated with methamphetamine and DRmQ or ibudilast had significantly lower levels of MIP-2 in frontal cortex, as compared to mice treated with methamphetamine and vehicle (p > 0.05).
Discussion: By specifically targeting CD74, our DRQ constructs can block the signaling of MIF, inhibiting the downstream signaling and pro-inflammatory effects that contribute to and perpetuate methamphetamine addiction.
1. Introduction
Methamphetamine is an amphetamine-based stimulant drug that can result in psychosis (1), depression (2, 3), anxiety (4, 5), and cognitive deficits (6, 7). Its misuse results in chronic, relapsing diseases that have given methamphetamine use disorders increasing global prevalence. In fact, an estimated 27 million people globally used methamphetamine in 2019 (3). Afflicted individuals often experience a range of neuropsychiatric deficits in episodic memory, executive function, and emotional regulation (7, 8). Consequently, increased relapse rates, lower treatment retention rates, and reduced daily functioning oftentimes result from the mental and behavioral health problems associated with methamphetamine misuse. New interventions are needed to help people regain the functions that methamphetamine altered and refrain from continued use of the drug. A growing body of research illustrates the critical role that neuroimmune pathways play in the propagation of neuronal injury that leads to the neuropsychiatric symptoms characteristic of methamphetamine use disorders (9–11). Approaches implementing immunotherapeutic, anti-inflammatory strategies in preclinical (12–18) and clinical trials have shown encouraging results in addressing addictive behavior and associated neuropsychiatric impairments (19–21).
In this study, we tested two immunotherapeutic strategies for the treatment of methamphetamine-induced cognitive impairments and inflammation. The first was the mouse-based partial major histocompatibility complex (pMHC) class II construct DRmQ (DRα1(L50Q)-mMOG-35-55) and the second was the phosphodiesterase inhibitor ibudilast (3-isobutyryl-2-isopropylpyrazolo-[1,5-a]pyridine). DRmQ and similar pMHCs have been shown to have neuroprotective and anti-inflammatory effects in several conditions, such as experimental stroke (22) and multiple sclerosis (23). DRmQs bind to and downregulate expression of CD74, a receptor that triggers a signaling cascade leading to neuroinflammation. CD74 is additionally the primary target receptor for macrophage migration inhibitory factor (MIF), a pro-inflammatory cytokine that is responsible for mediating the inflammatory effects in alcohol use disorders (24), depression (25), and neurodegenerative diseases (26). Ibudilast is another anti-inflammatory compound that inhibits MIF (27) and has been tested, with mixed results, for the treatment of methamphetamine use disorder (28, 29). Ibudilast is a non-selective phosphodiesterase inhibitor that also suppresses the production of nitric oxide, reactive oxygen species, interleukin (IL)-1β, IL-6, and tumor necrosis factor-alpha (TNF-α) and enhances the production of anti-inflammatory factors, including nerve growth factor, glia-derived neurotrophic factor, and neurotrophin-4 in activated microglia (30, 31). These mechanisms may be therapeutic, given that methamphetamine can cause persistent microglia activation in humans recently abstinent from methamphetamine use (32).
The purpose of this study was to test the effects of DRmQ and ibudilast in improving cognitive function and reducing inflammation in mice exposed to a neurotoxic methamphetamine regime (described in section 2.2.1 Methamphetamine exposure). To accomplish this goal, mice were exposed to methamphetamine or saline, followed by treatment with either DRmQ, ibudilast, or vehicle and behavioral testing (novel object recognition test). Luminex multiplex assays were used to measure concentrations of pro-and anti-inflammatory cytokines in the frontal cortex, a region critical for addiction-relevant cognitive functions such as memory, impulse control, and problem solving (33–36). Based on past studies testing MHC class II recombinant T-cell receptor ligands (RTLs) (14, 15) and ibudilast (13, 37), we hypothesized that these immunotherapeutic treatments would improve cognitive function and decrease concentrations of inflammatory cytokines, therefore combatting the neurotoxic effects of methamphetamine exposure.
2. Materials and methods
2.1. Animals
Ninety-six female and male C57BL/6 J mice [Jackson Laboratories (Bar Harbor, ME, United States); average age of 3 months and body weights (SD) of 21.5 (3.7) grams (g)] were tested in two cohorts that investigated the effects of immunotherapies (DRmQ or ibudilast) on cognitive function (cohort 1) and inflammation (cohort 2). For cohort 1, 48 male mice were assigned to one of eight treatment groups: (1) MA-VEH1: mice with methamphetamine (MA) exposure and without immunotherapy (received vehicle for DRmQ) (n = 3), (2) MA-VEH2: mice with methamphetamine exposure and without immunotherapy (received vehicle for ibudilast) (n = 3), (3) MA-DRmQ: mice with methamphetamine exposure and DRmQ immunotherapy (n = 8), (4) MA-IBU: mice with methamphetamine exposure and with ibudilast immunotherapy (n = 7). Groups 5–8 were identical to groups 1–4 except that mice were administered saline (SAL) instead of methamphetamine: (5) SAL-VEH1 (n = 4), (6) SAL-VEH2 (n = 4), (7) SAL-DRmQ (n = 8), and (8) SAL-IBU (n = 8). For cohort 2, 48 female mice were assigned to one of eight treatment groups, identical to that of cohort 1: (1) MA-VEH1 (n = 4), (2) MA-VEH2 (n = 4), (3) MA-DRmQ (n = 8), (4) MA-IBU (n = 8), (5) SAL-VEH1 (n = 4), (6) SAL-VEH2 (n = 4), (7) SAL-DRmQ (n = 8), and (8) SAL-IBU (n = 8). For cohort 1, there was attrition within the MA-IBU, MA-VEH1 and MA-VEH2 groups due to morbidity and mortality (n = 1 for each group) (Table 1).
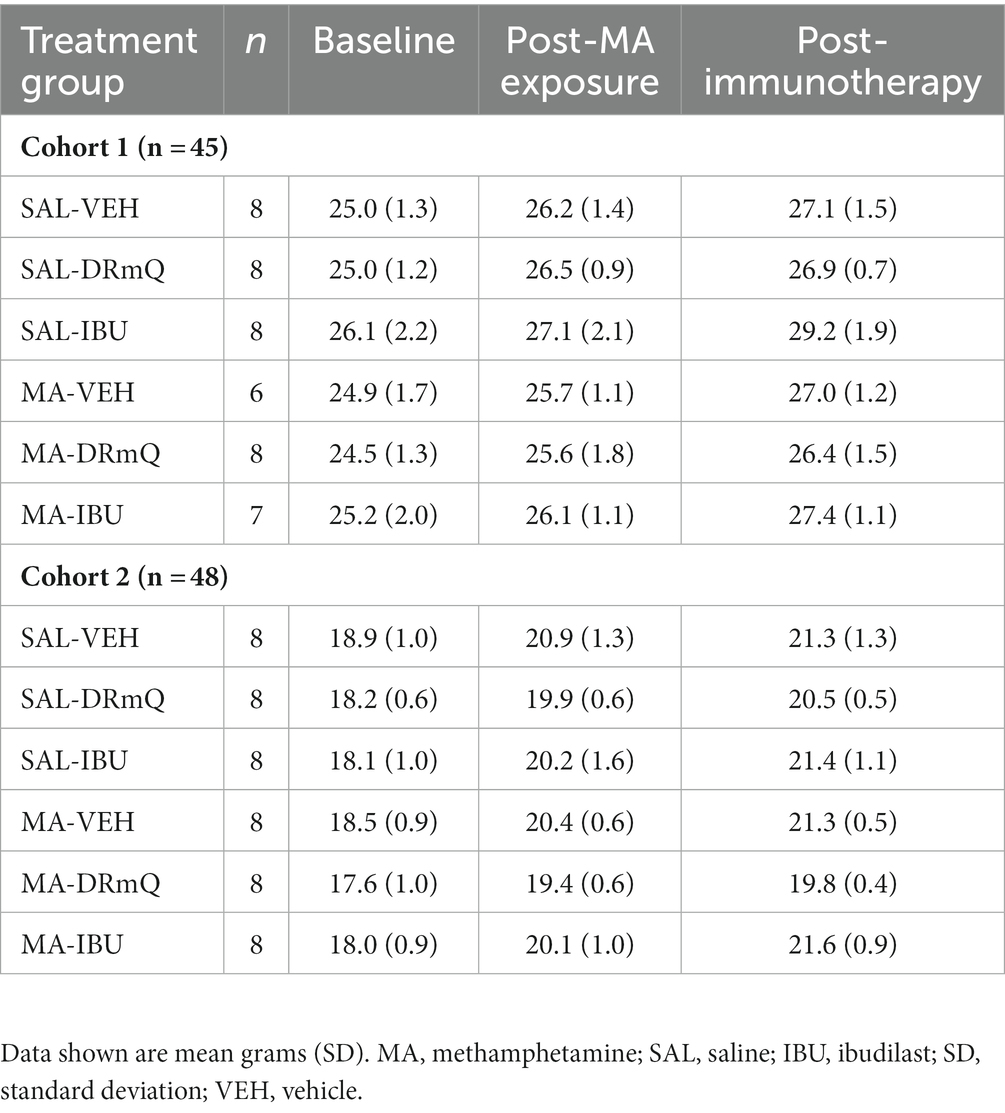
Table 1. Cohort numbers and average weights of mice in the experimental groups before and after methamphetamine exposure and immunotherapy.
2.2. Experimental design
We evaluated the effects of methamphetamine and DRmQ or ibudilast immunotherapy on cognitive function and inflammation when treatment is administered during early remission from repeated binge exposure to methamphetamine (Figure 1). We have used this strategy previously (5, 15), as this parallels an ideal treatment approach in humans where treatment is provided during early recovery from addiction. This model mimics the CNS and neuropsychiatric impairments seen in humans with a history of methamphetamine addiction (3, 5, 33, 38) and provides a measurable signal to treat during an early remission period.
All experimental procedures were approved by the Veterans Affairs Portland Health Care System Institutional Animal Care and Use Committee. Experiments complied with the ARRIVE guidelines (39) and were carried out in accordance with the National Institutes of Health Guide for the Care and Use of Laboratory Animals.
2.2.1. Methamphetamine exposure
Animals were exposed to methamphetamine every other day to model “binge and crash” use in humans [reviewed in (40)], which can result in long-term neural toxicity (41). This repeated methamphetamine exposure procedure using a 10 mg/kg dose recapitulates methamphetamine-induced neurotoxic effects observed in humans, including dopamine toxicity [(e.g., 42)], and has been used previously without adverse consequences to the health of the animals (5, 15). Specifically, mice were exposed to methamphetamine via subcutaneous (s.c.) injections of methamphetamine (10 mg/kg) administered four times per day every other day for 14 days (7 days of methamphetamine exposure). On methamphetamine exposure days, each of the four injections was separated by 2 hours. The s.c. route was used for the methamphetamine injections to reduce potential trauma, as the needle penetrates only the skin. In addition, the rate of absorption for s.c. administration is slower than i.p. increasing the duration of methamphetamine exposure.
2.2.2. Immunotherapy treatment
DRmQ (m = mouse) is comprised of the HLA-DRα1 domain with an L50Q (L = leucine; Q = glutamine) amino acid substitution (to enhance binding affinity for CD74) linked to an autoantigen peptide (myelin oligodendroglial cell glycoprotein, i.e., MOG-35-55 peptide). DRQ was derived by the Vandenbark lab from soluble major histocompatibility complex (MHC) Class II α1-β1-antigenic peptide constructs called Recombinant T-cell receptor Ligands (RTLs). The physical properties and mechanisms of action of RTLs have been described in detail previously [e.g., reviewed in (43)]. Ibudilast was obtained lyophilized (Selleck Chemicals, LLC., Houston, TX, United States) and reconstituted following vendor’s recommendations based on concentration needed for injection and pre-determined solubility information provided upon receipt of product.
Mice received daily s.c. injections of either DRmQ (100 μg), ibudilast (13 mg/kg), or vehicle [DRmQ vehicle: 20 mM Tris, pH 8.5; IBU vehicle: 35% polyethylene glycol (PEG) in saline] daily for 5 days. For cohort 2, the vehicle for ibudilast was modified (changed to 35% PEG in 10% DMSO in saline) to facilitate solubility.
2.2.3. Novel object recognition test
Cognitive function was assessed using the novel object recognition test (NORT), with modifications based on published methods (44, 45). The NORT testing occurred across 3 days and consisted of habituation, training, and retention sessions. During the habituation session, mice were individually habituated to an open-field box (29 cm × 36 cm), without objects for 10 min. Following habituation, two of three objects (i.e., objects of similar size but different in shape) were symmetrically attached to the floor of the box and mice were given 10 min to explore (i.e., training session). Twenty-four hours later mice were placed back in the box for the retention session, and one of the objects was replaced with one not previously encountered. During the retention session, mice were given 5 min to explore. Behavior was recorded and measured using the EthoVision XT 10 video-tracking system (Noldus Information Technology Inc., Leesburg, VA, United States). Exploration of an object was defined as directing the nose at a distance ≤2 cm to the object and/or touching it with the nose, while turning around or sitting on the object was not considered exploration. Novel object exploration time was used to evaluate cognitive function (a measure of recognition memory). Exploratory preferences during the retention session were also calculated as (i) a preference index [amount of time spent exploring the novel object/the total time spent exploring both objects (during the retention session)] and (ii) a discrimination index [(amount of time spent exploring the novel object − familiar object)/(amount of time spent exploring the novel object + familiar object)], according to the method described (46, 47).
2.2.4. Brain tissue preparation
Frontal cortices were microdissected on a cold block, immediately frozen in liquid nitrogen and then stored at −80°C until assayed. Brain tissue lysates were prepared in a 1:20 ratio, using 20 μL of lysis buffer [150 mM sodium chloride, 0.1% Triton X-100, 0.5% sodium deoxycholate, 0.1% SDS, 50 mM Tris-HCl, pH 8.0; Halt™ Protease and Phosphatase Inhibitor Single-Use Cocktail (100X) (Thermo Fisher Scientific, Waltham, MA, United States)] per 1 mg of tissue. Tissue from each animal was homogenized manually in a 1.5 mL Eppendorf tube on ice with a disposable pellet pestle. The samples were centrifuged at 12,000 rpm for 20 min at 4°C. Supernatants were aliquoted to fresh 1.5 mL Eppendorf tubes and used for multiplex immunoassays. Total protein concentration was determined using Pierce™ BCA (bicinchoninic acid) protein assay kits (Thermo Fisher Scientific, Waltham, MA, United States) and absorbance reader (BioRad 680).
2.2.5. Multiplex immunoassay
MILLIPLEX MAP Mouse Cytokine/Chemokine Magnetic Bead Panel Immunology Multiplex Assay (MCYTOMAG-70K, Millipore Sigma, Burlington, MA, United States) was used to measure immune factors with a putative role in methamphetamine-induced CNS effects or CD74 signaling—i.e., monocyte chemoattractant protein-1 (MCP-1, a.k.a. CCL2) (38), interferon-gamma (IFN-γ) (48), IFN-γ induced protein-10 (IP-10) (49), interleukin-10 (IL-10) (50), IL-1β (51), IL-2 (15), macrophage inflammatory protein-1 alpha (MIP-1α) and MIP-2 (52). The immunoassay kit was performed according to the manufacturer’s instructions. Briefly, brain lysate samples were formulized to 2 μg/μL before being added into the 96-well plates (25 μL/well). Equal protein amounts were used in each well. The concentrations of cytokines were determined using a Luminex® 200 system and 5-parameter curve-fitting method, as previously reported (15).
2.2.6. Approach for unbiased data collection
Each mouse was assigned an ID number that did not denote group assignment. This information was uploaded in a secure database. Additionally, male and female research technicians worked with animals throughout experiments to prevent handling bias (53).
2.3. Statistical analyses
GraphPad Prism, version 8.1.2 software (La Jolla, CA, United States) was used for all statistical analyses. One-way analysis of variance (ANOVA) was used to compare body weight, cognitive function, and inflammatory factor concentration among the treatment groups, followed by Holm-Šidák’s multiple comparison or Kolmogorov–Smirnov post hoc tests, when appropriate. A p-value ≤0.05 denoted a statistically significant difference. Initial analyses found no significant differences between the VEH1 and VEH2 groups (as described in section 2.1), so VEH1 and VEH2 groups were combined for final analyses and reporting. Bar graphs shown in the figures illustrate group means ± SEM.
3. Results
3.1. Methamphetamine exposure, immunotherapy, and body weights
The average body weights of mice in the experimental groups before and after methamphetamine exposure and immunotherapy are shown in Table 1. There were no significant differences in body weights among the eight groups for cohorts 1 and 2 at baseline, post-methamphetamine exposure, or post-immunotherapy. Figure 2 shows average body weights over time, illustrating that methamphetamine exposure resulted in an acute sign of stimulant activity for mice in cohort 1 (Figure 2A) but not cohort 2 (Figure 2B). Mice treated with methamphetamine lost an average of 6% of their body weight (1.5 g) during the first 3 days of drug exposure, as compared with saline-treated mice whose weights remained stable during the same time period.
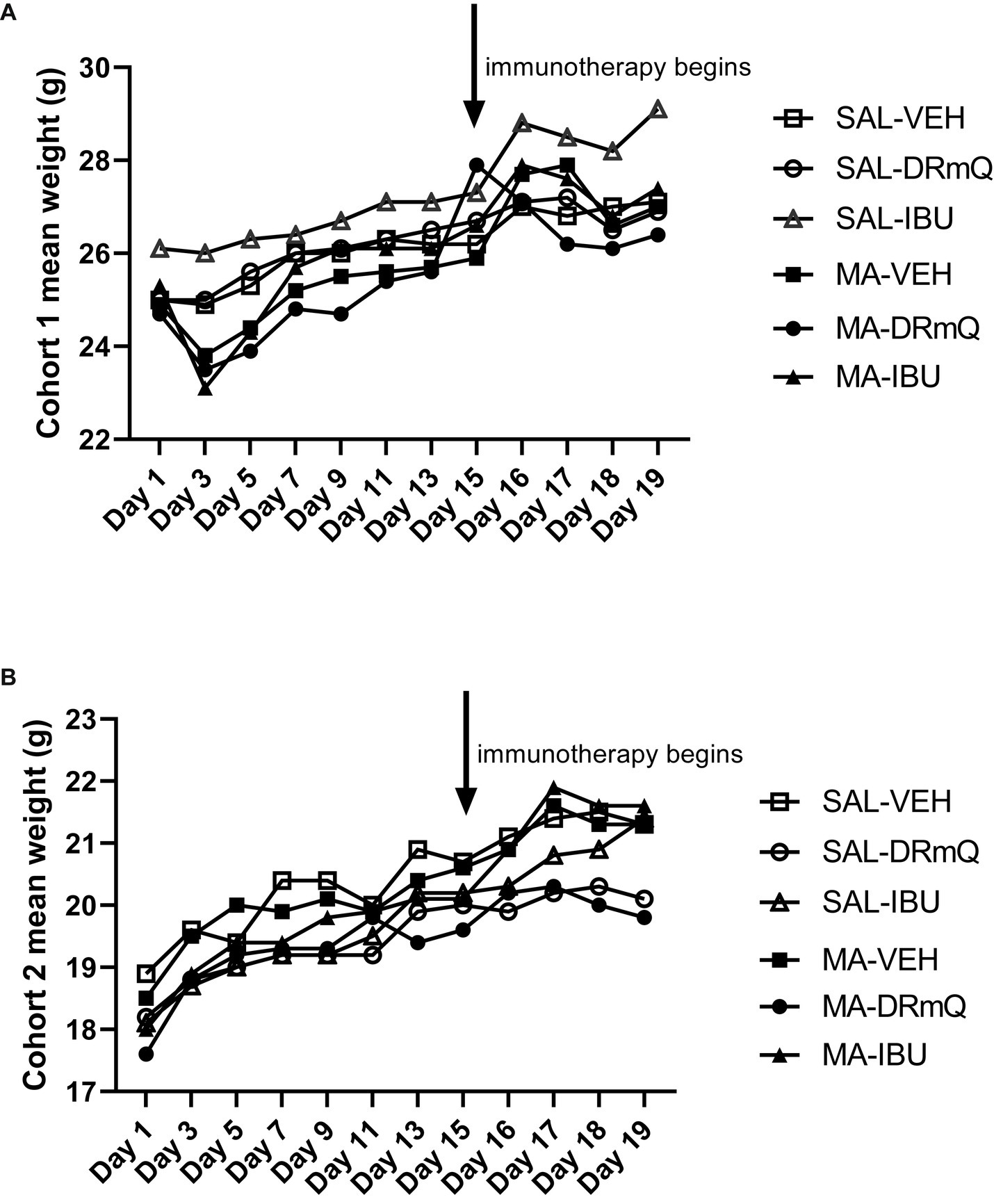
Figure 2. Body weights by treatment group over time during methamphetamine exposure and immunotherapy (A) cohort 1; (B) cohort 2.
3.2. Methamphetamine exposure, immunotherapy, and cognitive function
To evaluate the effects of methamphetamine exposure and immuotherapeutic treatments on cognitive function, mice were tested using the NORT, a behavioral test that assesses recognition memory function (44). Behavioral assessments were conducted over a three-day period (habituation, training, and retention sessions). During the retention session of the NORT, analyses indicated that across groups, mice spent similar amounts of time exploring both objects (total exploration: F = 1.40, p = 0.25) (Figure 3A). There was a significant between-group difference in the duration of time spent exploring the novel object (p = 0.05); post hoc analyses indicated non-significant trending differences between SAL-VEH and MA-VEH (p = 0.06) and MA-VEH and MA-DRmQ (p = 0.07) groups, with the MA-VEH group showing decreased exploration as compared to the SAL-VEH group and the MA-DRmQ group showing increased exploration as compared to the MA-VEH group (Figure 3B). The frequency of novel object recognition events (i.e., the number of times the mouse came within 2 cm of the novel object) was not significantly different across groups (F = 1.59, p = 0.19) (Figure 3C). There were also no statistically significant differences found for the time it took (latency) to initially explore the novel object (F = 0.45, p = 0.81) (Figure 3D). The groups did not show significant differences in preference for the novel object (F = 0.88, p = 0.51) (Figure 3E) or in abilities to discriminate the novel from the familiar object (F = 0.88, p = 0.51) (Figure 3F).
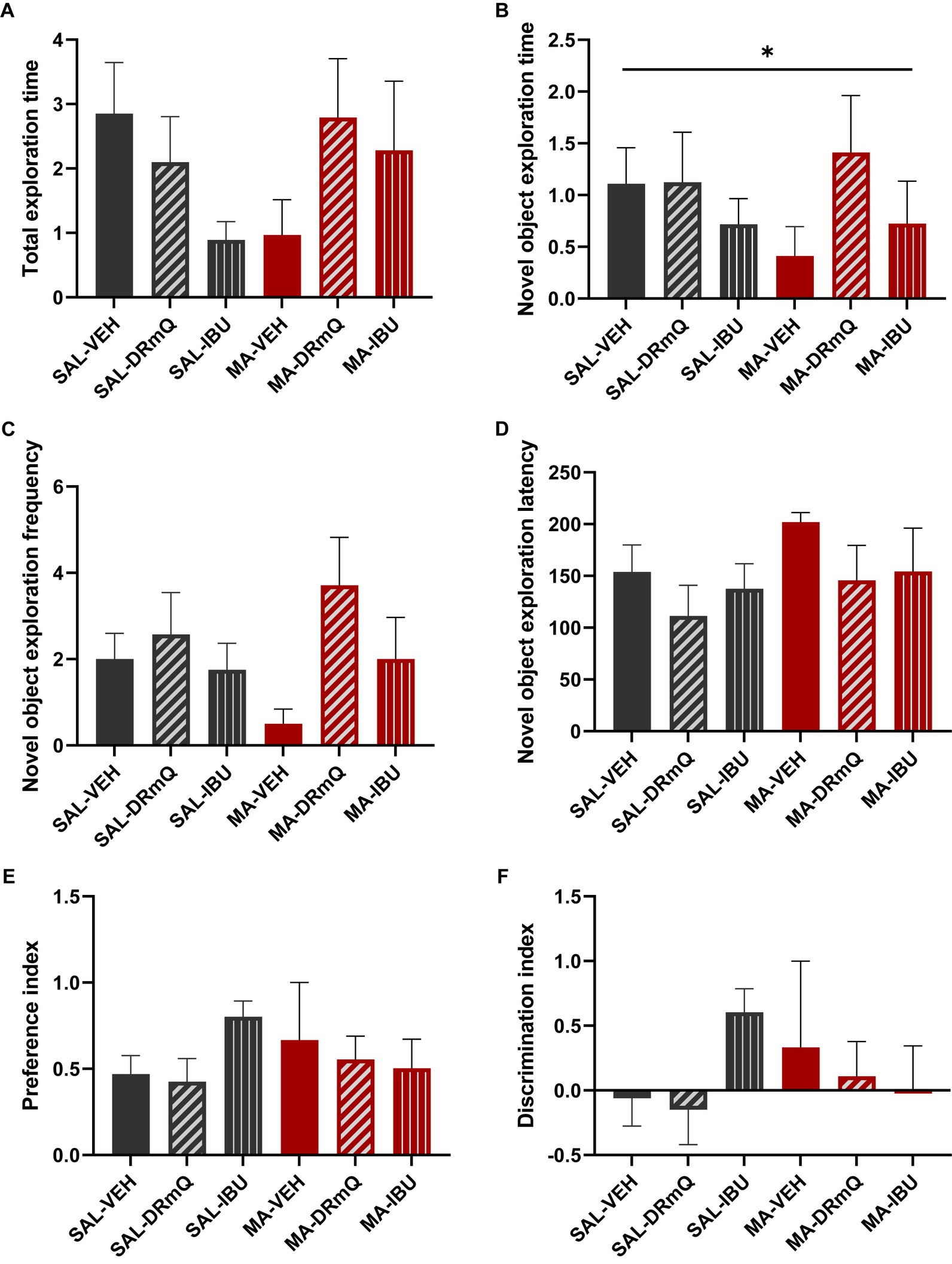
Figure 3. Cognitive impairment associated with methamphetamine exposure and immunotherapy treatment. Panels show results from the retention session of the novel object recognition test (NORT) conducted with mice in the six treatment groups. Data were analyzed using ANOVA, followed by post hoc comparisons as appropriate. (A) The total exploration time (i.e., time spent exploring both the familiar and novel objects during the retention trial) was not significantly different across the groups. (B) There was a significant between-group difference in the duration of time spent exploring the novel object (*p ≤ 0.05, one-way ANOVA); post hoc analyses indicated non-significant trending differences between SAL-VEH and MA-VEH (p = 0.06) and MA-VEH and MA-DRmQ (p = 0.07) groups. (C,D) The frequency of novel object recognition events (i.e., the number of times the mouse came within 2 cm of the novel object) and the latency to initially explore the novel object were not significantly different across groups. (E,F) The groups did not show significant differences in preference for the novel object or in abilities to discriminate the novel from the familiar object.
3.3. Methamphetamine exposure, immunotherapy, and frontal cortex inflammatory factors
A panel of pro- and anti-inflammatory factors were measured in the frontal cortices of mice exposed to methamphetamine (or saline) and treated with immunotherapy (or vehicle). The mean concentrations of IFN-γ, IL-1β, IL-2, IL-10, IP-10, MCP-1, MIP-1α, and MIP-2 are shown in Figures 4A–H. One-way ANOVA detected a significant difference across the groups for MIP-2 (F = 2.879, p = 0.03). Holm-Šidák’s multiple comparisons test indicated that the MA-VEH group had significantly higher levels of MIP-2, as compared to the SAL-VEH group (t = 2.20, p = 0.05); methamphetamine-exposed animals treated with either DRmQ (t = 2.74, p < 0.05) or IBU (t = 2.78, p < 0.05) had significantly lower levels of MIP-2, as compared to vehicle (Figure 4H). There were no statistically significant between-group differences for the other immune factors measured (Figures 4A–G).
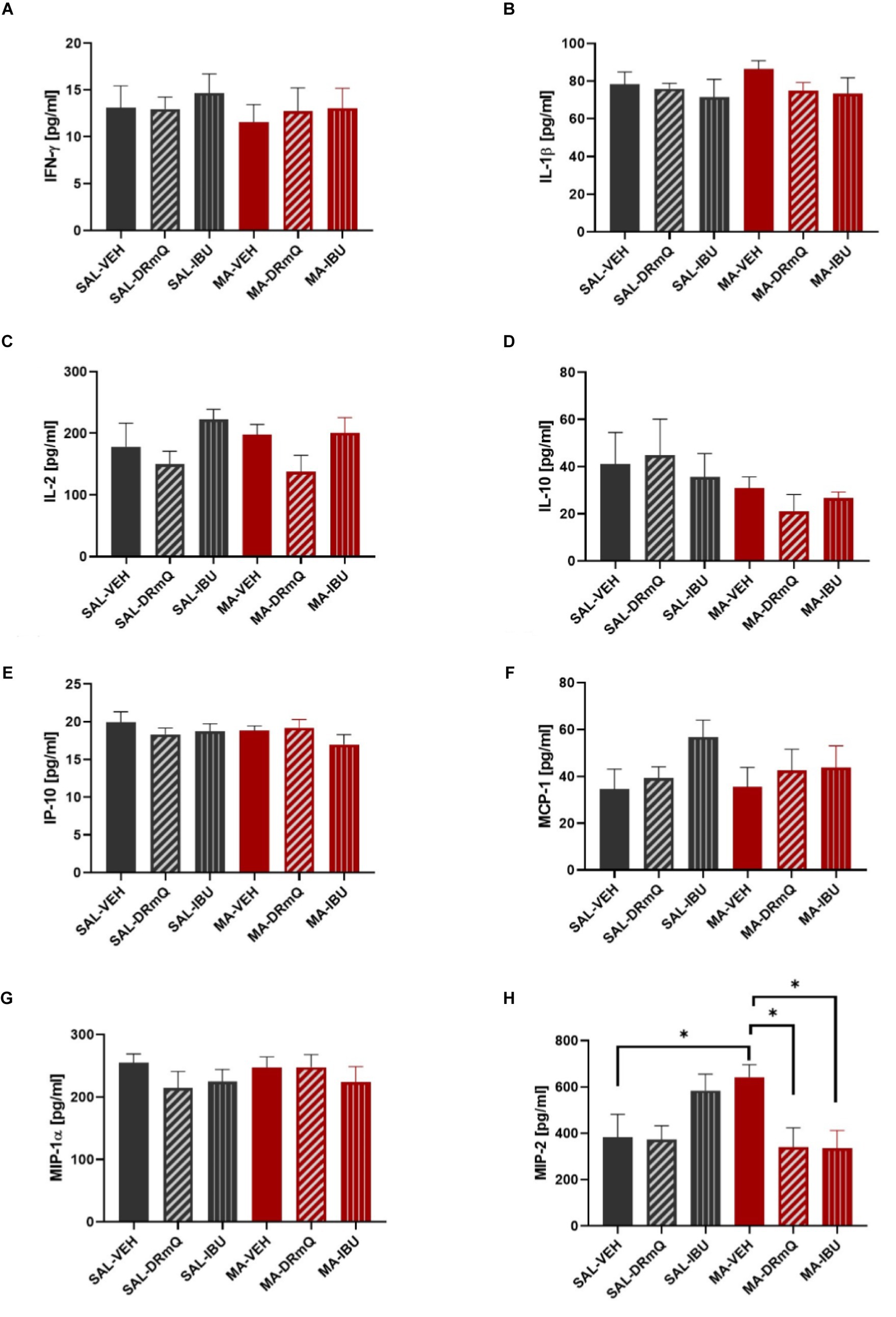
Figure 4. Cytokine levels in frontal cortices of mice treated with methamphetamine and immunotherapy. Panels (A–H) show mean (± SEM) concentrations of IFN-γ, IL-1β, IL-2, IL-10, IP-10, MCP-1, MIP-1α, and MIP-2, respectively. One-way ANOVA detected a significant difference across the groups (p = 0.03) for MIP-2; Holm-Šidák’s multiple comparisons test indicated that methamphetamine increased MIP-2 levels in the frontal cortex (MA-VEH group versus SAL-VEH group) and that methamphetamine-exposed animals treated with either DRmQ or IBU had significantly lower levels of MIP-2, as compared to methamphetamine-exposed mice treated with vehicle (*p < 0.05).
4. Discussion
A growing body of literature has shown that methamphetamine alters immune function in the peripheral and central nervous systems, increasing the expression of pro-inflammatory cytokines and chemokines that affect neuropsychiatric function. In this study, we tested two immunotherapeutic strategies for the treatment of methamphetamine-induced cognitive impairments and cortical inflammation. The frontal cortex is a particularly critical region for compulsive drug-taking behavior (54), craving, and decision-making in methamphetamine use disorder (55). Here, we show that novel object exploration time on the NORT, which measures declarative (episodic) memory [(e.g., 56)] [a cognitive domain that is relevant to individuals with methamphetamine use disorder (57–59)], was reduced in MA-VEH treated mice, as compared to SAL-VEH treated mice (p = 0.06, non-significant trend). This effect of methamphetamine on cognitive function is consistent with other stimulant exposure. In mice, the pyrrolidine analog of methamphetamine, α-pyrrolidinopropiophenone (α-PPP), decreases exploration time in the NORT and impairs spontaneous alternation performance (a measure of spatial working memory) in the Y-maze (60). Similarly, in rats, chronic administration of methamphetamine has impairing effects on recognition memory (NORT) and spontaneous alternation performance (Y-maze) (61–63). With the goal of treating methamphetamine-induced cognitive impairments, we found that in contrast to ibudilast, DRmQ immunotherapy increased novel object exploration time, as compared to MA-VEH treated mice (p = 0.07, non-significant trend), suggesting the potential for improved cognitive function in our mouse model of methamphetamine binge exposure.
The mouse-based pMHC construct DRmQ may be able to address the cognitive and neuroimmune effects of methamphetamine addiction via its anti-inflammatory effects and ability to promote remyelination. The pMHC moiety produces an antigen non-specific inhibitory effect after binding to and downregulating CD74 (the class II invariant chain) mainly on macrophages, including those that cross the BBB after CNS damage. Down regulation of CD74 expression blocks MIF signaling, promotes neuroprotection, inhibits recruitment of inflammatory cells to brain, and reduces inflammation (64–66) (Figure 5). In a recently published manuscript (67), the function of myelinated axons in two different white matter tracts (i.e., corpus callosum and optic nerves) were shown to improve in a mouse model of multiple sclerosis (EAE) following treatment with DRhQ. Myelin damage (92–95) is associated with methamphetamine exposure as research shows that there is altered expression of myelin sheath components [e.g., MOG, myelin basic protein (MBP)] following methamphetamine and cocaine exposure (96, 97) and other addictive substances are associated with the development of antibodies to MBP (98). Thus, this dual action of DRmQ is believed to contribute to its therapeutic effect and may explain why DRmQ showed a non-significant trend to improve cognitive function (p= 0.07), even though DRmQ and ibudilast both decreased MIP-2 levels (p< 0.05) (Figure 4H). The initial pMHC constructs (i.e. RTL1000) were designed to target MOG-35-55 specific T cells. The lack of the DR2 β1 domain in DRQ constructs enables the therapeutic to be administered to patients regardless of DR2 status but also likely reduces the ability of DRQ to function as a T cell receptor ligand.
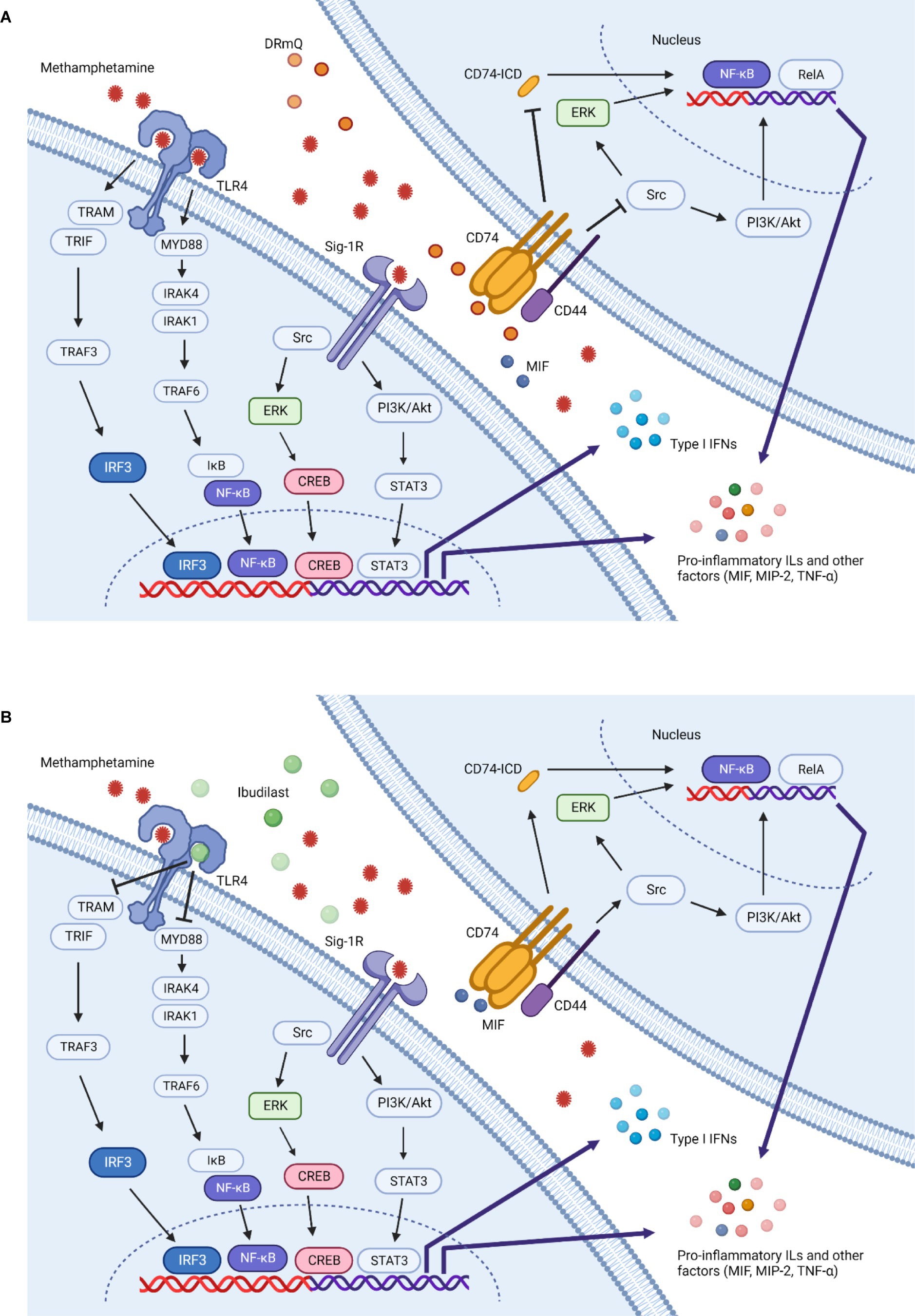
Figure 5. Methamphetamine-induced inflammation and immunotherapeutic mechanisms of action. Multiple mechanisms contribute to the inflammation induced by methamphetamine. This simplified schematic illustrates several potential inflammatory pathways and the effects of DRmQ and ibudilast on these signals. Methamphetamine binds to the sigma-1 receptor (Sig-1R) (67, 68) and Toll-like receptor 4 (TLR4) complex (69), triggering signaling pathways which ultimately upregulate the expression of inflammatory cytokines including interleukins (IL-1, IL-2, IL-6, IL-8), interferons (IFNs), MCP-1, MIF, MIP-2 [recently reviewed in (51)], and other factors relevant to methamphetamine-induced pathology, such as brain derived neurotrophic factor (BDNF) (70) and intracellular adhesion molecule-1 (ICAM-1) (71) (not shown). At the Sig-1R, methamphetamine activates downstream signaling pathways, including nuclear factor kappa light chain enhancer of activated B cells (NF-κB), mitogen-activated protein kinase (MAPK) and phosphoinositide 3-kinase (PI3K)/protein kinase B (Akt) pathways (72, 73). Rat primary astrocytes exposed to methamphetamine show increased expression of Sig-1R via the activation of Src, extracellular signal-regulated kinase 1/2 (ERK) (belongs to the MAPK family), and downstream cAMP response element binding protein (CREB) pathways (74). Activation of CREB promotes inflammation through expression of various inflammatory cytokines (75). Further, methamphetamine-induced microglial activation involves Sig-1R binding, reactive oxygen species (ROS) generation, activation of the MAPK and PI3K/Akt pathways, and pro-inflammatory protein expression (72, 76). At the TLR4 complex, methamphetamine can activate both myeloid differentiation primary response protein 88 (Myd88)-dependent and non Myd88-dependent pathways (77). In the Myd88-dependent pathway, signal transduction occurs through interleukin 1 receptor associated kinase 4 and 1 (IRAK4 and IRAK1) and TNF receptor associated factor 6 (TRAF6). The activation of TRAF6 leads to phosphorylation of inhibitors of nuclear factor κB kinases (IKKs), which in turn activates IκB. The activation of IκB leads to its degradation and the activation of NFκB, thereby mediating the production of pro-inflammatory cytokines (e.g., TNF-α, IL-1β and IL-6) (17, 78–80). In the non Myd88-dependent pathway, TLR4 triggers the activation of the TRIF-related adaptor molecule (TRAM), followed by the activation of TRAF3, TANK-binding kinase 1 (TBK1) and IKKε (not shown), which phosphorylates IFN regulatory factor 3 (IRF3). IRF3 then translocates to the nucleus and promotes the transcription of type 1 IFNs. In the later inflammatory response process, TRAM can also activate NF-κB (not shown) (72, 81, 82). (A) DRmQ is theorized to prevent methamphetamine-induced upregulation of pro-inflammatory factors by acting as a competitive inhibitor of CD74—the primary receptor for MIF. MIF binding to CD74 and recruits CD44 (cell surface adhesion receptor). Phosphorylation of CD74 and CD44 activates the Src-family tyrosine kinase Lck, in turn activating the ERK or PI3K/Akt signaling pathways and inducing the expression of pro-inflammatory cytokines through the NF-kB pathway. MIF also signals via the RelA (NF-κB-family pathway) pathway activating genes involved in inflammatory responses. The interaction of MIF and CD74 can also promote the cleavage of CD74 to produce CD74 intracellular domain (CD74-ICD), which is thought to provide a further activation signal to induce the production of several pro-inflammatory cytokines, including interleukins (IL-1, IL-2, IL-6, IL-8), TNF-α, and MCP-1 (83–85). Thus, DRmQ may disrupt the CD74 binding and subsequent pro-inflammatory signaling cascades. (B) Ibudilast can block methamphetamine-induced inflammation by acting as a competitive inhibitor of TLR4. TLR-4 blocking may lead to the reduced production of pro-inflammatory cytokines via pathways that also implicate NF-κΒ, IRAK1 and TRAF6. Additional anti-inflammatory mechanisms of ibudilast have been proposed (86) but for clarity are not shown in this figure.
Ibudilast has neuroprotective and immunomodulatory properties and has shown therapeutic benefit for substance use disorders, including methamphetamine use disorder. It is a relatively non-selective inhibitor of several phosphodiesterases (PDEs), including PDE3, PDE4, PDE10 and PDE11, as well as MIF and Toll-like receptor-4 (TLR-4) (31, 98, 99) (Figure 5). Ibudilast can reduce glial cell activation, pro-inflammatory cytokines levels (e.g., TNF-α, IL-1β and IL-6), and methamphetamine self-administration (13, 21, 31, 37). Ibudilast has also been shown to reduce methamphetamine-induced locomotor activity and stress-induced methamphetamine reinstatement (37). In clinical trials, ibudilast lessened craving for methamphetamine (29) but failed to demonstrate a significant difference between ibudilast and placebo in reducing methamphetamine use (28).
To assess inflammatory effects, we measured immune factors with evidence for a role in methamphetamine-induced CNS effects or CD74 signaling. Although some studies show increases in pro-inflammatory cytokines such as IFN-γ and IL-1β following methamphetamine exposure [reviewed in (51, 100)], we did not find significant methamphetamine-induced increases in these factors (Figures 4A,B). The timing of our sample collection and tissue type (frontal cortex) may have contributed to the different observations. However, we found that MIP-2, a factor which plays an important role in the progression and perpetuation of inflammation, was increased following methamphetamine (Figure 4H). Consistent with the anti-inflammatory properties of DRmQ and ibudilast, the concentration of MIP-2 in the frontal cortex was significantly lower in mice treated with methamphetamine and DRmQ or ibudilast, as compared to mice treated with methamphetamine and vehicle. MIP-2 is one of the CXC chemokines [also known as chemokine CXC ligand (CXCL2)] and is produced by a variety of cell types, such as glial cells, macrophages, monocytes, epithelial cells, and hepatocytes, in response to infection or injury (101, 102). Studies show associations between MIP-2 levels and organ inflammation and injury, such as in cortical damage (103), pneumonia (104), and alcohol-induced liver injury [reviewed in (101)]. MIP-2 is increased in rodent cortical cultures exposed to cocaine (105), but to date, there are no published reports on methamphetamine’s effects on MIP-2 expression. There are similarly limited data on the role of MIP-2 in cognitive function; however, one recent study found elevated concentrations of MIP-2 in peripheral blood and hippocampus in rats with cognitive dysfunction (106).
This present study contributes to research appreciating the importance of cognitive function in recovery from substance use disorders (107–110). Methamphetamine-induced cognitive deficits reflect changes in the underlying cortical, subcortical, and neuro-modulatory mechanisms that underpin cognition and can interfere with treatment outcomes (111, 112). Methamphetamine use has been linked to a broad range of cognitive deficits involving domains of complex attention, reasoning, memory, impulse control, and executive functions (57, 113). Of particular interest is the role that episodic memory and executive functions play in methamphetamine use. Findings suggest that individuals with methamphetamine dependence experience difficulty in the retrieval of future intentions, which has implications for recovery efforts and everyday functioning (114). Further, in a longitudinal study of participants with a history of methamphetamine use, those who relapsed to methamphetamine use showed worse episodic memory performance than those who remained abstinent as well as those with continued use, suggesting that relapse to methamphetamine use may affect episodic memory differently than it affects the other cognitive functions measured (59).
Our study had limitations. To assess cognitive function, we utilized only one behavioral test (i.e., NORT) and did not include other measures to assess different cognitive domains or general locomotor activity. Another limitation is that our neurochemical investigations measured immune factor levels but not neurotransmitter levels, particularly those affected by methamphetamine. It would be of interest to know, for example, how the immune factor changes observed relate to changes in monoamine neurochemistry or functional dynamics such as loss of tonic dopamine levels. Despite these limitations, the evidence reported herein contributes to a growing consensus that an immunotherapeutic approach has the potential to reduce the inflammatory and adverse neuropsychiatric effects of methamphetamine.
Data availability statement
The raw data supporting the conclusions of this article will be made available by the authors, without undue reservation.
Ethics statement
The animal study was approved by the Institutional Animal Care and Use Committee, VA Portland Health Care System, Portland, OR. The study was conducted in accordance with the local legislation and institutional requirements.
Author contributions
JL: Conceptualization, Funding acquisition, Investigation, Resources, Supervision, Writing – original draft, Writing – review & editing. SR: Visualization, Writing – original draft. EF: Investigation, Methodology, Writing – review & editing. RH: Investigation, Writing – review & editing, Formal analysis. AL-C: Investigation, Writing – review & editing, Methodology. KM: Writing – review & editing. AV: Writing – review & editing, Resources. RS: Writing – review & editing, Conceptualization, Funding acquisition.
Funding
The author(s) declare financial support was received for the research, authorship, and/or publication of this article. This work was funded by National Institutes of Health U18 DA052351 (JL), 1R21NS094881 (AV) and NS120676 (KM), and the Department of Veterans Affairs, Veterans Health Administration, Office of Research and Development, Biomedical Laboratory Research and Development Merit Review Awards I01 BX002061 (JL) and 2I01 BX000226 (AV), BLR&D Merit Review for Pre-IND studies of Drugs and Biologics Award 5I01 BX005112 (AV) and Senior Research Career Scientist Award 1IK6BX004209 (AV). This research was also supported by the Community Foundation of North Louisiana and the Department of Pharmacology, Toxicology & Neuroscience. Figures 1, 5 were created with BioRender.com.
Acknowledgments
The authors acknowledge Leela Daniel for her contributions to Figure 5. The authors also thank the Veterinary Medical Unit at the Veterans Affairs Portland Health Care System for their care and support of the animals used in this research study.
Conflict of interest
Author RS was employed by company Virogenomics BioDevelopment, Inc. The Department of Veterans Affairs (VA) and Oregon Health & Science University (OHSU) own the RTL technology used in the RTL research that is described in this report. The VA, OHSU, JL and AV have rights to royalties from the licensing agreement with Artielle Immunotherapeutics. These potential conflicts of interest have been reviewed and managed by the Conflict of Interest Committees at the VA Portland Health Care System and OHSU. Virogenomics BioDevelopment, Inc. and RS have a financial interest in Artielle Immunotherapeutics.
The remaining authors declare that the research was conducted in the absence of any commercial or financial relationships that could be construed as a potential conflict of interest.
The author(s) declared that they were an editorial board member of Frontiers, at the time of submission. This had no impact on the peer review process and the final decision.
Publisher’s note
All claims expressed in this article are solely those of the authors and do not necessarily represent those of their affiliated organizations, or those of the publisher, the editors and the reviewers. Any product that may be evaluated in this article, or claim that may be made by its manufacturer, is not guaranteed or endorsed by the publisher.
References
1. Glasner-Edwards, S, and Mooney, LJ. Methamphetamine psychosis: epidemiology and management. CNS Drugs. (2014) 28:1115–26. doi: 10.1007/s40263-014-0209-8
2. Semple, SJ, Zians, J, Strathdee, SA, and Patterson, TL. Psychosocial and behavioral correlates of depressed mood among female methamphetamine users. J Psychoactive Drugs. (2007) 39:353–66. doi: 10.1080/02791072.2007.10399897
3. Huckans, M, Fuller, BE, Chalker, ALN, Adams, M, and Loftis, JM. Plasma inflammatory factors are associated with anxiety, depression, and cognitive problems in adults with and without methamphetamine dependence: an exploratory protein array study. Front Psychiatry. (2015) 6:178. doi: 10.3389/fpsyt.2015.00178
4. Glasner-Edwards, S, Mooney, LJ, Marinelli-Casey, P, Hillhouse, M, Ang, A, and Rawson, R. Anxiety disorders among methamphetamine dependent adults: association with post-treatment functioning. Am J Addict. (2010) 19:385–90. doi: 10.1111/j.1521-0391.2010.00061.x
5. Huckans, M, Wilhelm, CJ, Phillips, TJ, Huang, ET, Hudson, R, and Loftis, JM. Parallel effects of methamphetamine on anxiety and CCL3 in humans and a genetic mouse model of high methamphetamine intake. Neuropsychobiology. (2018) 75:169–77. doi: 10.1159/000485129
6. Proebstl, L, Kamp, F, Koller, G, and Soyka, M. Cognitive deficits in methamphetamine users: how strong is the evidence? Pharmacopsychiatry. (2018) 51:243–50. doi: 10.1055/s-0043-123471
7. Huckans, M, Boyd, S, Moncrief, G, Hantke, N, Winters, B, Shirley, K, et al. Cognition during active methamphetamine use versus remission. J Clin Exp Neuropsychol. (2021) 43:599–610. doi: 10.1080/13803395.2021.1976734
8. Bernheim, A, See, RE, and Reichel, CM. Chronic methamphetamine self-administration disrupts cortical control of cognition. Neurosci Biobehav Rev. (2016) 69:36–48. doi: 10.1016/j.neubiorev.2016.07.020
9. Crews, FT, Zou, J, and Qin, L. Induction of innate immune genes in brain create the neurobiology of addiction. Brain Behav Immun. (2011) 25:S4–S12. doi: 10.1016/j.bbi.2011.03.003
10. Loftis, JM, and Janowsky, A. Neuroimmune basis of methamphetamine toxicity. Int Rev Neurobiol. (2014) 118:165–97. doi: 10.1016/B978-0-12-801284-0.00007-5
11. Davidson, M, Mayer, M, Habib, A, Rashidi, N, Filippone, RT, Fraser, S, et al. Methamphetamine induces systemic inflammation and anxiety: the role of the gut-immune-brain axis. Int J Mol Sci. (2022) 23:11224. doi: 10.3390/ijms231911224
12. Poland, RS, Hahn, Y, Knapp, PE, Beardsley, PM, and Bowers, MS. Ibudilast attenuates expression of behavioral sensitization to cocaine in male and female rats. Neuropharmacology. (2016) 109:281–92. doi: 10.1016/j.neuropharm.2016.06.024
13. Snider, SE, Hendrick, ES, and Beardsley, PM. Glial cell modulators attenuate methamphetamine self-administration in the rat. Eur J Pharmacol. (2013) 701:124–30. doi: 10.1016/j.ejphar.2013.01.016
14. Loftis, JM, Navis, T, Taylor, J, Hudson, R, Person, U, Lattal, KM, et al. Partial MHC/neuroantigen peptide constructs attenuate methamphetamine-seeking and brain chemokine (C-C motif) ligand 2 levels in rats. Eur J Pharmacol. (2020) 880:173175. doi: 10.1016/j.ejphar.2020.173175
15. Loftis, JM, Wilhelm, CJ, Vandenbark, AA, and Huckans, M. Partial MHC/neuroantigen peptide constructs: a potential neuroimmune-based treatment for methamphetamine addiction. PLoS One. (2013) 8:e56306. doi: 10.1371/journal.pone.0056306
16. Nguyen, JD, Bremer, PT, Hwang, CS, Vandewater, SA, Collins, KC, Creehan, KM, et al. Effective active vaccination against methamphetamine in female rats. Drug Alcohol Depend. (2017) 175:179–86. doi: 10.1016/j.drugalcdep.2017.03.005
17. Lwin, T, Yang, J-L, Ngampramuan, S, Viwatpinyo, K, Chancharoen, P, Veschsanit, N, et al. Melatonin ameliorates methamphetamine-induced cognitive impairments by inhibiting neuroinflammation via suppression of the TLR4/MyD88/NFκB signaling pathway in the mouse hippocampus. Prog Neuro-Psychopharmacol Biol Psychiatry. (2021) 111:110109. doi: 10.1016/j.pnpbp.2020.110109
18. Baek, JJ, Kline, H, Deveau, CM, and Yamamoto, BK. Roflumilast treatment during forced abstinence reduces relapse to methamphetamine seeking and taking. Addict Biol. (2022) 27:e13082. doi: 10.1111/adb.13082
19. Birath, JB, Briones, M, Amaya, S, Shoptaw, S, Swanson, AN, Tsuang, J, et al. Ibudilast may improve attention during early abstinence from methamphetamine. Drug Alcohol Depend. (2017) 178:386–90. doi: 10.1016/j.drugalcdep.2017.05.016
20. Azadbakht, A, Salehi, M, Maracy, MR, and Banafshe, HR. The effects of oxytocin on craving, mental health parameters, and stress hormones in methamphetamine-dependent patients undergoing matrix treatment model: a randomized, double-blind clinical trial. Eur Addict Res. (2022) 28:340–9. doi: 10.1159/000525443
21. Li, MJ, Briones, MS, Heinzerling, KG, Kalmin, MM, and Shoptaw, SJ. Ibudilast attenuates peripheral inflammatory effects of methamphetamine in patients with methamphetamine use disorder. Drug Alcohol Depend. (2020) 206:107776. doi: 10.1016/j.drugalcdep.2019.107776
22. Lee, JY, Castelli, V, Bonsack, B, Coats, AB, Navarro-Torres, L, Garcia-Sanchez, J, et al. A novel partial MHC class II construct, DRmQ, inhibits central and peripheral inflammatory responses to promote neuroprotection in experimental stroke. Transl Stroke Res. (2019) 11:831–6. doi: 10.1007/s12975-019-00756-1
23. Meza-Romero, R, Benedek, G, Gerstner, G, Kent, G, Nguyen, H, Offner, H, et al. Increased CD74 binding and EAE treatment efficacy of a modified DRα1 molecular construct. Metab Brain Dis. (2019) 34:153–64. doi: 10.1007/s11011-018-0331-2
24. Petralia, MC, Mazzon, E, Mangano, K, Fagone, P, Di Marco, R, Falzone, L, et al. Transcriptomic analysis reveals moderate modulation of macrophage migration inhibitory factor superfamily genes in alcohol use disorders. Exp Ther Med. (2020) 19:1755–62. doi: 10.3892/etm.2020.8410
25. Petralia, MC, Mazzon, E, Fagone, P, Basile, MS, Lenzo, V, Quattropani, MC, et al. Pathogenic contribution of the macrophage migration inhibitory factor family to major depressive disorder and emerging tailored therapeutic approaches. J Affect Disord. (2020) 263:15–24. doi: 10.1016/j.jad.2019.11.127
26. Basile, MS, Battaglia, G, Bruno, V, Mangano, K, Fagone, P, Petralia, MC, et al. The dichotomic role of macrophage migration inhibitory factor in neurodegeneration. Int J Mol Sci. (2020) 21:3023. doi: 10.3390/ijms21083023
27. Cho, Y, Crichlow, GV, Vermeire, JJ, Leng, L, Du, X, Hodsdon, ME, et al. Allosteric inhibition of macrophage migration inhibitory factor revealed by ibudilast. Proc Natl Acad Sci U S A. (2010) 107:11313–8. doi: 10.1073/pnas.1002716107
28. Heinzerling, KG, Briones, M, Thames, AD, Hinkin, CH, Zhu, T, Wu, YN, et al. Randomized, placebo-controlled trial of targeting neuroinflammation with ibudilast to treat methamphetamine use disorder. J Neuroimmune Pharmacol. (2019) 15:238–48. doi: 10.1007/s11481-019-09883-w
29. Worley, MJ, Heinzerling, KG, Roche, DJ, and Shoptaw, S. Ibudilast attenuates subjective effects of methamphetamine in a placebo-controlled inpatient study. Drug Alcohol Depend. (2016) 162:245–50. doi: 10.1016/j.drugalcdep.2016.02.036
30. Mizuno, T, Kurotani, T, Komatsu, Y, Kawanokuchi, J, Kato, H, Mitsuma, N, et al. Neuroprotective role of phosphodiesterase inhibitor ibudilast on neuronal cell death induced by activated microglia. Neuropharmacology. (2004) 46:404–11. doi: 10.1016/j.neuropharm.2003.09.009
31. Suzumura, A, Ito, A, Yoshikawa, M, and Sawada, M. Ibudilast suppresses TNFα production by glial cells functioning mainly as type III phosphodiesterase inhibitor in the CNS. Brain Res. (1999) 837:203–12. doi: 10.1016/S0006-8993(99)01666-2
32. Sekine, Y, Ouchi, Y, Sugihara, G, Takei, N, Yoshikawa, E, Nakamura, K, et al. Methamphetamine causes microglial activation in the brains of human abusers. J Neurosci. (2008) 28:5756–61. doi: 10.1523/JNEUROSCI.1179-08.2008
33. Gong, M, Shen, Y, Liang, W, Zhang, Z, He, C, Lou, M, et al. Impairments in the default mode and executive networks in methamphetamine users during short-term abstinence. Int J Gen Med. (2022) 15:6073–84. doi: 10.2147/IJGM.S369571
34. Zhang, Y, Ku, Y, Sun, J, Daskalakis, ZJ, and Yuan, T-F. Intermittent theta burst stimulation to the left dorsolateral prefrontal cortex improves working memory of subjects with methamphetamine use disorder. Psychol Med. (2023) 53:2427–36. doi: 10.1017/S003329172100430X
35. Armenta-Resendiz, M, Assali, A, Tsvetkov, E, Cowan, CW, and Lavin, A. Repeated methamphetamine administration produces cognitive deficits through augmentation of GABAergic synaptic transmission in the prefrontal cortex. Neuropsychopharmacology. (2022) 47:1816–25. doi: 10.1038/s41386-022-01371-9
36. Huang, S, Dai, Y, Zhang, C, Yang, C, Huang, Q, Hao, W, et al. Higher impulsivity and lower grey matter volume in the bilateral prefrontal cortex in long-term abstinent individuals with severe methamphetamine use disorder. Drug Alcohol Depend. (2020) 212:108040. doi: 10.1016/j.drugalcdep.2020.108040
37. Beardsley, PM, Shelton, KL, Hendrick, E, and Johnson, KW. The glial cell modulator and phosphodiesterase inhibitor, AV411 (ibudilast), attenuates prime- and stress-induced methamphetamine relapse. Eur J Pharmacol. (2010) 637:102–8. doi: 10.1016/j.ejphar.2010.04.010
38. Loftis, JM, Choi, D, Hoffman, W, and Huckans, MS. Methamphetamine causes persistent immune dysregulation: a cross-species, translational report. Neurotox Res. (2011) 20:59–68. doi: 10.1007/s12640-010-9223-x
39. Kilkenny, C, Browne, WJ, Cuthill, IC, Emerson, M, and Altman, DG. Improving bioscience research reporting: the ARRIVE guidelines for reporting animal research. PLoS Biol. (2010) 8:e1000412. doi: 10.1371/journal.pbio.1000412
40. Panenka, WJ, Procyshyn, RM, Lecomte, T, MacEwan, GW, Flynn, SW, Honer, WG, et al. Methamphetamine use: a comprehensive review of molecular, preclinical and clinical findings. Drug Alcohol Depend. (2013) 129:167–79. doi: 10.1016/j.drugalcdep.2012.11.016
41. Chung, YA, Peterson, BS, Yoon, SJ, Cho, SN, Chai, S, Jeong, J, et al. In vivo evidence for long-term CNS toxicity, associated with chronic binge use of methamphetamine. Drug Alcohol Depend. (2010) 111:155–60. doi: 10.1016/j.drugalcdep.2010.04.005
42. Gibson, AS, West, PJ, and Keefe, KA. Effects of methamphetamine-induced neurotoxicity on striatal long-term potentiation. Psychopharmacology. (2022) 239:93–104. doi: 10.1007/s00213-021-06055-8
43. Vandenbark, AA, Meza-Romero, R, Benedek, G, and Offner, H. A novel neurotherapeutic for multiple sclerosis, ischemic injury, methamphetamine addiction, and traumatic brain injury. J Neuroinflammation. (2019) 16:14. doi: 10.1186/s12974-018-1393-0
44. Leger, M, Quiedeville, A, Bouet, V, Haelewyn, B, Boulouard, M, Schumann-Bard, P, et al. Object recognition test in mice. Nat Protoc. (2013) 8:2531–7. doi: 10.1038/nprot.2013.155
45. Loftis, JM, Taylor, J, Hudson, R, and Firsick, EJ. Neuroinvasion and cognitive impairment in comorbid alcohol dependence and chronic viral infection: an initial investigation. J Neuroimmunol. (2019) 335:577006. doi: 10.1016/j.jneuroim.2019.577006
46. Ennaceur, A, and Delacour, J. A new one-trial test for neurobiological studies of memory in rats. 1: behavioral data. Behav Brain Res. (1988) 31:47–59. doi: 10.1016/0166-4328(88)90157-X
47. Bianchi, M, Fone, KF, Azmi, N, Heidbreder, CA, Hagan, JJ, and Marsden, CA. Isolation rearing induces recognition memory deficits accompanied by cytoskeletal alterations in rat hippocampus. Eur J Neurosci. (2006) 24:2894–902. doi: 10.1111/j.1460-9568.2006.05170.x
48. Hozumi, H, Asanuma, M, Miyazaki, I, Fukuoka, S, Kikkawa, Y, Kimoto, N, et al. Protective effects of interferon-gamma against methamphetamine-induced neurotoxicity. Toxicol Lett. (2008) 177:123–9. doi: 10.1016/j.toxlet.2008.01.005
49. Wu, H, Zhang, Z, Ma, Y, Chen, F, Xiong, P, Xie, Z, et al. Dynamic immune and exosome transcriptomic responses in patients undergoing psychostimulant methamphetamine withdrawal. Front Cell Neurosci. (2022) 16:961131. doi: 10.3389/fncel.2022.961131
50. Yang, X, Zhao, H, Liu, X, Xie, Q, Zhou, X, Deng, Q, et al. The relationship between serum cytokine levels and the degree of psychosis and cognitive impairment in patients with methamphetamine-associated psychosis in Chinese patients. Front Psychiatry. (2020) 11:594766. doi: 10.3389/fpsyt.2020.594766
51. Bravo, J, Magalhães, C, Andrade, EB, Magalhães, A, and Summavielle, T. The impact of psychostimulants on central and peripheral neuro-immune regulation: a scoping review of cytokine profiles and their implications for addiction. Front Cell Neurosci. (2023) 17:1109611. doi: 10.3389/fncel.2023.1109611
52. Takahashi, K, Koga, K, Linge, HM, Zhang, Y, Lin, X, Metz, CN, et al. Macrophage CD74 contributes to MIF-induced pulmonary inflammation. Respir Res. (2009) 10:33. doi: 10.1186/1465-9921-10-33
53. Faraji, J, Ambeskovic, M, Sauter, N, Toly, J, Whitten, K, Lopes, NA, et al. Sex-specific stress and biobehavioral responses to human experimenters in rats. Front Neurosci. (2022) 16:965500. doi: 10.3389/fnins.2022.965500
54. Duan, Y, Tsai, P-J, Salmeron, BJ, Hu, Y, Gu, H, Lu, H, et al. Compulsive drug-taking is associated with habenula-frontal cortex connectivity. Proc Natl Acad Sci U S A. (2022) 119:e2208867119. doi: 10.1073/pnas.2208867119
55. Wang, W, Zhu, Y, Wang, L, Mu, LL, Zhu, L, Ding, D, et al. High-frequency repetitive transcranial magnetic stimulation of the left dorsolateral prefrontal cortex reduces drug craving and improves decision-making ability in methamphetamine use disorder. Psychiatry Res. (2022) 317:114904. doi: 10.1016/j.psychres.2022.114904
56. Peters, J, Scofield, MD, and Reichel, CM. Chemogenetic activation of the perirhinal cortex reverses methamphetamine-induced memory deficits and reduces relapse. Learn Mem. (2018) 25:410–5. doi: 10.1101/lm.046797.117
57. Scott, JC, Woods, SP, Matt, GE, Meyer, RA, Heaton, RK, Atkinson, JH, et al. Neurocognitive effects of methamphetamine: a critical review and meta-analysis. Neuropsychol Rev. (2007) 17:275–97. doi: 10.1007/s11065-007-9031-0
58. Dean, AC, Groman, SM, Morales, AM, and London, ED. An evaluation of the evidence that methamphetamine abuse causes cognitive decline in humans. Neuropsychopharmacology. (2013) 38:259–74. doi: 10.1038/npp.2012.179
59. Simon, SL, Dacey, J, Glynn, S, Rawson, R, and Ling, W. The effect of relapse on cognition in abstinent methamphetamine abusers. J Subst Abus Treat. (2004) 27:59–66. doi: 10.1016/j.jsat.2004.03.011
60. Ray, A, Chitre, NM, Daphney, CM, Blough, BE, Canal, CE, and Murnane, KS. Effects of the second-generation “bath salt” cathinone alpha-pyrrolidinopropiophenone (α-PPP) on behavior and monoamine neurochemistry in male mice. Psychopharmacology. (2019) 236:1107–17. doi: 10.1007/s00213-018-5044-z
61. Razavi, Y, Shabani, R, Mehdizadeh, M, and Haghparast, A. Neuroprotective effect of chronic administration of cannabidiol during the abstinence period on methamphetamine-induced impairment of recognition memory in the rats. Behav Pharmacol. (2020) 31:385–96. doi: 10.1097/FBP.0000000000000544
62. Vieira-Brock, PL, McFadden, LM, Nielsen, SM, Smith, MD, Hanson, GR, and Fleckenstein, AE. Nicotine administration attenuates methamphetamine-induced novel object recognition deficits. Int J Neuropsychopharmacol. (2015) 18:pyv073. doi: 10.1093/ijnp/pyv073
63. Schröder, N, O’Dell, SJ, and Marshall, JF. Neurotoxic methamphetamine regimen severely impairs recognition memory in rats. Synapse. (2003) 49:89–96. doi: 10.1002/syn.10210
64. Wang, GBG, Gold, BG, Kaler, LJ, Yu, X, Afentoulis, ME, Burrows, GG, et al. Antigen-specific therapy promotes repair of myelin and axonal damage in established EAE. J Neurochem. (2006) 98:1817–27. doi: 10.1111/j.1471-4159.2006.04081.x
65. Sinha, S, Subramanian, S, Proctor, TM, Kaler, LJ, Grafe, M, Dahan, R, et al. A promising therapeutic approach for multiple sclerosis: recombinant T-cell receptor ligands modulate experimental autoimmune encephalomyelitis by reducing interleukin-17 production and inhibiting migration of encephalitogenic cells into the CNS. J Neurosci. (2007) 27:12531–9. doi: 10.1523/JNEUROSCI.3599-07.2007
66. Sharkey, J, Glen, KA, Wolfe, S, and Kuhar, MJ. Cocaine binding at sigma receptors. Eur J Pharmacol. (1988) 149:171–4. doi: 10.1016/0014-2999(88)90058-1
67. Zerimech, S, Nguyen, H, Vandenbark, AA, Offner, H, and Baltan, S. Novel therapeutic for multiple sclerosis protects white matter function in EAE mouse model. Front. Mol. Med. (2023) 3. doi: 10.3389/fmmed.2023.1237078
68. Kaushal, N, and Matsumoto, RR. Role of sigma receptors in methamphetamine-induced neurotoxicity. Curr Neuropharmacol. (2011) 9:54–7. doi: 10.2174/157015911795016930
69. Wang, X, Northcutt, AL, Cochran, TA, Zhang, X, Fabisiak, TJ, Haas, ME, et al. Methamphetamine activates toll-like receptor 4 to induce central immune signaling within the ventral tegmental area and contributes to extracellular dopamine increase in the nucleus accumbens shell. ACS Chem Neurosci. (2019) 10:3622–34. doi: 10.1021/acschemneuro.9b00225
70. Moaveni, A, Fayaz Feyzi, Y, Tayebeh Rahideh, S, and Arezoomandan, R. The relationship between serum brain-derived neurotrophic level and neurocognitive functions in chronic methamphetamine users. Neurosci Lett. (2022) 772:136478. doi: 10.1016/j.neulet.2022.136478
71. Leitão, RA, Fontes-Ribeiro, CA, and Silva, AP. The effect of parthenolide on methamphetamine-induced blood-brain barrier and astrocyte alterations. Eur J Clin Investig. (2022) 52:e13694. doi: 10.1111/eci.13694
72. Kim, B, Yun, J, and Park, B. Methamphetamine-induced neuronal damage: neurotoxicity and neuroinflammation. Biomol Ther. (2020) 28:381–8. doi: 10.4062/biomolther.2020.044
73. Jia, J, Cheng, J, Wang, C, and Zhen, X. Sigma-1 receptor-modulated neuroinflammation in neurological diseases. Front Cell Neurosci. (2018) 12:314. doi: 10.3389/fncel.2018.00314
74. Zhang, Y, Lv, X, Bai, Y, Zhu, X, Wu, X, Chao, J, et al. Involvement of sigma-1 receptor in astrocyte activation induced by methamphetamine via up-regulation of its own expression. J Neuroinflammation. (2015) 12:29. doi: 10.1186/s12974-015-0250-7
75. Krasnova, IN, Justinova, Z, and Cadet, JL. Methamphetamine addiction: involvement of CREB and neuroinflammatory signaling pathways. Psychopharmacology. (2016) 233:1945–62. doi: 10.1007/s00213-016-4235-8
76. Chao, J, Zhang, Y, du, L, Zhou, R, Wu, X, Shen, K, et al. Molecular mechanisms underlying the involvement of the sigma-1 receptor in methamphetamine-mediated microglial polarization. Sci Rep. (2017) 7:11540. doi: 10.1038/s41598-017-11065-8
77. Billod, J-M, Lacetera, A, Guzmán-Caldentey, J, and Martín-Santamaría, S. Computational approaches to toll-like receptor 4 modulation. Molecules. (2016) 21:994. doi: 10.3390/molecules21080994
78. Ghosh, S, Khatua, S, Dasgupta, A, and Acharya, K. Crude polysaccharide from the milky mushroom, Calocybe indica, modulates innate immunity of macrophage cells by triggering MyD88-dependent TLR4/NF-κB pathway. J Pharm Pharmacol. (2021) 73:70–81. doi: 10.1093/jpp/rgaa020
79. Kawai, T, and Akira, S. The role of pattern-recognition receptors in innate immunity: update on toll-like receptors. Nat Immunol. (2010) 11:373–84. doi: 10.1038/ni.1863
80. du, SH, Qiao, D-F, Chen, C-X, Chen, S, Liu, C, Lin, Z, et al. Toll-like receptor 4 mediates methamphetamine-induced neuroinflammation through caspase-11 signaling pathway in astrocytes. Front Mol Neurosci. (2017) 10:409. doi: 10.3389/fnmol.2017.00409
81. Wu, R, and Li, J-X. Toll-like receptor 4 signaling and drug addiction. Front Pharmacol. (2020) 11:603445. doi: 10.3389/fphar.2020.603445
82. Wang, J, Hu, Y, Deng, WW, and Sun, B. Negative regulation of toll-like receptor signaling pathway. Biomol Ther. (2009) 11:321–7. doi: 10.1016/j.micinf.2008.12.011
83. Kang, I, and Bucala, R. The immunobiology of MIF: function, genetics and prospects for precision medicine. Int J Oncol. (2021) 15:427–37. doi: 10.1038/s41584-019-0238-2
84. Leng, L, Metz, CN, Fang, Y, Xu, J, Donnelly, S, Baugh, J, et al. MIF signal transduction initiated by binding to CD74. J Exp Med. (2003) 197:1467–76. doi: 10.1084/jem.20030286
85. Martin-Ventura, JL, Madrigal-Matute, J, Munoz-Garcia, B, Blanco-Colio, LM, van Oostrom, M, Zalba, G, et al. Increased CD74 expression in human atherosclerotic plaques: contribution to inflammatory responses in vascular cells. Cardiovasc Res. (2009) 83:586–94. doi: 10.1093/cvr/cvp141
86. Angelopoulou, E, Pyrgelis, E-S, and Piperi, C. Emerging potential of the phosphodiesterase (PDE) inhibitor ibudilast for neurodegenerative diseases: an update on preclinical and clinical evidence. Molecules. (2022) 27:8448. doi: 10.3390/molecules27238448
87. Prasad, A, Kulkarni, R, Shrivastava, A, Jiang, S, Lawson, K, and Groopman, JE. Methamphetamine functions as a novel CD4+ T-cell activator via the sigma-1 receptor to enhance HIV-1 infection. Sci Rep. (2019) 9:958. doi: 10.1038/s41598-018-35757-x
88. Potula, R, Hawkins, BJ, Cenna, JM, Fan, S, Dykstra, H, Ramirez, SH, et al. Methamphetamine causes mitrochondrial oxidative damage in human T lymphocytes leading to functional impairment. J Immunol. (2010) 185:2867–76. doi: 10.4049/jimmunol.0903691
89. Mata, MM, Napier, TC, Graves, SM, Mahmood, F, Raeisi, S, and Baum, LL. Methamphetamine decreases CD4 T cell frequency and alters pro-inflammatory cytokine production in a model of drug abuse. Eur J Pharmacol. (2015) 752:26–33. doi: 10.1016/j.ejphar.2015.02.002
90. Harms, R, Morsey, B, Boyer, CW, Fox, HS, and Sarvetnick, N. Methamphetamine administration targets multiple immune subsets and induces phenotypic alterations suggestive of immunosuppression. PLoS One. (2012) 7:e49897. doi: 10.1371/journal.pone.0049897
91. Ding, J, Huang, J, Xia, B, Hu, S, Fan, H, Dai, J, et al. Transfer of α-synuclein from neurons to oligodendrocytes triggers myelin sheath destruction in methamphetamine administration mice. Toxicol Lett. (2021) 352:34–45. doi: 10.1016/j.toxlet.2021.09.005
92. Salo, R, Nordahl, TE, Buonocore, MH, Natsuaki, Y, Waters, C, Moore, CD, et al. Cognitive control and white matter callosal microstructure in methamphetamine-dependent subjects: a diffusion tensor imaging study. Biol Psychiatry. (2009) 65:122–8. doi: 10.1016/j.biopsych.2008.08.004
93. Chung, A, Lyoo, IK, Kim, SJ, Hwang, J, Bae, SC, Sung, YH, et al. Decreased frontal white-matter integrity in abstinent methamphetamine abusers. Int J Neuropsychopharmacol. (2007) 10:765–75. doi: 10.1017/S1461145706007395
94. Cloak, CC, Ernst, T, Fujii, L, Hedemark, B, and Chang, L. Lower diffusion in white matter of children with prenatal methamphetamine exposure. Neurology. (2009) 72:2068–75. doi: 10.1212/01.wnl.0000346516.49126.20
95. Yang, L, Guo, Y, Huang, M, Wu, X, Li, X, Chen, G, et al. Thioredoxin-1 protects spinal cord from demyelination induced by methamphetamine through suppressing endoplasmic reticulum stress and inflammation. Front Neurol. (2018) 9:49. doi: 10.3389/fneur.2018.00049
96. Albertson, DN, Pruetz, B, Schmidt, CJ, Kuhn, DM, Kapatos, G, and Bannon, MJ. Gene expression profile of the nucleus accumbens of human cocaine abusers: evidence for dysregulation of myelin. J Neurochem. (2004) 88:1211–9. doi: 10.1046/j.1471-4159.2003.02247.x
97. Jankovic, BD, Horvat, J, Djordjijevic, D, Ramah, A, Fridman, V, and Spahic, O. Brain-associated autoimmune features in heroin addicts: correlation to HIV infection and dementia. Int J Neurosci. (1991) 58:113–26. doi: 10.3109/00207459108987188
98. Gibson, LCD, Hastings, SF, McPhee, I, Clayton, RA, Darroch, CE, Mackenzie, A, et al. The inhibitory profile of ibudilast against the human phosphodiesterase enzyme family. Eur J Pharmacol. (2006) 538:39–42. doi: 10.1016/j.ejphar.2006.02.053
99. Wu, N-C, and Wang, J-J. Ibudilast, a phosphodiesterase inhibitor and toll-like receptor-4 antagonist, improves hemorrhagic shock and reperfusion-induced left ventricular dysfunction by reducing myocardial tumor necrosis factor α. Transplant Proc. (2020) 52:1869–74. doi: 10.1016/j.transproceed.2020.02.145
100. Kita, T, Miyazaki, I, Asanuma, M, Takeshima, M, and Wagner, GC. Dopamine-induced behavioral changes and oxidative stress in methamphetamine-induced neurotoxicity. Int Rev Neurobiol. (2009) 88:43–64. doi: 10.1016/S0074-7742(09)88003-3
101. Qin, C-C, Liu, Y-N, Hu, Y, Yang, Y, and Chen, Z. Macrophage inflammatory protein-2 as mediator of inflammation in acute liver injury. World J Gastroenterol. (2017) 23:3043–52. doi: 10.3748/wjg.v23.i17.3043
102. Nygårdas, PT, Määttä, JA, and Hinkkanen, AE. Chemokine expression by central nervous system resident cells and infiltrating neutrophils during experimental autoimmune encephalomyelitis in the BALB/c mouse. Eur J Immunol. (2000) 30:1911–8. doi: 10.1002/1521-4141(200007)30:7<1911::AID-IMMU1911>3.0.CO;2-E
103. Hausmann, EH, Berman, NE, Wang, YY, Meara, JB, Wood, GW, and Klein, RM. Selective chemokine mRNA expression following brain injury. Brain Res. (1998) 788:49–59. doi: 10.1016/s0006-8993(97)01160-8
104. Osakabe, N, Takano, H, Sanbongi, C, Yasuda, A, Yanagisawa, R, Inoue, KI, et al. Anti-inflammatory and anti-allergic effect of rosmarinic acid (RA); inhibition of seasonal allergic rhinoconjunctivitis (SAR) and its mechanism. Biofactors. (2004) 21:127–31. doi: 10.1002/biof.552210125
105. Aksenova, M, Sybrandt, J, Cui, B, Sikirzhytski, V, Ji, H, Odhiambo, D, et al. Inhibition of the dead box RNA helicase 3 prevents HIV-1 tat and cocaine-induced neurotoxicity by targeting microglia activation. J Neuroimmune Pharmacol. (2020) 15:209–23. doi: 10.1007/s11481-019-09885-8
106. Wang, Y, Zhi, H, and Zhang, X. Effect of Huangdisan grain on improving cognitive impairment in VD rats and its mechanism in immune inflammatory response. J Neuroimmunol. (2023) 377:578058. doi: 10.1016/j.jneuroim.2023.578058
107. Behle, N, Kamp, F, Proebstl, L, Hager, L, Riebschläger, M, Schacht-Jablonowsky, M, et al. Treatment outcome, cognitive function, and psychopathology in methamphetamine users compared to other substance users. World J Psychiatry. (2022) 12:944–57. doi: 10.5498/wjp.v12.i7.944
108. Ramey, T, and Regier, PS. Cognitive impairment in substance use disorders. CNS Spectr. (2019) 24:102–13. doi: 10.1017/S1092852918001426
109. Loftis, JM, and Huckans, M. Cognitive enhancement in combination with “brain repair” may be optimal for the treatment of stimulant addiction. Addiction. (2011) 106:1021–2. doi: 10.1111/j.1360-0443.2010.03354.x
110. St Peters, MM, Park, CHJ, Turner, A, Guerin, AA, and Kim, JH. Past and current drug repurposing clinical trials to treat cognition in methamphetamine use: a scoping review of pharmacotherapy candidates. Addict Neurosci. (2023) 5:100064. doi: 10.1016/j.addicn.2023.100064
111. Ersche, KD, Clark, L, London, M, Robbins, TW, and Sahakian, BJ. Profile of executive and memory function associated with amphetamine and opiate dependence. Neuropsychopharmacology. (2006) 31:1036–47. doi: 10.1038/sj.npp.1300889
112. Rogers, RD, and Robbins, TW. Investigating the neurocognitive deficits associated with chronic drug misuse. Curr Opin Neurobiol. (2001) 11:250–7. doi: 10.1016/s0959-4388(00)00204-x
113. Ellis, C, Hoffman, W, Jaehnert, S, Plagge, J, Loftis, JM, Schwartz, D, et al. Everyday problems with executive dysfunction and impulsivity in adults recovering from methamphetamine addiction. Addict Disord Their Treat. (2016) 15:1–5. doi: 10.1097/ADT.0000000000000059
Keywords: addiction, chemokine, drug discovery, frontal cortex, inflammation, methamphetamine, MIP-2, psychostimulant
Citation: Loftis JM, Ramani S, Firsick EJ, Hudson R, Le-Cook A, Murnane KS, Vandenbark A and Shirley RL (2023) Immunotherapeutic treatment of inflammation in mice exposed to methamphetamine. Front. Psychiatry. 14:1259041. doi: 10.3389/fpsyt.2023.1259041
Edited by:
Wendy J. Lynch, University of Virginia, United StatesReviewed by:
Maria Cecilia G. Marcondes, San Diego Biomedical Research Institute, United StatesOksana Polesskaya, University of California, San Diego, United States
Copyright © 2023 Loftis, Ramani, Firsick, Hudson, Le-Cook, Murnane, Vandenbark and Shirley. This is an open-access article distributed under the terms of the Creative Commons Attribution License (CC BY). The use, distribution or reproduction in other forums is permitted, provided the original author(s) and the copyright owner(s) are credited and that the original publication in this journal is cited, in accordance with accepted academic practice. No use, distribution or reproduction is permitted which does not comply with these terms.
*Correspondence: Jennifer M. Loftis, bG9mdGlzakBvaHN1LmVkdQ==; amVubmlmZXIubG9mdGlzMkB2YS5nb3Y=
†These authors have contributed equally to this work