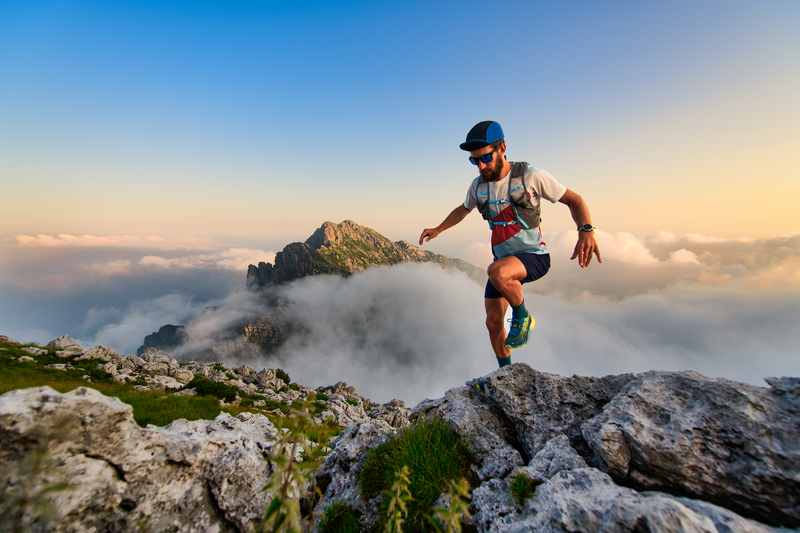
95% of researchers rate our articles as excellent or good
Learn more about the work of our research integrity team to safeguard the quality of each article we publish.
Find out more
REVIEW article
Front. Psychiatry , 26 June 2023
Sec. Psychopharmacology
Volume 14 - 2023 | https://doi.org/10.3389/fpsyt.2023.1197890
This article is part of the Research Topic Psychedelics as Treatments for Substance Use Disorders: Exploring therapeutic potential, risks, underlying mechanisms of action, and implementation challenges View all 8 articles
Background: Ketamine and psychedelics have abuse liability. They can also induce “transformative experiences” where individuals experience enhanced states of awareness. This enhanced awareness can lead to changes in preexisting behavioral patterns which could be beneficial in the treatment of substance use disorders (SUDs). Preclinical and clinical studies suggest that ketamine and psychedelics may alter markers associated with synaptic density, and that these changes may underlie effects such as sensitization, conditioned place preference, drug self-administration, and verbal memory performance. In this scoping review, we examined studies that measured synaptic markers in animals and humans after exposure to ketamine and/or psychedelics.
Methods: A systematic search was conducted following PRISMA guidelines, through PubMed, EBSCO, Scopus, and Web of Science, based on a published protocol (Open Science Framework, DOI: 10.17605/OSF.IO/43FQ9). Both in vivo and in vitro studies were included. Studies on the following synaptic markers were included: dendritic structural changes, PSD-95, synapsin-1, synaptophysin-1, synaptotagmin-1, and SV2A.
Results: Eighty-four studies were included in the final analyses. Seventy-one studies examined synaptic markers following ketamine treatment, nine examined psychedelics, and four examined both. Psychedelics included psilocybin/psilocin, lysergic acid diethylamide, N,N-dimethyltryptamine, 2,5-dimethoxy-4-iodoamphetamine, and ibogaine/noribogaine. Mixed findings regarding synaptic changes in the hippocampus and prefrontal cortex (PFC) have been reported when ketamine was administered in a single dose under basal conditions. Similar mixed findings were seen under basal conditions in studies that used repeated administration of ketamine. However, studies that examined animals during stressful conditions found that a single dose of ketamine counteracted stress-related reductions in synaptic markers in the hippocampus and PFC. Repeated administration of ketamine also counteracted stress effects in the hippocampus. Psychedelics generally increased synaptic markers, but results were more consistently positive for certain agents.
Conclusion: Ketamine and psychedelics can increase synaptic markers under certain conditions. Heterogeneous findings may relate to methodological differences, agents administered (or different formulations of the same agent), sex, and type of markers. Future studies could address seemingly mixed results by using meta-analytical approaches or study designs that more fully consider individual differences.
Approximately 20.4 million people in the United States met diagnostic criteria for substance use disorders (SUDs) in 2021 (1). SUDs have been associated with homelessness, incarceration, violence, poor health, and premature death (2–6). Although initially rewarding, chronic use of alcohol and drugs may lead to progressively restricted, habitual, and maladaptive patterns of compulsive seeking and administration of substances (7). The difficulty of treating SUDs is reflected by the lack of medications with indications from the Food and Drug Administration (FDA) for specific types of SUDs (e.g., cocaine and cannabis), while others (e.g., opioids, alcohol, and tobacco) have high relapse rates despite available FDA-approved pharmacotherapies (8–14). Despite their controlled nature and possibility—or concerns—of abuse, ketamine, and psychedelics have potential therapeutic value in treating SUDs and other psychiatric conditions.
The therapeutic potential of ketamine and psychedelics may involve neuronal synaptic plasticity arising from dendritic connections. Originally characterized in the 19th century, dendritic spines are small neuronal protrusions that represent sites of neuronal contact (15). Dendrites on pyramidal neurons can be classified as basal or apical. Basal dendrites are shorter, denser, and receive input from the base of pyramidal cells near the soma, whereas apical dendrites are longer, less dense, and emerge from the neuronal apex (16). A single apical dendrite emerges from the top/apex of a pyramidal neuron and is orientated towards the pial surface. Multiple basilar dendrites emanate from the bottom sides of a pyramidal neuron and extend laterally. Dendritic spine growth occurs as part of synaptic plasticity, which underlies learning phenomena relevant to substance use. This process includes consolidation of memory for the drug along with drug-associated cues and contexts (17), behavioral sensitization (18, 19), conditioned place preference (20, 21), and drug self-administration (22, 23). In cannabis use disorder, verbal memory performance was also shown to be affected (24).
Robinson and Kolb demonstrated that exposure to several drugs of abuse in animals (i.e., amphetamine, cocaine, morphine, nicotine) can alter the number of spines and branches of dendrites in the nucleus accumbens (NAc) and prefrontal cortex (PFC), sometimes in opposite directions (25–28). In addition, animal studies show that synaptic markers can change with intoxication vs. withdrawal (21, 29). In humans with cocaine use disorder (CUD), our group was the first to show lower synaptic markers in the anterior cingulate cortex (ACC), ventromedial PFC, and medial orbitofrontal cortex (OFC), compared to control subjects without CUD (30). Furthermore, individuals with cannabis use disorder were shown to have lower synaptic markers in the hippocampus (24). Drug-induced structural plasticity may underlie protective adaptations to addiction or promote addictive behaviors such as compulsive drug use, craving, and vulnerability to relapse despite sustained abstinence (28). Synaptic proteins can be quantified in both humans and in animal models, offering an indirect measure of both pre-synaptic (e.g., synapsin-1, synaptophysin-1, synaptotagmin-1, SV2A) (31–35) and post-synaptic [e.g., post-synaptic density protein-95 (PSD-95)] (36) function. Synaptic protein markers have been studied in conjunction with dendritic structural changes (37–41)—the growth of dendritic spines can induce precise, synapse-specific effects which affect behavior (24, 42–44). Critically, a gap exists in our understanding of how synaptic protein densities change with psychedelics and drugs of abuse which may facilitate improved treatment of SUDs (12).
Ketamine and psychedelic agents [e.g., psilocybin, lysergic acid diethylamide (LSD), and ibogaine] induce structural plasticity and offer a promising avenue for improving addiction-related outcomes (45). Ketamine and psychedelics have been hypothesized to help shift maladaptive behavioral patterns in addictions, possibly because they can facilitate “transformative experiences” or “spiritual awakenings”. During these transformative experiences individuals report enhanced states of awareness that can produce lasting positive effects on subjective well-being, social attitude, and perceived meaning in life (46–49). Preclinical studies show that these psychoactive substances can modify spine density (50–53) or synaptic proteins (54–58) in brain structures that are involved in cognitive control as well as learning and memory. Despite abuse liability, data suggest potential beneficial effects of ketamine and psychedelics in the treatment of SUDs caused by alcohol (59–62), cocaine (63–67) or opioids (68–70). Ketamine-induced spine growth in the PFC appears necessary for its antidepressant effects (71), raising the possibility that structural plasticity may represent a common mechanism underlying ketamine’s therapeutic effects across psychiatric disorders.
Due to the literature suggesting that alterations to synaptic markers are associated with SUD outcomes in preclinical models, we conducted a scoping review wherein we explored preclinical and clinical literature on the association between administration of ketamine and/or psychedelics and the subsequent changes to synaptic markers.
This review was performed according to the preferred reporting of items for systematic reviews and meta-analysis extension for scoping reviews (PRISMA-ScR) (72), following the framework proposed by the Joanna Briggs Institute (73).
A protocol was created and published in advance, describing the envisioned search strategy, eligibility criteria, study screening and selection process, and data extraction. The protocol was registered on the Open Science Framework (DOI: 10.17605/OSF.IO/43FQ9) and is available online at: https://osf.io/43fq9/.
Both in vitro and in vivo (preclinical and clinical) studies were included. In vivo was defined as any study in a living vertebrate animal, inclusive of humans, excluding non-vertebrates. In vitro was defined as studies of cell lines, organoids, or biological molecules outside their normal biological contexts. Studies on the following synaptic markers were included: structural dendritic changes (e.g., dendritic spine density, arborization), PSD-95, synapsin-1, synaptophysin-1, synaptotagmin-1, and SV2A. Inclusion criteria were as follows: studies analyzing use of ketamine or psychedelics evaluating synaptic markers and published in English. Exclusion criteria included lack of synaptic marker analysis, combination treatment, animal models not related to stress or addiction (e.g., neuronal injury, schizophrenia, dyskinesia), use of immune cells, and use of transgenic animals or genetic strains with significant health abnormalities that could affect synaptic markers (e.g., thyroid dysgenesis) (74). We did not include spine shape classifications in our analyses since they are based on arbitrary criteria and prone to bias (75). Finally, although there are data on structural neural plasticity with non-hallucinogenic psychedelics (e.g., tabernanthalog) (50), the present review did not include those here, given their lack of capacity to induce altered perception, which is in line with the definition of a psychedelic (76).
The search was performed through PubMed, EBSCO, Scopus, and Web of Science up to January 01, 2023. Grey literature was not considered. The search strategy encompassed the concepts of “synaptic density,” “ketamine,” and “psychedelics,” using the terms psilocybin, psilocin, lysergic acid diethylamide, LSD, N,N-Dimethyltryptamine, DMT, mescaline, ibogaine, ayahuasca, 2,5-dimethoxy-4-iodoamphetamine, DOI, ketamine, synaptic density, SV2A, synapsin, synaptotagmin, synaptophysin, PSD 95, dendrit*. The search was applied and adapted according to required syntax for each database. For example, the following search applied to PubMed.
(psilocybin[tiab] OR psilocin[tiab] OR “lysergic acid diethylamide”[tiab] OR LSD[tiab] OR N,N-dimethyltryptamine[tiab] OR DMT[tiab] OR mescaline[tiab] OR ibogaine[tiab] OR ayahuasca[tiab] OR 2,5-Dimethoxy-4-iodoamphetamine[tiab] OR DOI[tiab] OR ketamine[tiab]) AND (“synaptic density”[tiab] OR SV2A[tiab] OR synapsin[tiab] OR synaptotagmin[tiab] OR synaptophysin[tiab] OR “PSD 95”[tiab] OR dendrit*[tiab])
The search with their respective results is presented in Supplementary material. Duplicates were removed with aid of EndNote 20 (Clarivate Analytics, Philadelphia, Pennsylvania, United States). The search was also conducted using the term “synap*” instead of “synaptic density.” Even though the number of papers retrieved was higher, the number of studies included did not change.
For study selection, authors SZ and HO participated in the searching and screening of papers. For studies in which the two reviewers did not reach agreement, a third reviewer was consulted (GA). The screening was performed in two stages. Titles and abstracts were screened first, followed by a full-text screening during the second stage. If the papers met inclusion criteria in stage one, they were moved forward to the stage two. If they did not meet inclusion criteria in either stage, they were excluded.
The data were extracted to a table, with the following information: author, year of publication, agent, dose, route of administration, duration, in vivo or in vitro, animal, line, sex, region, synaptic marker(s), method, time between last administration and evaluation, main outcomes, model (basal and/or stress) and paradigm (administration pre-, mid-, or post-stress) (Supplementary Table S1).
Eighty-four studies were included in the final analysis (Table 1 and Figure 1). Seventy-one studies examined synaptic markers following ketamine treatment, nine examined psychedelics, and four examined both. All were conducted exclusively in animals, except for four (77–80).
A complete list of study characteristics is presented in Supplementary Table S1.
Fourteen studies have examined ketamine’s effect on synaptic markers in vitro, and there was no consistent pattern of outcomes observed across these studies. Instead, different results were found for synaptic markers based on the dosages used and the evaluation times. In rat hippocampal neurons, ketamine (2 μM) did not change spine density or colocalization with synapsin-1 after 1 h of treatment, whereas it increased colocalization of spines with synapsin-1 (suggesting increased presynaptic contacts) after 24 h of treatment (81). However, another study found synapsin-1 was dose-dependently reduced by S-ketamine (3–25 μM) in rat hippocampal neurons (82). There is also evidence that ketamine (100 μM) lowered phosphorylated synapsin (P-S9-synapsin), without affecting synapsin-1 in mouse PFC neurons, suggesting increased presynaptic release potential (83). At lower doses, ketamine (0.1–10 μM) increased dendritic arbor complexity, spine density, and synaptic markers [as measured by colocalization of PSD-95 and vesicular glutamate transporter 1 (VGLUT1)] in rat cortical neurons, mouse mesencephalic dopaminergic neurons, and human pluripotent stem cell-derived dopaminergic neurons (38, 39, 50, 77). Additionally, 4 days of ketamine (100 μM) reversed reduction of PSD-95 expression and spine density in response to 4 days of dexamethasone exposure in primary rat hippocampal cultures (41). By contrast, ketamine (100–500 μM) decreased spine density and/or synaptophysin puncta per μM of dendrites in both rat cortical and hippocampal neurons as well as human striatal projection neurons (79, 80, 84, 85). Finally, in rat GABAergic neurons, ketamine reduced the number of dendritic branching points at higher doses (10 and 20 μg/mL) when treated for 1 h, or at much lower doses (0.01, 0.1 and 1 μg/mL) when neurons were treated for up to 96 h (86, 87).
Thirty-two studies have examined effects of administration of a single dose of ketamine in vivo. Thirty-one such studies involved rodents, and one included monkeys and human subjects. In rodent studies, 0.1–5 mg/kg of ketamine had no effect on PSD-95, synapsin-1, dendritic spine density, or branching in the hippocampus or PFC (88–93). On the other hand, ketamine (1 mg/kg) increased hippocampal PSD-95 and dendritic spine density (94, 95). Interestingly, analysis of sex differences showed that ketamine at 5 mg/kg increased PSD-95 and synapsin-1 in PFC among male, but not female rodents, although neither 2.5 or 5 mg/kg changed spine density (40).
Studies have examined effects of ketamine on both apical and basal dendrite synaptic markers. Ketamine (7.5 mg/kg) increased apical spine markers within the hippocampal CA1 region (96). Furthermore, ketamine (10 mg/kg) increased apical spine density, PSD-95, and synapsin-1 in the mPFC (79, 97–101). Regarding basal spines, ketamine (10 mg/kg) increased spine density in the prelimbic cortex (99). The same dose of ketamine also increased apical spine density in the cerebral cortex (85) and overall spine density in the PFC (38).
In other studies, ketamine (10 mg/kg) did not change PSD-95 levels in the OFC and hippocampus, but it reduced PSD-95 phosphorylation on Thr-19 in hippocampal membranes, suggesting decreased GluA1 receptor internalization (102, 103). Ketamine (10 mg/kg) also increased PSD-95 levels and mPFC post-synaptic density while decreasing PSD-95 levels and hippocampal post-synaptic density. Interestingly, analysis of the homogenate showed ketamine decreased PSD-95 in the mPFC but had no effect in the hippocampus (57). In another study, S-ketamine (10 mg/kg) decreased synapsin-1 level in the hippocampus (104). At a higher dose, S-ketamine (15 mg/kg) increased hippocampal synapsin-1 expression, but decreased synaptotagmin-1 expression (105). In addition, 15 mg/kg of ketamine increased dendritic spine density and arborization in dorsolateral striatal spiny projection neurons (106). At 25 and 50 mg/kg of ketamine, PSD-95 mRNA expression was reduced in the dorsomedial striatum (107). At 150 mg/kg of ketamine, PSD-95 expression was increased in the cerebral cortex (108).
Pregnant rodents that received ketamine (intravenous infusion at a rate of 40–60 mg/kg/h for 2–3 h, or 200 mg/kg infusion for 3 h) produced offspring with reduced dendritic spine density, arborization, and branch number as well as reduced PSD-95, synapsin-1, and synaptophysin-1 in the hippocampus (109–112). The PFC showed increased dendritic spine density, arborization, branch number, and PSD-95, but reduced synaptophysin-1 in the offspring of rodents who were administered ketamine (mean infusion dose = 144 mg/kg over two hours) during pregnancy (113).
Ketamine (0.5 mg/kg) administered to monkeys and humans with major depression/post-traumatic stress disorder (PTSD) did not change SV2A binding 24 h later in the dorsolateral prefrontal cortex (dlPFC), ACC, or hippocampus, as measured with 11C-UCB-J positron emission tomography (PET) imaging (78). However, a post-hoc analysis showed that lower SV2A binding at baseline was associated with ketamine-induced increases in SV2A binding in these regions in humans.
Eight studies in rodents have examined repeated administration of ketamine. At a low dose (0.5 mg/kg; once daily for 11 days) hippocampal synapsin-1 expression was increased (54). In the NAc, ketamine self-administration (0.5 mg/kg/infusion; 3 days a week) for 4 weeks had no effect on spine density (114). When sex differences were considered, ketamine (2.5 and 5 mg/kg; once a week for 7 weeks) was associated with spine density increases among male rodents in the NAc shell, but not in the NAc core, whereas among females, only the 5 mg/kg dose increased spine density in the NAc shell and core (115). Additionally, 10 mg/kg of ketamine (once daily for 21 days) increased hippocampal synapsin-1 expression among males, but not females (116). Finally, ketamine (5–80 mg/kg; daily 5–14 days) decreased dendritic spine density, arborization, PSD-95 expression, and/or post-synaptic density thickness in the hippocampus, dorsal striatum, and/or vmPFC (117–120).
Eight studies have examined ketamine under chronic unpredictable stress (CUS). One study found that CUS did not change PSD-95 or synapsin-1 levels in the PFC, and these markers remained unchanged following ketamine (1 mg/kg) post-treatment (93). However, ketamine pre-treatment (3 mg/kg) or post-treatment (10 mg/kg) increased spine density and/or arborization in the PFC and hippocampal CA1 among resilient rodents and increased spine density in the CA3 among rodents showing anhedonic behavior in the sucrose preference test following CUS exposure (75, 121). Additionally, post-treatment administration of racemic or S-ketamine (10–20 mg/kg) reversed CUS-induced deficits in dendritic spine density, PSD-95, post-synaptic density thickness, and synapsin-1 in the hippocampus and/or PFC (56, 122–125).
Six studies have examined ketamine and chronic corticosterone. Two studies did not find ketamine post-treatment (0.1–1 mg/kg) to effect PSD-95 or synapsin-1 in the hippocampus and PFC of rodents treated chronically with corticosterone (89, 126). By contrast, four studies found that ketamine (1–5 mg/kg) pre- and/or post-treatment ameliorated corticosterone-reduced dendritic arborization, PSD-95, and/or synapsin-1 in the hippocampus (92, 94, 95, 127).
Five studies have examined ketamine with social defeat stress (SDS). SDS decreased dendritic spine density and PSD-95 in the hippocampal DG and CA3 regions and prelimbic cortex/PFC. These effects were reversed by 10 mg/kg ketamine post-treatment with racemic as well as R- and S-ketamine formulations (128–132). By contrast, no form of ketamine altered increased spine density and PSD-95 in the NAc caused by SDS (128, 129, 132).
Four studies have examined ketamine under chronic restraint stress (CRS). Pre-treatment with racemic or R-ketamine (5–10 mg/kg) reversed CRS-induced PSD-95 deficits in the PFC (91, 133). Ketamine post-treatment reversed CRS-induced decreases in synaptophysin-1 in the hippocampus and PFC, with sub-anesthetic doses not reported (134). However, another study found CRS did not lead to hippocampal dendritic spine density decreases and ketamine (10 mg/kg) had no effect when given post-treatment daily for 3 days (135).
Four studies have examined ketamine with the novelty-suppressed feeding test (NSF). Ketamine (0.1–1 mg/kg) administered at the end of 24 h of food deprivation did not change hippocampal PSD-95 or synapsin-1 (136, 51) or spine density (137) in rodents that subsequently underwent NSF participation. Contradictory to these results other studies showed ketamine (1 mg/kg) administration increased hippocampal PSD-95 and synapsin-1 expression (138), as well as dendritic spine density in the same paradigm (51).
Three studies have examined ketamine with foot-shock stress. Ketamine (10 mg/kg) post-treatment reversed decreases in PSD-95 and synapsin-1 in the PFC induced by inescapable foot-shock stress (97). In another study, ketamine (10 mg/kg) post-treatment reversed decreased PSD-95 in the PFC induced by exposure to foot-shock stress conditioning but did not affect increased PSD-95 in the amygdala (139). Finally, acute foot-shock stress did not alter dendritic spine density in the PFC, and this measure did not change after ketamine (10 mg/kg) post-treatment (140).
Two studies have examined ketamine in tail-suspension and open-field tests. Ketamine (0.1 mg/kg) pre-treatment had no effect on dendritic spine density, PSD-95, or synapsin-1 in the hippocampus or PFC (88, 90).
One study has examined ketamine with chronic intermittent cold stress (CIC). Ketamine (10 mg/kg) post-treatment reversed CIC-induced increases in PSD-95 levels in the OFC (103).
One study has examined ketamine with social isolation (SI). Ketamine (5 mg/kg) post-treatment reversed SI-induced reductions in PSD-95, synapsin-1, and dendritic spine density in the PFC of male rodents. Ketamine did not affect synaptic markers following SI in the PFC of female rodents (40).
One study has examined ketamine with respect to lipopolysaccharide administration. R-ketamine (10 mg/kg) post-treatment reversed lipopolysaccharide reductions in dendritic spine density in the prelimbic cortex and hippocampal CA3 and DG regions (141).
Seven studies have examined 2,5-dimethoxy-4-iodoamphetamine (DOI); four were conducted in vitro and three in vivo. The in vitro studies that administered 1–3 μM of DOI found no effect on spine density in cortical neurons or on spine density, PSD-95, or synapsin-1 in hippocampal neurons (142–144). At a higher dose of DOI (10 μM) in vitro, there were increases in dendritic arbor complexity, dendritic branches, spine density, and synaptic markers (measured via colocalization of PSD-95 and VGLUT1) in cortical neurons (38). In vivo, a single-dose of DOI (2 mg/kg) increased spine density in the frontal cortex among rodents with intact 5-HT2A receptors, but not among 5-HT2A-receptor-knockout rodents (145). However, the same dose of DOI had no effect on liposaccharide-induced reductions in spine density in the hippocampus or mPFC (141). Finally, in vivo treatment with DOI 5 μg/0.5 μL injected directly into the left OFC once a week for 3 weeks reduced dendritic spine density and PSD-95 (146).
Three studies have examined psilocin/psilocybin. One study was conducted in vitro and two in vivo. Psilocin (10 μM) in vitro increased dendritic branches and arbor complexity in cortical neurons (38). In vivo studies showed that administration of psilocybin, at doses of 0.08 to 8 mg/kg, increased both PSD-95 and SV2A expression in the PFC and increased SV2A in the hippocampus (55, 58).
Three studies have examined LSD. Two studies were conducted in vitro and one in vivo. LSD (10 μM) in vitro increased dendritic arbor complexity, dendritic branches, spine density, and synaptic markers (measured via colocalization of PSD-95 and VGLUT1) in cortical neurons (38, 39). Moreover, in vivo administration of LSD (30 μg/kg) once daily for 7 days increased spine density in the PFC and reversed CRS-induced reductions in spine density in the PFC of rodents when CRS was administered mid-stress (147).
Two in vitro studies have examined ibogaine/noribogaine. One study reported noribogaine (10 μM) in vitro increased dendritic arbor complexity in cortical neurons while ibogaine did not (38). In the other, both ibogaine and noribogaine (dose not reported) increased dendritic arbor complexity, and ibogaine increased spine density in cortical neurons (50).
Two studies have examined DMT. DMT (90 μM) in vitro increased dendritic branches and arbor complexity in cortical neurons. In vivo DMT (10 mg/kg) also increased dendritic spines in the PFC (38). On the other hand, in vivo DMT (1 mg/kg) every 3rd day for 7 weeks decreased spine density in the PFC of female but not male rodents (148).
A schematic image (Figure 2) illustrates features of included studies investigating ketamine and psychedelics.
Figure 2. Schematic overlapping shapes illustrating features (i.e., number of studies, cells studied, species, doses, etc.) for in vitro and in vivo studies investigating ketamine and psychedelics.
We reviewed in vitro and in vivo studies across species that investigated effects of ketamine or psychedelics on synaptic markers. Data suggest heterogenous findings when ketamine was administered under basal conditions. However, ketamine consistently prevented or reversed stress-induced reductions in synaptic markers in the hippocampus and/or PFC. Existing studies suggest that some psychedelics (e.g., LSD and psilocybin) induce structural plasticity in prefrontal cortical dendrites, although further studies are needed.
Structural plasticity effects can vary by brain region, marker, and behavioral task for ketamine and psychedelics, in some ways reflecting what has been reported for substance use. Studies of substance use suggest mixed results relating to synaptic markers depending on subregions of the brain investigated, whether drugs are experimentally delivered vs. self-administered, and the species being studied (24, 28, 30, 149, 150). Protein quantification (e.g., PSD-95) (57), dose timing (79, 86, 87), and dose-dependent effects (117, 118, 120) may all influence synaptic markers. For example, repeated and/or high-dose treatment with ketamine leads to persistent depression of glutamatergic signaling and prevention of synaptogenesis (84, 107, 117, 118), unlike single treatment with lower doses which can enhance plasticity, particularly when measured with two-photon longitudinal imaging [(e.g., 97–99, 101)]. Ketamine’s ability to reduce synaptic markers at high doses may not be surprising since a meta-analysis showed that single-dose ketamine produces dose- and plasma-level-dependent cognitive impairment (151). Regarding psychedelics, dose effects were examined in one study with psilocybin, but no clear dose-response was observed (55). Too few studies exist per agent to make definitive conclusions regarding dose effects of psychedelics.
The type of agent administered (or different formulations of the same agent) is another potential confounding factor between studies. In general, LSD was the most consistently effective at increasing synaptic markers (38, 147, 39), whereas the data for DOI were the least robust. The ability of psychedelics to increase levels of synaptic markers is believed to be related to activation of 5-HT2A receptors. Stimulation of these receptors leads to enhancement of membrane excitability, most notably in proximal apical dendrites (152). Further support for this hypothesis stems from data showing that the selective serotonergic 5-HT2A/2C antagonist ketanserin completely blocked the ability of LSD, DMT, and DOI to promote both neuritogenesis and spinogenesis (38). Also, DOI enhancement of spine density was absent in 5-HT2A-receptor-knockout animals (145). Interestingly, of psychedelics, LSD has the highest affinity for 5-HT2A receptors, which may explain its effectiveness at increasing synaptic markers (153, 154).
Differences across studies may also be explained by different formulations of ketamine. Blocking N-methyl-D-aspartate (NMDA) receptors with ketamine leads to increased release of glutamate, increased α-amino-3-hydroxy-5-methyl-4-isoxazolepropionic acid (AMPA) activity / receptor expression (155–159), activation of mammalian target of rapamycin complex 1 (mTOR1) (41, 54, 102), and brain-derived neurotrophic factor (BDNF) (41, 115, 129, 132, 138). S-ketamine leads to a maximum plasma level concentration approximately three-fold greater and a binding affinity for NMDA receptors that is approximately five-fold greater than R-ketamine (160, 161).
Sex may influence the effects of ketamine and DMT on synaptic markers. Intriguingly, many studies wherein ketamine failed to alter synaptic markers were conducted solely in females (78, 93, 126, 137, 142). Among studies that included and compared both sexes, a decrease in PFC spine density due to administration of micro-doses of DMT was observed only among female rodents (148). Additionally, a ketamine-induced increase in synaptic markers was found only among male rodents in the hippocampus (116) and PFC (40). Moreover, ketamine administration elevated synaptic markers among females in the NAc shell and core, whereas males exhibited elevations only in the shell (115). Previous studies have shown that levels of male and female sex hormones are positively associated with synaptic markers, which may in part explain sex differences observed across studies (162, 163). Taken together, these studies indicate that the same drug exposure can have different effects on synaptic markers in males vs. females. Thus, sex should be taken into consideration in future studies.
The type of marker is another important confounding variable in determining potential effects of ketamine and/or psychedelics on synaptic markers. Not all markers consistently show changes in the same direction. In some studies, structural dendritic changes are not accompanied by changes in protein markers, and vice-versa (51, 96), while other studies show opposite changes in specific protein markers (104, 113). It is possible that phosphorylation or colocalization of protein markers may be altered without changes to protein expression, but not many studies have examined these variables (38, 39, 83, 102). In addition, some presynaptic markers have been shown to be altered by ketamine, but not by psychedelics, such as synapsin-1 and synaptophysin-1 (55, 144). PSD-95 is a post-synaptic marker of synaptic density that can be changed by ketamine, but there is less evidence for psychedelics (55, 146). PSD-95 regulates synaptic expression and transmission of glutamatergic NMDA and AMPA receptors, which may be the reason behind the ability of ketamine to alter expression of this protein since ketamine works directly via activity at the NMDA receptor (164, 165). SV2A is a synaptic vesicle protein that regulates release of neurotransmitters via action potentials (166) and the only marker that is available to be imaged in vivo with PET imaging using the radioligand 11C-UCB-J in humans (167). Studies have validated SV2A as an alternate marker (to synaptophysin-1) of synaptic density (166). Recent clinical translational studies have documented for the first time decreases in the synaptic marker, SV2A, among persons with cocaine and cannabis use disorder (24, 30). In the studies reviewed herein, psilocybin increased SV2A under basal conditions in pigs (58), whereas ketamine increased SV2A only among humans with depression/PTSD who had low baseline SV2A expression in the hippocampus and PFC (78). Another clinical paper showed that among persons with stress-related mood and anxiety disorders, SV2A expression in the dlPFC was negatively associated with measures of worry and tension/anxiety (168). These data are aligned with other findings showing that excess glucocorticoids/stress negatively impacts spine density, which can be reversed by ketamine (41, 56, 92, 94, 95, 130, 169) and LSD (147). Interestingly, across several studies in which stress exposure did not lead to changes in synaptic markers, ketamine did not influence synaptic markers either (51, 88–90, 93, 126, 135, 140, 136). Future studies should examine changes to SV2A alongside other markers to better elucidate relationships between them when exposed to ketamine and psychedelics.
The present review has several strengths and limitations. It is the first to systematically examine changes to synaptic markers following administration of ketamine and psychedelics. Another strength is the inclusion of both in vivo and in vitro studies. Examining animals in vivo allows access to outcomes based on multiple components of the living organism, whereas measuring effects by in vitro assays may aid to better control for potentially confounding variables (170). We did not see a consistency within species between in vivo and in vitro studies when the treatment was comparable, but we cannot exclude this possibility since most studies utilized rodents. The inclusion of multiple synaptic markers (protein and structural) is another strength because it provides more comprehensive characterization of their associations with administration of ketamine or psychedelics. Although the inclusiveness of multiple markers and methodologies is a strength, at the same time, particular factors of each marker (i.e., level of detectability, etc.) may explain heterogenous findings. In particular, studies that have used two-photon longitudinal imaging to examine structural dendritic changes have repeatedly shown increases in synaptic density in response to single-dose administration of ketamine in vivo (97–99, 101); however, studies that have used other methods to measure dendritic branching or measured protein synaptic markers have shown mixed results, perhaps relating to the complex relationship between proteins, synaptic density, and dendritic architecture. Even though the pattern in which single administration of ketamine/psychedelics results in enhancement of synaptic markers (when measured with two-photon longitudinal imaging) is not well represented in the current results (Supplementary Table S1), this may in part reflect our inclusion/exclusion criteria. For instance, multiple studies using two-photon longitudinal imaging have shown increased synaptic markers using ketamine/psychedelics, but these were not included because they used transgenic animals, which were excluded in the present review (52, 71, 171, 172). A further limitation is that some of the markers reviewed herein are indirect estimates of synaptic density (166, 173, 174) and may only reflect synaptic density alterations to the extent that the gap between different amounts of these proteins within synapses and the actual (i.e., direct) number of synapses is small. Another limitation is that studies reviewed are not in the context of exposure to non-ketamine/non-psychedelic drugs of abuse (i.e., cocaine, opioids, etc.). Thus, the current findings cannot be generalized to such circumstances. There were also few studies directly comparing psychedelic drugs, which limits conclusions about their effects on synaptic markers. Pertaining to regions of interest, most studies have examined ketamine or psychedelic effects on synaptic density or proteins in the hippocampus and/or PFC. Other brain areas such as the striatum, NAc, and OFC may be implicated, but further research is needed to investigate. Finally, associations between microarchitecture, function, cognition, and behavior are not exclusive to quantification of dendrites or spines but also the morphology of each spine. Strong synaptic connections are formed by spines with large heads, which are stable and express large numbers of glutamatergic AMPA receptors, whereas weak, unstable synaptic connections are formed by spines with small heads (175). Here, we did not examine this outcome, which may limit our interpretation of dendritic structural changes.
In the present systematic scoping review, we examined potential effects of ketamine and psychedelics on synaptic markers under basal conditions and stress. The results indicate that, when administered once or repeatedly under basal conditions, ketamine produces mixed results in the hippocampus and PFC, regions implicated in the effects of drugs of abuse. The results for psychedelics also show that they can enhance synaptic markers under basal conditions and reverse deficits associated with stress, but the numbers of studies per agent is low. Some of the null or negative findings relating to ketamine and/or psychedelic effects on synaptic markers may be due to methodological differences, agents administered (or different formulation of the same agent), sex, and/or types of markers. Results also suggest that ketamine may produce more robust results when administered before or after stress to prevent or reverse deficits in synaptic markers in the hippocampus and PFC. Decreased synaptic markers in the hippocampus and PFC may be related to reduced tendencies/abilities to regulate emotion and behavior (176–178), while increases in the striatum may signal increased drug-seeking behavior and behavioral sensitization (19, 22, 179). “Normalization” of dysregulated levels of synaptic markers in some of these brain regions may underlie potential benefits of ketamine and psychedelics in the treatment of SUDs. Further research is required to elucidate relationships between changes to synaptic markers after administration of ketamine or psychedelics and improvements in SUD outcomes.
SZ, HO, LJ, AK, and GA made substantial contributions to the conception or design of the work, or the acquisition, analysis, or interpretation of data for the work. SZ, HO, LJ, AA, AK, MP, and GA made substantial contributions drafting the work or revising it critically for important intellectual content. SZ, HO, and GA agree to be accountable for all aspects of the work in ensuring that questions related to the accuracy or integrity of any part of the work are appropriately investigated and resolved. All authors contributed to the article and approved the submitted version.
Funding was provided by R21DA046030-02 (GA), R01DA052454-02 (MP, SZ, HO, and GA), 5P30DA046345-04 (GA and HO), BBRF NARSAD Young Investigator Award (AK), and NIMH K08 MH122733-01 (AK), which covered percent effort for several co-authors.
AK receives or has received research funding from Transcend Therapeutics and Freedom Biosciences, and has filed a provisional patent for combination psychedelic pharmacotherapies in PTSD.
The remaining authors declare that the research was conducted in the absence of any commercial or financial relationships that could be construed as a potential conflict of interest.
All claims expressed in this article are solely those of the authors and do not necessarily represent those of their affiliated organizations, or those of the publisher, the editors and the reviewers. Any product that may be evaluated in this article, or claim that may be made by its manufacturer, is not guaranteed or endorsed by the publisher.
The Supplementary material for this article can be found online at: https://www.frontiersin.org/articles/10.3389/fpsyt.2023.1197890/full#supplementary-material
1. Substance Abuse and Mental Health Services Administration (SAMSHA) . Key substance use and mental health indicators in the United States: results from the 2020 National Survey on Drug Use and Health (HHS publication no. PEP21-07-01-003, NSDUH series H-56). Rockville, MD: Center for Behavioral Health Statistics and Quality, Substance Abuse and Mental Health Services Administration (2021) Available at: https://www.samhsa.gov/data/.
2. Cornelius, JR , Reynolds, M , Martz, BM , Clark, DB , Kirisci, L , and Tarter, R . Premature mortality among males with substance use disorders. Addict Behav. (2008) 33:156–60. doi: 10.1016/j.addbeh.2007.05.001
3. El-Bassel, N , Shaw, SA , Dasgupta, A , and Strathdee, SA . Drug use as a driver of HIV risks: re-emerging and emerging issues. Curr Opin HIV AIDS. (2014) 9:150–5. doi: 10.1097/COH.0000000000000035
4. Goldman-Hasbun, J , Nosova, E , Kerr, T , Wood, E , and Debeck, K . Homelessness and incarceration associated with relapse into stimulant and opioid use among youth who are street-involved in Vancouver, Canada. Drug Alcohol Rev. (2019) 38:428–34. doi: 10.1111/dar.12921
5. Johnson, EM , and Belfer, ML . Substance abuse and violence: cause and consequence. J Health Care Poor Underserved. (1995) 6:113–21. doi: 10.1353/hpu.2010.0578
6. Moss, R , and Munt, B . Injection drug use and right sided endocarditis. Heart. (2003) 89:577–81. doi: 10.1136/heart.89.5.577
7. Koob, GF , and Volkow, ND . Neurocircuitry of addiction. Neuropsychopharmacology. (2010) 35:217–38. doi: 10.1038/npp.2009.110
8. Allenby, CE , Boylan, KA , Lerman, C , and Falcone, M . Precision medicine for tobacco dependence: development and validation of the nicotine metabolite ratio. J Neuroimmune Pharmacol. (2016) 11:471–83. doi: 10.1007/s11481-016-9656-y
9. Alterman, AI , Mckay, JR , Mulvaney, FD , Cnaan, A , Cacciola, JS , Tourian, KA, et al. Baseline prediction of 7-month cocaine abstinence for cocaine dependence patients. Drug Alcohol Depend. (2000) 59:215–21. doi: 10.1016/S0376-8716(99)00124-6
10. Gossop, M , Stewart, D , Browne, N , and Marsden, J . Factors associated with abstinence, lapse or relapse to heroin use after residential treatment: protective effect of coping responses. Addiction. (2002) 97:1259–67. doi: 10.1046/j.1360-0443.2002.00227.x
11. Maehira, Y , Chowdhury, EI , Reza, M , Drahozal, R , Gayen, TK , Masud, I, et al. Factors associated with relapse into drug use among male and female attendees of a three-month drug detoxification-rehabilitation programme in Dhaka, Bangladesh: a prospective cohort study. Harm Reduct J. (2013) 10:14. doi: 10.1186/1477-7517-10-14
12. Martins, B , Rutland, W , De Aquino, JP , Kazer, BL , Funaro, M , Potenza, MN, et al. Helpful or harmful? The therapeutic potential of medications with varying degrees of abuse liability in the treatment of substance use disorders. Curr Addict Rep. (2022) 9:647–59. doi: 10.1007/s40429-022-00432-9
13. Ullrich, HS , Torbati, A , Fan, W , Arbona, C , Cano, MA , Essa, S, et al. Race, psychosocial characteristics, and treatment outcomes among individuals undergoing treatment for cannabis use disorder: a latent profile analysis based on preferred method of using cannabis. J Subst Abus Treat. (2021) 131:108561. doi: 10.1016/j.jsat.2021.108561
14. Yoshimura, A , Kimura, M , Matsushita, S , Yoneda, JI , Maesato, H , Komoto, Y, et al. Alcohol dependence severity determines the course of treatment-seeking patients. Alcohol Clin Exp Res. (2021) 45:2335–46. doi: 10.1111/acer.14707
15. Lippman, J , and Dunaevsky, A . Dendritic spine morphogenesis and plasticity. J Neurobiol. (2005) 64:47–57. doi: 10.1002/neu.20149
16. Henze, DA , Cameron, WE , and Barrionuevo, G . Dendritic morphology and its effects on the amplitude and rise-time of synaptic signals in hippocampal CA3 pyramidal cells. J Comp Neurol. (1996) 369:331–4. doi: 10.1002/(SICI)1096-9861(19960603)369:3<331::AID-CNE1>3.0.CO;2-6
17. Avchalumov, Y , and Mandyam, CD . Plasticity in the hippocampus, neurogenesis and drugs of abuse. Brain Sci. (2021) 11:404. doi: 10.3390/brainsci11030404
18. Chen, Q , Zhu, X , Zhang, Y , Wetsel, WC , Lee, TH , and Zhang, X . Integrin-linked kinase is involved in cocaine sensitization by regulating PSD-95 and synapsin I expression and GluR1 Ser845 phosphorylation. J Mol Neurosci. (2010) 40:284–94. doi: 10.1007/s12031-009-9218-3
19. Guitart-Masip, M , Johansson, B , Cañete, T , Fernández-Teruel, A , Tobeña, A , Terenius, L, et al. Regional adaptations in PSD-95, NGFI-A and secretogranin gene transcripts related to vulnerability to behavioral sensitization to amphetamine in the Roman rat strains. Neuroscience. (2008) 151:195–208. doi: 10.1016/j.neuroscience.2007.09.072
20. Fan, XY , Shi, G , and Zhao, P . Reversal of oxycodone conditioned place preference by oxytocin: promoting global DNA methylation in the hippocampus. Neuropharmacology. (2019) 160:107778. doi: 10.1016/j.neuropharm.2019.107778
21. Muñoz-Cuevas, FJ , Athilingam, J , Piscopo, D , and Wilbrecht, L . Cocaine-induced structural plasticity in frontal cortex correlates with conditioned place preference. Nat Neurosci. (2013) 16:1367–9. doi: 10.1038/nn.3498
22. Ferrario, CR , Gorny, G , Crombag, HS , Li, Y , Kolb, B , and Robinson, TE . Neural and behavioral plasticity associated with the transition from controlled to escalated cocaine use. Biol Psychiatry. (2005) 58:751–9. doi: 10.1016/j.biopsych.2005.04.046
23. Mcintosh, S , Howell, L , and Hemby, SE . Dopaminergic dysregulation in prefrontal cortex of rhesus monkeys following cocaine self-administration. Front Psychiatry. (2013) 4:88. doi: 10.3389/fpsyt.2013.00088
24. D’Souza, DC , Radhakrishnan, R , Naganawa, M , Ganesh, S , Nabulsi, N , Najafzadeh, S, et al. Preliminary in vivo evidence of lower hippocampal synaptic density in cannabis use disorder. Mol Psychiatry. (2021) 26:3192–200. doi: 10.1038/s41380-020-00891-4
25. Robinson, TE , Gorny, G , Savage, VR , and Kolb, B . Widespread but regionally specific effects of experimenter-versus self-administered morphine on dendritic spines in the nucleus accumbens, hippocampus, and neocortex of adult rats. Synapse. (2002) 46:271–9. doi: 10.1002/syn.10146
26. Robinson, TE , and Kolb, B . Persistent structural modifications in nucleus accumbens and prefrontal cortex neurons produced by previous experience with amphetamine. J Neurosci. (1997) 17:8491–7. doi: 10.1523/JNEUROSCI.17-21-08491.1997
27. Robinson, TE , and Kolb, B . Morphine alters the structure of neurons in the nucleus accumbens and neocortex of rats. Synapse. (1999) 33:160–2. doi: 10.1002/(SICI)1098-2396(199908)33:2<160::AID-SYN6>3.0.CO;2-S
28. Robinson, TE , and Kolb, B . Structural plasticity associated with exposure to drugs of abuse. Neuropharmacology. (2004) 47:33–46. doi: 10.1016/j.neuropharm.2004.06.025
29. Rasakham, K , Schmidt, HD , Kay, K , Huizenga, MN , Calcagno, N , Pierce, RC, et al. Synapse density and dendritic complexity are reduced in the prefrontal cortex following seven days of forced abstinence from cocaine self-administration. PLoS One. (2014) 9:e102524. doi: 10.1371/journal.pone.0102524
30. Angarita, GA , Worhunsky, PD , Naganawa, M , Toyonaga, T , Nabulsi, NB , Li, CR, et al. Lower prefrontal cortical synaptic vesicle binding in cocaine use disorder: An exploratory 11C-UCB-J positron emission tomography study in humans. Addict Biol. (2022) 27:e13123. doi: 10.1111/adb.13123
31. Bajjalieh, SM , Frantz, GD , Weimann, JM , Mcconnell, SK , and Scheller, RH . Differential expression of synaptic vesicle protein 2 (SV2) isoforms. J Neurosci. (1994) 14:5223–35. doi: 10.1523/JNEUROSCI.14-09-05223.1994
32. Goelz, SE , Nestler, EJ , Chehrazi, B , and Greengard, P . Distribution of protein I in mammalian brain as determined by a detergent-based radioimmunoassay. Proc Natl Acad Sci U S A. (1981) 78:2130–4. doi: 10.1073/pnas.78.4.2130
33. Huttner, WB , Schiebler, W , Greengard, P , and De Camilli, P . Synapsin I (protein I), a nerve terminal-specific phosphoprotein. III. Its association with synaptic vesicles studied in a highly purified synaptic vesicle preparation. J Cell Biol. (1983) 96:1374–88. doi: 10.1083/jcb.96.5.1374
34. Masliah, E , Terry, RD , Alford, M , and Deteresa, R . Quantitative immunohistochemistry of synaptophysin in human neocortex: an alternative method to estimate density of presynaptic terminals in paraffin sections. J Histochem Cytochem. (1990) 38:837–44. doi: 10.1177/38.6.2110586
35. Yao, J , Nowack, A , Kensel-Hammes, P , Gardner, RG , and Bajjalieh, SM . Cotrafficking of SV2 and synaptotagmin at the synapse. J Neurosci. (2010) 30:5569–78. doi: 10.1523/JNEUROSCI.4781-09.2010
36. De Bartolomeis, A , and Fiore, G . Postsynaptic density scaffolding proteins at excitatory synapse and disorders of synaptic plasticity: implications for human behavior pathologies. Int Rev Neurobiol. (2004) 59:221–54. doi: 10.1016/S0074-7742(04)59009-8
37. Báez-Becerra, C , Filipello, F , Sandoval-Hernández, A , Arboleda, H , and Arboleda, G . Liver X receptor agonist GW3965 regulates synaptic function upon amyloid beta exposure in hippocampal neurons. Neurotox Res. (2018) 33:569–79. doi: 10.1007/s12640-017-9845-3
38. Ly, C , Greb, AC , Cameron, LP , Wong, JM , Barragan, EV , Wilson, PC, et al. Psychedelics promote structural and functional neural plasticity. Cell Rep. (2018) 23:3170–82. doi: 10.1016/j.celrep.2018.05.022
39. Ly, C , Greb, AC , Vargas, MV , Duim, WC , Grodzki, ACG , Lein, PJ, et al. Transient stimulation with psychoplastogens is sufficient to initiate neuronal growth. ACS Pharmacol Transl Sci. (2020) 4:452–60. doi: 10.1021/acsptsci.0c00065
40. Sarkar, A , and Kabbaj, M . Sex differences in effects of ketamine on behavior, spine density, and synaptic proteins in socially isolated rats. Biol Psychiatry. (2016) 80:448–56. doi: 10.1016/j.biopsych.2015.12.025
41. Seo, MK , Hien, LT , Park, MK , Choi, AJ , Seog, DH , Kim, SH, et al. AMPA receptor-mTORC1 signaling activation is required for neuroplastic effects of LY341495 in rat hippocampal neurons. Sci Rep. (2020) 10:993. doi: 10.1038/s41598-020-58017-3
42. Gao, C , Tronson, NC , and Radulovic, J . Modulation of behavior by scaffolding proteins of the post-synaptic density. Neurobiol Learn Mem. (2013) 105:3–12. doi: 10.1016/j.nlm.2013.04.014
43. Kauer, JA , and Malenka, RC . Synaptic plasticity and addiction. Nat Rev Neurosci. (2007) 8:844–58. doi: 10.1038/nrn2234
44. Lai, CSW , Adler, A , and Gan, WB . Fear extinction reverses dendritic spine formation induced by fear conditioning in the mouse auditory cortex. Proc Natl Acad Sci U S A. (2018) 115:9306–11. doi: 10.1073/pnas.1801504115
45. Kelmendi, B , Kaye, AP , Pittenger, C , and Kwan, AC . Psychedelics. Curr Biol. (2022) 32:R63–7. doi: 10.1016/j.cub.2021.12.009
46. Cloutier-Gill, L , Wood, E , Millar, T , Ferris, C , and Eugenia Socias, M . Remission of severe opioid use disorder with ibogaine: a case report. J Psychoactive Drugs. (2016) 48:214–7. doi: 10.1080/02791072.2016.1180467
47. Forstmann, M , Yudkin, DA , Prosser, AMB , Heller, SM , and Crockett, MJ . Transformative experience and social connectedness mediate the mood-enhancing effects of psychedelic use in naturalistic settings. Proc Natl Acad Sci U S A. (2020) 117:2338–46. doi: 10.1073/pnas.1918477117
48. Mollaahmetoglu, OM , Keeler, J , Ashbullby, KJ , Ketzitzidou-Argyri, E , Grabski, M , and Morgan, CJA . This is something that changed my life: a qualitative study of patients’ experiences in a clinical trial of ketamine treatment for alcohol use disorders. Front Psychiatry. (2021) 12:695335. doi: 10.3389/fpsyt.2021.695335
49. Reiff, CM , Richman, EE , Nemeroff, CB , Carpenter, LL , Widge, AS , Rodriguez, CI, et al. Psychedelics and psychedelic-assisted psychotherapy. Am J Psychiatry. (2020) 177:391–410. doi: 10.1176/appi.ajp.2019.19010035
50. Cameron, LP , Tombari, RJ , Lu, J , Pell, AJ , Hurley, ZQ , Ehinger, Y, et al. A non-hallucinogenic psychedelic analogue with therapeutic potential. Nature. (2021) 589:474–9. doi: 10.1038/s41586-020-3008-z
51. Fraga, DB , Costa, AP , Olescowicz, G , Camargo, A , Pazini, FL , Moretti, M, et al. Ascorbic acid presents rapid behavioral and hippocampal synaptic plasticity effects. Prog Neuro-Psychopharmacol Biol Psychiatry. (2020) 96:109757. doi: 10.1016/j.pnpbp.2019.109757
52. Jefferson, SJ , Gregg, I , Dibbs, M , Liao, C , Wu, H , Davoudian, PA, et al. 5-MeO-DMT modifies innate behaviors and promotes structural neural plasticity in mice. Neuropsychopharmacology. (2023). doi: 10.1038/s41386-023-01572-w
53. Shao, LX , Liao, C , Gregg, I , Davoudian, PA , Savalia, NK , Delagarza, K, et al. Psilocybin induces rapid and persistent growth of dendritic spines in frontal cortex in vivo. Neuron. (2021) 109:2535–2544.e2534. doi: 10.1016/j.neuron.2021.06.008
54. Akinfiresoye, L , and Tizabi, Y . Antidepressant effects of AMPA and ketamine combination: role of hippocampal BDNF, synapsin, and mTOR. Psychopharmacology. (2013) 230:291–8. doi: 10.1007/s00213-013-3153-2
55. Jefsen, OH , Elfving, B , Wegener, G , and Müller, HK . Transcriptional regulation in the rat prefrontal cortex and hippocampus after a single administration of psilocybin. J Psychopharmacol. (2021) 35:483–93. doi: 10.1177/0269881120959614
56. Li, N , Liu, RJ , Dwyer, JM , Banasr, M , Lee, B , Son, H, et al. Glutamate N-methyl-D-aspartate receptor antagonists rapidly reverse behavioral and synaptic deficits caused by chronic stress exposure. Biol Psychiatry. (2011) 69:754–61. doi: 10.1016/j.biopsych.2010.12.015
57. Piva, A , Caffino, L , Mottarlini, F , Pintori, N , Castillo Díaz, F , Fumagalli, F, et al. Metaplastic effects of ketamine and MK-801 on glutamate receptors expression in rat medial prefrontal cortex and Hippocampus. Mol Neurobiol. (2021) 58:3443–56. doi: 10.1007/s12035-021-02352-7
58. Raval, NR , Johansen, A , Donovan, LL , Ros, NF , Ozenne, B , Hansen, HD, et al. A single dose of psilocybin increases synaptic density and decreases 5-HT(2A) receptor density in the pig brain. Int J Mol Sci. (2021) 22:835. doi: 10.3390/ijms22020835
59. Bogenschutz, MP , Forcehimes, AA , Pommy, JA , Wilcox, CE , Barbosa, PC , and Strassman, RJ . Psilocybin-assisted treatment for alcohol dependence: a proof-of-concept study. J Psychopharmacol. (2015) 29:289–99. doi: 10.1177/0269881114565144
60. Krebs, TS , and Johansen, P . Lysergic acid diethylamide (LSD) for alcoholism: meta-analysis of randomized controlled trials. J Psychopharmacol. (2012) 26:994–1002. doi: 10.1177/0269881112439253
61. Krupitsky, EM , and Grinenko, AY . Ketamine psychedelic therapy (KPT): a review of the results of ten years of research. J Psychoactive Drugs. (1997) 29:165–83. doi: 10.1080/02791072.1997.10400185
62. Rothberg, RL , Azhari, N , Haug, NA , and Dakwar, E . Mystical-type experiences occasioned by ketamine mediate its impact on at-risk drinking: results from a randomized, controlled trial. J Psychopharmacol. (2021) 35:150–8. doi: 10.1177/0269881120970879
63. Dakwar, E , Hart, CL , Levin, FR , Nunes, EV , and Foltin, RW . Cocaine self-administration disrupted by the N-methyl-D-aspartate receptor antagonist ketamine: a randomized, crossover trial. Mol Psychiatry. (2017) 22:76–81. doi: 10.1038/mp.2016.39
64. Dakwar, E , Levin, F , Foltin, RW , Nunes, EV , and Hart, CL . The effects of subanesthetic ketamine infusions on motivation to quit and cue-induced craving in cocaine-dependent research volunteers. Biol Psychiatry. (2014) 76:40–6. doi: 10.1016/j.biopsych.2013.08.009
65. Dakwar, E , Nunes, EV , Hart, CL , Foltin, RW , Mathew, SJ , Carpenter, KM, et al. A single ketamine infusion combined with mindfulness-based behavioral modification to treat cocaine dependence: a randomized clinical trial. Am J Psychiatr. (2019) 176:923–30. doi: 10.1176/appi.ajp.2019.18101123
66. Mash, DC , Duque, L , Page, B , and Allen-Ferdinand, K . Ibogaine detoxification transitions opioid and cocaine abusers between dependence and abstinence: clinical observations and treatment outcomes. Front Pharmacol. (2018) 9:529. doi: 10.3389/fphar.2018.00529
67. Mash, DC , Kovera, CA , Pablo, J , Tyndale, RF , Ervin, FD , Williams, IC, et al. Ibogaine: complex pharmacokinetics, concerns for safety, and preliminary efficacy measures. Ann N Y Acad Sci. (2000) 914:394–401. doi: 10.1111/j.1749-6632.2000.tb05213.x
68. Krupitsky, E , Burakov, A , Romanova, T , Dunaevsky, I , Strassman, R , and Grinenko, A . Ketamine psychotherapy for heroin addiction: immediate effects and two-year follow-up. J Subst Abus Treat. (2002) 23:273–83. doi: 10.1016/S0740-5472(02)00275-1
69. Krupitsky, EM , Burakov, AM , Dunaevsky, IV , Romanova, TN , Slavina, TY , and Grinenko, AY . Single versus repeated sessions of ketamine-assisted psychotherapy for people with heroin dependence. J Psychoactive Drugs. (2007) 39:13–9. doi: 10.1080/02791072.2007.10399860
70. Mash, DC , Kovera, CA , Pablo, J , Tyndale, R , Ervin, FR , Kamlet, JD, et al. Ibogaine in the treatment of heroin withdrawal. Alkaloids Chem Biol. (2001) 56:155–71. doi: 10.1016/s0099-9598(01)56012-5
71. Moda-Sava, RN , Murdock, MH , Parekh, PK , Fetcho, RN , Huang, BS , Huynh, TN, et al. Sustained rescue of prefrontal circuit dysfunction by antidepressant-induced spine formation. Science. (2019) 364:eaat8078. doi: 10.1126/science.aat8078
72. Peters, MD , Marnie, C , Tricco, AC , Pollock, D , Munn, Z , Alexander, L, et al. Updated methodological guidance for the conduct of scoping reviews. JBI Evid Synth. (2020) 18:2119–26. doi: 10.11124/JBIES-20-00167
73. Tricco, A , Lillie, E , Zarin, W , O’Brien, KK , Colquhoun, H , Levac, D, et al. PRISMA extension for scoping reviews (PRISMA-ScR): checklist and explanation. Ann Intern Med. (2018) 169:467–73. doi: 10.7326/M18-0850
74. Nakamura, T , Ichii, O , Sunden, Y , Elewa, YHA , Yoshiyasu, T , Hattori, H, et al. Slc:Wistar/ST rats develop unilateral thyroid dysgenesis: a novel animal model of thyroid hemiagenesis. PLoS One. (2019) 14:e0221939. doi: 10.1371/journal.pone.0221939
75. Krzystyniak, A , Baczynska, E , Magnowska, M , Antoniuk, S , Roszkowska, M , Zareba-Koziol, M, et al. Prophylactic ketamine treatment promotes resilience to chronic stress and accelerates recovery: correlation with changes in synaptic plasticity in the CA3 subregion of the hippocampus. Int J Mol Sci. (2019) 20:1726. doi: 10.3390/ijms20071726
77. Cavalleri, L , Merlo Pich, E , Millan, MJ , Chiamulera, C , Kunath, T , Spano, PF, et al. Ketamine enhances structural plasticity in mouse mesencephalic and human iPSC-derived dopaminergic neurons via AMPAR-driven BDNF and mTOR signaling. Mol Psychiatry. (2018) 23:812–23. doi: 10.1038/mp.2017.241
78. Holmes, SE , Finnema, SJ , Naganawa, M , Dellagioia, N , Holden, D , Fowles, K, et al. Imaging the effect of ketamine on synaptic density (SV2A) in the living brain. Mol Psychiatry. (2022) 27:2273–81. doi: 10.1038/s41380-022-01465-2
79. Li, X , Saiyin, H , Zhou, JH , Yu, Q , and Liang, WM . HDAC6 is critical for ketamine-induced impairment of dendritic and spine growth in GABAergic projection neurons. Acta Pharmacol Sin. (2021) 42:861–70. doi: 10.1038/s41401-020-00521-3
80. Li, X , Saiyin, H , Chen, X , Yu, Q , Ma, L , and Liang, W . Ketamine impairs growth cone and synaptogenesis in human GABAergic projection neurons via GSK-3β and HDAC6 signaling. Mol Psychiatry. (2022):1–13. doi: 10.1038/s41380-022-01864-5
81. Waller, JA , Chen, F , and Sánchez, C . Vortioxetine promotes maturation of dendritic spines in vitro: a comparative study in hippocampal cultures. Neuropharmacology. (2016) 103:143–54. doi: 10.1016/j.neuropharm.2015.12.012
82. Sinner, B , Friedrich, O , Zink, W , Zausig, Y , and Graf, BM . The toxic effects of s(+)-ketamine on differentiating neurons in vitro as a consequence of suppressed neuronal Ca2+ oscillations. Anesth Analg. (2011) 113:1161–9. doi: 10.1213/ANE.0b013e31822747df
83. Lazarevic, V , Yang, Y , Flais, I , and Svenningsson, P . Ketamine decreases neuronally released glutamate via retrograde stimulation of presynaptic adenosine A1 receptors. Mol Psychiatry. (2021) 26:7425–35. doi: 10.1038/s41380-021-01246-3
84. Jiang, S , Hao, Z , Li, X , Bo, L , Zhang, R , Wang, Y, et al. Ketamine destabilizes growth of dendritic spines in developing hippocampal neurons in vitro via a rho-dependent mechanism. Mol Med Rep. (2018) 18:5037–43. doi: 10.3892/mmr.2018.9531
85. Zhang, X , Zhao, J , Chang, T , Wang, Q , Liu, W , and Gao, L . Ketamine exerts neurotoxic effects on the offspring of pregnant rats via the Wnt/β-catenin pathway. Environ Sci Pollut Res. (2020) 27:305–14. doi: 10.1007/s11356-019-06753-z
86. Vutskits, L , Gascon, E , Tassonyi, E , and Kiss, JZ . Effect of ketamine on dendritic arbor development and survival of immature GABAergic neurons in vitro. Toxicol Sci. (2006) 91:540–9. doi: 10.1093/toxsci/kfj180
87. Vutskits, L , Gascon, E , Potter, G , Tassonyi, E , and Kiss, JZ . Low concentrations of ketamine initiate dendritic atrophy of differentiated GABAergic neurons in culture. Toxicology. (2007) 234:216–26. doi: 10.1016/j.tox.2007.03.004
88. Camargo, A , Dalmagro, AP , Zeni, ALB , and Rodrigues, ALS . Guanosine potentiates the antidepressant-like effect of subthreshold doses of ketamine: possible role of pro-synaptogenic signaling pathway. J Affect Disord. (2020) 271:100–8. doi: 10.1016/j.jad.2020.03.186
89. Camargo, A , Dalmagro, AP , Wolin, IAV , Siteneski, A , Zeni, ALB , and Rodrigues, ALS . A low-dose combination of ketamine and guanosine counteracts corticosterone-induced depressive-like behavior and hippocampal synaptic impairments via mTORC1 signaling. Prog Neuropsychopharmacol Biol Psychiatry. (2021) 111:110371. doi: 10.1016/j.pnpbp.2021.110371
90. Camargo, A , Dalmagro, AP , Delanogare, E , Fraga, DB , Wolin, I , Zeni, ALB, et al. Guanosine boosts the fast, but not sustained, antidepressant-like and pro-synaptogenic effects of ketamine by stimulating mTORC1-driven signaling pathway. Eur Neuropsychopharmacol. (2022) 57:15–29. doi: 10.1016/j.euroneuro.2021.12.010
91. Camargo, A , Torrá, ACNC , Dalmagro, AP , Valverde, AP , Kouba, BR , Fraga, DB, et al. Prophylactic efficacy of ketamine, but not the low-trapping NMDA receptor antagonist AZD6765, against stress-induced maladaptive behavior and 4E-BP1-related synaptic protein synthesis impairment. Prog Neuro-Psychopharmacol Biol Psychiatry. (2022) 115:110509. doi: 10.1016/j.pnpbp.2022.110509
92. Fraga, DB , Camargo, A , Olescowicz, G , Padilha, DA , Mina, F , Budni, J, et al. Ketamine, but not fluoxetine, rapidly rescues corticosterone-induced impairments on glucocorticoid receptor and dendritic branching in the hippocampus of mice. Metab Brain Dis. (2021) 36:2223–33. doi: 10.1007/s11011-021-00743-2
93. Neis, VB , Bettio, LE , Moretti, M , Rosa, PB , Ribeiro, CM , Freitas, AE, et al. Acute agmatine administration, similar to ketamine, reverses depressive-like behavior induced by chronic unpredictable stress in mice. Pharmacol Biochem Behav. (2016) 150:108–14. doi: 10.1016/j.pbb.2016.10.004
94. Fraga, DB , Camargo, A , Olescowicz, G , Azevedo Padilha, D , Mina, F , Budni, J, et al. A single administration of ascorbic acid rapidly reverses depressive-like behavior and hippocampal synaptic dysfunction induced by corticosterone in mice. Chem Biol Interact. (2021) 342:109476. doi: 10.1016/j.cbi.2021.109476
95. Pazini, FL , Cunha, MP , Rosa, JM , Colla, AR , Lieberknecht, V , Oliveira, Á, et al. Creatine, similar to ketamine, counteracts depressive-like behavior induced by corticosterone via PI3K/Akt/mTOR pathway. Mol Neurobiol. (2016) 53:6818–34. doi: 10.1007/s12035-015-9580-9
96. Hladik, D , Buratovic, S , Von Toerne, C , Azimzadeh, O , Subedi, P , Philipp, J, et al. Combined treatment with low-dose ionizing radiation and ketamine induces adverse changes in CA1 neuronal structure in male murine hippocampi. Int J Mol Sci. (2019) 20:6103. doi: 10.3390/ijms20236103
97. Li, N , Lee, B , Liu, RJ , Banasr, M , Dwyer, JM , Iwata, M, et al. mTOR-dependent synapse formation underlies the rapid antidepressant effects of NMDA antagonists. Science. (2010) 329:959–64. doi: 10.1126/science.1190287
98. Liu, RJ , Lee, FS , Li, XY , Bambico, F , Duman, RS , and Aghajanian, GK . Brain-derived neurotrophic factor Val66Met allele impairs basal and ketamine-stimulated synaptogenesis in prefrontal cortex. Biol Psychiatry. (2012) 71:996–1005. doi: 10.1016/j.biopsych.2011.09.030
99. Liu, RJ , Ota, KT , Dutheil, S , Duman, RS , and Aghajanian, GK . Ketamine strengthens CRF-activated amygdala inputs to basal dendrites in mPFC layer V pyramidal cells in the prelimbic but not infralimbic subregion, a key suppressor of stress responses. Neuropsychopharmacology. (2015) 40:2066–75. doi: 10.1038/npp.2015.70
100. Liu, RJ , Duman, C , Kato, T , Hare, B , Lopresto, D , Bang, E, et al. GLYX-13 produces rapid antidepressant responses with key synaptic and behavioral effects distinct from ketamine. Neuropsychopharmacology. (2017) 42:1231–42. doi: 10.1038/npp.2016.202
101. Wu, M , Minkowicz, S , Dumrongprechachan, V , Hamilton, P , and Kozorovitskiy, Y . Ketamine rapidly enhances glutamate-evoked dendritic spinogenesis in medial prefrontal cortex through dopaminergic mechanisms. Biol Psychiatry. (2021) 89:1096–105. doi: 10.1016/j.biopsych.2020.12.022
102. Beurel, E , Grieco, SF , Amadei, C , Downey, K , and Jope, RS . Ketamine-induced inhibition of glycogen synthase kinase-3 contributes to the augmentation of α-amino-3-hydroxy-5-methylisoxazole-4-propionic acid (AMPA) receptor signaling. Bipolar Disord. (2016) 18:473–80. doi: 10.1111/bdi.12436
103. Paredes, D , Silva, JD , and Morilak, DA . Ketamine corrects a deficit in reversal learning caused by chronic intermittent cold stress in female rats. Int J Neuropsychopharmacol. (2018) 21:1109–13. doi: 10.1093/ijnp/pyy080
104. Wesseling, H , Rahmoune, H , Tricklebank, M , Guest, PC , and Bahn, S . A targeted multiplexed proteomic investigation identifies ketamine-induced changes in immune markers in rat serum and expression changes in protein kinases/phosphatases in rat brain. J Proteome Res. (2015) 14:411–21. doi: 10.1021/pr5009493
105. Müller, HK , Wegener, G , Liebenberg, N , Zarate, CA Jr, Popoli, M , and Elfving, B . Ketamine regulates the presynaptic release machinery in the hippocampus. J Psychiatr Res. (2013) 47:892–9. doi: 10.1016/j.jpsychires.2013.03.008
106. Xu, W , Li, H , Wang, L , Zhang, J , Liu, C , Wan, X, et al. Endocannabinoid signaling regulates the reinforcing and psychostimulant effects of ketamine in mice. Nat Commun. (2020) 11:5962. doi: 10.1038/s41467-020-19780-z
107. De Bartolomeis, A , Sarappa, C , Buonaguro, EF , Marmo, F , Eramo, A , Tomasetti, C, et al. Different effects of the NMDA receptor antagonists ketamine, MK-801, and memantine on postsynaptic density transcripts and their topography: role of homer signaling, and implications for novel antipsychotic and pro-cognitive targets in psychosis. Prog Neuro-Psychopharmacol Biol Psychiatry. (2013) 46:1–12. doi: 10.1016/j.pnpbp.2013.06.010
108. Salort, G , Álvaro-Bartolomé, M , and García-Sevilla, JA . Ketamine-induced hypnosis and neuroplasticity in mice is associated with disrupted p-MEK/p-ERK sequential activation and sustained upregulation of survival p-FADD in brain cortex: involvement of GABAA receptor. Prog Neuro-Psychopharmacol Biol Psychiatry. (2019) 88:121–31. doi: 10.1016/j.pnpbp.2018.07.006
109. Li, Y., Li, X., Guo, C., Li, L., Wang, Y., Zhang, Y., et al. (2017). Long-term neurocognitive dysfunction in offspring via NGF/ERK/CREB signaling pathway caused by ketamine exposure during the second trimester of pregnancy in rats. Oncotarget. 8:30956. doi: 10.18632/oncotarget.16042
110. Li, X., Guo, C., Li, Y., Li, L., Wang, Y., Zhang, Y., et al. (2017). Ketamine administered pregnant rats impair learning and memory in offspring via the CREB pathway. Oncotarget. 8, 32433–32449.
111. Zhang, Z , Zhang, J , Li, J , Zhang, J , Chen, L , Li, Y, et al. Ketamine regulates phosphorylation of CRMP2 to mediate dendritic spine plasticity. J Mol Neurosci. (2020) 70:353–64. doi: 10.1007/s12031-019-01419-4
112. Zhao, T , Li, Y , Wei, W , Savage, S , Zhou, L , and Ma, D . Ketamine administered to pregnant rats in the second trimester causes long-lasting behavioral disorders in offspring. Neurobiol Dis. (2014) 68:145–55. doi: 10.1016/j.nbd.2014.02.009
113. Zhao, T , Li, C , Wei, W , Zhang, H , Ma, D , Song, X, et al. Prenatal ketamine exposure causes abnormal development of prefrontal cortex in rat. Sci Rep. (2016) 6:26865. doi: 10.1038/srep26865
114. Strong, CE , Wright, KN , and Kabbaj, M . Sex and individual differences in alcohol intake are associated with differences in ketamine self-administration behaviors and nucleus accumbens dendritic spine density. eNeuro. (2019) 6:ENEURO.0221-19.2019. doi: 10.1523/ENEURO.0221-19.2019
115. Strong, CE , Schoepfer, KJ , Dossat, AM , Saland, SK , Wright, KN , and Kabbaj, M . Locomotor sensitization to intermittent ketamine administration is associated with nucleus accumbens plasticity in male and female rats. Neuropharmacology. (2017) 121:195–203. doi: 10.1016/j.neuropharm.2017.05.003
116. Thelen, C , Sens, J , Mauch, J , Pandit, R , and Pitychoutis, PM . Repeated ketamine treatment induces sex-specific behavioral and neurochemical effects in mice. Behav Brain Res. (2016) 312:305–12. doi: 10.1016/j.bbr.2016.06.041
117. Chen, ST , Hsieh, CP , Lee, MY , Chen, LC , Huang, CM , Chen, HH, et al. Betaine prevents and reverses the behavioral deficits and synaptic dysfunction induced by repeated ketamine exposure in mice. Biomed Pharmacother. (2021) 144:112369. doi: 10.1016/j.biopha.2021.112369
118. Ogundele, OM , and Lee, CC . CaMKIIα expression in a mouse model of NMDAR hypofunction schizophrenia: putative roles for IGF-1R and TLR4. Brain Res Bull. (2018) 137:53–70. doi: 10.1016/j.brainresbull.2017.11.007
119. Suárez-Santiago, JE , Orozco-Suárez, S , Vega-García, A , Bautista-Orozco, LÁ , and Picazo, O . Repeated ketamine administration induces recognition memory impairment together with morphological changes in neurons from ventromedial prefrontal cortex, dorsal striatum, and hippocampus. Behav Pharmacol. (2020) 31:633–40. doi: 10.1097/FBP.0000000000000571
120. Yin, Y , Li, H , Wang, J , Kong, Y , Chang, J , and Chu, G . Implication of microglia in ketamine-induced long-term cognitive impairment in murine pups. Hum Exp Toxicol. (2022) 41:9603271221128739. doi: 10.1177/09603271221128739
121. Tornese, P , Sala, N , Bonini, D , Bonifacino, T , La Via, L , Milanese, M, et al. Chronic mild stress induces anhedonic behavior and changes in glutamate release, BDNF trafficking and dendrite morphology only in stress vulnerable rats. The rapid restorative action of ketamine. Neurobiology of. Neurobiol Stress. (2019) 10:100160. doi: 10.1016/j.ynstr.2019.100160
122. Hou, L , Miao, J , Meng, H , Liu, X , Wang, D , Tan, Y, et al. Sirtuin type 1 mediates the antidepressant effect of S-ketamine in a chronic unpredictable stress model. Front Psychiatry. (2022) 13:855810. doi: 10.3389/fpsyt.2022.855810
123. Wen, G , Yao, H , Li, Y , Ding, R , Ren, X , Tan, Y, et al. Regulation of tau protein on the antidepressant effects of ketamine in the chronic unpredictable mild stress model. Front Psychiatry. (2019) 10:287. doi: 10.3389/fpsyt.2019.00287
124. Zhang, WJ , Wang, HH , Lv, YD , Liu, CC , Sun, WY , and Tian, LJ . Downregulation of Egr-1 expression level via GluN2B underlies the antidepressant effects of ketamine in a chronic unpredictable stress animal model of depression. Neuroscience. (2018) 372:38–45. doi: 10.1016/j.neuroscience.2017.12.045
125. Zhu, X , Zhang, F , You, Y , Wang, H , Yuan, S , Wu, B, et al. S-ketamine exerts antidepressant effects by regulating Rac1 GTPase mediated synaptic plasticity in the hippocampus of stressed rats. Cell Mol Neurobiol. (2022) 43:299–314. doi: 10.1007/s10571-021-01180-6
126. Neis, VB , Bettio, LB , Moretti, M , Rosa, PB , Olescowicz, G , Fraga, DB, et al. Single administration of agmatine reverses the depressive-like behavior induced by corticosterone in mice: comparison with ketamine and fluoxetine. Pharmacol Biochem Behav. (2018) 173:44–50. doi: 10.1016/j.pbb.2018.08.005
127. Camargo, A , Dalmagro, AP , De Souza, MM , Zeni, ALB , and Rodrigues, ALS . Ketamine, but not guanosine, as a prophylactic agent against corticosterone-induced depressive-like behavior: possible role of long-lasting pro-synaptogenic signaling pathway. Exp Neurol. (2020) 334:113459. doi: 10.1016/j.expneurol.2020.113459
128. Dong, C , Zhang, JC , Yao, W , Ren, Q , Ma, M , Yang, C, et al. Rapid and sustained antidepressant action of the mGlu2/3 receptor antagonist MGS0039 in the social defeat stress model: comparison with ketamine. Int J Neuropsychopharmacol. (2017) 20:228–36. doi: 10.1093/ijnp/pyw089
129. Yang, B , Zhang, JC , Han, M , Yao, W , Yang, C , Ren, Q, et al. Comparison of R-ketamine and rapastinel antidepressant effects in the social defeat stress model of depression. Psychopharmacology. (2016) 233:3647–57. doi: 10.1007/s00213-016-4399-2
130. Yang, C , Shirayama, Y , Zhang, JC , Ren, Q , Yao, W , Ma, M, et al. R-ketamine: a rapid-onset and sustained antidepressant without psychotomimetic side effects. Transl Psychiatry. (2015) 5:e632. doi: 10.1038/tp.2015.136
131. Zhang, J , Qu, Y , Chang, L , Pu, Y , and Hashimoto, K . (R)-ketamine rapidly ameliorates the decreased spine density in the medial prefrontal cortex and hippocampus of susceptible mice after chronic social defeat stress. Int J Neuropsychopharmacol. (2019) 22:675–9. doi: 10.1093/ijnp/pyz048
132. Zhang, JC , Yao, W , Dong, C , Yang, C , Ren, Q , Ma, M, et al. Comparison of ketamine, 7,8-dihydroxyflavone, and ANA-12 antidepressant effects in the social defeat stress model of depression. Psychopharmacology. (2015) 232:4325–35. doi: 10.1007/s00213-015-4062-3
133. Ma, L , Wang, L , Chang, L , Shan, J , Qu, Y , Wang, X, et al. A key role of miR-132-5p in the prefrontal cortex for persistent prophylactic actions of (R)-ketamine in mice. Transl Psychiatry. (2022) 12:417. doi: 10.1038/s41398-022-02192-6
134. Lyu, D , Wang, F , Zhang, M , Yang, W , Huang, H , Huang, Q, et al. Ketamine induces rapid antidepressant effects via the autophagy-NLRP3 inflammasome pathway. Psychopharmacology. (2022) 239:3201–12. doi: 10.1007/s00213-022-06201-w
135. Pochwat, B , Misztak, P , Masternak, J , Bączyńska, E , Bijata, K , Roszkowska, M, et al. Combined hyperforin and lanicemine treatment instead of ketamine or imipramine restores behavioral deficits induced by chronic restraint stress and dietary zinc restriction in mice. Front Pharmacol. (2022) 13:933364. doi: 10.3389/fphar.2022.933364
136. Camargo, A., Pazini, F. L., Rosa, J. M., Wolin, I. A. V., Moretti, M., Rosa, P. B., et al. (2019). Augmentation effect of ketamine by guanosine in the novelty-suppressed feeding test is dependent on mTOR signaling pathway. J Psychiatr Res. 115, 103–112.
137. Rosa, PB , Bettio, LEB , Neis, VB , Moretti, M , Kaufmann, FN , Tavares, MK, et al. Antidepressant-like effect of guanosine involves activation of AMPA receptor and BDNF/TrkB signaling. Purinergic Signal. (2021) 17:285–301. doi: 10.1007/s11302-021-09779-6
138. Pazini, FL , Rosa, JM , Camargo, A , Fraga, DB , Moretti, M , Siteneski, A, et al. mTORC1-dependent signaling pathway underlies the rapid effect of creatine and ketamine in the novelty-suppressed feeding test. Chem Biol Interact. (2020) 332:109281. doi: 10.1016/j.cbi.2020.109281
139. Lee, CW , Chen, YJ , Wu, HF , Chung, YJ , Lee, YC , Li, CT, et al. Ketamine ameliorates severe traumatic event-induced antidepressant-resistant depression in a rat model through ERK activation. Prog Neuro-Psychopharmacol Biol Psychiatry. (2019) 93:102–13. doi: 10.1016/j.pnpbp.2019.03.015
140. Sala, N , Paoli, C , Bonifacino, T , Mingardi, J , Schiavon, E , La Via, L, et al. Acute ketamine facilitates fear memory extinction in a rat model of PTSD along with restoring glutamatergic alterations and dendritic atrophy in the prefrontal cortex. Front Pharmacol. (2022) 13:353. doi: 10.3389/fphar.2022.759626
141. Qu, Y , Chang, L , Ma, L , Wan, X , and Hashimoto, K . Rapid antidepressant-like effect of non-hallucinogenic psychedelic analog lisuride, but not hallucinogenic psychedelic DOI, in lipopolysaccharide-treated mice. Pharmacol Biochem Behav. (2022) 222:173500. doi: 10.1016/j.pbb.2022.173500
142. Jones, KA , Srivastava, DP , Allen, JA , Strachan, RT , Roth, BL , and Penzes, P . Rapid modulation of spine morphology by the 5-HT2A serotonin receptor through kalirin-7 signaling. Proc Natl Acad Sci U S A. (2009) 106:19575–80. doi: 10.1073/pnas.0905884106
143. Mi, Z , Si, T , Kapadia, K , Li, Q , and Muma, NA . Receptor-stimulated transamidation induces activation of Rac1 and Cdc42 and the regulation of dendritic spines. Neuropharmacology. (2017) 117:93–105. doi: 10.1016/j.neuropharm.2017.01.034
144. Roppongi, RT , Kojima, N , Hanamura, K , Yamazaki, H , and Shirao, T . Selective reduction of drebrin and actin in dendritic spines of hippocampal neurons by activation of 5-HT(2A) receptors. Neurosci Lett. (2013) 547:76–81. doi: 10.1016/j.neulet.2013.04.061
145. De La Fuente Revenga, M , Zhu, B , Guevara, CA , Naler, LB , Saunders, JM , Zhou, Z, et al. Prolonged epigenomic and synaptic plasticity alterations following single exposure to a psychedelic in mice. Cell Rep. (2021) 37:109836. doi: 10.1016/j.celrep.2021.109836
146. Xu, C , Ma, XM , Chen, HB , Zhou, MH , Qiao, H , and An, SC . Orbitofrontal cortex 5-HT2A receptor mediates chronic stress-induced depressive-like behaviors and alterations of spine density and Kalirin7. Neuropharmacology. (2016) 109:7–17. doi: 10.1016/j.neuropharm.2016.02.020
147. De Gregorio, D , Inserra, A , Enns, JP , Markopoulos, A , Pileggi, M , El Rahimy, Y, et al. Repeated lysergic acid diethylamide (LSD) reverses stress-induced anxiety-like behavior, cortical synaptogenesis deficits and serotonergic neurotransmission decline. Neuropsychopharmacology. (2022) 47:1188–98. doi: 10.1038/s41386-022-01301-9
148. Cameron, LP , Benson, CJ , Defelice, BC , Fiehn, O , and Olson, DE . Chronic, intermittent microdoses of the psychedelic N,N-dimethyltryptamine (DMT) produce positive effects on mood and anxiety in rodents. ACS Chem Neurosci. (2019) 10:3261–70. doi: 10.1021/acschemneuro.8b00692
149. Russo, SJ , Dietz, DM , Dumitriu, D , Morrison, JH , Malenka, RC , and Nestler, EJ . The addicted synapse: mechanisms of synaptic and structural plasticity in nucleus accumbens. Trends Neurosci. (2010) 33:267–76. doi: 10.1016/j.tins.2010.02.002
150. Spiga, S , Puddu, MC , Pisano, M , and Diana, M . Morphine withdrawal-induced morphological changes in the nucleus accumbens. Eur J Neurosci. (2005) 22:2332–40. doi: 10.1111/j.1460-9568.2005.04416.x
151. Zhornitsky, S , Tourjman, V , Pelletier, J , Assaf, R , Li, CR , and Potvin, S . Acute effects of ketamine and esketamine on cognition in healthy subjects: a meta-analysis. Prog Neuro-Psychopharmacol Biol Psychiatry. (2022) 118:110575. doi: 10.1016/j.pnpbp.2022.110575
152. Savalia, NK , Shao, LX , and Kwan, AC . A dendrite-focused framework for understanding the actions of ketamine and psychedelics. Trends Neurosci. (2021) 44:260–75. doi: 10.1016/j.tins.2020.11.008
153. Halberstadt, AL , and Geyer, MA . Multiple receptors contribute to the behavioral effects of indoleamine hallucinogens. Neuropharmacology. (2011) 61:364–81. doi: 10.1016/j.neuropharm.2011.01.017
154. Schindler, EA , Dave, KD , Smolock, EM , Aloyo, VJ , and Harvey, JA . Serotonergic and dopaminergic distinctions in the behavioral pharmacology of (±)-1-(2,5-dimethoxy-4-iodophenyl)-2-aminopropane (DOI) and lysergic acid diethylamide (LSD). Pharmacol Biochem Behav. (2012) 101:69–76. doi: 10.1016/j.pbb.2011.12.002
155. Abdallah, CG , Sanacora, G , Duman, RS , and Krystal, JH . The neurobiology of depression, ketamine and rapid-acting antidepressants: is it glutamate inhibition or activation? Pharmacol Ther. (2018) 190:148–58. doi: 10.1016/j.pharmthera.2018.05.010
156. Ali, F , Gerhard, DM , Sweasy, K , Pothula, S , Pittenger, C , Duman, RS, et al. Ketamine disinhibits dendrites and enhances calcium signals in prefrontal dendritic spines. Nat Commun. (2020) 11:72. doi: 10.1038/s41467-019-13809-8
157. Koike, H , Iijima, M , and Chaki, S . Involvement of AMPA receptor in both the rapid and sustained antidepressant-like effects of ketamine in animal models of depression. Behav Brain Res. (2011) 224:107–11. doi: 10.1016/j.bbr.2011.05.035
158. Widman, AJ , and McMahon, LL . Disinhibition of CA1 pyramidal cells by low-dose ketamine and other antagonists with rapid antidepressant efficacy. Proc Natl Acad Sci U S A. (2018) 115:E3007–16. doi: 10.1073/pnas.1718883115
159. Witkin, JM , Monn, JA , Schoepp, DD , Li, X , Overshiner, C , Mitchell, SN, et al. The rapidly acting antidepressant ketamine and the mGlu2/3 receptor antagonist LY341495 rapidly engage dopaminergic mood circuits. J Pharmacol Exp Ther. (2016) 358:71–82. doi: 10.1124/jpet.116.233627
160. Ebert, B , Mikkelsen, S , Thorkildsen, C , and Borgbjerg, FM . Norketamine, the main metabolite of ketamine, is a non-competitive NMDA receptor antagonist in the rat cortex and spinal cord. Eur J Pharmacol. (1997) 333:99–104. doi: 10.1016/S0014-2999(97)01116-3
161. Zanos, P , Moaddel, R , Morris, PJ , Riggs, LM , Highland, JN , Georgiou, P, et al. Ketamine and ketamine metabolite pharmacology: insights into therapeutic mechanisms. Pharmacol Rev. (2018) 70:621–60. doi: 10.1124/pr.117.015198
162. Gould, E , Woolley, CS , Frankfurt, M , and McEwen, BS . Gonadal steroids regulate dendritic spine density in hippocampal pyramidal cells in adulthood. J Neurosci Off J Soc Neurosci. (1990) 10:1286–91. doi: 10.1523/JNEUROSCI.10-04-01286.1990
163. Hajszan, T , Milner, TA , and Leranth, C . Sex steroids and the dentate gyrus. Prog Brain Res. (2007) 163:399–415. doi: 10.1016/S0079-6123(07)63023-4
164. Chen, X , Levy, JM , Hou, A , Winters, C , Azzam, R , Sousa, AA, et al. PSD-95 family MAGUKs are essential for anchoring AMPA and NMDA receptor complexes at the postsynaptic density. Proc Natl Acad Sci U S A. (2015) 112:E6983–92. doi: 10.1073/pnas.1517045112
165. Won, S , Incontro, S , Nicoll, RA , and Roche, KW . PSD-95 stabilizes NMDA receptors by inducing the degradation of STEP61. Proc Natl Acad Sci U S A. (2016) 113:E4736–44. doi: 10.1073/pnas.1609702113
166. Finnema, SJ , Nabulsi, NB , Eid, T , Detyniecki, K , Lin, SF , Chen, MK, et al. Imaging synaptic density in the living human brain. Sci Transl Med. (2016) 8:348ra96. doi: 10.1126/scitranslmed.aaf6667
167. Toyonaga, T , Fesharaki-Zadeh, A , Strittmatter, SM , Carson, RE , and Cai, Z . PET imaging of synaptic density: challenges and opportunities of synaptic vesicle glycoprotein 2A PET in small animal imaging. Front Neurosci. (2022) 16:787404. doi: 10.3389/fnins.2022.787404
168. Asch, RH , Holmes, SE , Jastreboff, AM , Potenza, MN , Baldassarri, SR , Carson, RE, et al. Lower synaptic density is associated with psychiatric and cognitive alterations in obesity. Neuropsychopharmacology. (2022) 47:543–52. doi: 10.1038/s41386-021-01111-5
169. Liston, C , and Gan, WB . Glucocorticoids are critical regulators of dendritic spine development and plasticity in vivo. Proc Natl Acad Sci U S A. (2011) 108:16074–9. doi: 10.1073/pnas.1110444108
170. Le Feber, J . In vitro models of brain disorders. Adv Neurobiol. (2019) 22:19–49. doi: 10.1007/978-3-030-11135-9_2
171. Ng, LHL , Huang, Y , Han, L , Chang, RC-C , Chan, YS , and Lai, CSW . Ketamine and selective activation of parvalbumin interneurons inhibit stress-induced dendritic spine elimination. Transl Psychiatry. (2018) 8:272. doi: 10.1038/s41398-018-0321-5
172. Phoumthipphavong, V , Barthas, F , Hassett, S , and Kwan, AC . Longitudinal effects of ketamine on dendritic architecture in vivo in the mouse medial frontal cortex. eNeuro. (2016) 3:ENEURO.0133-15.2016. doi: 10.1523/ENEURO.0133-15.2016
173. Serrano, ME , Becker, G , Bahri, MA , Seret, A , Mestdagh, N , Mercier, J, et al. Evaluating the in vivo specificity of [18F]UCB-H for the SV2A protein, compared with SV2B and SV2C in rats using microPET. Molecules. (2019) 24:1705. doi: 10.3390/molecules24091705
174. Serrano, ME , Kim, E , Petrinovic, MM , Turkheimer, F , and Cash, D . Imaging synaptic density: the next holy grail of neuroscience? Front Neurosci. (2022) 25:796129. doi: 10.3389/fnins.2022.796129
175. Kasai, H , Matsuzaki, M , Noguchi, J , Yasumatsu, N , and Nakahara, H . Structure-stability-function relationships of dendritic spines. Trends Neurosci. (2003) 26:360–8. doi: 10.1016/S0166-2236(03)00162-0
176. Holmes, SE , Scheinost, D , Finnema, SJ , Naganawa, M , Davis, MT , Dellagioia, N, et al. Lower synaptic density is associated with depression severity and network alterations. Nat Commun. (2019) 10:1529. doi: 10.1038/s41467-019-09562-7
177. Kelley, NJ , Gallucci, A , Riva, P , Romero Lauro, LJ , and Schmeichel, BJ . Stimulating self-regulation: a review of non-invasive brain stimulation studies of goal-directed behavior. Front Behav Neurosci. (2019) 12:337. doi: 10.3389/fnbeh.2018.00337
178. Zhu, Y , Gao, H , Tong, L , Li, Z , Wang, L , Zhang, C, et al. Emotion regulation of hippocampus using real-time fMRI neurofeedback in healthy human. Front Hum Neurosci. (2019) 13:242. doi: 10.3389/fnhum.2019.00242
Keywords: synaptic density, SV2A, PSD-95, synapsin-1, synaptophysin-1, synaptotagmin-1, dendrite, addiction
Citation: Zhornitsky S, Oliva HNP, Jayne LA, Allsop ASA, Kaye AP, Potenza MN and Angarita GA (2023) Changes in synaptic markers after administration of ketamine or psychedelics: a systematic scoping review. Front. Psychiatry 14:1197890. doi: 10.3389/fpsyt.2023.1197890
Received: 31 March 2023; Accepted: 05 June 2023;
Published: 26 June 2023.
Edited by:
Brian Barnett, Cleveland Clinic, United StatesReviewed by:
Yan Li, Apellis Pharmaceuticals (United States), United StatesCopyright © 2023 Zhornitsky, Oliva, Jayne, Allsop, Kaye, Potenza and Angarita. This is an open-access article distributed under the terms of the Creative Commons Attribution License (CC BY). The use, distribution or reproduction in other forums is permitted, provided the original author(s) and the copyright owner(s) are credited and that the original publication in this journal is cited, in accordance with accepted academic practice. No use, distribution or reproduction is permitted which does not comply with these terms.
*Correspondence: Gustavo A. Angarita, Z3VzdGF2by5hbmdhcml0YUB5YWxlLmVkdQ==
†These authors have contributed equally to this work and share first authorship
Disclaimer: All claims expressed in this article are solely those of the authors and do not necessarily represent those of their affiliated organizations, or those of the publisher, the editors and the reviewers. Any product that may be evaluated in this article or claim that may be made by its manufacturer is not guaranteed or endorsed by the publisher.
Research integrity at Frontiers
Learn more about the work of our research integrity team to safeguard the quality of each article we publish.