- 1George A. Jervis Clinic, New York State Institute for Basic Research in Developmental Disabilities, Staten Island, NY, United States
- 2Department of Psychiatry, State University of New York Downstate Medical Center, Brooklyn, NY, United States
- 3Research Design and Analysis Service, New York State Institute for Basic Research in Developmental Disabilities, Staten Island, NY, United States
Introduction: Cyclic adenosine monophosphate (cAMP) levels in the lymphoblasts and leukocytes of patients with major depressive disorder (MDD) have been reported to be downregulated compared to in controls. cAMP is a derivative of adenosine triphosphate (ATP), and low ATP turnover has been reported in the state of hypometabolism associated with human MDD and with mammalian hibernation due to suppression of mitochondrial metabolism. Similarities have been noted between many state-dependent neurobiological changes associated with MDD in humans and with mammalian hibernation.
Methods: To compare cAMP levels between human MDD and mammalian hibernation and to investigate whether cAMP downregulation is another state-dependent neurobiological finding, we measured cAMP concentrations in lysed leukocytes, plasma, and serum in serial blood specimens from nine female captive black bears (Ursus americanus; CBBs), and cortisol levels in serum from 10 CBBs.
Results: Cortisol levels were significantly higher during hibernation in CBBs, confirming previous findings in hibernating black bears and similar to findings in humans with MDD. cAMP levels were significantly lower during hibernation versus active states (pre-hibernation and exit from hibernation) and were similar to the cAMP downregulation reported in MDD patients versus euthymic patients or controls. cAMP level changes during the different states (hibernation, pre-hibernation, active) confirm their state-dependent status.
Discussion: These findings are similar to the neurobiological findings associated with the hypometabolism (metabolic depression) observed during mammalian hibernation and reported during MDD. A sudden increase in cAMP levels was observed before entrance into pre-hibernation and during exit from hibernation. Further investigation is suggested into the possible role of elevated cAMP levels in initiation of the chain reaction of changes in gene expression, proteins, and enzymes leading to the suppression of mitochondrial metabolism and to low ATP turnover. This process leads to hypometabolism, the old adaptive mechanism that is used by organisms for energy preservation and is associated with both mammalian hibernation and human MDD.
Introduction
The cyclic adenosine monophosphate (cAMP) signaling pathway is a second messenger, and because its regulation at the intracellular level is critical for the transduction of cellular response, the role of cAMP generation in bipolar disorder and especially major depressive disorder (MDD) has been studied extensively [see review, (1)]. Most of these studies, using different materials, specimens, and methods, reported a downregulation in the production of cAMP in patients with MDD versus normal controls and patients in a euthymic or a manic state (1).
Mean levels of cAMP in the plasma of patients with bipolar disorder were not different between the different phases of their illness or compared to controls (2). Beta adrenoreceptor–mediated cAMP formation, using histamine, prostaglandin E1, or isoproterenol, was not different in the lymphoblasts of depressed patients versus controls (3), and no difference was found either in the affinity or the density of beta receptors on lymphoblasts between patients with bipolar disorder and controls (3, 4). Studies of histamine-stimulated and beta adrenoreceptor–mediated cAMP formation in leukocytes from patients with MDD and controls reported lower cAMP levels (i.e., downregulation) in patients with MDD versus controls (5–7). When cAMP formation was measured in isoproterenol-stimulated lymphocytes and not in leukocytes, lower cAMP levels were found only in patients with MDD and psychomotor agitation, not in patients with psychomotor retardation (8, 9). Reduction in beta-adrenoreceptor–linked cAMP-dependent protein kinase (PKA) was reported in patients with MDD and melancholic features versus controls (10), suggesting low levels of cAMP.
The heterogeneity of the subjects and the differences in disease severity, in the specimens in which cAMP was measured (plasma, stimulated leukocytes/lymphocytes, and platelets), and in the methods used for determination of cAMP levels may have contributed to the different, and in certain studies, opposite, findings (1).
The theory of cAMP dysfunction as a cause of MDD and of depression in general was proposed (11) on the basis of findings of cAMP-signaling downregulation in MDD and its upregulation with chronic administration of antidepressants in one postmortem study in humans (12) and in studies in rodents (13). This theory was further supported by findings of decreased cAMP signaling in the brains of unmedicated depressed patients and increased cAMP signaling after treatment with a selective serotonin reuptake inhibitor using 11C-(R)-rolipram PET scan to image phosphodiesterase 4 (PDE4) (14). Neither of these studies nor any cAMP studies investigated whether the reported changes are state-dependent or not.
The mitochondrial dysfunction theory of MDD was proposed to explain the downregulation of cAMP and the hypometabolism observed during MDD (15, 16). However, recent studies have linked suppression of mitochondrial function, including low adenosine triphosphate (ATP) turnover, but not mitochondrial dysfunction, to MDD and have suggested the need for further investigation of mitochondrial changes during MDD (17–20).
A theory explaining the origin of the hypometabolism observed during MDD was proposed by the first author in 2005 (21), arguing that a form of metabolic depression, a primitive adaptive process for energy preservation that is homologous to the one responsible for mammalian hibernation—and not mitochondrial dysfunction—is the process underlying the observed hypometabolism, state-dependent neurobiological changes (22, 23), and vegetative symptoms of MDD in humans. Supporting evidence for this metabolic depression theory of MDD and for its link to the process of mammalian hibernation (21) is the finding that suppression of mitochondrial metabolism is the process responsible for mammalian hibernation (24, 25), including low ATP turnover (26, 27), as well as the similarities to the findings from mitochondrial studies in MDD (17–20).
The following state-dependent neurobiological changes that have been documented as being similar between MDD in humans and mammalian hibernation offer further support for the metabolic depression theory of MDD (21): reversible subclinical hypothyroidism (28, 29), increased serum cortisol concentration (30–32), acute phase protein response (33–38), low respiratory quotient (39–42), oxidative stress response (43–45), and decreased neurotransmitter levels (39, 46, 47). Black bears (Ursus americanus) were suggested and used as an animal model for MDD to study the process of hypometabolism during hibernation (21) because many of the similar neurobiological changes between MDD and mammalian hibernation had been observed in studies of bears, especially black bears, during hibernation (29, 32, 36, 37, 40, 45). The full rationale for using this model has been explained previously [(21); see pp. 830–831]. Black bears in the wild withdraw into dens in October, and by November 1st, most are in the state of hibernation proper without becoming hypothermic (32, 40, 41). Hypothermia, which is common in all other hibernating mammals, suppresses gene expression and the translation and transcriptional machinery of many proteins (48).
Mammalian hibernation is a state of metabolic stress, which leads to the dephosphorylation of ATP through the activation of adenylyl cyclase and the formation of cAMP, which is converted to adenosine 5’ nucleotidase (5’ AMP) (1, 49). According to these findings (49), higher cAMP concentration would be expected in the lysed leukocytes of black bears before entrance into hibernation, the basic state of hypometabolism, when the process shifts from normal to the state of metabolic depression, and low cAMP levels are expected during hibernation proper. To compare our expected findings of cAMP level changes during mammalian hibernation with the reported findings during MDD in humans, to investigate the state-dependent status of cAMP levels, and to obtain additional supporting evidence for the metabolic depression theory of MDD (21), we measured the baseline changes of cAMP levels in serial blood samples obtained every 15 days during active, pre-hibernation, and hibernation states from nine female captive black bears (CBBs) kept in captivity from October to May 15. Serum cortisol levels also were measured from the serial samples obtained from these same CBBs plus one additional CBB. We report our findings here.
Materials and methods
Materials
The cyclic AMP (direct) colorimetric ELISA kit and the cortisol ELISA kit were obtained from Assay Designs Inc. (Ann Arbor, MI, United States). All other chemicals were of reagent grade.
Subjects
Serial blood samples were obtained every 15 days from 11 female CBBs 3 to 21 years of age (mean age 11, SD 6.67), six of which were pregnant. These CBBs were kept in captivity from October to April at the Center for Ursid Research at Virginia Polytechnic Institute and State University (VPI) in Blacksburg, VA, United States. One of these CBBs was captive from October 2002 to May 15, 2003; seven, from October 2003 to May 15, 2004; and three, from October 2004 to May 15, 2005. Ages of the CBBs from which specimens were obtained for analysis were 3–8 years (n = 3 for cAMP levels, and n = 4 for cortisol levels), 9–15 years (n = 4), and older than 15 years (n = 2). Although specimens were obtained from 11 CBBs from October 2002 to May 2005, only nine were included in the analyses for cAMP levels, and 10 for serum cortisol levels. One CBB, 2 years of age, was excluded from the cortisol and cAMP level analyses because it never fell into hibernation, and a second one was excluded from cAMP analyses because only one cAMP specimen was obtained. During the active state after captivity, in October, only a few cAMP specimens were received and thus were excluded from analysis. The serum cortisol specimens obtained in October also were excluded from analysis, because of the sudden increase in levels resulting from the stress of captivity.
Trapping and immobilization of animals
All of the CBBs were captured in the George Washington National Forest and Jefferson National Forest in western Virginia. They were trapped in either Aldrich leg-hold snares or culvert traps, and all were anesthetized with a 2:1 mixture of ketamine hydrochloride (Ketaset®, Fort Dodge Animal Health, Fort Dodge, IA, United States) and xylazine hydrochloride (Rompum®, Bayer Corporation, Shawnee Mission, KS, United States) (concentration 300 mg/mL) at a dose rate of 1 mL/45 kg body mass. Telazol® was not used. No CBBs were in a trap for more than 20 h, and most were in traps for less than 12 h. All CBBs were healthy when the blood samples were drawn. Protocols for handling wild black bears and CBBs were approved by the VPI Animal Care Committee and the Institutional Animal Care and Use Committee at the New York State Institute for Basic Research in Developmental Disabilities (IBR). For further details, see Tsiouris et al. (37) and Donahue et al. (50).
Captive black bears entered hibernation proper between late December and January 1—not by November 1, as in most black bears in the wild (32, 41)—because of food availability and the higher temperature of the quarters in which they were kept captive. Each CBB was fed a daily ration of 2,000 g of dry high-protein (23%) dog food until November 30. Rations were cut to 1,000 g on December 1, to 500 g on December 10, and to 250 g on December 20. Feeding was terminated on December 30 and not resumed until April 1. Pregnant CBBs gave birth to their cubs during the last week of January without exiting the hibernation state. CBBs exited the hibernation state and became active in late March through April 1st similarly to black bears in the wild.
States during captivity
Active State: After captivity; October 1–October 31.
Pre-hibernation State: November 1–December 18 (early December).
Proper Hibernation State: December 19 (late December)–March 18 (early March).
Exit from hibernation/Active State: March 19 (late March)–May 15.
Black bear blood and sera
The number of blood specimens obtained from the nine CBBs for cAMP levels was 58, and of serum specimens obtained from 10 CBBs for cortisol levels was 137. Sera were prepared on-site and stored frozen at VPI until shipped. Whole-blood samples were collected in heparinized tubes and immediately shipped overnight to IBR on ice packs. Because only one specimen was obtained from each of four CBBs at the end of April after exit from hibernation, they were not included in the analysis.
Harvesting of black bear leukocytes
Whole blood was centrifuged for 10 min @ 2,000 × g (4°C). The buffy coat was collected and washed once in tris-buffered saline (TBS). The cells were collected by centrifugation, and the pellets were suspended in TBS, aliquoted, and stored at −80°C until needed.
Assay of protein concentration
Protein concentrations were determined by the method of Lowry et al. (51) by using bovine serum albumin as standard.
cAMP levels in black bear leukocytes
Preparation of leukocytes
Blood was drawn from female CBBs in heparinized (green-top) tubes and shipped by overnight express from VPI to IBR. The tubes were centrifuged at 800 × g for 15 min at room temperature (RT). The plasma and buffy-coat were removed to clean tubes and were centrifuged at 2,000 × g for 15 min at RT. The plasma was removed, and the cells were washed with an isotonic tris-buffered saline (TBS; pH 8.0) and re-centrifuged. The washing was repeated once. The cells were re-suspended in TBS, quick-frozen on dry ice, and stored at −80°C.
Assay of adenosine 3’, 5’–cAMP
The concentration of cAMP in leukocytes was determined with direct cAMP colorimetric ELISA kit (Assay Designs, Inc., Ann Arbor, MI, United States), following the manufacturer’s protocol. This kit is designed for use with cell culture and tissue and includes 0.1 M HCl, which is used to lyse the cells, stop endogenous PDE activity, and stabilize the released cAMP. The 0.1% Hcl-treated samples are then analyzed directly in a microtiter plate without extraction, drying, and reconstitution. Because the sample-to-sample yield of leukocytes was variable, aliquots of the cells were removed to measure the total protein concentration, after which the cAMP values were normalized to 100 μg of total protein.
Cortisol levels in black bear sera
The levels of cortisol in black bear sera were determined by using the cortisol ELISA kit (Assay Designs, Inc.) following the manufacturer’s protocol.
Results
Assays of cAMP levels were performed on 137 serum samples, 58 plasma samples, and 58 samples of lysed leukocytes. Levels of cAMP from plasma samples and from lysed leukocytes were positively correlated (r = 0.49, p = 0.0001, N = 57), although samples from serum were negatively correlated with measurements of both plasma (r = −0.50, p = 0.0002, N = 50) and lysed leukocytes (r = −0.33, p = 0.02, N = 51). However, these correlations are in large part autocorrelations among repeated measures; correlations adjusted for autocorrelation are much lower (lysed leukocytes and plasma, rho = 0.07, p = 0.62; lysed leukocytes and serum, rho = −0.01, p = 0.94; plasma and serum, rho = −0.004, p = 0.9815) (52, 53). Measurements of cAMP levels in lysed leukocytes were used for analyses for both robustness of measurements and comparison with published research.
Before the analyses were performed, distributions of cAMP and cortisol measurements were tested for normality using the Shapiro-Wilk W test. Measurements of both factors were found to be severely skewed to the right. A square-root transformation was applied to both variables, making them acceptably normal. Comparisons of groups were performed using generalized estimating equations (GEEs) (54, 55) to avoid the distortion of variance estimates arising from autocorrelation of each bear’s multiple measurements. Robust estimates of variance were employed in all analyses. Analyses were performed using Stata version 16.1 (56).
Tables 1, 2 provide the number of measurements, their means, and the standard deviations for cortisol and cAMP levels by state of hibernation, overall, and divided by pregnancy status and age group.
Figures 1–3 show cortisol and cAMP levels throughout captivity, overall, and divided by pregnancy status and age group.
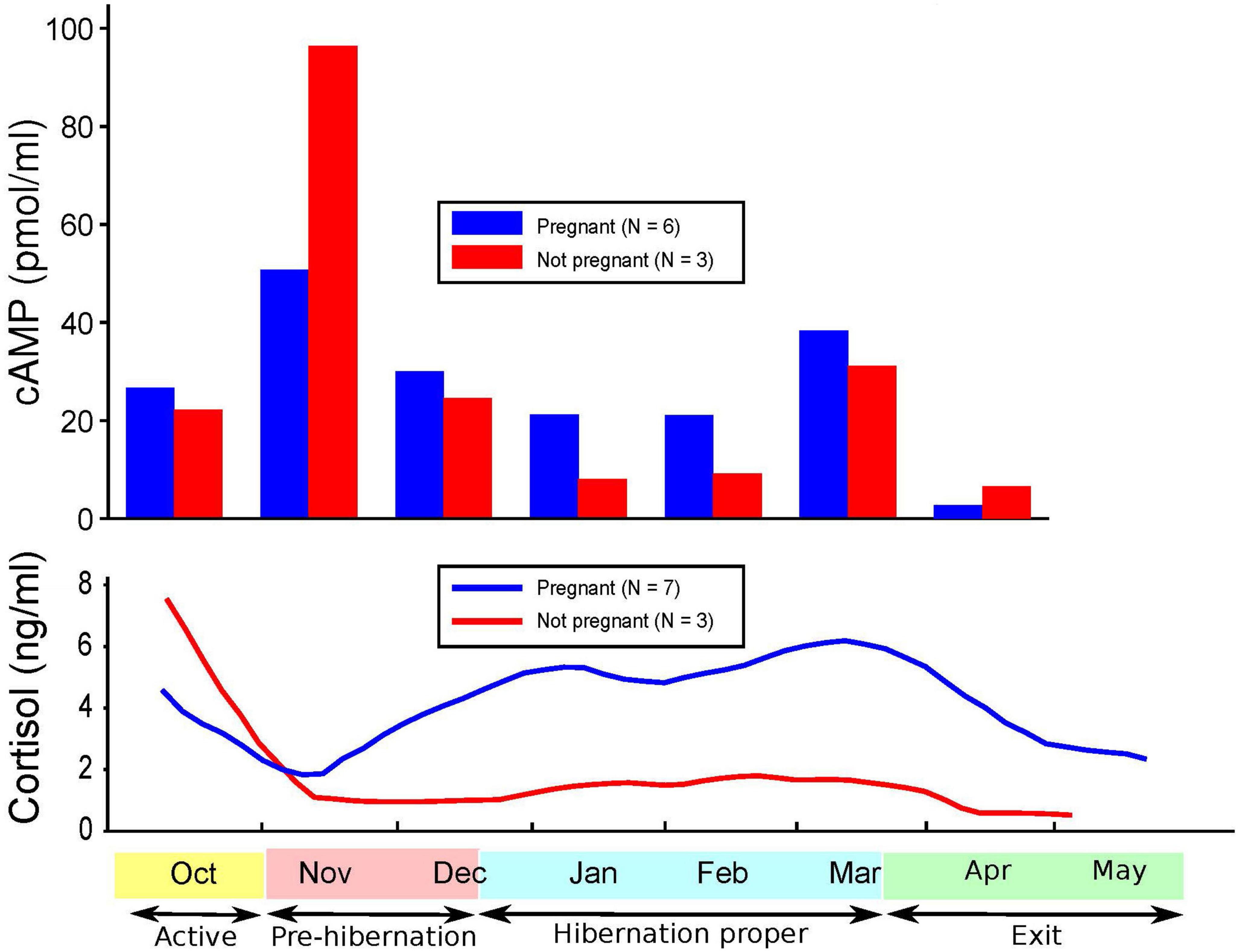
Figure 2. Levels of cyclic adenosine monophosphate (cAMP) and cortisol over time and pregnancy status.
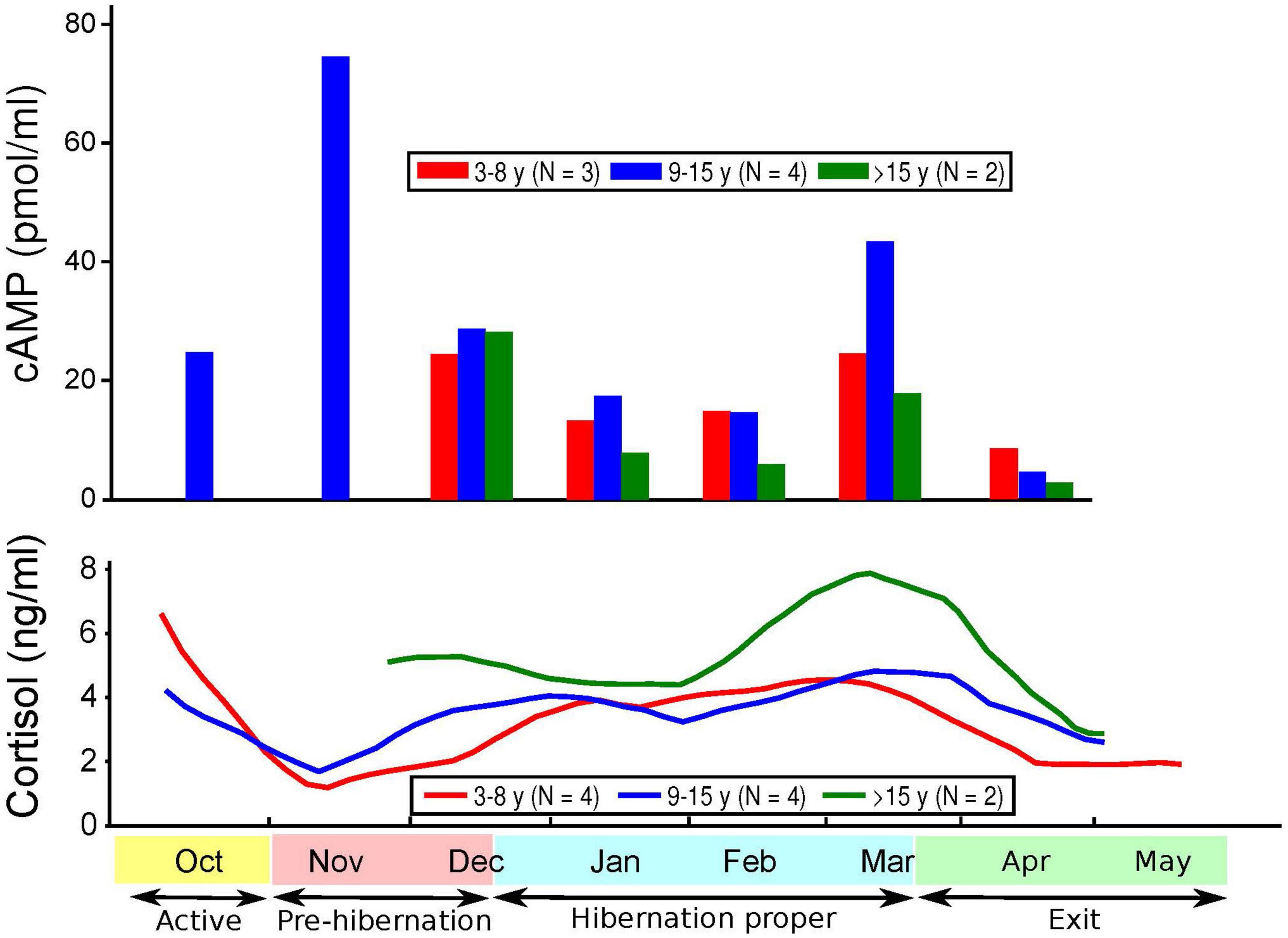
Figure 3. Levels of cyclic adenosine monophosphate (cAMP) and cortisol over time and age of subjects.
Cortisol levels were found to be very high in the sera of the CBBs after their captivity. The levels of cortisol decreased in the weeks after the start of captivity during the month of November, increased during hibernation, and decreased again after the exit from hibernation into the active state. The age and pregnancy status of the CBBs influenced the levels of cortisol, but not the direction of level changes, in the sera during the active and hibernation states. Cortisol levels were significantly higher during hibernation (mean 4.28, SD 4.71, N = 65) than during pre-hibernation and the exit state immediately following hibernation (mean 3.22, SD 4.22, N = 68, Wald chi2(1) = 9.88, p = 0.0017).
Cyclic adenosine monophosphate levels in the lysed leukocytes of CBBs were higher in the active state after captivity and before the entrance into pre-hibernation (November and early December) than during the hibernation state (late December–early March). Levels increased again moderately at the end of hibernation (late March) during exit from hibernation (active state). The age and pregnancy status of the CBBs influenced the levels of cAMP, but not the direction of level changes, in the lysed leukocytes during the different active and hibernation states. Levels of cAMP were significantly lower during hibernation (mean 17.44, SD 13.97, N = 30) than during pre-hibernation and the exit state immediately following hibernation (mean 38.16, SD 29.84, N = 26, Wald chi2(1) = 4.24, p = 0.0395).
Thus, the changes in the levels of cAMP in lysed leukocytes and of cortisol in serum were in opposite directions with entry into pre-hibernation: cAMP levels increased, and cortisol levels decreased. Both levels increased in late March during the exit from hibernation.
Discussion
Our findings of significantly increased cortisol levels in the serum of hibernating CBBs confirm similar previous findings (32, 57) and are similar to the increased cortisol levels reported in the severe type of MDD in humans (30, 31).
The high levels of cortisol measured in the serum of the CBBs immediately after their captivity are due to the glucocorticoid stress responses to the capture of wild, free-living mammals (58) and have similarities to the stress response in humans (59).
Our findings of significantly lower cAMP levels in CBBs during hibernation versus their active state are similar to the findings of lower cAMP levels in humans with MDD versus in controls (5–7, 10) and in humans with MDD with psychomotor agitation (8). The lack of differences reported in the cAMP levels in the plasma of patients in different states (depressed, manic, euthymic) and matched controls (2) could be explained by our findings of a very weak correlation between the cAMP levels in leukocytes and plasma in CBBs. These findings suggest that plasma is not a good medium for documenting changes in cAMP levels in different states. cAMP levels in the serum of CBBs were negatively correlated with both plasma and leukocyte cAMP levels. Also, our findings were opposite to the lack of differences observed in cAMP production between patients diagnosed with schizophrenia or MDD versus drug-free normal controls (60). The increase in cAMP levels during the entrance into pre-hibernation, especially in pregnant and 5–15 years-old CBBs, and the decrease in cAMP levels in the following weeks are a new finding, which must be replicated in male and female black bears in the wild and in captivity, and in humans when entering into severe MDD, especially with melancholic/catatonic features.
The age and pregnancy status of CBBs influenced the degree of changes in both cortisol and cAMP levels but not the direction of the changes. Similar variability in the findings has been reported in cAMP levels in human MDD (1).
Metabolic stress triggers the dephosphorylation of ATP and the formation of cAMP (49). Whether the increase in cAMP levels in CBBs in the month of November is associated with low levels of ATP due to dephosphorylation and formation of cAMP in mammalian hibernation (27, 58, 59) and possibly in MDD (17–20) must be investigated.
Low cAMP levels, which decrease activation of PKA, and lower ATP turnover are both associated with reduced energy production, which leads to hypometabolism (metabolic depression), the process underlying mammalian hibernation (24, 26, 27, 61, 62). There are no published studies measuring cAMP level changes during active or hibernation states in bears or other large mammals that hibernate without becoming hypothermic (40) against which our findings can be compared. Research will be required to confirm our findings and their similarities with the findings in MDD.
Research also will be required to determine whether the drop in cAMP level after its increase with the initiation of pre-hibernation in CBBs and with the possible initiation of hibernation in black bears in the wild is associated with the increased production of 5’ AMP through the enzymatic hydrolysis of cAMP carried out by PDE4. PDE4 cleaves the 3;5’ PDE bond in cAMP, thus inhibiting the phosphorylating activity of PKA (49).
5’ AMP levels have been found to be elevated in mice during dark/dark periods, and injection of 5’ AMP in light/dark periods in non-hibernating mice produced torpor-like changes (63). 5’ AMP has been reported to be one of the triggers (circadian signals) for the initiation of the hypometabolic state and the molecular process involved in 5’ AMP-induced hypometabolism, but this process occurs at the metabolic interconversion level rather than at the gene or protein expression level, as it has been observed that widespread suppression of energy-generating metabolic pathways occurs during this process (64).
Hypometabolism is the result of reduced energy production (19), which occurs secondary to decreased ATP turnover respiration in depressed patients versus controls (18), and to decreased brain levels of ATP and differentially expressed proteins in depressed patients, which are mainly related to deregulation (downregulation) or energy metabolism pathways (17).
The major characteristics of MDD in humans, as per the DSM-5 (65), are lack of energy, fatigue, lack of interest, lack of motivation, avoidance of interaction, weight loss, insomnia, and psychomotor retardation, all of which are secondary to hypometabolism and are associated with low ATP turnover (18, 19). This condition, according to Engel and Schmale (66), was called “conservation withdrawal.” It appears that if untreated, some patients with MDD develop melancholic or catatonic features (the prototype of metabolic depression in MDD) or a constant state of agitation possibly dependent on their temperament (genetics), personality features, and environmental stressors. The symptoms of diminished ability to think are secondary to lack of energy, and the depressed mood, feelings of worthlessness, hopelessness, or excessive guilt appear to be secondary to the negative reevaluation of self, rumination of past actions perceived as negative due to the lack of energy and strength and to weakness, for which patients blame themselves, as often, no medical explanation for their condition is possible. The symptoms of suicidal phenomena (suicidal ideation, suicide threats/attempts, and suicide death) have been considered to be plans and actions of the person asking for help, trying to escape from or terminating the unbearable condition created by the core symptoms associated with hypometabolism. The findings of cAMP level changes in response to treatment of humans with MDD with antidepressants (12–14) and of changes in the levels of cAMP in different states (pre-hibernation, hibernation, and active) in CBBs suggest the state-dependent quality of cAMP, as was reported previously (5), and are similar to the other neurobiological changes associated with MDD in humans and with hibernation in mammals investigated to date (21). The decreased cAMP levels in CBBs during hibernation and in patients with MDD suggest similarities in the cAMP levels in the two conditions and can be added to the observations of many neurobiological changes that are similar in the two conditions (21). These findings do not support the theory of cAMP dysfunction in MDD in humans that has been proposed by Fujita et al. (11, 14).
Our study has the following limitations: the small total number of CBBs from which specimens were obtained, although the adequate number of serial specimens obtained compensated for this; the sample comprising entirely female CBBs, two-thirds of which were pregnant; the captivity status; the few specimens obtained for determination of cAMP levels during the active state after the CBBs’ exit from hibernation; and that cAMP levels were analyzed for lysed leukocytes during hibernation and the states before it and after exit from it in CBBs, which was different from the methods used to determine cAMP levels in lymphoblasts, lymphocytes, and leukocytes in only one specimen obtained from patients with MDD versus euthymic patients or controls. However, the longitudinal nature of the data collected and the analysis and comparison of both cAMP and cortisol levels in this study are unusual if not unique, offering insight into both the entry into, and the emergence from, a state resembling, in some ways, MDD in humans. Also, this is the first study to document cAMP level changes in the leukocytes of black bears during active and hibernation states, revealing their similarities with the cAMP level changes reported in patients with MDD versus controls, and confirming the state-dependent changes of cAMP.
In conclusion, this study is the first to document changes in cAMP levels during mammalian hibernation and has established that the downregulation of cAMP levels is similar during hibernation of female CBBs and in patients with MDD; that there is a significant increase in cAMP levels with the entrance to the pre-hibernation state during captivity in the month of November, which corresponds to the month of entrance into hibernation proper of black bears in the wild, whereas the levels of serum cortisol are low (opposite direction); and that there is a mild increase in cAMP levels during the exit from hibernation (late March), whereas the serum cortisol levels are high, as they have been during hibernation proper. Finally, this study strongly suggests that the changes in cAMP levels in CBBs are state-dependent, as are most of the similar neurobiological findings associated with MDD in humans and mammalian hibernation. These findings dispute the theory of cAMP dysfunction as a cause of MDD, as was recently suggested (11, 14).
In-depth studies of the biological process of mammalian hibernation are under way (24, 25, 64, 67). Research on the cause of hypometabolism in MDD in humans has recently questioned the mitochondrial dysfunction theory of MDD and has suggested instead that suppression of mitochondrial function, which leads to the low ATP turnover observed in MDD, is the cause of hypometabolism in MDD (17–20). The findings of downregulation of cAMP levels during hibernation in female CBBs must be replicated in lysed leukocytes and other cells obtained through serial specimens from black bears (male and female) and from other hibernating mammals in the wild and in captivity, and from patients with recurrent MDD in different states of severity and in euthymic states. Confirmation of our findings, together with the previous findings of low ATP turnover in MDD (17–20), would offer more supportive evidence of the theory that a form of metabolic depression that is homologous to the one responsible for mammalian hibernation is the underlying adaptive process responsible for MDD in humans (21).
In spite of vigorous research, a full understanding of the etiology and neurobiology of MDD has been elusive to date (68), and no explanatory theory exists for most of the abnormal findings that have been proven to date to be state-dependent. The similarities in the findings of research regarding suppression of mitochondrial metabolism and neurobiological changes between human MDD and mammalian hibernation call for a paradigm shift in MDD research.
This new paradigm must seriously consider that the core symptoms of MDD are secondary to hypometabolism, a form of metabolic depression (21) homologous to one that is responsible for mammalian hibernation and also for torpor and estivation in many organisms (27, 64, 69, 70). It is hoped that this paradigm will inspire new basic research into the process of entering into and exiting from the state of hypometabolism in both MDD and mammalian hibernation and an extensive collaboration between researchers involved in the study of hypometabolism in these conditions. Understanding and viewing MDD as an old adaptive process that is activated in about 20% of humans under different adverse conditions (71) could open many new pathways for research into the etiology and neurobiology of MDD. We propose again [as previously (21)] that the process responsible for the documented hypometabolism in mammalian hibernation—suppression of mitochondrial function—is partially or fully activated in 20% of humans in their lifetime (71), leading to MDD, especially in humans exposed to environmental stressors (72, 73) and predisposed to MDD by behavior inhibition by temperament (74), anxiety disorders (75), and early life traumatic experience (76).
If future research fully confirms the theory that a form of hypometabolism that is homologous to the one responsible for mammalian hibernation is the underlying old adaptive process responsible for human MDD, the stigma associated with MDD could be reduced, and our diagnostic and treatment strategies for MDD could be improved.
Data availability statement
The raw data supporting the conclusions of this article will be made available by the authors, without undue reservation.
Ethics statement
The animal study was reviewed and approved by the Institutional Animal Care and Use Committee, New York State Institute for Basic Research in Developmental Disabilities, Staten Island, NY, United States, and the Animal Care Committee, Virginia Polytechnic Institute, Blacksburg, VA, United States.
Author contributions
JAT conceived and designed the study, obtained the blood and serum specimens, oversaw the execution of the study, and wrote and revised the first draft of the manuscript. MF analyzed the data and wrote the results section. Both authors approved the final manuscript.
Funding
This study was supported by the New York State Office for People With Developmental Disabilities.
Acknowledgments
The authors thank the late Michael R. Vaughan and Colleen Olfenbuttel, of the Center for Ursid Research at Virginia Polytechnic Institute and State University, Blacksburg, VA, for care of the black bears in the study and for preparation of specimens and data, respectively, and Warren Spivack and Maureen Marlow, of the New York State Institute for Basic Research in Developmental Disabilities, Staten Island, NY, for analysis of specimens and editorial assistance, respectively. Data from this study were presented at the World Psychiatric Association Congress, March 12–15, 2005, in Athens, Greece, and April 1–4, 2009, in Florence, Italy.
Conflict of interest
The authors declare that the research was conducted in the absence of any commercial or financial relationships that could be construed as a potential conflict of interest.
Publisher’s note
All claims expressed in this article are solely those of the authors and do not necessarily represent those of their affiliated organizations, or those of the publisher, the editors and the reviewers. Any product that may be evaluated in this article, or claim that may be made by its manufacturer, is not guaranteed or endorsed by the publisher.
References
1. Dwivedi Y, Pandey GN. Adenylyl cyclase-cyclicAMP signaling in mood disorders: role of the crucial phosphorylating enzyme protein kinase A. Neuropsychiatr Dis Treat. (2008) 4:161–76. doi: 10.2147/ndt.s2380
2. Maj M, Ariano MG, Arena F, Kemali D. Plasma cortisol, catecholamine and cyclic AMP levels, response to dexamethasone suppression test and platelet MAO activity in manic-depressive patients. A longitudinal study. Neuropsychobiology. (1984) 11:168–73. doi: 10.1159/000118071
3. Kay G, Sargeant M, McGuffin P, Whatley S, Marchbanks R, Baldwin D, et al. The lymphoblast beta-adrenergic receptor in bipolar depressed patients: characterization and down-regulation. J Affect Disord. (1993) 27:163–72. doi: 10.1016/0165-0327(93)90004-4
4. Berrettini WH, Cappellari CB, Nurnberger JI, Gershon ES. Beta-adrenergic receptors on lymphoblasts. A study of manic-depressive illness. Neuropsychobiology. (1987) 17:15–8. doi: 10.1159/000118334
5. Klysner R, Geisler A, Rosenberg R. Enhanced histamine–and beta-adrenoceptor-mediated cyclic AMP formation in leukocytes from patients with endogenous depression. J Affect Disord. (1987) 13:227–32. doi: 10.1016/0165-0327(87)90041-3
6. Pandey GN, Dysken MW, Garver DL, Davis JM. Beta-adrenergic receptor function in affective illness. Am J Psychiatry. (1979) 136:675–8. doi: 10.1176/ajp.136.5.675
7. Ebstein RP, Lerer B, Shapira B, Shemesh Z, Moscovich DG, Kindler S. Cyclic AMP second-messenger signal amplification in depression. Br J Psychiatry. (1988) 152:665–9. doi: 10.1192/bjp.152.5.665
8. Mann JJ, Brown RP, Halper JP, Sweeney JA, Kocsis JH, Stokes PE, et al. Reduced sensitivity of lymphocyte beta-adrenergic receptors in patients with endogenous depression and psychomotor agitation. N Engl J Med. (1985) 313:715–20. doi: 10.1056/NEJM198509193131202
9. Mizrahi C, Stojanovic A, Urbina M, Carreira I, Lima L. Differential cAMP levels and serotonin effects in blood peripheral mononuclear cells and lymphocytes from major depression patients. Int Immunopharmacol. (2004) 4:1125–33. doi: 10.1016/j.intimp.2004.05.001
10. Shelton RC, Manier DH, Peterson CS, Ellis TC, Sulser F. Cyclic AMP-dependent protein kinase in subtypes of major depression and normal volunteers. Int J Neuropsychopharmacol. (1999) 2:187–92. doi: 10.1017/S1461145799001509
11. Fujita M, Hines CS, Zoghbi SS, Mallinger AG, Dickstein LP, Liow JS, et al. Downregulation of brain phosphodiesterase type IV measured with 11C-(R)-rolipram positron emission tomography in major depressive disorder. Biol Psychiatry. (2012) 72:548–54. doi: 10.1016/j.biopsych.2012.04.030
12. Dowlatshahi D, MacQueen GM, Wang JF, Young LT. Increased temporal cortex CREB concentrations and antidepressant treatment in major depression. Lancet. (1998) 352:1754–5. doi: 10.1016/S0140-6736(05)79827-5
13. Duman RS. Structural alterations in depression: cellular mechanisms underlying pathology and treatment of mood disorders. CNS Spectr. (2002) 7:140–2. doi: 10.1017/S1092852900017454
14. Fujita M, Richards EM, Niciu MJ, Ionescu DF, Zoghbi SS, Hong J, et al. cAMP signaling in brain is decreased in unmedicated depressed patients and increased by treatment with a selective serotonin reuptake inhibitor. Mol Psychiatry. (2017) 22:754–9. doi: 10.1038/mp.2016.171
15. Kato T, Kato N. Mitochondrial dysfunction in bipolar disorder. Bipolar Disord. (2000) 2(Pt 1):180–90.
16. Modica-Napolitano JS, Renshaw PF. Ethanolamine and phosphoethanolamine inhibit mitochondrial function in vitro: implications for mitochondrial dysfunction hypothesis in depression and bipolar disorder. Biol Psychiatry. (2004) 55:273–7. doi: 10.1016/s0006-3223(03)00784-4
17. Martins-de-Souza D, Guest PC, Harris LW, Vanattou-Saifoudine N, Webster MJ, Rahmoune H, et al. Identification of proteomic signatures associated with depression and psychotic depression in post-mortem brains from major depression patients. Transl Psychiatry. (2012) 2:e87. doi: 10.1038/tp.2012.13
18. Karabatsiakis A, Böck C, Salinas-Manrique J, Kolassa S, Calzia E, Dietrich DE, et al. Mitochondrial respiration in peripheral blood mononuclear cells correlates with depressive subsymptoms and severity of major depression. Transl Psychiatry. (2014) 4:e397. doi: 10.1038/tp.2014.44
19. Allen J, Romay-Tallon R, Brymer KJ, Caruncho HJ, Kalynchuk LE. Mitochondria and mood: mitochondrial dysfunction as a key player in the manifestation of depression. Front Neurosci. (2018) 12:386. doi: 10.3389/fnins.2018.00386
20. Allen J, Caruncho HJ, Kalynchuk LE. Severe life stress, mitochondrial dysfunction, and depressive behavior: a pathophysiological and therapeutic perspective. Mitochondrion. (2021) 56:111–7. doi: 10.1016/j.mito.2020.11.010
21. Tsiouris JA. Metabolic depression in hibernation and major depression: an explanatory theory and an animal model of depression. Med Hypotheses. (2005) 65:829–40.
22. Manji HK, Drevets WC, Charney DS. The cellular neurobiology of depression. Nat Med. (2001) 7:541–7. doi: 10.1038/87865
23. Nestler EJ, Barrot M, DiLeone RJ, Eisch AJ, Gold SJ, Monteggia LM. Neurobiology of depression. Neuron. (2002) 34:13–25. doi: 10.1016/S0896-6273(02)00653-0
24. Staples JF. Metabolic suppression in mammalian hibernation: the role of mitochondria. J Exp Biol. (2014) 217(Pt 12):2032–6. doi: 10.1242/jeb.092973
25. Mathers KE, Staples JF. Differential posttranslational modification of mitochondrial enzymes corresponds with metabolic suppression during hibernation. Am J Physiol Regul Integr Comp Physiol. (2019) 317:R262–9. doi: 10.1152/ajpregu.00052.2019
26. English TE, Storey KB. Enzymes of adenylate metabolism and their role in hibernation of the white-tailed prairie dog, Cynomys leucurus. Arch Biochem Biophys. (2000) 376:91–100. doi: 10.1006/abbi.1999.1686
27. Heldmaier G, Ortmann S, Elvert R. Natural hypometabolism during hibernation and daily torpor in mammals. Respir Physiol Neurobiol. (2004) 141:317–29. doi: 10.1016/j.resp.2004.03.014
28. Wang SY, Shin SJ. Alterations in thyroid function tests in major depression. Taiwan Yi Xue Hui Za Zhi. (1989) 88:143–7.
29. Tomasi TE, Hellgren EC, Tucker TJ. Thyroid hormone concentrations in black bears (Ursus americanus): hibernation and pregnancy effects. Gen Comp Endocrinol. (1998) 109:192–9. doi: 10.1006/gcen.1997.7018
30. Gibbons JL, McHugh PR. Plasma cortisol in depressive illness. J Psychiatr Res. (1962) 1:162–71. doi: 10.1016/0022-3956(62)90006-7
31. Carpenter WT, Bunney WE. Adrenal cortical activity in depressive illness. Am J Psychiatry. (1971) 128:31–40. doi: 10.1176/ajp.128.1.31
32. Harlow H, Beck TD, Walters LM, Greenhouse SS. Seasonal serum glucose, progesterone, and cortisol levels of black bears (Ursus americanus). Can J Zool. (1990) 68:183–7. doi: 10.1139/z90-025
33. Pitharouli MC, Hagenaars SP, Glanville KP, Coleman JRI, Hotopf M, Lewis CM, et al. Elevated C-reactive protein in patients with depression, independent of genetic, health, and psychosocial factors: results from the UK Biobank. Am J Psychiatry. (2021) 178:522–9 doi: 10.1176/appi.ajp.2020.20060947
34. Dowlati Y, Herrmann N, Swardfager W, Liu H, Sham L, Reim EK, et al. A meta-analysis of cytokines in major depression. Biol Psychiatry. (2010) 67:446–57. doi: 10.1016/j.biopsych.2009.09.033
35. Maes M, Scharpe S, Bosmans E, Vandewoude M, Suy E, Uyttenbroeck W, et al. Disturbances in acute phase plasma proteins during melancholia: additional evidence for the presence of an inflammatory process during that illness. Prog Neuropsychopharmacol Biol Psychiatry. (1992) 16:501–15. doi: 10.1016/0278-5846(92)90056-k
36. Sheikh AM, Chauhan V, Tsiouris JA, Mehta PD, Burguess K, Fenko MD, et al. Elevated levels of serum alpha(2) macroglobulin in wild black bears during hibernation. Biochimie. (2003) 85:1027–32. doi: 10.1016/s0300-9084(03)00133-0
37. Tsiouris JA, Chauhan VPS, Chauhan A, Malik M, Vaughan V. Similarities in acute phase protein response during hibernation in black bears and major depression in humans: a response to underlying metabolic depression. Can J Zool. (2004) 82:1468–76.
38. Srere HK, Belke D, Wang LC, Martin SL. alpha 2-Macroglobulin gene expression during hibernation in ground squirrels is independent of acute phase response. Am J Physiol. (1995) 268:R1507–12. doi: 10.1152/ajpregu.1995.268.6.R1507
39. Lambert G, Johansson M, Agren H, Friberg P. Reduced brain norepinephrine and dopamine release in treatment-refractory depressive illness: evidence in support of the catecholamine hypothesis of mood disorders. Arch Gen Psychiatry. (2000) 57:787–93. doi: 10.1001/archpsyc.57.8.787
40. Hock RJ. Metabolic rates and rectal temperatures of active and “hibernating” black bears. Fed Proc. (1957) 16:440.
41. Nelson RA, Wahner HW, Jones JD, Ellefson RD, Zollman PE. Metabolism of bears before, during, and after winter sleep. Am J Physiol. (1973) 224:491–6. doi: 10.1152/ajplegacy.1973.224.2.491
42. Boyer BB, Barnes BM. Molecular and metabolic aspects of mammalian hibernation. Bioscience. (1999) 49:713–24. doi: 10.2307/1313595
43. Sarandol A, Sarandol E, Eker SS, Erdinc S, Vatansever E, Kirli S. Major depressive disorder is accompanied with oxidative stress: short-term antidepressant treatment does not alter oxidative-antioxidative systems. Hum Psychopharmacol. (2007) 22:67–73. doi: 10.1002/hup.829
44. Lindqvist D, Dhabhar FS, James SJ, Hough CM, Jain FA, Bersani FS, et al. Oxidative stress, inflammation and treatment response in major depression. Psychoneuroendocrinology. (2017) 76:197–205. doi: 10.1016/j.psyneuen.2016.11.031
45. Chauhan VP, Tsiouris JA, Chauhan A, Sheikh AM, Brown WT, Vaughan M. Increased oxidative stress and decreased activities of Ca(2+)/Mg(2+)-ATPase and Na(+)/K(+)-ATPase in the red blood cells of the hibernating black bear. Life Sci. (2002) 71:153–61. doi: 10.1016/s0024-3205(02)01619-3
46. Schildkraut JJ, Orsulak PJ, LaBrie RA, Schatzberg AF, Gudeman JE, Cole JO, et al. Toward a biochemical classification of depressive disorders. II. Application of multivariate discriminant function analysis to data on urinary catecholamines and metabolites. Arch Gen Psychiatry. (1978) 35:1436–9. doi: 10.1001/archpsyc.1978.01770360040004
47. Draskóczy PR, Lyman CP. Turnover of catecholamines in active and hibernating ground squirrels. J Pharmacol Exp Ther. (1967) 155:101–11.
48. Frerichs KU, Smith CB, Brenner M, DeGracia DJ, Krause GS, Marrone L, et al. Suppression of protein synthesis in brain during hibernation involves inhibition of protein initiation and elongation. Proc Natl Acad Sci USA. (1998) 95:14511–6. doi: 10.1073/pnas.95.24.14511
49. Bennaroch EE. Adenosine and its receptors: multiple modulatory functions and potential therapeutic targets for neurologic disease. Neurology. (2008) 70:231–6. doi: 10.1212/01.wnl.0000297939.18236.ec
50. Donahue SW, Vaughan MR, Demers LM, Donahue HJ. Serum markers of bone metabolism show bone loss in hibernating bears. Clin Orthop Relat Res. (2003) 408:295–301. doi: 10.1097/00003086-200303000-00040
51. Lowry OH, Rosebrough NJ, Farr AL, Randall RJ. Protein measurement with the Folin phenol reagent. J Biol Chem. (1951) 193:265–75. doi: 10.1016/S0021-9258(19)52451-6
52. Bland JM, Altman DG. Calculating correlation coefficients with repeated observations: part 1–correlation within subjects. BMJ. (1995) 310:446. doi: 10.1136/bmj.310.6977.446
53. Linden A. RMCORR: Stata Module to Compute a Correlation for Data with Repeated Measures. Statistical Software Components S458971. Chestnut Hill, MA: Boston College Department of Economics (2021).
54. Liang K-Y, Zeger SL. Longitudinal data analysis using generalized linear models. Biometrika. (1986) 73:13–22. doi: 10.1093/biomet/73.1.13
55. Zeger SL, Liang KY. Longitudinal data analysis for discrete and continuous outcomes. Biometrics. (1986) 42:121–30. doi: 10.2307/2531248
57. Hellgren EC, Rogers LL, Seal US. Serum chemistry and hematology of black bears: physiological indices of habitat quality or seasonal patterns. J Mammal. (1993) 74:304–15.
58. Romero LM, Meister CJ, Cyr NE, Kenagy GJ, Wingfield JC. Seasonal glucocorticoid responses to capture in wild free-living mammals. Am J Physiol Regul Integr Comp Physiol. (2008) 294:R614–22. doi: 10.1152/ajpregu.00752.2007
60. Kanof PD, Coccaro EF, Johns CA, Davidson M, Siever LJ, Davis KL. Cyclic-AMP production by polymorphonuclear leukocytes in psychiatric disorders. Biol Psychiatry. (1989) 25:413–20. doi: 10.1016/0006-3223(89)90194-7
61. Doherty JC, Kronon MT, Rotermund AJ. The effects of short term cold storage upon ATP and 2,3-BPG levels in the blood of euthermic and hibernating thirteen-lined ground squirrels Spermophilus tridecemlineatus. Comp Biochem Physiol Comp Physiol. (1993) 104:87–91. doi: 10.1016/0300-9629(93)90013-t
62. Koschinski A, Zaccolo M. Activation of PKA in cell requires higher concentration of cAMP than in vitro: implications for compartmentalization of cAMP signalling. Sci Rep. (2017) 7:14090. doi: 10.1038/s41598-017-13021-y
63. Zhang J, Kaasik K, Blackburn MR, Lee CC. Constant darkness is a circadian metabolic signal in mammals. Nature. (2006) 439:340–3. doi: 10.1038/nature04368
64. Zhao Z, Van Oort A, Tao Z, O’Brien WG, Lee CC. Metabolite profiling of 5’-AMP-induced hypometabolism. Metabolomics. (2014) 10:63–76. doi: 10.1007/s11306-013-0552-7
65. American Psychiatric Association [APA]. Diagnostic and Statistical Manual of Mental Disorders (DSM-5). 5th ed. Washington, DC: American Psychiatric Association [APA] (2013). doi: 10.1176/appi.books.9780890425596
66. Engel GL, Schmale AH. Conservation withdrawal: a primary regulatory process for organismic homeostasis. CIBA Found Symp. (1972) 8:57–85. doi: 10.1002/9780470719916.ch5
67. Chazarin B, Storey KB, Ziemianin A, Chanon S, Plumel M, Chery I, et al. Metabolic reprogramming involving glycolysis in the hibernating brown bear skeletal muscle. Front Zool. (2019) 16:12. doi: 10.1186/s12983-019-0312-2
68. Nemeroff CB. The state of our understanding of the pathophysiology and optimal treatment of depression: glass half full or half empty? Am J Psychiatry. (2020) 177:671–85. doi: 10.1176/appi.ajp.2020.20060845
69. Lyman CP, Willis JS, Malan A, Wang LCH. Hibernation and Torpor in Mammals and Birds. New York, NY: Academic Press (1982). doi: 10.1016/B978-0-12-460420-9.50008-8
70. Geiser F. Reduction of metabolism during hibernation and daily torpor in mammals and birds: temperature effect or physiological inhibition? J Comp Physiol B. (1988) 158:25–37. doi: 10.1007/BF00692726
71. Kessler RC, McGonagle KA, Zhao S, Nelson CB, Hughes M, Eshleman S, et al. Lifetime and 12-month prevalence of DSM-III-R psychiatric disorders in the United States: results from the national comorbidity survey. Arch Gen Psychiatry. (1994) 51:8–19. doi: 10.1001/archpsyc.1994.03950010008002
72. Paykel ES, Myers JK, Dienelt MN, Klerman GL, Lindenthal JJ, Pepper MP. Life events and depression. A controlled study. Arch Gen Psychiatry. (1969) 21:753–60. doi: 10.1001/archpsyc.1969.01740240113014
73. Kessler RC. The effects of stressful life events on depression. Annu Rev Psychol. (1997) 48:191–214. doi: 10.1146/annurev.psych.48.1.191
74. Kagan J, Snidman N. Early childhood predictors of adult anxiety disorders. Biol Psychiatry. (1999) 46:1536–41. doi: 10.1016/S0006-3223(99)00137-7
75. Kendler KS, Kuhn J, Prescott CA. The interrelationship of neuroticism, sex, and stressful life events in the prediction of episodes of major depression. Am J Psychiatry. (2004) 161:631–6. doi: 10.1176/appi.ajp.161.4.631
Keywords: cAMP downregulation, leukocytes, hypometabolism, mammalian hibernation, black bears, major depressive disorder
Citation: Tsiouris JA and Flory M (2023) Downregulation of cyclic adenosine monophosphate levels in leukocytes of hibernating captive black bears is similar to reported cyclic adenosine monophosphate findings in major depressive disorder. Front. Psychiatry 14:1123279. doi: 10.3389/fpsyt.2023.1123279
Received: 13 December 2022; Accepted: 24 February 2023;
Published: 16 March 2023.
Edited by:
Çiçek Hocaoğlu, Recep Tayyip Erdoğan University, TürkiyeReviewed by:
Gözde Bacik Yaman, Süleyman Demirel University, TürkiyeXiangyang Xu, Huazhong University of Science and Technology, China
Copyright © 2023 Tsiouris and Flory. This is an open-access article distributed under the terms of the Creative Commons Attribution License (CC BY). The use, distribution or reproduction in other forums is permitted, provided the original author(s) and the copyright owner(s) are credited and that the original publication in this journal is cited, in accordance with accepted academic practice. No use, distribution or reproduction is permitted which does not comply with these terms.
*Correspondence: John A. Tsiouris, am9obi50c2lvdXJpc0BvcHdkZC5ueS5nb3Y=