- 1Faculty of Medicine, Institute of Molecular Biomedicine, Comenius University in Bratislava, Bratislava, Slovakia
- 2First Faculty of Medicine, Institute of Physiology, Charles University, Prague, Czechia
- 3Department of Psychology, University of Wisconsin-Milwaukee, Milwaukee, WI, United States
Anxiety disorders are one of the most prevalent mood disorders that can lead to impaired quality of life. Current treatment of anxiety disorders has various adverse effects, safety concerns, or restricted efficacy; therefore, novel therapeutic targets need to be studied. Sex steroid hormones (SSHs) play a crucial role in the formation of brain structures, including regions of the limbic system and prefrontal cortex during perinatal development. In the brain, SSHs have activational and organizational effects mediated by either intracellular or transmembrane G-protein coupled receptors. During perinatal developmental periods, the physiological concentrations of SSHs lead to the normal development of the brain; however, the early hormonal dysregulation could result in various anxiety diorders later in life. Sex differences in the prevalence of anxiety disorders suggest that SSHs might be implicated in their development. In this review, we discuss preclinical and clinical studies regarding the role of dysregulated SSHs signaling during early brain development that modifies the risk for anxiety disorders in a sex-specific manner in adulthood. Moreover, our aim is to summarize potential molecular mechanisms by which the SSHs may affect anxiety disorders in preclinical research. Finally, the potential effects of SSHs in the treatment of anxiety disorders are discussed.
Introduction
As specified in the newest (5th) edition of the Diagnostic and Statistical Manual of Mental Disorders (DSM-5), anxiety disorders belong to the category of affective disorders, also known as mood disorders (1), which represent a worldwide health problem (2–4). The global overall prevalence of anxiety disorders is 3.6% with as much as 4.6% among women and 2.6% among men, with differences between age groups 18 and over (3, 5). The most widespread treatment for anxiety disorders and their related disorders, such as panic attacks or phobia-associated disorders, is a combination of psychotherapy—cognitive behavioral therapy, and pharmacotherapy including the use of benzodiazepines (6), serotonin reuptake inhibitors—SSRI, serotonin-norepinephrine reuptake inhibitors—SNRI (4), and tricyclic antidepressants (7). Although the medications are considered relatively safe, there are several negative side effects (8–11). Therefore, the importance of research focusing on studying novel pharmacological approaches is rising, and one of the considered candidates appear to be the sex steroid hormones (SSHs).
Sex steroid hormones, including progesterone, androgens, and estrogens, shape the brain during important prenatal and perinatal periods of development. This is also known as “organizational windows,” when hormones interact with immature neuronal cells. During these periods, SSHs may cause permanent changes in the structure and function of the brain, resulting in sex-specific differences in cognition, such as masculinization of the nervous system, and behavior (12, 13). The main processes participating in the sexual differentiation of the brain include cell birth, cell death, cell migration, and cell differentiation (14). During the postnatal period, another “organizational window” appears—puberty and adolescence—a point at which SSHs may further shape brain development in a way that has long-term effects on behavior in adulthood (15, 16). Masculinization of brain structures starts in early development and ends in early adulthood (17, 18). In rodents, prenatal estrogen exposure leads to defeminization and masculinization of the brain and behavior via estrogen receptors (ERs), with a protective effect of estrogen binding protein—α-fetoprotein, against estrogens in females (17). On the other hand, in non-human primates and humans, the masculinization and defeminization of genitalia and behavior are established by testosterone (T) metabolite—dihydrotestosterone (DHT) (18). Early androgen signaling via androgen receptor (AR) is important for genital masculinization but does not alter juvenile or adult behavior in non-human male primates. An important period for organizational effects of SSHs on the brain structures during prenatal development appears to be the last part of gestation, which is the period significant for synaptogenesis as well (18). Nevertheless, cellular mechanisms facilitating SSHs actions are particularly dissimilar within and between brain regions (19). SSHs are just one of the factors in these highly complex processes, with many variations at different timespoints along the brain development. The final phenotypic outcome thus can vary greatly (19).
In addition to organizational effects, SSHs have activational effects on brain structures, physiology, and behavior, all depending on fluctuations of SSH concentrations during diurnal, circadian, circatrigintan, or circannual cycles. Activational effects of SSHs are acute and reversible, regulating transient physiological and behavioral responses in adults (12, 13). They generally appear post-puberty and act independently or in combination with organizational effects (15). Therefore, both organizational and activational effects of SSHs on the brain could affect behavioral outcomes later in life, particularly in the development of anxiety.
To exert these effects, SSHs must activate the signaling cascade via binding to an appropriate receptor, either intracellular, membrane-associated, or transmembrane. The actions of androgens and estrogens can act via classical, slow intracellular AR and ERs, or through non-classical membrane-associated AR/ERs, as well as transmembrane G-protein coupled androgen—zinc transporter protein 9 (ZIP9) and estrogen G-protein-coupled estrogen receptor 1 (GPER1) receptors. Slow, classical processes, which last usually several hours, are mediating the transcription of specific genes in the nucleus. On the other hand, fast, non-classical signaling stimulates various extranuclear downstream cascades regulating different cellular responses, such as DNA synthesis, cell proliferation, migration, or survival (20). The dysregulation of physiological concentrations of SSHs during prenatal and early postnatal development may influence the formation of brain structures and lead to various affective, neurodevelopmental, or neurodegenerative disorders (12, 13, 21, 22). The mean onset of anxiety disorder symptoms starts between late adolescence and early adulthood (23). The frequency and risk of mood, neurological, and psychiatric disorders increase with aging along with a decline of SSHs that occurs in both women and men (24). However, there are significant sex differences in their prevalence. Women are twice as likely to suffer from anxiety disorders when compared to men. The higher prevalence may be related to fluctuations of SSHs during different life periods, such as puberty, pre-menstruum, pregnancy, postpartum, and menopause (25). Moreover, it has long been hypothesized that women are more prone to experience chronic stress and to engage in rumination than males (26). In addition, sex chromosome complement (XX vs. XY) can also be a contributing factor. Expression of sex-determining region Y (Sry) gene on the male Y chromosome is responsible for testes differentiation and therefore perinatal T secretion. On the other hand, the absence of the Sry gene in the XX genotype leads to the development of ovaries in females. In the recent review of Mir et al. (27), authors discussed the potential contribution of sex chromosome complement-biased sex differences in rodent models of anxiety-like behavior. Here, the X chromosome appears to affect the development of anxiety-like behavior. For more information, please see the review (27). Described associations suggest that SSHs may be contributing factors in the development and pathophysiology of affective disorders (26, 28, 29). Thus, the attention of this review has been focused on trying to understand the effects of SSHs on the brain and behavior in relation to anxiety in both animals (30–32) and humans (32–37).
Brain regions involved in anxiety disorders
As mentioned earlier, human brain development is influenced by the organizational and activational effects of SSHs in a sex-dependent manner (13, 16). Brain regions including the amygdala (AMY), hippocampus (HIP), prefrontal cortex (PFC), and hypothalamus (HYP) are implicated in emotional processing, stress response, memory, and cognition. The involvement of each can be sex specific. Dysregulation or damage to these structures may result in affective disorders (38). For this review, the article will concentrate on anxiety disorders.
The PFC is implicated in many cognitive abilities that involve socio-emotional and executive functions (39, 40). The ability to plan complex responses, decision-making, attention and memory, speech and language development (41, 42), mental flexibility, attention, self-control, and self-regulation (43) belong to such executive functions. In addition, a reciprocal connection between the PFC and AMY (basolateral) suggests that PFC may be partially implicated in the regulation of anxiety disorder, and impairment of these neural circuits and functional connectivity during development or in adulthood may contribute to the development of anxiety and/or fear in both male and female rodents and non-human primates (44).
The HIP is predominantly involved in learning and memory, but it also plays a role in spatial navigation, emotions, and the regulation of function in the HYP (45). Structural and functional neuroimaging studies have further revealed the significant involvement of the HIP in the pathophysiology of anxiety (46, 47). The dorsal part of the HIP is mostly involved in spatial memory consolidation, whereas the ventral portion of the HIP, which is connected to AMY is mostly involved in emotional memory (46–48). Bannerman et al. (48) showed that lesions in ventral HIP decrease anxiety in light/dark exploration and hyponeophagia tests and do not affect the spatial memory of male rats in elevated T-maze and water maze (48). Moreover, ventral HIP lesions reduce conditioned freezing, thus, not only AMY but also ventral HIP plays a role in contextual fear conditioning, which is a test, related to anxiety-like behavior in rodents (49).
The AMY plays an important role in processing fearful and threatening stimuli, regulation of emotional responsiveness, facial perception (50), as well as emotional learning and memory for example in reaction to stressful or fear stimuli (51–53). Due to its functions, the AMY is associated with anxiety disorders in animals (54) and humans (51). Finally, the HYP is a part of dominant neuroendocrine systems, such as hypothalamus-pituitary-adrenal (HPA) or hypothalamus-pituitary-gonadal (HPG) axes. Both the HPA and HPG axes are implicated not only in the regulation of reproduction, but also in stress response, and both are related to the regulation of mood and mood disorders including anxiety (55–57).
Meta-analysis of neuroimaging studies also confirmed that the PFC, HIP, and AMY are implicated in anxiety disorders (58). Several studies proposed that the symptoms of anxiety may result in part from disrupted activity in the emotional or cognitive centers in the brain (59), such as the PFC (responsible for a higher cognitive and executive function) and parts of the limbic system, especially the HIP, HYP, and AMY where memory and emotion-processing brain regions are located (60–62). These brain regions express several types of SSHs receptors, such as intracellular/membrane-associated androgen, estrogen, or progesterone receptors (AR, ERs, and PRs, respectively), along with the transmembrane GPER1. Interestingly, expression of the transmembrane receptor for androgen—ZIP9 has also been detected in the brain although primarily found in the prostate (63–65).
Furthermore, the perinatal influence of androgens, estrogens, and progesterone on the PFC, HIP, HYP, and AMY in the context of anxiety disorder development will be discussed. Secondly, the molecular mechanisms and potential treatment of anxiety disorders by SSH for adults will be debated.
Anxiety disorders
According to the DSM-5, anxiety disorders include several conditions that share common features, such as excessive fear and anxiety, generalized anxiety disorder, panic disorder, separation anxiety disorder, selective mutism, medication-induced anxiety disorder, and different phobia-related disorders (social and agoraphobia) (66, 67). Normal or moderate amounts of anxiety naturally occur in a healthy individual.
It has been previously reported that people can use anxiety for self-motivation (so-called anxiety motivation) (68). In addition, Wirtz et al. (69) have shown that anxiety operates on preparedness behavior, and in turn, it can affect precautionary behavior, both positively and negatively (69). On the other hand, pathological anxiety is characterized by excessive, not adequate anxiety response occurring in situations that would not elicit anxiety in healthy people (70). Pathological anxiety is defined by different features, including excessive worry, physiological arousal, and avoidance behavior (70). Patients suffering from pathological anxiety display affective symptoms, including nervousness, frustration, impatience, and fearfulness (71). Cognitively, it is characterized by hypervigilance for threat, poor concentration, and impaired memory (70, 71). Behaviorally, patients with pathological anxiety display higher readiness to respond to danger, restlessness, and agitation (71).
Preclinical research
Laboratory rodents, such as mice and rats, are by far the most widely used animals in preclinical anxiety research. Behavioral tests such as open field, novelty suppressed feeding, elevated plus maze, light/dark box, and stress-induced hyperthermia, are widely used to identify anxiety-like behavior (72) and to study the effect of anti-anxiety medication/mechanisms.
Prenatal effects of androgen exposure may mirror the organizational effect of T on the development of brain structures (12, 13). During the prenatal development of rodents, androgen excess (hyperandrogenism), present in mothers with polycystic ovary syndrome (PCOS), may result in a higher risk of anxiety-like behaviors in offspring (22). Female offspring of dams with PCOS showed significantly higher levels of anxiety-like behavior using open field and elevated plus maze in comparison to male offspring (21, 73, 74). In another study in which PCOS was induced by administration of DHT prenatally in dams, female offspring in adulthood showed higher anxiety-like behavior in the elevated plus maze and open-field test when compared to male counterparts. The female offspring had up-regulated genes related to anxiety-like behavior, such as adrenoceptor-α-1B (noradrenergic excitatory input) and corticotropin-releasing hormone (Crh) receptor 2 (mediator of stress-related behavior) in the AMY (73). Moreover, adult female offspring of dams with a PCOS phenotype had upregulated Crh and downregulated its Crh receptor 1 in the HYP. In adult male offspring, the expression of Crh was higher only in a group with a high-fat, high-sucrose diet for dams, but not in the PCOS group, thus suggesting sex-dependent mechanisms behind the onset of anxiety-like behavior based on high-androgen excess (73).
The higher prenatal concentration of androgens may also affect the expression of genes, such as brain-derived neurotrophic factor (BDNF) in the brain, especially in females. BDNF and its high-affinity receptor—tropomyosin receptor kinase B (TrkB) in the brain are responsible for the development, differentiation, and survival of neurons, growth of neurites, synaptic plasticity, synthesis of differentiating factors, and homeostasis. They are also involved in responses to social stress and modulate affective-related behaviors (75–78). The western blot analysis showed increased BDNF in HIP and cortex of anxious offspring exposed to higher prenatal androgen concentrations compared to control female offspring of vehicle-treated Wistar rats (22). The increased BDNF concentrations may be an adaptive response of the brain to inhibitory neuron reduction caused by higher prenatal androgen concentrations (22). Experimental studies suggest a link between impaired hippocampal BDNF systems and anxiety disorders. High prenatal androgen excess may lead to dysregulation of neuropeptide Y, parvalbumin, and BDNF, leading to increased anxiety-like behavior. A potential mechanism for this increase may be elevated BDNF concentrations in the HIP and cortex and decreased neuropeptide Y and parvalbumin interneurons in the CA1 region of the HIP (22). Higher concentrations of BDNF in the HIP may be due to adaptive reactions of the brain to the prenatal androgen-induced reduction of hippocampal inhibitory neurons in female rats. It has been shown that inhibition of T aromatization in the brain during the prenatal period via the administration of aromatase inhibitor leads to a decrease in basal activity of the hypophyseal-adrenocortical system in male offspring (PND 10) and longer hormonal stress responses in both sexes of adult rats (79). Regarding the behavior of rats, prenatal hyperandrogenization resulted in higher anxiety in the open field and elevated plus maze test in both sexes of adult rats (79–81). Consequently, maternal hyperandrogenization alters the sex-dependent anxiety and the behavioral response to a novel environment in both male and female adult rats (81). In addition, Cheng et al. (82) have shown that a hyperandrogenic intrauterine environment induced by aromatase inhibitor—letrozole—resulted in higher anxiety-like behavior in adolescent rats of both sexes, and it was associated with decreased neurogenesis in the hippocampal dentate gyrus (82).
On the other hand, perinatal (during lactation) exposure to endogenous disruptors (having an anti-androgenic effect) led to higher anxiety-like behavior in elevated plus maze test in young males (postnatal day, PND 45 and 60); however, no changes in anxiety-like behavior of perinatal endogenous disruptor-exposed females were detected at the same age. In addition, in males perinatally exposed to endogenous disruptors, a lower serum concentration of T was found (PND 60). It has been suggested that the anti-androgenic action of endogenous disruptors could be one of the possible mechanisms underlying anxiogenic-like behavior produced by perinatal endogenous disruptor exposure in 60-day-old male rats (83). In contrast, Xu et al. (84) have shown that perinatal (GD 7-PND 21) exposure to endogenous disruptors results in higher anxiety-like behavior in the elevated plus maze and light/dark box in both pubertal males and females, suggesting that exposure to endogenous disruptors in the perinatal period and not just during lactation (as reported by Carbone et al. (83)) has anxiogenic effect in pubertal offspring regardless of sex (84). Furthermore, while in pubertal males, perinatal exposure to endogenous disruptors reduces expression of hippocampal AR, in pubertal females, lower expression of hippocampal ERβ was observed.
It has been shown that an insult, such as prenatal stress, during gestation can reduce the concentration of fetal testicular T in male offspring (85) and, in turn, it can induce a long-term imbalance in circulating T concentrations in males in adulthood (86). It has been demonstrated that prenatal stress leads to a reduction of ERα expression in PFC and HIP of adult male offspring (86, 87). Behaviorally, prenatal stress results in a robust anxiogenic response in adult male rats (88–90) or both sexes of rats (91, 92) and mice (93) in elevated plus maze test in comparison to prenatally non-stressed animals that might be related to reduction in GABA A (benzodiazepine) receptor levels in HIP and AMY observed in prenatally stressed animals (91). Assuming that stress induced during the gestation period leads to higher anxiety-like behavior in male adult offspring (88–90), prenatal impairment of T metabolism might also be related to the anxiogenic responses of male offspring in adulthood.
In conclusion, there is a sex-dependent response to high prenatal effects of androgens, with a higher risk of anxiety-like behavior in female offspring in adulthood caused by upregulation of genes associated with stress response in the AMY and HIP. Although estrogens and progesterone may also be involved, there is not yet enough literature on the effects of prenatal dysregulation of these hormones and/or their metabolites on the brain, and on cognitive or behavioral consequences later in life.
Clinical research
In humans, it is difficult to directly measure the T concentrations during prenatal development. The second-to-fourth digit ratio (2D:4D) is defined as the ratio of the lengths of the second digit (the “index” finger, 2D) and the fourth digit (the “ring” finger, 4D) of the same hand (94). In men, the 2D is shorter than 4D, while in women, 2D is the same length or longer than 4D. Thus, in females, in general, the 2D:4D is higher than in males (95). The 2D:4D is frequently used as an indirect and non-invasive marker of prenatal T exposure (95), as it has been shown that a lower 2D:4D is the result of higher intrauterine exposure to T, while a higher 2D:4D ratio reflects a lower exposure to T (i.e., higher estrogen exposure) in utero (96). Additionally, the fetal concentrations of luteinizing hormone, 17β-estradiol (E2), and prolactin are positively associated with the 2D:4D ratio (97, 98).
Several studies reported that males with feminine finger ratios score higher on a test for anxiety (99) in adulthood. The mechanism behind remains unknown, but it is suggested that high prenatal T with low 2D:4D positively correlates with CAG repeats (coding amino acid glutamine) for AR (100). In turn, the higher number of CAG repeats in AR is related to a less pronounced effect of T response and lower gene transcription. Subsequently, the anxiety level is positively associated with the length of CAG repeats in AR in men (101). Indeed, there are some contradictory results (102); however, at least a portion of the variation in psychopathology is believed to be due to the organizational effects of sex hormones. Thus, the 2D:4D might help to assess the relationship between prenatal androgen factors and a variety of behaviors, including anxiety.
Similar to animal studies, the effect of high prenatal T concentration on the increased risk for anxiety-like behavior in females, but not in male offspring (21, 73, 74) was observed in humans (103, 104). In one recent study, daughters of mothers with PCOS had a 78% risk increase of anxiety disorders compared to daughters of mothers without PCOS, whereas no significant increase in risk was observed in sons (103). In men, a low 2D:4D ratio was significantly associated with higher trait sociability, bigger personal social capital, and a larger personal social network size rather than social anxiety disorders (104). Together, these data support a sex-dependent effect of high prenatal T exposure, which increases the risk for anxiety disorders, particularly in adult women. Clinical studies on the effects of prenatal dysregulation of estrogens and progesterone and/or their metabolites on the brain, and on cognitive or behavioral consequences in adulthood, are lacking.
Molecular mechanisms of sex steroid hormones action implicated in the regulation of anxiety-like behavior
In multiple animal and human studies, the anxiolytic effects of SSHs were observed through classical signaling pathways of SSH receptors, such as the AR (105, 106), ERs (31, 107–110), and PRs (30, 111). However, non-classical signaling via transmembrane receptors, such as GPER1 also appears to play a significant role in the pathophysiology of anxiety disorders in both males and females. The negative correlation of classical effects of E2 was contrasted with the positive correlation of non-classical effects of E2 via GPER1 in anxiety disorders (107, 112, 113) in both sexes. The concentration of GPER1 in serum is higher in patients with anxiety than in the controls. Therefore, in humans, GPER1 protein could be a possible candidate for a peripheral biomarker in anxiety disorders (113). Unlike humans, the effect of GPER1 signaling on the anxiety-like behavior of rodents appears to be mostly anxiolytic (112, 114, 115). The relationship between the transmembrane AR ZIP9 and anxiety disorders has not yet been described. Nevertheless, its expression has been detected not only in the gonads but also in the brain (64), suggesting a potential role of the AR ZIP9 receptor in the development of affective disorders. Yet, this is the subject of future research.
The role of sex steroid hormones receptors in the regulation of anxiety-like behavior
The outcomes of the animal studies lead to the conclusion that high concentrations of prenatal T may lead to a higher risk of anxiety-like behavior mostly in female offspring in adulthood (21, 22, 73). Anxiety-like behavior in female offspring after prenatal androgen exposure as well as intra-AMY microinjections of T appears to be mediated by the effect of T via AR on the fetal amygdala (21). These studies also showed a sex- (females) and region-specific (AMY) anxiogenic role of T via AR (21, 22, 73).
Activation of ARs appears to have anxiolytic effects in male rats, where the effects of T are blocked with an intramuscular administration of flutamide (selective antagonist of AR). The relationship between the classical effect of T via AR and anxiety appears to be non-linear (116). In male rats, the anxiolytic effect via AR signaling pathways could be partially mediated by intrahippocampal administration of the T metabolite DHT (117). The anxiolytic effect of the AR signaling pathway has been also supported in the study using AR-knockout male mice (allele altered by recombination using CreLox technology resulted in non-functional AR due to premature termination of AR transcription) since these males show higher anxiety-like behavior than control mice (106). In males, the role of ARs in anxiety appears to be region specific. Although the anxiolytic effect of T via AR signaling was observed in the basolateral AMY and suprachiasmatic nucleus of the HYP, no effect was observed in the HIP, medial PFC, paraventricular nucleus of the HYP, bed nucleus of the stria terminalis, or dorsal periaqueductal gray regions (106). Conversely, in male studies comparing wild-type and AR deficient male rats gonadectomized right after birth, gonadectomized rats exhibited lower anxiety-like behavior in both groups compared to their gonadally intact male counterparts. No significant differences between wild-type and AR-deficient male rats indicate that aromatization to E2 rather than AR signaling mediates anxiogenic effects in males (118).
The results from rodent studies on the role of ERα signaling in anxiety-like behavior are inconsistent. In both males and females, ERα signaling has some or no effect on anxiety-like behavior (119, 120). This mechanism may be potentially explained by the action of ERα in the HYP (especially the HPA system), as both intra-paraventricular and peripheral administration of ERα agonist leads to a higher concentration of adrenocorticotropin hormones. These outcomes suggest that ERα may regulate anxiety-like behavior in both male and female rodents via stimulating the function of the HPA axis (120).
On the other hand, the studies using ERβ and ERα selective agonists showed that anxiolytic-like behavior is mediated via ERβ signaling in adult female mice (8–10 weeks or 7–8 months old) (31, 107) and rats (2–3 months old) (109). ERβ knockout mice exhibited higher anxiety-like behavior in the open field test and elevated plus maze, an effect that was predominant in female mice (107, 121). A reduction of anxiety-like behavior through the ERβ signaling pathway was shown in ovariectomized female mice infused with ERβ selective modulators into the HIP (122) or subcutaneously administered with ERβ selective agonist diarylpropionitrile (109). The signaling via ERβ may modulate anxiety-like behavior in female mice via its effect on serotonergic or dopaminergic systems. ERβ KO female mice had significantly reduced concentrations of serotonin in numerous regions of the brain (HIP, the bed nucleus of stria terminalis, preoptic area), together with a reduction of dopamine and dihydroxyphenyl acetate concentrations in the striatum (121).
Moreover, it is possible that gamma-aminobutyric acid (GABA) signaling (the transcription/post-translational modifications of GABA A receptor subunit/regulation of the transmembrane chloride gradient) may be influenced by the loss of ERβ in ERβKO female mice with anxiety-like behavior, causing functional changes (53, 107). This would suggest that ERβ may play a crucial role in the manifestation of anxiety-like behavior in female mice by affecting the GABA A receptor (107). In addition, the ERβ signaling pathway may significantly reduce E2 deficiency-induced anxiety-like behavior in female mice through the modulation of “nucleotide oligomerization domain-like receptor family, pyrin domain-containing 3 inflammasome” (110). ERβ signaling has been suggested to be involved in the synthesis of serotonin in males as well as females. The concentration of serotonin precursor (5-hydroxytryptophan) was reduced in the frontal cortex and striatum in ERβKO male mice (123). Regarding the role of ERs on the HPA axis, unlike ERα, ERβ agonist administration into paraventricular nuclei decreases the concentration of adrenocorticotropin hormones in male rats. Furthermore, the peripheral administration of ERβ agonist decreases adrenocorticotropin hormones in ovariectomized mice. These results indicate that ERβ may regulate anxiety-like behavior via its inhibitory effects on the function of the HPA axis in both male and female rodents (120).
In humans, higher Crh RNA levels were detected in depressed patients postmortem when compared to matched controls. This is accompanied by a higher expression of ERα, involved in the activation of neurons releasing CRH (124). Promoters for the Crh gene include estrogen-responsive elements that start the transcription of this gene (125), and androgen-responsive elements, which repress it (126, 127). The results of the above-mentioned studies suggest that disturbed balance in receptor expression could lead to activation of the HPA axis in patients with anxiety.
The role of non-sex steroid receptors in anxiety disorders
While estrogens regulate anxiety predominantly through ERβ receptors, progesterone (P4) and its metabolites allopregnanolone (ALLO) and pregnanolone appear to promote anxiolytic behavior in female and male rodents through GABA A system (128–132). In the study of Reddy et al. (132), female mice with both a null mutation in the PR gene and wild-type mice had increased concentrations of ALLO in plasma and exhibited lower anxiety-like behavior in the elevated plus maze test. Moreover, pre-treatment of PRKO female mice with the inhibitor of 5α-reductase (finasteride) that blocks the P4 conversion to ALLO, led to higher anxiety-like behavior and, thus, prevented the anxiolytic effect of ALLO (132). P4 and its metabolites can directly modulate GABA A receptor activity via the steroid-binding site of the receptor. It has been also shown that GABA A receptor antagonist (picrotoxin) blocks the anxiolytic effect of P4 in ovariectomized rats (129). Another metabolite allosteric modulator of GABA A—pregnanolone showed to have an anxiolytic effect in adult male mice (CFW) (128). Therefore, these data suggest that the anxiolytic effects of P4 may be mediated via allosteric modulation of GABA A receptors by P4 metabolites (ALLO or pregnanolone) rather than PRs, although PRs may modulate the anxiolytic response in both males (128) and female rodents (129, 132).
Another system involves BDNF. BDNF is responsible for the development, differentiation, and survival of neurons, growth of neurites, synaptic plasticity, synthesis of differentiating factors, and homeostasis as well as modulation of affective-related behaviors (75–77). There is evidence that higher prenatal concentration of androgens may result in a higher risk of anxiety-like behavior in female but not male offspring later in life (21, 73, 74). The molecular mechanisms behind this process are so far unresolved. One possible mechanism leading to anxiety-like behavior involves the BDNF/TrkB system in both male and female rodents. Specifically, female rats with anxiety-like behavior had increased BDNF concentration in the HIP and cortex and decreased neuropeptide Y as well as parvalbumin interneurons in the CA1 region. This may be caused by adaptive reactions of the brain to the prenatal androgen-induced reduction of hippocampal inhibitory neurons in anxious female rats (16). On the other hand, decreased expression of Bdnf in AMY leads to anxiety-like behavior in male rats (111). The effect of higher Bdnf expression on anxiety-like behavior appears to be dependent on the brain regions as well as sex (21, 73, 74, 133). Chen et al. (134) reported higher anxiety-like behavior in knock-in BdnfMet/Met mice confirming the results of clinical studies (134). Moreover, the administration of fluoxetine, SSRI, failed to normalize higher anxiety-like behavior in these mice supporting that the actions of SSRI require BDNF (135, 136). Moreover, the study of Dincheva et al. (137) reported higher anxiety-like behavior in young adult male BdnfMet/Met mice; however, these mice exhibited normal behavior in the adolescent period (137). In addition, the administration of fluoxetine in the early adolescent period led to lower anxiety-like behavior in adult male BdnfMet/Met mice, while its administration in the later phase of adolescence or adulthood failed to decrease anxiety-like behavior in adulthood. These results indicate that (a) Bdnf Val/Met allele is crucial in the modulation of anxiety-like behavior, (b) the impact of the BdnfMet/Met polymorphism or disruption of BDNF signaling occurs in adolescence, and (c) there is a critical time period when fluoxetine administration can normalize higher anxiety-like behavior in adult BdnfMet/Met mice (137).
Similarly, as in animals, lately a single nucleotide polymorphism of the Bdnf gene (Val66Met) has been identified in humans as a risk factor for anxiety disorders, including post-traumatic stress disorder (134, 138, 139). In addition, the BDNF Val66Met polymorphism appears to be associated with higher trait anxiety (140, 141). On the other hand, patients carrying the 66Met variant displayed stronger amygdala activation in response to emotional stimuli in comparison to neutral stimuli, suggesting that mainly 66Met is associated with higher anxiety (114, 134, 141). The neurotrophic factor nerve growth factor, a growth factor, like BDNF, is necessary for sympathetic and sensory neuron survival and maintenance and is involved in the regulation of stress responses via the HPA axis (142). SSHs such as T, E2, and P4 are implicated in the regulation of BDNF or nerve growth factor through which they may provide neuroprotection, neuron survival, and also anxiolytic effects in humans (143) and animals (144–148).
In sum, understanding the molecular mechanisms of SSHs’ effects on the brain is important because it would open the way to the development of novel therapeutic agents for affective disorders. The molecular mechanism of SSHs effect in anxiety disorders has been suggested to be sex- and region specific. The anxiolytic effects appear to involve signaling in the HIP, AMY, PFC, or HPA/HPG axes. These structures are altered via ERβ in females and AR signaling in males as well as increased BDNF/TrkB and GABA A receptor activation with serotonergic pathways in both female and male rodents and humans.
Potential targets for the treatment of anxiety disorders
The cause of anxiety appears to be multifactorial and current psychopharmacological treatments have several limitations as are adverse effects, safety concerns, or restricted efficacy (8–11, 149). Thus, the discovery of novel therapeutic agents would be highly beneficial to patients suffering from affective disorders. As such, understanding how SSHs influence anxiety may open new avenues for drug development to move the field beyond traditional anxiolytic treatments.
Findings about the initial positive effect of E2 on osteoporosis development (150), as well as mood disorders (151) in menopausal females, led to the development of postmenopausal hormone replacement therapies. Unfortunately, an increased risk of endometrial, breast, and ovarian cancer as well as the risk of coronary artery disease was found, and hormone replacement therapy is no longer recommended (152–155). However, the above-mentioned conditions may be mediated through the E2 signaling, and via ERα present in the uterus and mammary gland as was concluded by Liu et al. (156) in their comprehensive review (156). Currently, scientists are trying to find selective agonists for ERβ, as these are not associated with adverse side effects as if treated by the ERα signaling pathway. ERβ pathway can represent a potential therapeutic treatment for postmenopausal women suffering from cognitive deficits and generalized anxiety disorders. Several studies showed ERβ agonists to improve memory (147) and alleviate anxiety-like behavior (157) in young or old ovariectomized mice and rats. The most potent ERβ agonists appear to be cycloheptane-hydroxymethane-based agonists (157–159) and also selective estrogen receptor β modulators (SERBAs), which are highly selective (750-fold) and brain penetrant (120). Nevertheless, novel ERβ agonists are being developed. Regarding the chemical structure of estrogens, which contain four cyclic rings (A–D), the most potent and selective appears to be A–C estrogens without B and D steroid rings (159). Such SERBAs have already been shown to improve memory in ovariectomized young mice. In this experiment, the authors demonstrated the biological efficacy of SERBA that was administered via three routes: direct dorsal HIP infusion, intraperitoneal infusion, and oral gavage to young ovariectomized mice. For memory testing, object recognition and spatial memory consolidation tasks were used. Interestingly, the SERBAs enhanced object recognition and spatial memory consolidation regardless of the acute or chronic administration (159). Another study with EGX358 substance (another SERBA) led to the consolidation of the memory in young ovariectomized mice, and additionally improved drug-induced vasodilatation (160). Although the latter studies do not directly contribute to anxiety treatment, the ERβ agonists represent a promising treatment with further research necessary. Concerning the role of GPER1 signaling in anxiety, there is an evidence suggesting increased GPER1 signaling in patients with anxiety (113). Thus, the potential use of pharmacological manipulation of GPER1 as the anxiolytic drug may be considered in future research.
Allopregnanolone effects regarding anxiety in women appear to be more inconsistent. Indeed, in line with animal studies (30, 129, 131, 161, 162), lower concentrations of ALLO, which is a potent allosteric modulator of the GABA A receptor complex, were associated with anxiety disorders (163). Oral P4 administration to healthy women (19–39 years old) in the follicular phase was positively associated with the neuronal reactivity of the AMY. The oral administration of P4 led to higher plasma concentrations of ALLO, which mediated inhibitory actions of GABA and potentiated the acute effects of P4, i.e., increased AMY reactivity as shown by functional magnetic resonance imaging. Nevertheless, this study did not show any anxiolytic effect of ALLO previously observed in animals and even suggested that ALLO may paradoxically increase anxiety due to the increased neuronal activity in the AMY (164). In contrast, neurosteroid ALLO has been recently approved for the treatment of postpartum depression by the U.S. Food and Drug Administration (165), and more studies support that treatment with ALLO or combinations of SSHs and antidepressants, could be potentially useful for alleviating both anxieties in women.
The effect of SSHs on the anxiety of men is ambiguous. Indeed, there are many studies indicating the correlation between low T and higher anxiety (166–168) or anxiolysis (threat vigilance, reward processing, general fear reduction, stress resilience) induced by T described in another review (169). Many other modalities and treatments are under investigation in male anxiety. Nevertheless, of these, only a few consider T or other SSH as a treatment. Low-baseline and high-reactive endogenous T concentrations (HPG-axis reactivity) were associated with a decrease in the severity of social anxiety symptoms, such as social avoidance behavior and social fear in adult men 18–50 years old (170, 171). The treatment with P4 (50 and 100 mg) resulted in decreased concentrations of cortisol and increased concentrations of noradrenaline in plasma together with increased blood pressure (systolic blood pressure after 50 mg; diastolic blood pressure after 100 mg). These changes resulted in the attenuation of stress-induced increase in anger and frustration as well as better recovery from negative mood changes after stress exposure probably via P4 metabolites (172).
Discussion
The findings from rodent studies suggest that the effects of SSHs on anxiety-like behavior are very complex and context-dependent, determined by the type of SSHs (T, E2, and ALLO), brain region (HIP, AMY, HYP, and PFC), sex, and age (132, 161, 173). SSHs in the pathophysiology of anxiety disorders appear to include signaling in the HIP, AMY, PFC, or HPA/HPG axes via ERβ (or GPER1 concerning the antidepressant-like effects in female rats) in females and AR signaling in males. BDNF/TrkB signaling, GABA A receptor, and the serotonergic system can be implicated in both females and males. Also, there is strong evidence of the anxiolytic-like effects of reduced ALLO acting in the HIP of female rodents (129, 131, 132, 161). In the HIP of male rats, the anxiolytic-like effect on behavior appears to be provided via either the actions of T metabolites DHT (174) or E2 (175). Furthermore, high prenatal T has sex-dependent effects on anxiety-like behavior in rodents. Female offspring after high-prenatal T exposure have an increased risk of anxiety-like behavior in adulthood compared with male offspring. Understanding the molecular mechanisms of SSHs action in the brain affecting anxiety disorders is crucial for developing potent novel drugs (Figure 1A).
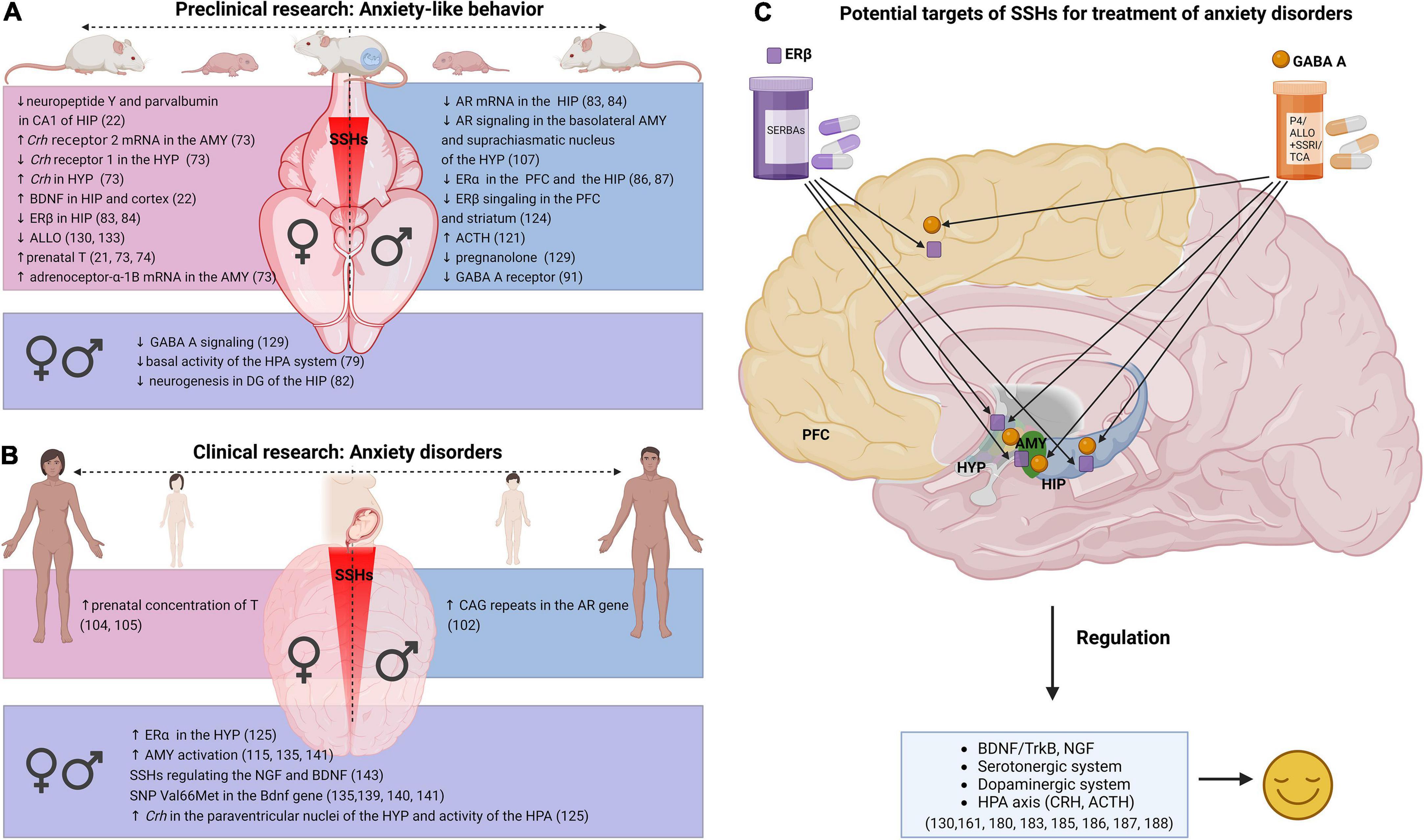
Figure 1. Relationship between SSHs and anxiety in research. (A) Dysregulation related to SSHs in anxiety-like behavior—preclinical research, (B) dysregulation related to SSHs in anxiety disorders—clinical research, (C) treatment approaches for anxiety disorders in adult women and men (Created with BioRender.com). SSHs (sex steroid hormones), AMY (amygdala), Crh (Corticotropin-releasing hormone), HYP (hypothalamus), BDNF (Brain-derived neurotrophic factor), TrkB (tropomyosin receptor kinase B), HIP (hippocampus), HPA (hypothalamic-pituitary-adrenal axis), ERβ (estrogen receptor β), ERα (estrogen receptor α), ALLO (allopregnanolone), GABA A (Gamma-aminobutyric acid A receptor), T (testosterone), AR (androgen receptor), DG (dentate gyrus), PFC (prefrontal cortex), ACTH (adrenocorticotropin hormone), SNP (single nucleotide polymorphism), NGF (nerve growth factor), P4 (progesterone), SERBAs (selective estrogen receptor β agonists), SSRI (selective serotonin reuptake inhibitors), TCA (tricyclic antidepressants).
Considering the positive effect of T, E2, or ALLO treatment on anxiety-like behavior in animals (32, 174, 176–181), clinical studies have been performed (Figure 1B). Of the animal studies, one of the most promising SSHs with antidepressant effects is combined treatment with ALLO (129, 177, 180, 182–184). A very promising pharmacological treatment that provides an anxiolytic effect (Figure 1C) appears to be cycloheptane-hydroxymethane-based agonists—SERBA, which are 750-fold more selective via ERβ receptors thus minimizing the side effects of non-selective agents (159). Lastly, a combination therapy using ALLO with either SSRI or tricyclic antidepressants or a combination of T with imipramine as well as E2 with agomelatine are being successfully tried in clinical studies (185).
To conclude, the studies discussed in this review indicate that potential treatment agents based on SSHs should not be considered as a universal treatment for anxiety disorders on one side, but rather should be personally tailored because their function is context-dependent (sex, age, hormones concentration, route of administration, dose, timing, health condition, genetic predisposition, type of affective disorder). Nevertheless, there are still gaps in the pathophysiology and molecular basis of anxiety that should be clarified by further experiments and studies.
Author contributions
MPi drafted the manuscript. VB revised it critically for important intellectual content and interpreting the relevant literature. MPa and VR revised the manuscript critically for important intellectual content. JH and KF substantially contributed to the conception and design of the manuscript. L’T drafted the manuscript, substantially contributed to the conception and design of the manuscript. All authors contributed to the article and approved the submitted version.
Funding
This study was supported by grant from the Slovak Research and Development Agency No. APVV-20-0185, the Grant Agency of Ministry of Education, Science, Research and Sport of the Slovak Republic VEGA 1/0635/20, SAIA NSP Scholarship, the Tatra bank grant, and the National Institutes of Health (United States) grant R01MH107886. This research was funded by Charles University, Cooperatio Program in Physiological Sciences.
Acknowledgments
We thank Lisa R. Taxier, Thomas T. Lovely, and George W. Sande for proofreading the manuscript for us in the English language.
Conflict of interest
KF was a co-founder of, and shareholder in, Estrigenix Therapeutics, Inc., a company which aims to improve women’s health by developing safe, clinically proven treatments for the mental and physical effects of menopause. She also serves as the company’s Chief Scientific Officer.
The remaining authors declare that the research was conducted in the absence of any commercial or financial relationships that could be construed as a potential conflict of interest.
Publisher’s note
All claims expressed in this article are solely those of the authors and do not necessarily represent those of their affiliated organizations, or those of the publisher, the editors and the reviewers. Any product that may be evaluated in this article, or claim that may be made by its manufacturer, is not guaranteed or endorsed by the publisher.
Abbreviations
2D, the second digit (index finger); 4D, the fourth digit (ring finger); ALLO, allopregnanolone; AMY, amygdala; AR, androgen receptor; BDNF/Bdnf, Brain-derived neurotrophic factor (protein/gene); CRH/Crh, corticotropin-releasing hormone (protein/gene); DHT, dihydrotestosterone; DSM-5, diagnostic and statistical manual of mental disorders; E2, 17β-estradiol; ER, estrogen receptor; GABA, gamma-aminobutyric acid; GPER1, G-protein coupled estrogen receptor 1; HIP, hippocampus; HPA, hypothalamus-pituitary-adrenal axis; HPG, hypothalamus-pituitary-gonadal axis; HYP, hypothalamus; P4, progesterone; PCOS, polycystic ovary syndrome; PFC, prefrontal cortex; PND, postnatal day; PR, progesterone receptor; SERBA, selective estrogen receptor β modulators; Sry, sex-determining region Y; SSHs, sex steroid hormones; SSRI/SNRI, selective serotonin/norepinephrine reuptake inhibitor; T, testosterone; TrkB, tropomyosin receptor kinase B; ZIP9, zinc transporter protein 9.
References
1. American Psychiatric Association. Mood disorder, Diagnostic and Statistical Manual of Mental Disorders. Washington, DC: American Psychiatric Association (2013).
2. Gater R, Tansella M, Korten A, Tiemens BG, Mavreas VG, Olatawura MO. Sex differences in the prevalence and detection of depressive and anxiety disorders in general health care settings: report from the World health organization collaborative study on psychological problems in general health care. Arch Gen Psychiatry. (1998) 55:405–13. doi: 10.1001/archpsyc.55.5.405
3. McLean C, Asnaani A, Litz B, Hofmann S, McLean CP, Asnaani A, et al. Gender differences in anxiety disorders: prevalence, course of illness, comorbidity and burden of illness. J Psychiatr Res. (2011) 45:1027–35. doi: 10.1016/j.jpsychires.2011.03.006
4. Charlson F, van Ommeren M, Flaxman A, Cornett J, Whiteford H, Saxena S. New WHO prevalence estimates of mental disorders in conflict settings: a systematic review and meta-analysis. Lancet. (2019) 394:240–8. doi: 10.1016/S0140-6736(19)30934-1
5. World Health Organization. Depression and Other Common Mental Disorders: Global Health Estimates. Geneva: World Health Organization (2017).
6. Crocco EA, Jaramillo S, Cruz-Ortiz C, Camfield K. Pharmacological management of anxiety disorders in the elderly. Curr Treat Options Psychiatry. (2017) 4:33–46. doi: 10.1007/s40501-017-0102-4
7. Garakani A, Murrough JW, Freire RC, Thom RP, Larkin K, Buono FD, et al. Pharmacotherapy of anxiety disorders: current and emerging treatment options. Front Psychiatry. (2020) 11:595584. doi: 10.3389/fpsyt.2020.595584
8. Laporte S, Chapelle C, Caillet P, Beyens M-N, Bellet F, Delavenne X, et al. Bleeding risk under selective serotonin reuptake inhibitor (SSRI) antidepressants: a meta-analysis of observational studies. Pharmacol Res. (2017) 118:19–32. doi: 10.1016/j.phrs.2016.08.017
9. Shin D, Oh YH, Eom C-S, Park SM. Use of selective serotonin reuptake inhibitors and risk of stroke: a systematic review and meta-analysis. J Neurol. (2014) 261:686–95. doi: 10.1007/s00415-014-7251-9
10. Wu Q, Bencaz AF, Hentz JG, Crowell MD. Selective serotonin reuptake inhibitor treatment and risk of fractures: a meta-analysis of cohort and case–control studies. Osteoporos Int. (2012) 23:365–75. doi: 10.1007/s00198-011-1778-8
11. Khanassov V, Hu J, Reeves D, van Marwijk H. Selective serotonin reuptake inhibitor and selective serotonin and norepinephrine reuptake inhibitor use and risk of fractures in adults: a systematic review and meta-analysis. Int J Geriatr Psychiatry. (2018) 33:1688–708. doi: 10.1002/gps.4974
12. Williams CL. A reevaluation of the concept of separable periods of organizational and activational actions of estrogens in development of brain and behavior. Ann N Y Acad Sci. (1986) 474:282–92. doi: 10.1111/j.1749-6632.1986.tb28019.x
13. Cooke B, Hegstrom CD, Villeneuve LS, Breedlove SM. Sexual differentiation of the vertebrate brain: principles and mechanisms. Front Neuroendocrinol. (1998) 19:323–62. doi: 10.1006/frne.1998.0171
14. McCarthy MM, De Vries GJ, Forger NG. 5.01 - Sexual differentiation of the brain: a fresh look at mode, mechanisms, and meaning. 3rd ed. In: Pfaff DW, Joëls M editors. Hormones, Brain and Behavior. Oxford: Academic Press (2017). p. 3–32.
15. Schulz KM, Sisk CL. The organizing actions of adolescent gonadal steroid hormones on brain and behavioral development. Neurosci Biobehav Rev. (2016) 70:148–58. doi: 10.1016/j.neubiorev.2016.07.036
16. Vigil P, Del Río JP, Carrera B, ArÁnguiz FC, Rioseco H, Cortés ME. Influence of sex steroid hormones on the adolescent brain and behavior: an update. Linacre Q. (2016) 83:308–29. doi: 10.1080/00243639.2016.1211863
17. Bakker J, De Mees C, Douhard Q, Balthazart J, Gabant P, Szpirer J, et al. Alpha-fetoprotein protects the developing female mouse brain from masculinization and defeminization by estrogens. Nat Neurosci. (2006) 9:220–6. doi: 10.1038/nn1624
18. Wallen K. Hormonal influences on sexually differentiated behavior in nonhuman primates. Front Neuroendocrinol. (2005) 26:7–26. doi: 10.1016/j.yfrne.2005.02.001
19. McCarthy MM. How it’s made: organisational effects of hormones on the developing brain. J Neuroendocrinol. (2010) 22:736–42. doi: 10.1111/j.1365-2826.2010.02021.x
20. Pillerová M, Borbélyová V, Hodosy J, Riljak V, Renczés E, Frick KM, et al. On the role of sex steroids in biological functions by classical and non-classical pathways. An update. Front Neuroendocrinol. (2021) 62:100926. doi: 10.1016/j.yfrne.2021.100926
21. Hu M, Richard JE, Maliqueo M, Kokosar M, Fornes R, Benrick A, et al. Maternal testosterone exposure increases anxiety-like behavior and impacts the limbic system in the offspring. Proc Natl Acad Sci U.S.A. (2015) 112:14348–53. doi: 10.1073/pnas.150751411
22. Rankov Petrovic B, Hrncic D, Mladenovic D, Simic T, Suvakov S, Jovanovic D, et al. Prenatal androgenization induces anxiety-like behavior in female rats, associated with reduction of inhibitory interneurons and increased BDNF in hippocampus and cortex. BioMed Res Int. (2019) 2019:3426092. doi: 10.1155/2019/3426092
23. Kessler RC, McGonagle KA, Zhao S, Nelson CB, Hughes M, Eshleman S, et al. Lifetime and 12-month prevalence of DSM-III-R psychiatric disorders in the United States: results from the National Comorbidity Survey. Arch Gen Psychiatry. (1994) 51:8–19. doi: 10.1001/archpsyc.1994.03950010008002
24. Mendell AL, MacLusky NJ. Neurosteroid metabolites of gonadal steroid hormones in neuroprotection: implications for sex differences in neurodegenerative disease. Front Mol Neurosci. (2018) 11:359. doi: 10.3389/fnmol.2018.00359
25. Solomon MB, Herman JP. Sex differences in psychopathology: of gonads, adrenals and mental illness. Physiol Behav. (2009) 97:250–8. doi: 10.1016/j.physbeh.2009.02.033
26. Nolen-Hoeksema S, Larson J, Grayson C. Explaining the gender difference in depressive symptoms. J Pers Soc Psychol. (1999) 77:1061–72. doi: 10.1037/0022-3514.77.5.1061
27. Mir FR, Rivarola MA. Sex differences in anxiety and depression: what can (and cannot) preclinical studies tell us? Sexes. (2022) 3:141–63. doi: 10.3390/sexes3010012
28. Montgomery JC, Brincat M, Tapp A, Appleby L, Versi E, Fenwick PBC, et al. Effect of oestrogen and testosterone implants on psychological disorders in the climacteric. Lancet. (1987) 329:297–9. doi: 10.1016/S0140-6736(87)92026-5
29. Fink G, Sumner B, Rosie R, Wilson H, McQueen J. Androgen actions on central serotonin neurotransmission: relevance for mood, mental state and memory. Behav Brain Res. (1999) 105:53–68. doi: 10.1016/S0166-4328(99)00082-0
30. Frye C, Walf A, Rhodes M, Harney J. Progesterone enhances motor, anxiolytic, analgesic, and antidepressive behavior of wild-type mice, but not those deficient in type 1 5??-reductase. Brain Res. (2004) 1004:116–24. doi: 10.1016/j.brainres.2004.01.020
31. Walf AA, Koonce CJ, Frye CA. Estradiol or diarylpropionitrile decrease anxiety-like behavior of wildtype, but not estrogen receptor beta knockout, mice. Behav Neurosci. (2008) 122:974–81. doi: 10.1037/a0012749
32. Walf AA, Frye CA. Estradiol reduces anxiety- and depression-like behavior of aged female mice. Physiol Behav. (2010) 99:169–74. doi: 10.1016/j.physbeh.2009.09.017
33. Le Mellédo J-M, Baker G. Role of progesterone and other neuroactive steroids in anxiety disorders. Exp Rev Neurother. (2004) 4:851–60. doi: 10.1586/14737175.4.5.851
34. Miller KK, Wexler TL, Zha AM, Lawson EA, Meenaghan EM, Misra M, et al. Androgen deficiency: association with increased anxiety and depression symptom severity in anorexia nervosa. J Clin Psychiatry. (2007) 68:959–65.
35. Barry JA, Hardiman PJ, Saxby BK, Kuczmierczyk A. Testosterone and mood dysfunction in women with polycystic ovarian syndrome compared to subfertile controls. J Psychosom Obstetr Gynecol. (2011) 32:104–11. doi: 10.3109/0167482X.2011.568129
36. Chaudhari AP, Mazumdar K, Mehta PD. Anxiety, depression, and quality of life in women with polycystic ovarian syndrome. Indian J Psychol Med. (2018) 40:239–46. doi: 10.4103/IJPSYM.IJPSYM_561_17
37. Gordon JL, Peltier A, Grummisch JA, Sykes Tottenham L. Estradiol fluctuation, sensitivity to stress, and depressive symptoms in the menopause transition: a pilot study. Front Psychol. (2019) 10:1319. doi: 10.3389/fpsyg.2019.01319
38. Goldstein JM, Seidman LJ, Horton NJ, Makris N, Kennedy DN, Caviness VS Jr, et al. Normal sexual dimorphism of the adult human brain assessed by in vivo magnetic resonance imaging. Cereb Cortex. (2001) 11:490–7. doi: 10.1093/cercor/11.6.490
39. Goldman-Rakic PS, Cools AR, Srivastava K, Roberts AC, Robbins TW, Weiskrantz L. The prefrontal landscape: implications of functional architecture for understanding human mentation and the central executive. Philos Trans R Soc Lond B Biol Sci. (1996) 351:1445–53. doi: 10.1098/rstb.1996.0129
40. Teffer K, Semendeferi K. Chapter 9 - Human prefrontal cortex: evolution, development, and pathology. In: Hofman MA, Falk D editors. Progress in Brain Research. Amsterdam: Elsevier (2012). p. 191–218. doi: 10.1016/B978-0-444-53860-4.00009-X
41. Funahashi S, Bruce CJ, Goldman-Rakic PS. Dorsolateral prefrontal lesions and oculomotor delayed-response performance: evidence for mnemonic” scotomas”. J Neurosci. (1993) 13:1479–97. doi: 10.1523/JNEUROSCI.13-04-01479.1993
42. Gabrieli JDE, Poldrack RA, Desmond JE. The role of left prefrontal cortex in language and memory. Proc Natl Acad Sci U.S.A. (1998) 95:906. doi: 10.1073/pnas.95.3.906
43. Miller EK, Cohen JD. An integrative theory of prefrontal cortex function. Ann Rev Neurosci. (2001) 24:167–202. doi: 10.1146/annurev.neuro.24.1.167
44. Guadagno A, Belliveau C, Mechawar N, Walker C-D. Effects of early life stress on the developing basolateral amygdala-prefrontal cortex circuit: the emerging role of local inhibition and perineuronal nets. Front Hum Neurosci. (2021) 15:669120. doi: 10.3389/fnhum.2021.669120
45. Anand KS, Dhikav V. Hippocampus in health and disease: an overview. Ann Indian Acad Neurol. (2012) 15:239–46. doi: 10.4103/0972-2327.104323
46. Barkus C, McHugh SB, Sprengel R, Seeburg PH, Rawlins JNP, Bannerman DM. Hippocampal NMDA receptors and anxiety: at the interface between cognition and emotion. Eur J Pharmacol. (2010) 626:49–56. doi: 10.1016/j.ejphar.2009.10.014
47. Frick KM. Molecular mechanisms underlying the memory-enhancing effects of estradiol. Horm Behav. (2015) 74:4–18. doi: 10.1016/j.yhbeh.2015.05.001
48. Bannerman D, Grubb M, Deacon R, Yee B, Feldon J, Rawlins J. Ventral hippocampal lesions affect anxiety but not spatial learning. Behav Brain Res. (2003) 139:197–213. doi: 10.1016/s0166-4328(02)00268-1
49. Phillips R, LeDoux J. Differential contribution of amygdala and hippocampus to cued and contextual fear conditioning. Behav Neurosci. (1992) 106:274. doi: 10.1037/0735-7044.106.2.274
50. Calder AJ, Lawrence AD, Young AW. Neuropsychology of fear and loathing. Nat Rev Neurosci. (2001) 2:352–63. doi: 10.1038/35072584
51. Feldman S, Conforti N, Weidenfeld J. Limbic pathways and hypothalamic neurotransmitters mediating adrenocortical responses to neural stimuli. Neurosci Biobehav Rev. (1995) 19:235–40. doi: 10.1016/0149-7634(94)00062-6
52. Öhman A. The role of the amygdala in human fear: automatic detection of threat. Psychoneuroendocrinology. (2005) 30:953–8. doi: 10.1016/j.psyneuen.2005.03.019
53. Baxter MG, Croxson PL. Facing the role of the amygdala in emotional information processing. Proc Natl Acad Sci U.S.A. (2012) 109:21180. doi: 10.1073/pnas.1219167110
54. Ressler KJ. Amygdala activity, fear, and anxiety: modulation by stress. Biol Psychiatry. (2010) 67:1117–9. doi: 10.1016/j.biopsych.2010.04.027
55. Derr R, Cameron S, Golden S. Pre-Analytic considerations for the proper assessment of hormones of the hypothalamic-pituitary axis in epidemiological research. Eur J Epidemiol. (2006) 21:217–26. doi: 10.1007/s10654-006-0011-0
56. Faravelli C, Lo Sauro C, Godini L, Lelli L, Benni L, Pietrini F, et al. Childhood stressful events, HPA axis and anxiety disorders. World J Psychiatry. (2012) 2:13–25. doi: 10.5498/wjp.v2.i1.13
57. Toufexis D, Rivarola MA, Lara H, Viau V. Stress and the reproductive axis. J Neuroendocrinol. (2014) 26:573–86. doi: 10.1111/jne.12179
58. Stevens JS, Hamann S. Sex differences in brain activation to emotional stimuli: a meta-analysis of neuroimaging studies. Neuropsychologia. (2012) 50:1578–93. doi: 10.1016/j.neuropsychologia.2012.03.011
59. Martin EI, Ressler KJ, Binder E, Nemeroff CB. The neurobiology of anxiety disorders: brain imaging, genetics, and psychoneuroendocrinology. Psychiatr Clin N Am. (2009) 32:549–75. doi: 10.1016/j.psc.2009.05.004
60. Shin LM, Liberzon I. The neurocircuitry of fear, stress, and anxiety disorders. Neuropsychopharmacology. (2010) 35:169–91. doi: 10.1038/npp.2009.83
61. Pilhatsch M, Vetter NC, Hübner T, Ripke S, Müller KU, Marxen M, et al. Amygdala-Function perturbations in healthy mid-adolescents with familial liability for depression. J Am Acad Child Adolesc Psychiatry. (2014) 53:559–68.e6. doi: 10.1016/j.jaac.2014.02.010
62. Hare BD, Duman RS. Prefrontal cortex circuits in depression and anxiety: contribution of discrete neuronal populations and target regions. Mol Psychiatry. (2020) 25:2742–58. doi: 10.1038/s41380-020-0685-9
63. Guerra-Araiza C, Coyoy-Salgado A, Camacho-Arroyo I. Sex differences in the regulation of progesterone receptor isoforms expression in the rat brain. Brain Res Bull. (2002) 59:105–9. doi: 10.1016/S0361-9230(02)00845-6
64. Berg AH, Rice CD, Rahman MS, Dong J, Thomas P. Identification and characterization of membrane androgen receptors in the ZIP9 zinc transporter subfamily: I. Discovery in female atlantic croaker and evidence ZIP9 mediates testosterone-induced apoptosis of ovarian follicle cells. Endocrinology. (2014) 155:4237–49. doi: 10.1210/en.2014-1198
65. Almey A, Milner TA, Brake WG. Estrogen receptors in the central nervous system and their implication for dopamine-dependent cognition in females. Horm Behav. (2015) 74:125–38. doi: 10.1016/j.yhbeh.2015.06.010
66. American Psychiatric Association. Anxiety Disorders, Diagnostic and Statistical Manual of Mental Disorders. Virginia, VI: American Psychiatric Association (2013).
67. Bandelow B, Michaelis S. Epidemiology of anxiety disorders in the 21st century. Dialog Clin Neurosci. (2015) 17:327–35. doi: 10.31887/DCNS.2015.17.3/bbandelow
68. dos Santos Gonçalves J, Lopes P, Esteves F, Fernández-Berrocal P. Must we suffer to succeed?: when anxiety boosts motivation and performance. J Individ Differ. (2017) 38:113–24. doi: 10.1027/1614-0001/a000228
69. Wirtz PW, Rohrbeck CA, Burns KM. Anxiety effects on disaster precautionary behaviors: a multi-path cognitive model. J Health Psychol. (2017) 24:1401–11. doi: 10.1177/1359105317720277
70. Kalin NH. Novel insights into pathological anxiety and anxiety-related disorders. Am J Psychiatry. (2020) 177:187–9. doi: 10.1176/appi.ajp.2020.20010057
72. Belovicova K, Bogi E, Csatlosova K, Dubovicky M. Animal tests for anxiety-like and depression-like behavior in rats. Interdiscip Toxicol. (2017) 10:40–3. doi: 10.1515/intox-2017-0006
73. Manti M, Fornes R, Qi X, Folmerz E, Lindén Hirschberg A, de Castro Barbosa T, et al. Maternal androgen excess and obesity induce sexually dimorphic anxiety-like behavior in the offspring. FASEB J. (2018) 32:4158–71. doi: 10.1096/fj.201701263RR
74. Stener-Victorin E, Manti M, Fornes R, Risal S, Lu H, Benrick A. Origins and impact of psychological traits in polycystic ovary syndrome. Med Sci. (2019) 7:86. doi: 10.3390/medsci7080086
75. Bibel M, Barde Y. Neurotrophins: key regulators of cell fate and cell shape in the vertebrate nervous system. Genes Dev. (2000) 14:2919–37. doi: 10.1101/gad.841400
76. Berton O, McClung CA, DiLeone RJ, Krishnan V, Renthal W, Russo SJ, et al. Essential role of BDNF in the mesolimbic dopamine pathway in social defeat stress. Science. (2006) 311:864–8. doi: 10.1126/science.1120972
77. Autry AE, Monteggia LM. Brain-derived neurotrophic factor and neuropsychiatric disorders. Pharmacol Rev. (2012) 64:238–58. doi: 10.1124/pr.111.005108
78. Numakawa T, Odaka H, Adachi N. Actions of brain-derived neurotrophin factor in the neurogenesis and neuronal function, and its involvement in the pathophysiology of brain diseases. Int J Mol Sci. (2018) 19:3650. doi: 10.3390/ijms19113650
79. Pivina SG, Akulova VK, Ordyan NÉ. Characteristics of behavior and stress reactivity of the hypophyseal-adrenocortical system in rats with prenatal inhibition of testosterone metabolism. Neurosci Behav Physiol. (2007) 37:53–8. doi: 10.1007/s11055-007-0149-6
80. Ordian NE, Pivina SG, Akulova VK. Effects of prenatal disturbance in the brain testosterone metabolism on anxiety level and behavior of rats in a novel environment. Neurosci Behav Physiol. (2007) 37:435–41.
81. Ordyan NE, Pivina SG, Akulova VK. Effects of impaired testosterone metabolism during prenatal ontogenesis on the level of anxiety and behavior of rats in a novel environment. Neurosci Behav Physiol. (2007) 37:435–41. doi: 10.1007/s11055-007-0032-5
82. Cheng J, Wu H, Liu H, Li H, Zhu H, Zhou Y, et al. Exposure of hyperandrogen during pregnancy causes depression- and anxiety-like behaviors, and reduced hippocampal neurogenesis in rat offspring. Front Neurosci. (2019) 13:436. doi: 10.3389/fnins.2019.00436
83. Carbone S, Ponzo OJ, Gobetto N, Samaniego YA, Reynoso R, Scacchi P, et al. Antiandrogenic effect of perinatal exposure to the endocrine disruptor di-(2-ethylhexyl) phthalate increases anxiety-like behavior in male rats during sexual maturation. Horm Behav. (2013) 63:692–9. doi: 10.1016/j.yhbeh.2013.01.006
84. Xu X, Yang Y, Wang R, Wang Y, Ruan Q, Lu Y. Perinatal exposure to di-(2-ethylhexyl) phthalate affects anxiety- and depression-like behaviors in mice. Chemosphere. (2015) 124:22–31. doi: 10.1016/j.chemosphere.2014.10.056
85. Shono T, Suita S. Disturbed pituitary-testicular axis inhibits testicular descent in the prenatal rat. BJU Int. (2003) 92:641–3. doi: 10.1046/j.1464-410X.2003.04436.x
86. Pallarés ME, Adrover E, Baier CJ, Bourguignon NS, Monteleone MC, Brocco MA, et al. Prenatal maternal restraint stress exposure alters the reproductive hormone profile and testis development of the rat male offspring. Stress. (2013) 16:429–40. doi: 10.3109/10253890.2012.761195
87. Pallarés ME, Baier CJ, Adrover E, Monteleone MC, Brocco MA, Antonelli MC. Age-Dependent effects of prenatal stress on the corticolimbic dopaminergic system development in the rat male offspring. Neurochem Res. (2013) 38:2323–35. doi: 10.1007/s11064-013-1143-8
88. Rimondini R, Ågren G, Börjesson S, Sommer W, Heilig M. Persistent behavioral and autonomic supersensitivity to stress following prenatal stress exposure in rats. Behav Brain Res. (2003) 140:75–80. doi: 10.1016/S0166-4328(02)00273-5
89. de Souza MA, Centenaro LA, Menegotto PR, Henriques TP, Bonini J, Achaval M, et al. Prenatal stress produces social behavior deficits and alters the number of oxytocin and vasopressin neurons in adult rats. Neurochem Res. (2013) 38:1479–89. doi: 10.1007/s11064-013-1049-5
90. Zuena AR, Mairesse J, Casolini P, Cinque C, Alemà GS, Morley-Fletcher S, et al. Prenatal restraint stress generates two distinct behavioral and neurochemical profiles in male and female rats. PLoS One. (2008) 3:e2170. doi: 10.1371/journal.pone.0002170
91. Barros VG, Rodríguez P, Martijena ID, Pérez A, Molina VA, Antonelli MC. Prenatal stress and early adoption effects on benzodiazepine receptors and anxiogenic behavior in the adult rat brain. Synapse. (2006) 60:609–18. doi: 10.1002/syn.20336
92. Palacios-García I, Lara-Vásquez A, Montiel JF, íaz-Véliz GFD, Sepúlveda H, Utreras E, et al. Prenatal stress down-regulates Reelin expression by methylation of its promoter and induces adult behavioral impairments in rats. PLoS One. (2015) 10:e0117680. doi: 10.1371/journal.pone.0117680
93. Akatsu S, Ishikawa C, Takemura K, Ohtani A, Shiga T. Effects of prenatal stress and neonatal handling on anxiety, spatial learning and serotonergic system of male offspring mice. Neurosci Res. (2015) 101:15–23. doi: 10.1016/j.neures.2015.07.002
94. Fusar-Poli L, Rodolico A, Sturiale S, Carotenuto B, Natale A, Arillotta D, et al. Second-to-fourth digit ratio (2D:4D) in psychiatric disorders: a systematic review of case-control studies. Clin Psychopharmacol Neurosci. (2020) 19:26–45. doi: 10.9758/cpn.2021.19.1.26
95. Zheng Z, Cohn MJ. Developmental basis of sexually dimorphic digit ratios. Proc Natl Acad Sci U.S.A. (2011) 108:16289–94. doi: 10.1073/pnas.110831210
96. Lutchmaya S, Baron-Cohen S, Raggatt P, Knickmeyer R, Manning JT. 2nd to 4th digit ratios, fetal testosterone and estradiol. Early Hum Dev. (2004) 77:23–8. doi: 10.1016/j.earlhumdev.2003.12.002
97. Manning JT, Scutt D, Wilson J, Lewis-Jones DI. The ratio of 2nd to 4th digit length: a predictor of sperm numbers and concentrations of testosterone, luteinizing hormone and oestrogen. Hum Reprod. (1998) 13:3000–4. doi: 10.1093/humrep/13.11.3000
98. Ventura T, Gomes MC, Pita A, Neto MT, Taylor A. Digit ratio (2D:4D) in newborns: influences of prenatal testosterone and maternal environment. Early Hum Dev. (2013) 89:107–12. doi: 10.1016/j.earlhumdev.2012.08.009
99. Evardone M, Alexander GM. Anxiety, sex-linked behaviors, and digit ratios (2D:4D). Arch Sex Behav. (2009) 38:442–55. doi: 10.1007/s10508-007-9260-6
100. Manning JT, Bundred PE, Newton DJ, Flanagan BF. The second to fourth digit ratio and variation in the androgen receptor gene. Evol Hum Behav. (2003) 24:399–405. doi: 10.1016/S1090-5138(03)00052-7
101. Schneider G, Nienhaus K, Gromoll J, Heuft G, Nieschlag E, Zitzmann M. Sex hormone levels, genetic androgen receptor polymorphism, and anxiety in =50-year-old males. J Sex Med. (2011) 8:3452–64. doi: 10.1111/j.1743-6109.2011.02443.x
102. Rodríguez-Ramos Á, Moriana JA, García-Torres F, Ruiz-Rubio M. Emotional stability is related to 2D:4D and social desirability in women: possible implications on subjective well-being and psychopathology. PLoS One. (2021) 16:e0248368. doi: 10.1371/journal.pone.0248368
103. Risal S, Manti M, Lu H, Fornes R, Larsson H, Benrick A, et al. Prenatal androgen exposure causes a sexually dimorphic transgenerational increase in offspring susceptibility to anxiety disorders. Transl Psychiatry. (2021) 11:45. doi: 10.1038/s41398-020-01183-9
104. Buchholz VN, Mühle C, Kornhuber J, Lenz B, Gmel G, Mohler-Kuo M, et al. Lower Digit Ratio (2D:4D) indicative of excess prenatal androgen is associated with increased sociability and greater social capital. Front Behav Neurosci. (2019) 13:246. doi: 10.3389/fnbeh.2019.00246
105. Su Q-R, Su L-Y, Su H-R, Chen Q, Ren G-Y, Yin YOU, et al. Polymorphisms of androgen receptor gene in childhood and adolescent males with first-onset major depressive disorder and association with related symptomatology. Int J Neurosci. (2007) 117:903–17. doi: 10.1080/00207450600910689
106. Chen CV, Brummet JL, Lonstein JS, Jordan CL, Breedlove SM. New knockout model confirms a role for androgen receptors in regulating anxiety-like behaviors and HPA response in mice. Horm Behav. (2014) 65:211–8. doi: 10.1016/j.yhbeh.2014.01.001
107. Krȩżel W, Dupont S, Krust A, Chambon P, Chapman PF. Increased anxiety and synaptic plasticity in estrogen receptor β-deficient mice. Proc Natl Acad Sci U.S.A. (2001) 98:12278. doi: 10.1073/pnas.221451898
108. ÖStlund H, Keller EVA, Hurd YL. Estrogen receptor gene expression in relation to neuropsychiatric disorders. Ann N Y Acad Sci. (2003) 1007:54–63. doi: 10.1196/annals.1286.006
109. Lund TD, Rovis T, Chung WC, Handa RJ. Novel actions of estrogen receptor-β on anxiety-related behaviors. Endocrinology. (2005) 146:797–807. doi: 10.1210/en.2004-1158
110. Xu Y, Sheng H, Bao Q, Wang Y, Lu J, Ni X. NLRP3 inflammasome activation mediates estrogen deficiency-induced depression- and anxiety-like behavior and hippocampal inflammation in mice. Brain Behav Immun. (2016) 56:175–86. doi: 10.1016/j.bbi.2016.02.022
111. Schüle C, Eser D, Baghai TC, Nothdurfter C, Kessler JS, Rupprecht R. Neuroactive steroids in affective disorders: target for novel antidepressant or anxiolytic drugs? Neuroscience. (2011) 191:55–77. doi: 10.1016/j.neuroscience.2011.03.025
112. Kastenberger I, Schwarzer C. GPER1 (GPR30) knockout mice display reduced anxiety and altered stress response in a sex and paradigm dependent manner. Horm Behav. (2014) 66:628–36. doi: 10.1016/j.yhbeh.2014.09.001
113. Fındıklı E, Camkurt MA, Karaaslan MF, Kurutas EB, Altun H, Ýzci F, et al. Serum levels of G protein-coupled estrogen receptor 1 (GPER1) in drug-naive patients with generalized anxiety disorder. Psychiatry Res. (2016) 244:312–6. doi: 10.1016/j.psychres.2016.04.098
114. Chen B, Dowlatshahi D, MacQueen GM, Wang J-F, Young LT. Increased hippocampal BDNF immunoreactivity in subjects treated with antidepressant medication. Biol Psychiatry. (2001) 50:260–5. doi: 10.1016/S0006-3223(01)01083-6
115. Tian Z, Wang Y, Zhang N, Guo Y-Y, Feng B, Liu S-B, et al. Estrogen receptor GPR30 exerts anxiolytic effects by maintaining the balance between GABAergic and glutamatergic transmission in the basolateral amygdala of ovariectomized mice after stress. Psychoneuroendocrinology. (2013) 38:2218–33. doi: 10.1016/j.psyneuen.2013.04.011
116. Hodosy J, Zelmanová D, Majzúnová M, Filová B, Malinová M, Ostatníková D, et al. The anxiolytic effect of testosterone in the rat is mediated via the androgen receptor. Pharmacol Biochem Behav. (2012) 102:191–5. doi: 10.1016/j.pbb.2012.04.005
117. Edinger KL, Frye CA. Intrahippocampal administration of an androgen receptor antagonist, flutamide, can increase anxiety-like behavior in intact and DHT-replaced male rats. Horm Behav. (2006) 50:216–22. doi: 10.1016/j.yhbeh.2006.03.003
118. Zuloaga DG, Poort JE, Jordan CL, Breedlove SM. Male rats with the testicular feminization mutation of the androgen receptor display elevated anxiety-related behavior and corticosterone response to mild stress. Horm Behav. (2011) 60:380–8. doi: 10.1016/j.yhbeh.2011.07.008
119. Zeidan MA, Igoe SA, Linnman C, Vitalo A, Levine JB, Klibanski A, et al. Estradiol modulates medial prefrontal cortex and amygdala activity during fear extinction in women and female rats. Biol Psychiatry. (2011) 70:920–7. doi: 10.1016/j.biopsych.2011.05.016
120. Borrow A, Handa RJ. Estrogen Receptors Modulation of Anxiety-Like Behavior, Vitamins and Hormones. Amsterdam: Elsevier (2017). p. 27–52.
121. Imwalle DB, Gustafsson J-Å, Rissman EF. Lack of functional estrogen receptor β influences anxiety behavior and serotonin content in female mice. Physiol Behav. (2005) 84:157–63. doi: 10.1016/j.physbeh.2004.11.002
122. Walf AA, Frye CA. Administration of estrogen receptor beta-specific selective estrogen receptor modulators to the hippocampus decrease anxiety and depressive behavior of ovariectomized rats. Pharmacol Biochem Behav. (2007) 86:407–14. doi: 10.1016/j.pbb.2006.07.003
123. Hughes ZA, Liu F, Platt BJ, Dwyer JM, Pulicicchio CM, Zhang G, et al. WAY-200070, a selective agonist of estrogen receptor beta as a potential novel anxiolytic/antidepressant agent. Neuropharmacology. (2008) 54:1136–42. doi: 10.1016/j.neuropharm.2008.03.004
124. Wang S, Kamphuis W, Huitinga I, Zhou J, Swaab DF. Gene expression analysis in the human hypothalamus in depression by laser microdissection and real-time PCR: the presence of multiple receptor imbalances. Mol Psychiatry. (2008) 13:786–99. doi: 10.1038/mp.2008.38
125. Vamvakopoulos NC, Chrousos GP. Evidence of direct estrogenic regulation of human corticotropin-releasing hormone gene expression. Potential implications for the sexual dimophism of the stress response and immune/inflammatory reaction. J Clin Investig. (1993) 92:1896–902. doi: 10.1172/JCI116782
126. Bao A-M, Swaab DF. The human hypothalamus in mood disorders: the HPA axis in the center. IBRO Rep. (2019) 6:45–53. doi: 10.1016/j.ibror.2018.11.008
127. Bao A, Fischer D, Wu Y, Hol E, Balesar R, Unmehopa U, et al. A direct androgenic involvement in the expression of human corticotropin-releasing hormone. Mol Psychiatry. (2006) 11:567–76. doi: 10.1038/sj.mp.4001800
128. Gomez C, Saldivar-Gonzalez A, Delgado G, Rodriguez R. Rapid anxiolytic activity of progesterone and pregnanolone in male rats. Pharmacol Biochem Behav. (2002) 72:543–50. doi: 10.1016/S0091-3057(02)00722-0
129. Bitran D, Shiekh M, McLeod M. Anxiolytic effect of progesterone is mediated by the neurosteroid allopregnanolone at brain GABAA receptors. J Neuroendocrinol. (1995) 7:171–7. doi: 10.1111/j.1365-2826.1995.tb00744.x
130. Frye C, Walf A. Estrogen and/or progesterone administered systemically or to the amygdala can have anxiety-, fear-, and pain-reducing effects in ovariectomized rats. Behav Neurosci. (2004) 118:306–13. doi: 10.1037/0735-7044.118.2.306
131. Flores RJ, Cruz B, Uribe KP, Correa VL, Arreguin MC, Carcoba LM, et al. Estradiol promotes and progesterone reduces anxiety-like behavior produced by nicotine withdrawal in female rats. Psychoneuroendocrinology. (2020) 119:104694. doi: 10.1016/j.psyneuen.2020.104694
132. Reddy DS, O’Malley BW, Rogawski MA. Anxiolytic activity of progesterone in progesterone receptor knockout mice. Neuropharmacology. (2005) 48:14–24. doi: 10.1016/j.neuropharm.2004.09.002
133. Maron E. S68-03 new insight into role of BDNF in anxiety. Eur Psychiatry. (2009) 24:S323. doi: 10.1016/S0924-9338(09)70556-5
134. Chen Z-Y, Jing D, Bath KG, Ieraci A, Khan T, Siao C-J, et al. Genetic variant BDNF (Val66Met) polymorphism alters anxiety-related behavior. Science. (2006) 314:140–3. doi: 10.1126/science.1129663
135. Duman RS, Monteggia LM. A neurotrophic model for stress-related mood disorders. Biol Psychiatry. (2006) 59:1116–27. doi: 10.1016/j.biopsych.2006.02.013
136. Castrén E, Kojima M. Brain-derived neurotrophic factor in mood disorders and antidepressant treatments. Neurobiol Dis. (2017) 97:119–26. doi: 10.1016/j.nbd.2016.07.010
137. Dincheva I, Yang J, Li A, Marinic T, Freilingsdorf H, Huang C, et al. Effect of early-life fluoxetine on anxiety-like behaviors in BDNF Val66Met mice. Am J Psychiatry. (2017) 174:1203–13. doi: 10.1176/appi.ajp.2017.15121592
138. Jiang X, Xu K, Hoberman J, Tian F, Marko AJ, Waheed JF, et al. BDNF variation and mood disorders: a novel functional promoter polymorphism and Val66Met are associated with anxiety but have opposing effects. Neuropsychopharmacology. (2005) 30:1353–61. doi: 10.1038/sj.npp.1300703
139. Frielingsdorf H, Bath KG, Soliman F, Difede J, Casey BJ, Lee FS. Variant brain-derived neurotrophic factor Val66Met endophenotypes: implications for posttraumatic stress disorder. Ann N Y Acad Sci. (2010) 1208:150–7. doi: 10.1111/j.1749-6632.2010.05722.x
140. Frustaci A, Pozzi G, Gianfagna F, Manzoli L, Boccia S. Meta-analysis of the brain-derived neurotrophic factor gene (BDNF) Val66Met polymorphism in anxiety disorders and anxiety-related personality traits. Neuropsychobiology. (2008) 58:163–70. doi: 10.1159/000182892
141. Montag C, Reuter M, Newport B, Elger C, Weber B. The BDNF Val66Met polymorphism affects amygdala activity in response to emotional stimuli: evidence from a genetic imaging study. Neuroimage. (2008) 42:1554–9. doi: 10.1016/j.neuroimage.2008.06.008
142. von Richthofen S, Lang UE, Hellweg R. Effects of different kinds of acute stress on nerve growth factor content in rat brain. Brain Res. (2003) 987:207–13. doi: 10.1016/s0006-8993(03)03338-9
143. Angelucci F, Ricci V, Gelfo F, Martinotti G, Brunetti M, Sepede G, et al. BDNF serum levels in subjects developing or not post-traumatic stress disorder after trauma exposure. Brain Cogn. (2014) 84:118–22. doi: 10.1016/j.bandc.2013.11.012
144. Rasika S, Alvarez-Buylla A, Nottebohm F. BDNF mediates the effects of testosterone on the survival of new neurons in an adult brain. Neuron. (1999) 22:53–62. doi: 10.1016/S0896-6273(00)80678-9
145. Walf AA, Frye CA. A review and update of mechanisms of estrogen in the hippocampus and amygdala for anxiety and depression behavior. Neuropsychopharmacology. (2006) 31:1097–111. doi: 10.1038/sj.npp.1301067
146. Morita K, Her S. Progesterone pretreatment enhances serotonin-stimulated BDNF gene expression in rat C6 glioma cells through production of 5α-reduced neurosteroids. J Mol Neurosci. (2008) 34:193–200. doi: 10.1007/s12031-007-9034-6
147. Carrier N, Kabbaj M. Extracellular signal-regulated kinase 2 signaling in the hippocampal dentate gyrus mediates the antidepressant effects of testosterone. Biol Psychiatry. (2012) 71:642–51. doi: 10.1016/j.biopsych.2011.11.028
148. Furuta M, Numakawa T, Chiba S, Ninomiya M, Kajiyama Y, Adachi N, et al. Estrogen, predominantly via estrogen receptor α, attenuates postpartum-induced anxiety- and depression-like behaviors in female rats. Endocrinology. (2013) 154:3807–16. doi: 10.1210/en.2012-2136
149. Bandelow B, Michaelis S, Wedekind D. Treatment of anxiety disorders. Dialog Clin Neurosci. (2017) 19:93–107. doi: 10.31887/DCNS.2017.19.2/bbandelow
150. Gambacciani M, Levancini M. Hormone replacement therapy and the prevention of postmenopausal osteoporosis. Prz Menopauzalny. (2014) 13:213–20. doi: 10.5114/pm.2014.44996
151. Toffol E, Heikinheimo O, Partonen T. Hormone therapy and mood in perimenopausal and postmenopausal women: a narrative review. Menopause. (2015) 22:564–78. doi: 10.1097/GME.0000000000000323
152. Harvey JM, Clark GM, Osborne CK, Allred DC. Estrogen receptor status by immunohistochemistry is superior to the ligand-binding assay for predicting response to adjuvant endocrine therapy in breast cancer. J Clin Oncol. (1999) 17:1474–81. doi: 10.1200/JCO.1999.17.5.1474
153. Grandi G, Caroli M, Cortesi L, Toss A, Tazzioli G, Facchinetti F. Postmenopausal hormone therapy in BRCA gene mutation carriers: to whom and which? Expert Opin Drug Saf. (2020) 19:1025–30. doi: 10.1080/14740338.2020.1791818
154. Minami CA, Freedman RA. Menopausal hormone therapy and long-term breast cancer risk: further data from the women’s health initiative trials. JAMA. (2020) 324:347–9. doi: 10.1001/jama.2020.9620
155. Weng CH, Okawa ER, Roberts MB, Park SK, Umbricht CB, Manson JE, et al. Breast cancer risk in postmenopausal women with medical history of thyroid disorder in the women’s health initiative. Thyroid. (2020) 30:519–30. doi: 10.1089/thy.2019.0426
156. Liu Y, Ma H, Yao J, Ralpha E. A key target for cancer therapy: a review. Onco Targets Ther. (2020) 13:2183–91. doi: 10.2147/OTT.S236532
157. Weiser MJ, Wu TJ, Handa RJ. Estrogen Receptor-β agonist diarylpropionitrile: biological activities of r- and s-enantiomers on behavior and hormonal response to stress. Endocrinology. (2009) 150:1817–25. doi: 10.1210/en.2008-1355
158. Liu F, Day M, Muniz LC, Bitran D, Arias R, Revilla-Sanchez R, et al. Activation of estrogen receptor-beta regulates hippocampal synaptic plasticity and improves memory. Nat Neurosci. (2008) 11:334–43. doi: 10.1038/nn2057
159. Hanson AM, Perera KLIS, Kim J, Pandey RK, Sweeney N, Lu X, et al. A–C Estrogens as potent and selective estrogen receptor-beta agonists (SERBAs) to enhance memory consolidation under low-estrogen conditions. J Med Chem. (2018) 61:4720–38. doi: 10.1021/acs.jmedchem.7b01601
160. Fleischer AW, Schalk JC, Wetzel EA, Hanson AM, Sem DS, Donaldson WA, et al. Long-term oral administration of a novel estrogen receptor beta agonist enhances memory and alleviates drug-induced vasodilation in young ovariectomized mice. Horm Behav. (2021) 130:104948. doi: 10.1016/j.yhbeh.2021.104948
161. Freeman E, Purdy R, Coutifaris C, Rickels K, Paul SM. Anxiolytic metabolites of progesterone: correlation with mood and performance measures following oral progesterone administration to healthy female volunteers. Neuroendocrinology. (1993) 58:478–84. doi: 10.1159/000126579
162. Frye CA, Walf AA. Changes in progesterone metabolites in the hippocampus can modulate open field and forced swim test behavior of proestrous rats. Horm Behav. (2002) 41:306–15. doi: 10.1006/hbeh.2002.1763
163. Schüle C, Nothdurfter C, Rupprecht R. The role of allopregnanolone in depression and anxiety. Prog Neurobiol. (2014) 113:79–87. doi: 10.1016/j.pneurobio.2013.09.003
164. van Wingen GA, van Broekhoven F, Verkes RJ, Petersson KM, Bäckström T, Buitelaar JK, et al. Progesterone selectively increases amygdala reactivity in women. Mol Psychiatry. (2008) 13:325–33. doi: 10.1038/sj.mp.4002030
165. Powell JG, Garland S, Preston K, Piszczatoski C. Brexanolone (Zulresso): finally, an FDA-approved treatment for postpartum depression. Ann Pharmacother. (2019) 54:157–63. doi: 10.1177/1060028019873320
166. Hackett G, Cole N, Bhartia M, Kennedy D, Raju J, Wilkinson P. Testosterone replacement therapy with long-acting testosterone undecanoate improves sexual function and quality-of-life parameters vs. placebo in a population of men with type 2 diabetes. J Sex Med. (2013) 10:1612–27. doi: 10.1111/jsm.12146
167. Kim C, Barrett-Connor E, Aroda VR, Mather KJ, Christophi CA, Horton ES, et al. Testosterone and depressive symptoms among men in the Diabetes Prevention Program. Psychoneuroendocrinology. (2016) 72:63–71. doi: 10.1016/j.psyneuen.2016.06.009
168. Cheng Y, Bateson D, Concepcion K, Stewart M, Lowy M, Sweeney S, et al. Factors associated with the initiation of testosterone replacement therapy in men from the 45 and Up Study. Aust J Gen Pract. (2018) 47:698–704. doi: 10.31128/ajgp-02-18-4480
169. Zitzmann M. Testosterone, mood, behaviour and quality of life. Andrology. (2020) 8:1598–605. doi: 10.1111/andr.12867
170. Cornwell BR, Johnson L, Berardi L, Grillon C. Anticipation of public speaking in virtual reality reveals a relationship between trait social anxiety and startle reactivity. Biol Psychiatry. (2006) 59:664–6. doi: 10.1016/j.biopsych.2005.09.015
171. Hutschemaekers MHM, de Kleine RA, Davis ML, Kampman M, Smits JAJ, Roelofs K. Endogenous testosterone levels are predictive of symptom reduction with exposure therapy in social anxiety disorder. Psychoneuroendocrinology. (2020) 115:104612. doi: 10.1016/j.psyneuen.2020.104612
172. Childs E, Van Dam NT, de Wit H. Effects of acute progesterone administration upon responses to acute psychosocial stress in men. Exp Clin Psychopharmacol. (2010) 18:78–86. doi: 10.1037/a0018060
173. Domonkos E, Hodosy J, Ostatníková D, Celec P. On the role of testosterone in anxiety-like behavior across life in experimental rodents. Front Endocrinol. (2018) 9:441. doi: 10.3389/fendo.2018.00441
174. Edinger KL, Frye CA. Testosterone’s anti-anxiety and analgesic effects may be due in part to actions of its 5alpha-reduced metabolites in the hippocampus. Psychoneuroendocrinology. (2005) 30:418–30. doi: 10.1016/j.psyneuen.2004.11.001
175. Carrier N, Saland SK, Duclot F, He H, Mercer R, Kabbaj M. The anxiolytic and antidepressant-like effects of testosterone and estrogen in gonadectomized male rats. Biol Psychiatry. (2015) 78:259–69. doi: 10.1016/j.biopsych.2014.12.024
176. Maayan R, Touati-Werner D, Ram E, Strous R, Keren O, Weizman A. The protective effect of frontal cortex dehydroepiandrosterone in anxiety and depressive models in mice. Pharmacol Biochem Behav. (2006) 85:415–21. doi: 10.1016/j.pbb.2006.09.010
177. Evans J, Sun Y, McGregor A, Connor B. Allopregnanolone regulates neurogenesis and depressive/anxiety-like behaviour in a social isolation rodent model of chronic stress. Neuropharmacology. (2012) 63:1315–26. doi: 10.1016/j.neuropharm.2012.08.012
178. Wainwright S, Workman J, Tehrani A, Hamson D, Chow C, Lieblich S, et al. Testosterone has antidepressant-like efficacy and facilitates imipramine-induced neuroplasticity in male rats exposed to chronic unpredictable stress. Horm Behav. (2016) 79:58–69. doi: 10.1016/j.yhbeh.2016.01.001
179. El-Khatib YA, Sayed RH, Sallam NA, Zaki HF, Khattab MM. 17β-Estradiol augments the neuroprotective effect of agomelatine in depressive- and anxiety-like behaviors in ovariectomized rats. Psychopharmacology. (2020) 237:2873–86. doi: 10.1007/s00213-020-05580-2
180. Shirayama Y, Fujita Y, Oda Y, Iwata M, Muneoka K, Hashimoto K. Allopregnanolone induces antidepressant-like effects through BDNF-TrkB signaling independent from AMPA receptor activation in a rat learned helplessness model of depression. Behav Brain Res. (2020) 390:112670. doi: 10.1016/j.bbr.2020.112670
181. Chen S, Gao L, Li X, Ye Y. Allopregnanolone in mood disorders: mechanism and therapeutic development. Pharmacol Res. (2021) 169:105682. doi: 10.1016/j.phrs.2021.105682
182. Akwa Y, Purdy RH, Koob GF, Britton KT. The amygdala mediates the anxiolytic-like effect of the neurosteroid allopregnanolone in rat. Behav Brain Res. (1999) 106:119–25. doi: 10.1016/S0166-4328(99)00101-1
183. Meltzer-Brody S, Kanes SJ. Allopregnanolone in postpartum depression: role in pathophysiology and treatment. Neurobiol Stress. (2020) 12:100212. doi: 10.1016/j.ynstr.2020.100212
184. Tsutsui K, Haraguchi S. Neuroprotective actions of cerebellar and pineal allopregnanolone on Purkinje cells. FASEB BioAdvances. (2020) 2:149–59. doi: 10.1096/fba.2019-00055
Keywords: mood disorders, sex steroid receptors, testosterone, brain structures, molecular mechanism
Citation: Pillerová M, Borbélyová V, Pastorek M, Riljak V, Hodosy J, Frick KM and Tóthová L’ (2022) Molecular actions of sex hormones in the brain and their potential treatment use in anxiety disorders. Front. Psychiatry 13:972158. doi: 10.3389/fpsyt.2022.972158
Received: 21 June 2022; Accepted: 17 August 2022;
Published: 08 September 2022.
Edited by:
Todd Denton Gould, University of Maryland, Baltimore, United StatesReviewed by:
Marta Cristina Antonelli, Consejo Nacional de Investigaciones Científicas y Técnicas (CONICET), ArgentinaKristen Delevich, Washington State University, United States
Christina Dalla, National and Kapodistrian University of Athens, Greece
Pavlina Pavlidi, National and Kapodistrian University of Athens, Greece, in collaboration with reviewer CD
Alicia A. Walf, Rensselaer Polytechnic Institute, United States
Copyright © 2022 Pillerová, Borbélyová, Pastorek, Riljak, Hodosy, Frick and Tóthová. This is an open-access article distributed under the terms of the Creative Commons Attribution License (CC BY). The use, distribution or reproduction in other forums is permitted, provided the original author(s) and the copyright owner(s) are credited and that the original publication in this journal is cited, in accordance with accepted academic practice. No use, distribution or reproduction is permitted which does not comply with these terms.
*Correspondence: L’ubomíra Tóthová, bHVib21pcmEudG90aG92YUBpbWJtLnNr