- 1Cell Therapy and Regenerative Medicine Research Center, Endocrinology and Metabolism Molecular-Cellular Sciences Institute, Tehran University of Medical Sciences, Tehran, Iran
- 2Proteomics Research Center, Shahid Beheshti University of Medical Sciences, Tehran, Iran
- 3Diabetes Research Center, Endocrinology and Metabolism Clinical Sciences Institute, Tehran University of Medical Sciences, Tehran, Iran
- 4Endocrinology and Metabolism Research Center, Endocrinology and Metabolism Clinical Sciences Institute, Tehran University of Medical Sciences, Tehran, Iran
Autism spectrum disorder (ASD) refers to a complicated range of childhood neurodevelopmental disorders which can occur via genetic or non-genetic factors. Clinically, ASD is associated with problems in relationships, social interactions, and behaviors that pose many challenges for children with ASD and their families. Due to the complexity, heterogeneity, and association of symptoms with some neuropsychiatric disorders such as ADHD, anxiety, and sleep disorders, clinical trials have not yielded reliable results and there still remain challenges in drug discovery and development pipeline for ASD patients. One of the main steps in promoting lead compounds to the suitable drug for commercialization is preclinical animal testing, in which the efficacy and toxicity of candidate drugs are examined in vivo. In recent years, zebrafish have been able to attract the attention of many researchers in the field of neurological disorders such as ASD due to their outstanding features. The presence of orthologous genes for ASD modeling, the anatomical similarities of parts of the brain, and similar neurotransmitter systems between zebrafish and humans are some of the main reasons why scientists draw attention to zebrafish as a prominent animal model in preclinical studies to discover highly effective treatment approaches for the ASD through genetic and non-genetic modeling methods.
Introduction
Autism spectrum disorder (ASD) is defined as a complex and lifelong neurodevelopmental disorder encompassing a range of conditions that cause challenges with social interactions and interpersonal communications as well as atypical patterns of behaviors, activities, and interests (1, 2), which manifests in childhood (3). The term “autism” was first coined by a psychiatrist known Eugen Bleuler in the early twentieth century (4). Many years later, Leo Kanner introduced the term “infantile autism” as a more accurate definition of autism to describe children with an inherent inability to social and verbal communication with others. One of the features that Kanner emphasized was the obsessive insistence of autistic children on maintaining the same. In years afterward, as studies progressed, another group of similar diseases was discovered by scientists that did not meet all the criteria presented by Kanner. Since then, there have been changes in the definition of autism disease (5). In 2013, the updated version of the book “Diagnostic and Statistical Manual of Mental Disorders (DSM-5),” has defined the diagnostic criteria of ASD as follows:
1 Challenges with social interactions and interpersonal communications:
1.1 Difficulties with socio-emotional interactions.
1.2 Difficulties with non-verbal communication skills.
1.3 Weaknesses in establishing, maintaining, and expanding relationships.
2 Atypical patterns of behaviors, activities, and interests:
2.1 Repetitive, restricted, and stimming behavioral patterns.
2.2 Insisting on performing regular routines and displaying ceremonial patterns, whether they are verbal or non-verbal behaviors.
2.3 Interests accompanied by unusual focus or intensity.
2.4 Displaying sensory over-responsivity or sensory under-responsivity and atypical sensory interests (1).
Studies imply that in the 1970s autism prevalence rate was very low worldwide (about 0.05% of children). However, in recent decades, the autism prevalence rate has risen dramatically (about 0.9–1.5% of children), which is sometimes referred to as the “autism epidemic” (6). Accordingly, a further increase in the prevalence rate of ASD can impose a heavy burden on more individuals and families (in terms of high costs of care, more stress and anxiety, reduced social support, etc.) (7) as well as government entities such as the healthcare and economic systems (8). In recent years, many efforts, such as pharmacological approaches (e.g., risperidone and aripiprazole) and non-pharmacological approaches (e.g., behavioral, developmental, and educational methods), have been made to treat patients. However, those approaches can only alleviate the symptoms. Hence, it can be concluded that no definite treatment has been found for ASD that results in a notable outcome (9). Despite the mentioned approaches toward improving the patient’s quality of life, the need to discover many effective treatment methods remains noteworthy. Hence, achieving a deep knowledge of the mechanisms underlying ASD can be a big step forward in finding new therapeutic approaches for the patients. In this regard, preclinical studies have a great contribution to bridging the theoretical knowledge to the clinical results in drug discovery and development platforms, in which animal models are an indispensable part. In recent decades, a wide range of animals has been studied in this field, among which mice are notable (10). Although mouse models are used traditionally as a prominent model in neurological disorders due to features such as the high similarity between nervous system function and related genes to those of humans as well as the possibility of genetic manipulation to model the diseases, they have their limitations and shortcomings (11–14). For instance, laboratory mice are mainly active during night-time. In addition, they are susceptible to changes in environmental conditions (e.g., temperature, light, and sound). It is also worth mentioning that mouse model-based experiments require considerable cost and effort (15). Moreover, monitoring and examining the social behaviors of mice takes up a great deal of time, whether in real-time laboratory experiments or video recorded, which can pose difficulties to translational research from bench to bedside (16). Non-human primate (NHP) has been also used as another animal model to simulate the behavioral part of ASD phenotype due to the similarity between their neurological circuits associated in behavior to those of humans. Moreover, NHP can open new doors to the study of immunological factors involved in the development of ASD (17). However, developing ASD NHPs models, which fall into the category of complex organisms in terms of structure and function, requires strict ethical considerations (18). In addition to the above, some animal models, such as Prairie vole, Songbird, Drosophila, Aplysia, and C. elegans, have been studied as candidate models for the development of ASD. However, even these models cannot properly demonstrate the complexities of ASD (17). Therefore, achieving more comprehensive information on the pathophysiology of ASD highlights the need to seek efficient animal models to pave the way for discovering more effective therapeutic approaches. In recent years, there has been a great interest in using zebrafish as a promising animal model in neurological disorders studies due to their outstanding features. Regarding the neurological disorders, the salient features of zebrafish can be examined in two categories:
1. General features: Zebrafish embryos develop independently of the maternal fish. In addition to the fast embryonic development, the zebrafish embryos have transparent bodies, which can facilitate directly monitoring of many biological and neurobiological processes. Moreover, the short life cycle and many offspring per reproductive period represent zebrafish as a convenient model for high throughput screening (HTS) (19). Furthermore, the small size of body, the low maintenance costs, and easy housing of a large number of them in a small aquarium are other advantages of zebrafish, which have made them as a model of interest in drug discovery and development pipelines (20).
2. Specialized features related to the nervous system: zebrafish show high a resemblance to mammals in terms of brain morphology and nervous system as well as neural and signaling pathways (19, 21). Additionally, zebrafish display excellent potential for the development of specific social attitudes that play an important role in simulating ASD phenotypes (22). Regarding genetic studies, it is worth mentioning that there is high orthology between zebrafish and human genes, which leads to the transcription of proteins with similar goals and ultimately similar functions of different body systems, especially the nervous system (21). Hereupon, this review will initially discuss the pathophysiology of ASD and reveal the related pharmacological and non-pharmacological therapeutic approaches. Then, this review is focused on zebrafish as a prominent animal model in ASD modeling. Finally, the studies on genetic and non-genetic modeling methods are described.
An Overview on Pathophysiology of Autism Spectrum Disorder
Autism spectrum disorder refers to a group of early childhood neurodevelopmental disabilities that lasts a lifetime and results in impaired social behavior, difficulties in communication, and repetitive type compulsive behaviors (23). Studies imply that ASD is a multifactorial disease (24, 25). Remarkable advances in the knowledge of ASD risk factors have revealed that genetic mutations are among the main factors involved in alterations of the cell and molecular processes (Figure 1) (26). According to preclinical and clinical studies, over 800 ASD-related genes have been reported (27), of which about 100 genes are significantly correlated with the development of autism-related behaviors (28). For instance, AVPR1a, DISC1, DYX1C1, ITGB3, SHANK3, SLC6A4, RELN, and RPL10 are among the genes which are implicated in the metabolic alterations related to the brain in ASD patients (29). To promote the research in this field, genetic studies related to ASD have been conducted in three main areas:
1. Monozygotic (MZs) and dizygotic (DZs) twins: In genetic studies, examining the inheritance of the disease in twins can be considered one of the important and practical approaches. In ASD condition, comparisons of the concordance rate between MZs twins and DZs twins indicate that if one of the MZs twins has autism, the risk of developing the disease in the other twin is high. However, due to the low rate of gene sharing in DZs twins, the risk of developing the disease in the other twin is lower than in MZs condition. Nevertheless, a high impact of the common prenatal environment on the development of autism has been reported compared to genetic factors in twins.
2. First-degree relatives: In this case, a term called “The broad autism phenotype (BAP)” states that cognitive and behavioral changes associated with autism are manifested more closely in individuals without autism, who are categorized as first-degree relatives of autistic people. In other words, the incidence of autism in a family member can be associated with increases in the odds of ASD-related mild impairments in social and communication skills development in non-autistic relatives (30).
3. Rare genetic syndromes comorbidity associated with autism: Individuals with rare genetic syndromes may be more likely to present degrees of ASD-like characteristics. For instance, Fragile X syndrome, Timothy syndrome, Rett syndrome, Tuberous sclerosis, Hamartoma tumor syndrome, Prader-Willi syndrome, Angelman syndromes, DiGeorge syndrome, and neurofibromatosis type 1 are among the most common known genetic syndromes associated with ASD (31). It should also be noted that, as the incidence of co-occurrence between the expressed genetic syndromes and ASD are different, the demonstration of characteristics associated with ASD in these patients can also be varied (30).
On the other hand, non-genetic factors such as environmental factors (e.g., exposure to infections, air pollutants, pesticides, and psychological stress, anti-epileptic drug administration, and folate deficiency) (32) or epigenetic factors (e.g., changes in DNA methylation patterns and microRNAs expression) also play an important role in the development of ASD symptoms (27).
To facilitate studies in this field, the ASD risk factors can be also classified into three other general categories, including (1) prenatal factors (e.g., advanced maternal age and race/ethnicity), (2) perinatal factors (e.g., maternal hypertension, overweight, and diabetes, infections during pregnancy, cesarean section delivery, and preterm birth), and (3) postnatal factors (e.g., postpartum hemorrhage, and abnormal brain growth). It should also be noted that exposure to air pollution in any of the three stages can increase the risk of ASD development (24).
After achieving a comprehensive understanding of known risk factors involved in autism, an important question arises. How do these risk factors give rise to autism? In response to this question, studies suggest that the effect of risk factors in autism is greater on early brain development. In other words, anatomical study of people with ASD reveals changes in the structure of their brains compared to healthy people (33). Hence, to promote the study in this field, a better understanding of differences in brain development in ASD patients’ needs to be highlighted, that will discuss in more detail in the following sections.
Autistic Brain Characteristics
Research has shown that ASD individuals have some abnormalities with brain connectivity often involving frontal or occipital regions. It has been shown that the connectivity between different brain regions (“long-range” connectivity) has been decreased in ASD patients, whereas the connectivity within some specialized regions (“short-range” connections) has been increased. “long-range” connectivity is important for global processing of the incoming information and integrating them. However, “short-range” connections are associated with details of the incoming information. Also, it has been demonstrated that in ASD patients, the left hemisphere plays a greater role in processing the information compared to the right hemisphere. Notably, the left hemisphere is more related to details, whereas the right hemisphere is more related to global and integrated processing. Putting abnormal connectivity and left lateralization, together can explain impairment in facial recognition and spatial skills in ASD individuals (35). Brain volume in ASD patients is another issue that has been addressed in investigations. The brain volume of children with ASD develops rapidly, particularly in the frontal and temporal lobes. But this growth stops at older ages. In addition, the brain volume of adults with ASD is unchanged or reduced compared to the control group (36). To achieve a better understanding of the mechanisms underlying ASD, it needs to zoom in and broaden our perspective to the level of the constituent parts of the brain. In this regard, physiological studies imply that the disturbance in four social areas of the brain, including the amygdala, the orbitofrontal cortex (OFC), the temporomandibular cortex (TPC), and the insula, have key roles in triggering the ASD symptoms (37). In addition to above, dysfunctionality and defect in some regions such as frontotemporal lobe, front parietal cortex, basal ganglia, anterior cingulate cortex (ACC), and hippocampus can have a great contribution to not only the development of ASD but also some disorders such as anxiety and schizophrenia (36). In addition, changes in the volume of white matter in different areas of the brain in people with ASD have been observed compared to normal populations, which may indicate a defect in oligodendrocytes and myelination in some areas of the central nervous system (CNS) under the influence of some genes and growth factors such as Olig1, Olig2, PGFRA, Sox10, Gpr17, EGF, IGF-1, and Nkx2-2. This evidence also suggests that the pattern of abnormal myelination in the brains of people with ASD varies from year to year. For example, in the first 15 years of life, a decrease in the amount of the myelin covering the axons of the arcuate, occipitofrontal fasciculus, and external capsule can be observed. While the amount of myelin in some areas, such as the hippocampus and fusiform gyrus, is highly more than normal. Some of these areas tend to normalize over time, but others, such as the cerebellum and the internal capsule remain hypo-myelinated (38). The pathophysiology of ASD can also be traced to the level of the cells that comprise nervous tissue. For example, microglia cells are dedicated as resident macrophages in the CNS that functions as an innate immune cells to respond to pathogens and neurological injuries. In case of inflammation, nerve damage, and tissue hemostasis disturbance, microglia cells transit from the rest state to the active state, which is mainly accompanied by changes in the genetic and cellular process as well as morphological alterations (39). According to ASD clinical investigations, the activity of microglia in three parts of the brain, including white matter, cortical regions, and cerebellum of postmortem are significantly increased. In addition, an increase in microglia density can be observed in the dorsolateral prefrontal, the fronto-insular, and the visual cortex. Morphologically, the soma size, the filopodia extension, and the dynamic process extension and retraction are also increased in ASD patients’ fronto-insular and visual cortex. However, the observations imply that the granule and Purkinje cell numbers as well as numbers of axons located in the Purkinje cell layer (PCL) in the ASD cerebellum decreased. Also, researches on the brains of ASD patients show that the microglia and neurons in the dorsolateral prefrontal cortex (PFC) are located very close to each other (more than normal), which can justify the over-destruction of healthy neurons and the demolition of dysfunctional synapses by microglia. It should also be noted that, although the level of ionized calcium-binding adapter molecule 1 (IBA1) decreases in the cerebellum, the hyperactivation of three genes, including TREM2, DAP12/KARAP, and CX3CR1 can be observed in PFC (40). Defects in synaptic pruning and disruption of the brain’s excitatory vs. inhibitory (E/I) ratio (effective factor in the development of social withdrawal, language communication difficulties, and restrictive interests or repetitive behaviors in ASD) are some of the microglia dysfunctionalities which can be developed in the brain of ASD patients (41). In addition to microglia, astrocytes have been studied as another important group of cells in the ASD pathogenesis mechanism. This heterogeneous group of cells, which are resident in CNS, have a crucial role in different biological process like maintenance of extracellular ion balance, the formation of new neurons and synapses, and regulation of blood-brain barrier integrity. In case of CNS lesions, astrocytes can trigger the inflammatory state of the CNS as a result of increased expression of glial fibrillary acidic protein (GFAP) (42). Additionally, compared to microglia, astrocytes express more types and numbers of excitatory amino acid transporters (EAATs), which are responsible for the glutamate uptake and release. Therefore, it can be concluded that astrocytes play a more important role than microglia in glutamate homeostasis in the CNS (40). In addition to the stated roles for astrocytes, attention to the interaction between astrocytes and microglia is necessary. In a simple expression, astrocytes activate microglia by secreting ATP. Conversely, microglia affect the activity of astrocytes by secreting cytokines and chemokines. The mentioned cross-talk between astrocytes and microglia is involved in regulating synapse function, maintaining synaptic plasticity, and mediated immune response in the body. Therefore, in ASD condition, any abnormality in these type of cells can lead to the emergence of ASD symptoms such as unusual behaviors and cognitive problems in patients. Moreover, the level of GFAP in the PFC and cerebellum increases dramatically in case of ASD (40).
Relationship Between Gastrointestinal Tract and Brain in Autism Spectrum Disorder
In recent years, specific alterations in gastrointestinal functions have been explored as a new perspective on the pathophysiology of patients with autism. Mainly, ASD patients suffer more digestive problems in comparison with non-ASD people, one of the most common of which is constipation. It has also been shown that digestive problems can lead to displaying some autistic behaviors such as aggression, anger, and lack of proper sleep in patients. In contrast, sometimes diarrhea and bloating are among the causes of atypical behaviors in ASD patients. The cause of diarrhea and bloating problems can be found in the metabolism and absorption impairments of carbohydrates in the patient’s small intestine. More specifically, impaired absorption of mono- and disaccharides in the small intestine causes the transfer of these carbohydrates to the large intestine. As a result, competition between polysaccharide-degrading bacteria and mono- and disaccharide-degrading bacteria increases, which in turn leads to the production of specific bacterial compounds as well as diarrhea and bloating. Microbial dysbiosis is another disorder observed in ASD patients. In addition, cellular and molecular studies indicate that mitochondrial dysfunctionality and high susceptibility of enterocytes to oxidative stress, which can eventually lead to digestive problems in ASD patients. ASD condition is also accompanied by increased intestinal permeability. It is probably due to two reasons: intestinal barrier-forming proteins are less expressed, and conversely, pore-forming proteins of tight junctions are overexpressed in the cells. As a result, these alterations can facilitate the transition of bacterial metabolites through the intestinal barrier, which can trigger inflammation of the brain. In addition, ASD patients show the presence of specific bacterial metabolites [e.g., short-chain fatty acids (SCFAs), indoles, and lipopolysaccharides (LPS)] in the analysis. The production of such bacterial products can trigger some symptoms in ASD patients due to the connection between the intestine and the nervous system. One of the main evidences for the connection between the gastrointestinal and the nervous system is the presence of vagal fibers. But the main question is how this bridge of communication works. In response to this question, evidence shows that the afferent vagal fibers have a synapse with a type of cells resident in intestinal epithelium called enteroendocrine cells, which have a crucial role as epithelial biosensors and can be stimulated by lumen nutrients and bacteria. Therefore, any production of specific and unusual metabolites can lead to an effect on the gut-microbiota-brain axis. Also, high levels of neurotransmitters such as serotonin, inhibitory neurotransmitter gamma-aminobutyric acid (GABA), and noradrenaline, which are also produced by certain species of bacteria, can affect the CNS through intestinal cells and development of various symptoms of ASD in patients (43).
Neurotransmitter Systems in Autism
Neurotransmitters are a wide range of chemical substances, which can boost, balance, and transfer neurological signals from a neuron to the target cell to maintain the proper nervous system and the whole body functions at the end. In addition to transmitting nerve impulses, neurotransmitters play an important role in vital processes such as the growth and differentiation of neurons as well as the development of neural circuits. Due to the vital role of neurotransmitters in the nervous system, it can be understood that any defect or problem in this system can lead to emerging various diseases, such as neurodevelopmental disorders (44). Over the past years, based on the efforts to scrutinize the mechanisms underlying ASD, it has been realized that the neurotransmitter system dysfunctionality is one of the causes of symptoms development in autistic patients. Among the wide range of neurotransmitters, the altered GABAergic system has been considered one of the main reasons linked to ASD pathophysiology, which can lead to cognitive impairments. Conventionally, GABA is involved in vital processes such as the differentiation and maturation of neurons, cell migration, and neuronal-network wiring with an inhibitory influence on the human cortex. However, in ASD condition, the expression of GAD65 and GAD67 decrease, which ultimately lead to GABAergic dysfunctionality via disrupting the conversion of glutamate to GABA. Additionally, in ASD patients, GABA platelet, GABAA, and GABAB levels have been reported lower than normal, and some of these defects are probably related to genetic mutations. In addition to GABA, glutamate (the anion of glutamic acid) as an excitatory neurotransmitter is implicated in ASD pathophysiology. This type of neurotransmitter plays an important role in vital processes such as neuronal development, memory, cell migration, and synaptic plasticity under normal physiological conditions. However, in ASD patients, the glutamatergic system encounters dysfunctionalities. However, it is not clear exactly whether the dysfunctionality is due to hypoglutamatergic or hyperglutamatergic states. To scrutinize these hypotheses, various preclinical and clinical assessments have been conducted. Nevertheless, due to the complexity of the ASD pathophysiology, some studies place more emphasis on the precedence of the hypoglutamatergic hypothesis than on the hyperglutamatergic state, and some studies vice versa. On the whole, it should be emphasized that whether the disturbance of the glutamatergic system is caused by a hypoglutamatergic or hyperglutamatergic state, it will eventually result in the development of ASD symptoms. Similar to glutamate, there are two possible hypotheses for the role of serotonin in ASD, which include hyposerotonin and hyperserotonin states. Although there are some controversies on the validity of one of the hypotheses as the main factor involved in ASD development, observations suggest that some ASD patients get involved with low serotonin levels in the brain as well as high serotonin levels in the blood. In addition to the above, studies at the level of neurotransmitters show abnormalities in the dopaminergic, and subsequently the catecholaminergic systems. Additionally, tests performed on patients’ blood, urine, and cerebrospinal fluid (CSF) showed that patients with ASD had high serum norepinephrine levels (45). In addition to the neurotransmitter systems mentioned above, preclinical and clinical studies have shown evidence of an important role of cholinergic system disturbance in the appearance of some symptoms such as repetitive behaviors, difficulties with social communication skills, cognitive impairment, and unusual attention in ASD patients. Observations on the brain of ASD patients indicate that the cholinergic basal forebrain neurons differs in number and structure manner. In addition, evidence show the unusual nicotinic-cholinergic receptor (nAChR) subunits in some brain regions such as cerebellum, neocortex, striatum, and thalamus, some of which have been the result of mutations in genes CHRNA7 and CHRNB2. It is worth mentioning that the regulation of some of the neurotransmitters functions are under control of histaminergic system. Similar to the other neurotransmitter systems, any disturbance in histaminergic system can also develop ASD symptoms such as cognitive problems. Therefore, understanding the mechanisms underlying histaminergic system can pave the way for discovery new therapeutic approaches to improve ASD symptoms (46).
Diagnosis and Treatment Approaches for Autism Spectrum Disorder Patients
Accurate diagnosis of ASD is at the top challenges list of the patient’s treatment. Although evidence suggests that some methods such as DNA analysis for fragile X syndrome, regular karyotyping, and auditory testing can be complementary approaches to ASD diagnosis, there are no accurate blood tests or imaging techniques to determine the disease (47). In addition, the complexity of the disease and the presence of some identical symptoms between ASD and other psychological disorders can pose problems in early and accurate diagnosis. Therefore, most diagnostic approaches are based on caregiver’s and patients’ reviews, the family history assessment, and real-time patient behaviors investigations. In this regard, some approaches such as Childhood Autism Rating Scale (CARS), The Developmental, Dimensional, and Diagnostic Interview (3di), The Autism Spectrum Disorder-Observation for Children (ASD-OC), The Autism Diagnostic Interview-Revised (ADI-R), The Asperger Syndrome Diagnostic Interview (ASDI), The Diagnostic Interview for Social and Communication Disorders (DISCO), and The Autism Spectrum Disorder–Diagnosis Scale for Intellectually Disabled Adults (ASD-DA) are considered as common diagnostic approaches, which can be applied for ASD patients. After the accurate diagnosis of the disease, the most important issue is to apply effective treatment approaches. As mentioned in the previous sections, there is no definitive treatment for ASD patients. However, applying some pharmacological and non-pharmacological interventions can have a major contribution to relieving symptoms and subsequently improving the quality of life of patients with ASD, which have been discussed in more detail in Table 1 (48).
Zebrafish as an Appropriate Animal Model for Autism Spectrum Disorder
Despite the advances in ASD pathophysiology understanding, there are still challenges in the accurate diagnosis and treatment of the disease (64). For instance, children who struggle with ASD represent heterogeneity in the phenotypic features (65). In addition, ASD is often accompanied by some disorders, such as attention-deficit/hyperactivity disorder (ADHD) (66), emotional and behavioral dysregulation (67), anxiety (68), and sleep disorders which can widen the spectrum even further (69). Moreover, the lack of adequate understanding and comprehensive knowledge of the disease (70), inadequate study design, and failure of clinical trial efficiency bring challenges to drug discovery and development pipelines (64). Hence, conducting preclinical animal testing can not only help decipher the complicated mechanisms underlying ASD but also pave the way for discovering newer and more efficient therapeutic approaches by developing a biological platform (71). Preclinical studies as a bridge between theoretical knowledge and clinical studies are of great importance in drug discovery and development pipelines. Since animal models are an integral part of this stage, observance of guidelines and ethical principles is one of the first and most important measures in preclinical studies. In this regard, the guidelines of preclinical studies establish some strict policies to ensure the observation of the highest standards and ethical principles of working with animals. For instance, a developing well-designed study and applying methods appropriate to the research goals, selecting animal models appropriate to the purpose of the study, employing a sufficient number of animals, taking any necessary measures to maintain animal welfare and safety, and trying to use alternatives to animal models, are among the most critical ethics that should be considered in animal studies (72). Regarding the mentioned ethical principles and international and regional guidelines, over the last decades, Zebrafish has attracted attention as a prominent animal model in various in vivo experiments (73), such as ASD studies due to its prominent neurological characteristics (74). Proper understanding of the zebrafish nervous system function as an outstanding animal model can be an important step in examining the various aspects of drugs and treatment approaches for disorders related to the human nervous system in drug discovery. It has been demonstrated that despite the obvious differences between Zebrafish and mammalians, relative neuroanatomical similarities in both CNS and peripheral nervous system (PNS) as well as some identical molecules in cell signaling pathways and genes involved in the function of the nervous system, have been observed in Zebrafish. Additionally, homologous structures of the brain such as cortex, amygdaloid complex, hippocampus, and basal ganglia have been created Zebrafish as a prominent in vivo system for discovering of drugs (75). In addition to some similarities between mammals and zebrafish, some difference has been reported. For instance, there is no midbrain dopaminergic populations (e.g., the substantia nigra and ventral tegmental area) in zebrafish (22, 76). However, zebrafish display behaviors attributed to these areas, which surprisingly are sensitive to dopamine (22, 77). When it comes to the optimal brain neurochemical properties of zebrafish, talking about associated neurotransmitters seems logical. In this case, it should be noticed that, the main neurotransmitter systems in mammals, such as GABA, glutamate, dopamine, norepinephrine, serotonin, histamine, and acetylcholine are presented in zebrafish (75, 78) allowing the researcher the opportunity to trust the result of animal model designing to an acceptable extent. This similarity has also been approved by pharmacological methods of neurotransmitter targeting (78–81). For instance, similar effects of drug usage on sleep between mammals and zebrafish were reported in a specific psychologic screening (78, 81). Neural regeneration researches also show that the mammalian CNS lacks any regenerative ability, so the number of treatment options available to overcome this limitation is few. In contrast, nervous system regeneration is a common feature among amphibians and also Zebrafish, which is a prominent animal model can repair neuropathies in PNS as well as damaged brain and spinal cord amputation in CNS. Analysis performed on Zebrafish shows that both CNS and PNS have a high capacity for neurogenesis, regeneration of the spinal cord, and re-growth of the axon in the damaged area where glial cells play an important role in the axon targeted orientation and correct synapse formation with postsynaptic cells (82, 83). If a scientific view is taken from the anatomical level of the zebrafish brain and nervous system to the psychological level of the fish, it can be concluded that due to some genetic and anatomical similarities, this animal model can be expected to perform some social behaviors in interaction with the surrounded environment or other living organisms. It has been demonstrated that as social behaviors are an important part of human brain function defects in these behaviors can be considered symptoms of ASD. In this regard, zebrafish sociality studies indicated that the larvae, juvenile, and adult zebrafish have been able to open new doors to the study of social behaviors associated with ASD, such as social preference, social plasticity, social inference, social recognition, shoaling, mimicry, aggression, and restricted or repetitive behaviors (84). To promote the research in this field, some of the most common tests to examine the social behavior of zebrafish are discussed as detailed below.
Social Preference Test
The Zebrafish social preference test refers to tests that examine social attraction and behavioral disturbances of animal in relation to social stimuli with aim of promoting translational medicine and drug discovery and development pipelines in neurological diseases researches. In Zebrafish modeling condition, the social preference tests are performed in two stages, including habituation (fish swimming in the test environment alone) and communication with a social stimulus (placing one or two real populations of the same species, using recorded videos of fish movement, or using virtual reality in the adjacent chambers). The amount of time spent near stimuli is a measure of fish social preference, which is obtained by recorded videos of fish movement in the test environment. It should also be noted that, the consideration of effects of physiological traits and interpersonal behaviors in both test and stimuli fish is necessary (85). For instance, in 2020, Landin et al. indicated that the zebrafish oxytocin system can regulate social behavior, which was indicated via treatment of L-368,899 as an inhibitor of Zebrafish oxytocin receptors (86). In addition, attention to environmental factors are obviously of great importance for studying social preference in Zebrafish (85).
Shoaling Test
Shoaling refers to the behavior that a fish performs by being in a population of its kind to receive some benefits such as protection from the predator, forage finding, and successful mating. To study the shoaling behavior, the target Zebrafish is placed in a tank with a group of its kind. Hence, the measurement of distances between the target and other Zebrafish can indicate different aspects of shoaling (87). In recent years, various studies have been conducted to investigate how shoal behavior is modeled in Zebrafish. For instance, in 2010, a study by B et al. showed that applying 1% ethanol concentration could prevent shoal behavior in Zebrafish models, whereas low concentrations of ethanol (<1%) stimulate the emergence of shoaling (88). In another study conducted in 2012, the effect of hallucinogenic drugs, including phencyclidine (PCP) and mescaline was studied on Zebrafish shoal behavior. The results of this study indicate that shoaling was tighter at 20 mg/l mescaline in comparison to the control group. However, PCP did not affect the emergence of Zebrafish shoal behavior in this experiment (89). In addition to the above, a recent study performed two-dimensional and three-dimensional analyzes of Zebrafish’s behavior after treatment with two anxiogenic drugs, including conspecific alarm substance (CAS) and caffeine (CAF), and an anxiolytic drug including diazepam (DZP). The specific results of this experiment indicate that both CAS and CAF have reduced the volume of shoaling. Additionally, as a result of treatment with these two drugs, the rate of shoal geotaxis has increased and zebrafish got closer to the centroid point. Moreover, the effect of CAS, not CAF, formed a tight shoal. However, in contrast to CAS and CAF, DZP had positive effects on shoal behavior (90). Regarding shoal behavior investigation in Zebrafish, it is noteworthy to mention that some factors such as sex, phenotype (91), age (92), group size, test area space (93), and water flow should also be considered (94).
Inhibitory Avoidance Test
This type of test is generally used to analyze learning and memory processes based on training and/or testing of animals to evaluate the relationship between brain and behavior. The test consists in placing the Zebrafish inside the black and white box and tracking the Zebrafish’s reaction to receiving an electric shock from the device embedded in the black section for many days. Any delay in the entry of fish from the white section to the dark part in the following days after receiving the shock indicates that the avoidance behavior has formed in the model. The results obtained from the study by Manuel et al. in 2014 showed that both electric shock and the emergence of inhibitory avoidance lead to increase in cortisol levels of the Zebrafish to cause flight and fight response. However, upregulation of genes associated with mineralocorticoid receptor (nr3c2), glucocorticoid receptor alpha [nr3c1 (alpha)], and cocaine- and amphetamine-regulated transcript (cart4) is observed only in the case of receiving electric shock (95). In recent years, various studies have been performed to investigate the effects of different compounds or conditions on the quality of Zebrafish inhibitory avoidance. For instance, Amorim et al. conducted investigations in the field of the effects of alcohol on the inhibitory avoidance paradigm in the Zebrafish model. Although the result of this experiment showed the ineffectiveness of acute alcohol exposure in inhibitory avoidance behavior, the authors of this study stated that chronic consumption of alcohol and then quitting can lead to learning impairment (96). But in another study, Manuel et al. focused their study on the separate effect of age, Zebrafish habitat enrichment, and the effect of both of these factors on inhibitory avoidance behavior. In this experiment, cellular and molecular studies have shown that 6- and 12-month-old fish have less inhibitory avoidance behavior due to reduced mRNA expression in some telencephalon-related genes, including pcna, neurod, cart4, and cnr1, and increased in nr3c2/nr3c1(α) and nr3c1(β)/nr3c1(α) ratio. However, such a decrease in this behavior has not been observed in 24-month-old fish. Instead, 24-month-old fish showed delays in avoidance behavior due to decreased expression of three genes, including bdnf, pcna, and cart4 (97). In the same year, another study showed that giving food as a reward to fish could change the behavior of inhibitory avoidance. In this experiment, like the previous study, a decrease in the expression of the cnr1 gene associated with Zebrafish telencephalon has been discovered. However, this study reported the overexpression of crf gene in this model. Based on these cellular and molecular findings, the authors stated that Zebrafish feeding, as a reward, could reduce the behavior of inhibitory avoidance after the first electric shock (98).
Aggression Test
Aggression can be categorized as an adaptive and complex behavior in Zebrafish model. Aggressive behavior can be observed in the Zebrafish with signs such as raising the fin, approaching, biting, wave-like body motion, opening the mouth, changing in body pigmentation, swimming in a circular direction, charging, and chasing to defend and show courage and bravery against the enemy (99). To investigate this type of behavior, various techniques, such as an inclined mirror, two flat mirrors with different acclimation periods, live conspecific stimulus, clay-model stimulus, and video stimulus are used in Zebrafish. In 2015, Way et al. examined the effects of using the six mentioned techniques and stated that two behaviors, including bite and darts, were more pronounced in the flat mirror or live conspecific and inclined mirror stimulus, respectively (100). It should also be noted that consideration of the effect of some environmental factors, such as the enrichment of laboratory tank is of great importance for aggressive behavior studies in Zebrafish models (101).
Repetitive Behavior Test
Repetitive behaviors known as a symptom of neuropsychiatric disorders such as ASD that can also be modeled in animal models such as Zebrafish. Generally, the examining repetitive behavior in Zebrafish is based on the use of novel tank test and tracking the movement of Zebrafish in the studied environment. However, inducing the repetitive behavior can be done through various modeling approaches in Zebrafish populations. For instance, in 2018, Liu et al. revealed that CRISPR/Cas9-induced shank3b mutant zebrafish show abnormal repetitive movements in Zebrafish (102). In addition to the use of genetic techniques, the use of some compounds, such as quinpirole (103), ketamine (104), and ibogaine can induce the repetitive behavior in Zebrafish model (105). However, a study indicated that exposure to moderate levels of ethanol can reduce such behaviors in Zebrafish (Figure 2) (106).
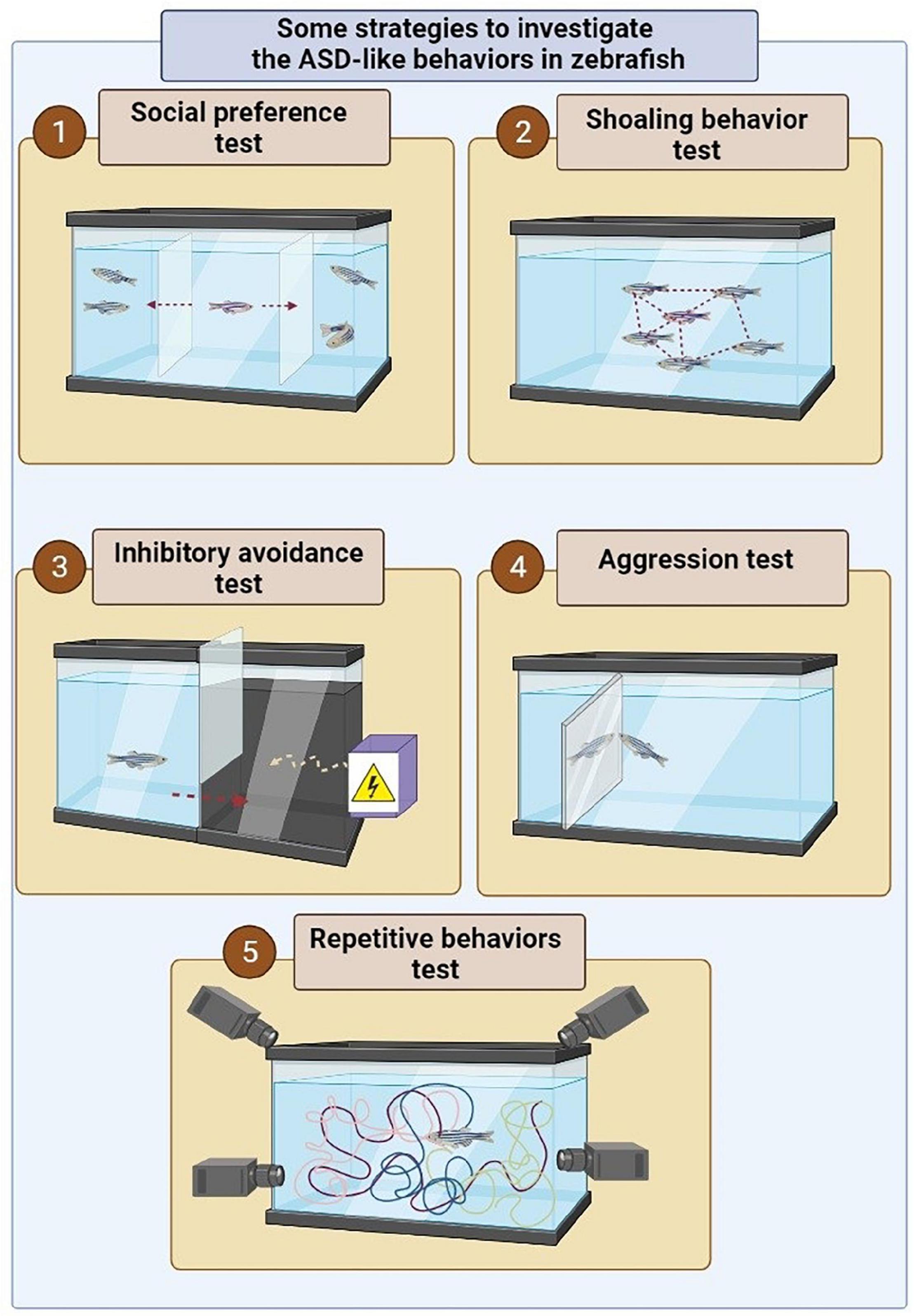
Figure 2. Some strategies to investigate the ASD-like behaviors in zebrafish. (1) In order to assess social preferences, a live zebrafish or robot or photo or zebrafish video is used in an adjacent tank or multi-chamber tank to show the conspecific fish. In this experiment, the social preference behavior is examined by measuring the distance of the fish from the conspecific fish (photo, video, or robot) in the adjacent tank. (2) In order to investigate shoal behavior in the zebrafish, the studied model is placed among a group of conspecific fish inside a tank. In this experiment, the incidence of shoal behavior is determined based on the average distance between the fish in a tank. (3) In order to investigate behavioral avoidance, one of the chamber of the tank becomes dark, which is instinctively more desirable for zebrafish than a brighter environment. However, the presence of the electric shock in a dark environment is considered a risk factor for zebrafish. Hence the conflict between swimming into the dark environment instinctively and avoiding electric shock can be used to investigate behavioral avoidance. (4) In order to assess the aggression behavior, a mirror is applied inside the tank of the zebrafish. (5) The locomotor behavior can be assessed by tracking the position of zebrafish in 3D space and swim path characteristics (22).
Autism Spectrum Disorder Modeling Methods in Zebrafish
Genetic Manipulation Techniques
Zebrafish is considered a suitable animal model for studying genetic diseases due to the high orthology of the genome with humans. According to the results obtained from the Zebrafish genome sequencing project in 2001, zebrafish share about 70% of human genes. In contrast, 69% of human genes have the orthology to those of zebrafish (107). In addition, 82% of human disease-associated genes have orthologues in zebrafish. It should also be noted that more genetic data are also online available in the ZFIN database1 (ZFIN is a comprehensive authoritative resource of zebrafish genetic and genomic data which can be a considerable step toward enhancing the insight into human disease processes) (108). Regarding ASD pathophysiology, genetic studies imply that zebrafish share about 62% of the 858 genes involved in human ASD, which is significantly valuable for preclinical studies. To promote research on the ASD and also pave the way for discovering new therapeutic approaches, the Simons Foundation Autism Research Initiative, or SFARI for short, created the gene loss-of-function lines with mutations in 12 ASD risk genes of zebrafish, which have been described as detailed in the Table 2 (109).
The increase of interest in the Zebrafish model was in parallel with the development of various disease modeling methods. In this regard, to achieve a comprehensive knowledge of the mechanisms involved in Autism spectrum disorder and evaluate the effects of different candidate drugs, various genetic methods, including N-ethyl N-nitrosourea (ENU), transcription activator-like effector nucleases (TALEN), clustered regularly interspaced short palindromic repeats and CRISPR-associated protein 9 (CRISPR-Cas9), Targeted Induced Local Lesions in Genomes (TILLING) method, and zinc finger nucleases (ZFN), have been applied in zebrafish researches, which are discussed as detailed below (78):
N-Ethyl N-Nitrosourea
N-ethyl N-nitrosourea is a mutagenic chemical used in both forward and reverse genetics approaches. The function of ENU is based on generating random point mutations in the genome of the target organism (135). Over the past years, ENU-generated Zebrafish have been employed as one of the animal models in the search for ASD mechanisms. For instance, in 2009, Marjo et al. focused their study on Fragile X syndrome (FXS), which is caused by a mutation in a gene called FMR1. As a result of mutations, in gene FMR1, intellectual and developmental disabilities and some behaviors, such as hyperactivity, seizures, and autistic behaviors develop in the individuals. In this experiment, two FMR1 knockout alleles (hu2787, hu2898) in zebrafish were generated by combining ENU and TILLING methods. As a result of this experiment it was revealed that mRNA derived from the knockout hu2787 allele in zebrafish is not as stable as the normal type. Also, the expression of Fmr protein has increased in some regions of the brain, including the cerebellum and the telencephalon. In addition, they showed that mutant zebrafish are capable to live and showed no signs of craniofacial abnormalities or defects in neurite branching in Rohon–Beard neurons (136). Similar to the previous study, In addition, another study has been performed on the integration of ENU with TALENs to investigate the autism-associated gene neurobeachin (nbea) function and the relationship between the formation of electrical and chemical synapses (137). Moreover, in other studies the integration of two ENU and CRISPR methods have also been conducted to investigate stereotyped behaviors, anxiety, hyperactivity (138), shoaling (139), atypical behaviors, learning and memory disorders, and craniofacial abnormalities in zebrafish (140). Furthermore, the other study focused on the assessment of Rett syndrome, as a neurological disorder with autism-like features, via applying the combination of two ENU and the morpholino methods (141). Although the method of using ENU is recognized as one of the common methods in the study of animal models such as zebrafish, this method is neither low cost nor effortless. Therefore, a method called TILLING was introduced to identify mutations with fewer issues (142).
Targeted Induced Local Lesions in Genomes
Targeted Induced Local Lesions in Genomes method is considered one of the reverse genetic strategies that detect specific mutations in target genes by combining chemical mutagenesis (like ENU) with a high throughput screening (HTS) method. In order to use the TILLING approach in zebrafish studies, a large library of ENU-mutagenized zebrafish populations is needed, which can be prepared from one of two strategies, including (1) cel1 enzyme and (2) resequencing methods (143). Over the past years, the use of TILLING method has also helped to model ASD and related symptoms in zebrafish. For instance, in 2013, Pietri et al. applied the TILLING methods to advance their research in ENU-mutagenized zebrafish for ASD by investigating abnormalities of evoked somatosensory and abnormal thigmotaxis through the mecp2 gene analyzing (119). Despite the advantages of TILLING, there are several disadvantages. For instance, in comparison to other genetic modeling strategies, the performing TILLING method requires considerable effort and time in zebrafish-based preclinical studies. Because many human genes may have two zebrafish orthologs due to the duplicated genome of zebrafish. In addition, the probability of finding the desired mutations by this method is low (78). Therefore, to overcome these problems, some methods such as transcription activator-like effector nucleases (TALEN) and zinc finger nucleases (ZFN) have been introduced as two novel gene-editing methods, which can have a major contribution to making rapid gene modification possible (78, 144–147).
Zinc Finger Nucleases
Zinc finger nucleases is recognized as DNA binding proteins with the capability of generating double-strand breaks in target DNA by their DNA-cutting endonuclease domain fused to zinc finger domains (148). Over the past years, the ZFN approach could also provide the opportunity to promote the knowledge of different disorders such as ASD as a potential strategy for disease modeling and drug discovery. In 2017, an investigation of the role of the CNTNAP2 gene with ASD was presented by Hoffman et al. to investigate phenotypic suppressors and pathways with the intention to discover new therapeutic approaches through generating mutant zebrafish. The authors indicated that the loss of function in the CNTNAP2 gene can lead to dysfunctionalities in GABAergic neurons as well as GABAergic and glutamatergic signaling pathways. In this study, a high rate of sensitivity to drug-induced seizures and hyperactivity during the nighttime was also reported. Accordingly, it can be stated that the CNTNAP2 gene can be used as an effective drug target for the treatment of ASD (124). It is worth mentioning that like any other method, the ZFN technique has some cons as well as pros. In this regard, evidence indicated that ZFN engineering requires high expertise. In addition, there are fewer target sites for the ZFN approach. Moreover, the ZFN approach is accompanied by more off-target mutations which can pose difficulties to preclinical studies (148).
Transcription Activator-Like Effector Nucleases
Transcription activator-like effector nucleases method refers to one of the genome editing techniques that is used to create cuts and mutations in specific sequences of DNA of the organism genome by performing endonuclease activity. According to studies on the effectiveness of the TALENs technique in zebrafish, the mutation rate in both somatic and germ cells of zebrafish have been reported to be dramatically high. Accordingly, it can be considered that TALENs method is one of the effective methods in the Zebrafish modeling process (149). Similar to the previous methods mentioned, the TALENs method has also been used as an effective approach to assessing the symptoms associated with ASD. For example, in 2017, the major role of DYRK1A mutation as a factor involved in the emergence of autistic or intellectual disability induced by microcephaly in Down syndrome was investigated by Kim et al., in which the target gene was mutated through the TALENs method in zebrafish (150). In addition, another study conducted in 2020, examined the link between the oxytocin signaling and social behavior associated with ASD including, social preference and social recognition through generating zebrafish mutants with mutations in the oxtr gene by the TALENs method (151). The comparison between TALENs with ZFN implies that the design of TALENs for various researches is more facile than ZFN. TALENs design is also possible in a shorter time with different lengths of TALE repeat arrays. In addition, the selection range of the target site in TALENs method is wider than ZFN. Moreover, the rate of off-target mutations is lower than ZFN. Furthermore, the results of one study showed that depending on the type of ZFN consumed, ZFN-induced cytotoxicity is more likely. However, it is worth noting that the TALENs technique, also has some limitations and disadvantages in addition to its advantages and high efficiency in zebrafish modeling. For instance, the cDNA encoding the TALENs is much larger than that of ZFN, which can complicate therapeutic research based on the TALENs. In addition, the TALENs delivery by viral vectors remains a challenge due to the presence of the repeat array in TALENs (148).
CRISPR/Cas System
Compared to other genetic modeling methods, it can be declared that CRISPR/Cas technique is one of the newest and most advanced genome editing technologies in the bench to bedside studies (148, 152). Functionally, CRISPR/Cas system is based on generating double-strand breaks into the target DNA, by the cooperation of two complementary sequences of CRISPR and Cas and their endonuclease activities (148). Over the past years, the high efficacy and versatility of the CRISPR technique have gained interest in applying this genome editing approach in research based on zebrafish modeling (153), particularly in neurological disorders such as ASD. For instance, in 2018, Liu et al. published a paper in which they developed zebrafish with mutations in the SHANK3 gene to indicate the association of the mutated SHANK3 with autism-like behaviors (102). In addition, in 2020, Ruzzo et al. conducted investigations in the field of the rare genetic variants’ impacts on ASD development by applying whole-genome sequencing to a wide spectrum of families with autistic children. They also revealed that the mutation in the nr3c2 gene, which has been conducted through CRISPR/Cas9 in zebrafish, can lead to disruption in social interactions and sleep difficulties (154). Regrading to CRISPR-Cas9, it should be noted that CRISPR can perform the gene modification process in a more efficient way than the two previous ones. In a simple expression, a single guide RNA (sgRNA) with the mRNA encoding Cas9 enzyme is directed into the zebrafish embryo, and the Cas9 enzyme can make DNA cleavage in a way that makes insertion-deletion mutations. Therefore, in comparison to previous methods, this technique is easy to use as well as its widespread accessibility to many laboratories (78).
Morpholino
In addition to the above, morpholinos is another common genetic method in zebrafish modeling. Nowadays, because of low cost, ease of use, and a few numbers of rapidly generating genetic mutants, a large number of studies are using morpholinos to repress a specific gene functioning. In detail, morpholinos is a reverse genetic method (21, 78, 155), in which the mRNA splicing or translation processes can be blocked with the intention to knock down a target gene (78, 156, 157). If the applications of morpholino expanded to ASD disease, many related studies can be found in this regard such as studies on the ARX gene for assessment of intellectual disability (158), AUTS2 gene (159), kcnj10a gene (160), cep41 gene (161), ctnnd2b gene (162), syngap1b gene, and shank3a gene for evaluating ASD–associated behaviors (134).
However, there are some shortcomings in the use of morpholinos such as inducing transient effects and the ability to generate off-target effects (78, 163, 164). Another drawback of the morpholino modeling method is the activation of p53, which can trigger the apoptosis pathway and subsequently, create adverse effects such as changes in head size or brain structure (78, 165, 166). In order to efficiently use the morpholino technique, two control items have been explained: (1) the necessity of using two different morpholino targeting sites, and (2) the warranty to rescue the phenotype by using mRNA lacking the morpholino target site (78, 165). Based on some studies, using these controls doesn’t give an equivalent result to gene mutation (78, 167). In order to provide this equality, a new guideline has been introduced, which makes requires morpholino-induced phenotypes to be confirmed in genetic mutants. Nowadays, CRISPR makes this necessity reachable (78, 167). It is worth mentioning in mind that concerning morpholinos application in preclinical studies, it is important to follow the guidelines in the future due to the probable non-specific effects of morpholinos on zebrafish models which can make the phenotype interpretation challenging (78).
Non-genetic Manipulation Techniques
In addition to genetic methods, the use of non-genetic methods is considered one of the effective approaches in zebrafish modeling to scrutinize the mechanisms involved in ASD development and discover efficient drugs through drug discovery and development platforms. According to the pathophysiology of ASD, current non-genetic methods in modeling ASD can be divided into two groups chemical and non-chemical approaches (109). One of the known chemical methods in creating zebrafish models for ASD is valproic acid (VPA). In a recent study conducted by Joseph et al., the effect of VAP on the development of ASD-like behaviors was investigated in zebrafish. As a result of this study, it was found that zebrafish treated with VPA have low viability. It was also reported that the dechorionation of larvae has decreased. In addition, some morphological and anatomical alterations were observed in the zebrafish. For instance, examinations indicate that the tail and the spinal cord are deformed. Moreover, the lack of swim bladder and the incidence of pericardial edema have been observed in zebrafish. However, the most important results were related to the occurrence of some ASD symptoms including hyperactivity and social communication problems. Regarding the results obtained from the study, Joseph et al. applied Duloxetine (DLX) as a candidate drug for alleviating the ASD symptoms which led to significant results. For instance, DLX could improve hyperactivity, social interactions, and anxiety. In addition, at the cell and molecular level, DLX was succeed in the regulation of the acetylcholinesterase -activity and the PI3K/AKT/mTOR pathway (168). In addition to VAP, Pentylenetetrazole (PTZ) is another chemical compound that can be effective in modeling ASD-like behaviors in zebrafish. In order to investigate the effects of PTZ on zebrafish and to discover new therapeutic approaches, in 2015, Torres-Hernández et al., conducted a study. In this regard, zebrafish were first immersed in a tank containing valerenic acid, valerian extracts, or antiepileptic drugs (AEDs). After treating the fish with the mentioned drugs, the zebrafish were placed in a tank containing PTZ. As a result of this experiment, it was determined that the zebrafish displayed stereotyped behaviors and PTZ-induced seizures after PTZ treatment. However, valerenic acid and valerian extracts could postpone the onset of PTZ-induced seizures. In addition, both valerenic acid and valerian extracts had an effective interaction with clonazepam in this process. It should also be noted that the action to delay the onset of PTZ-induced seizures by Valerian extracts has been increasingly better than the function of valerenic acid (169). Regarding non-chemical approaches, the novel tank test is one of the common modeling approaches, which have been developed and introduced over the past years to model anxiety-like behavior in zebrafish (170–173). According to a study conducted in 2019 by Haghani et al., the zebrafish examined by the novel tank test demonstrated the anxiety-like behaviors and a reduction in erratic swimming or darting. In addition, a different rate of acclimation and high levels of thigmotaxis in the light conditions were observed between different ages of zebrafish models (174). Since this non-chemical strategy has been successful in causing anxiety-like behaviors, some researchers have investigated the effects of drugs on zebrafish modeled by the novel tank test. For instance, Levin et al. introduced the Nicotine as a therapeutic approach for relieving the anxiousness induced by the novel tank test in zebrafish. As a result of this study, it was found that nicotine-treated zebrafish exhibit behaviors such as decreased diving to the bottom of the tank. In addition, an increase in locomotor activity, exploration, and two-point discrimination learning were reported, which indicated a reduction in the anxiousness of zebrafish models (175). In recent years, the association between the gastrointestinal tract and the brain has been cited as one of the key mechanisms involved in the pathogenesis of ASD. In this regard, the prominent similar characteristics of zebrafish gastrointestinal tract with those of mammalians, such as having some similar main cells in the gastrointestinal tract, highly conserved gastrointestinal tract function and physiology, innervation by the enteric nervous system, and expression of some similar genes involved in the structure and function of the gastrointestinal system have made the Zebrafish as a suitable animal model for evaluating studies on diseases or disorders related to the gastrointestinal system or gut-brain axis (176). Since gastrointestinal disorders can be categorized as one of the symptoms of ASD, in 2019 James et al. conducted a study based on the effect of a SHANK3 mutated gene on gastrointestinal distress. In this study, they initially developed the Phelan-McDermid syndrome (PMS) model of zebrafish via producing shank3abΔC± and shank3abΔC−/− mutants by applying the CRISPR/Cas9 technique. As a result of this experiment, a reduction in peristaltic contractions and an increase in passage time of compounds in the gastrointestinal tract of shank3abΔC ± mutant Zebrafish were reported, while the number of serotonin-positive enteroendocrine cells (EECs) in both mutant models decreased. It should also be noted that the larvae which were developed after injection of the transcript of human SHANK3 isoform into shank3abΔC± mutant Zebrafish, could empty their intestinal bulb-like wild type. However, the posterior motility of their intestine was still impaired (132). In addition to the above, many studies have also focused on the investigation of the gut-microbiota-brain axis in the development of ASD (177–179). To promote the research in this regard, the use of the germ-free (GF) approach and the application of Gnotobiology science have been expanded in the Zebrafish model. Due to the high growth rate and transparent body of zebrafish, the researchers can easily process them and established them as GF zebrafish models for ASD. Although the use of GF zebrafish models in studies still needs further investigation, some observations have been found that indicate a link between microbiota and the occurrence of social behaviors in the organism (109).
Conclusion
Autism spectrum disorder is recognized as one of the childhood neurodevelopmental disorders, which are accompanied by difficulties in social communications and interactions as well as displaying atypical behaviors and intellectual disability. Due to the symptoms and disorders associated with ASD, major challenges are faced by patients. On the other hand, there is insufficient understanding of the mechanisms involved in the pathophysiology of ASD due to the complexity of the disease. Accordingly, detailed studies of the mechanisms underlying ASD can pave the way for discovering new approaches with high therapeutic potential to maximize the quality of life of patients with ASD as well as their caregivers (180). In recent years, to promote the studies, zebrafish has been used as a leading model in neurological disorders and injuries research (73, 181) such as ASD to investigate the pathophysiology of disease and to evaluate the potency of candidate drugs (22). However, the use of animal models in preclinical studies is still controversial. Because the use of animals in scientific studies requires the observance of strict ethical principles (72, 182–184). Thus, in recent years, studies in the field of neurological disorders have led to the use of new technologies such as organ-on-a-chip. Since this strategy is based on the use of the patient’s stem cells, some of the issues accompanied by applying animal models during the drug discovery and development platforms can be almost addressed and the studies can be upgraded to a more accurate level (183). Moreover, the application of new technologies such as artificial intelligence (AI) and its subsets (e.g., machine learning) as cutting-edge technologies could revolutionize biomedical studies, particularly ASD diagnosis and treatment (185–187). Even, some studies have even directly examined the behaviors of people with autism using advanced technologies. For example, in a recent study, the use of software to record children’s dragging patterns was suggested. In this experiment, the coordinates of the dragging patterns of 60 children were recorded by working with a tablet and related software. This study showed that the analysis of recorded patterns using supervised machine learning algorithms has been able to diagnose autism with 93% accuracy in children (188). In other studies, the application of autism diagnosis approaches using computer-aided eye tracking (189) and automatic facial expression recognition software is suggested, which can facilitate more accurate and better examination of emotions and thoughts expressions. Therefore, it can be concluded that the introduction of computing technologies and computer software can be revolutionized the timely diagnosis of neurological diseases such as ASD in the near future (190).
Author Contributions
AT-B, SH, MA, and HS drafted the manuscript. MT, NN, and BL participated in the study design and interpretation and finalized the manuscript. AT-B and BA supervised the project from a scientific view of point and advised on the study design. All authors read, provided feedback, and approved the final manuscript.
Conflict of Interest
The authors declare that the research was conducted in the absence of any commercial or financial relationships that could be construed as a potential conflict of interest.
Publisher’s Note
All claims expressed in this article are solely those of the authors and do not necessarily represent those of their affiliated organizations, or those of the publisher, the editors and the reviewers. Any product that may be evaluated in this article, or claim that may be made by its manufacturer, is not guaranteed or endorsed by the publisher.
Footnotes
References
1. Hodges H, Fealko C, Soares N. Autism spectrum disorder: definition, epidemiology, causes, and clinical evaluation. Transl Pediatr. (2020) 9:S55.
2. Park HR, Lee JM, Moon HE, Lee DS, Kim B-N, Kim J, et al. A short review on the current understanding of autism spectrum disorders. Exp Neurobiol. (2016) 25:1–13. doi: 10.5607/en.2016.25.1.1
3. Larijani B, Alavi-Moghadam S, Goodarzi P, Rezaei-Tavirani M, Payab M, Gholami M, et al. Cell therapy targets for autism spectrum disorders: hopes, challenges and future directions. Adv Exp Med Biol. (2020) 1341:107–24.
4. Evans B. How autism became autism: the radical transformation of a central concept of child development in Britain. Hist Hum Sci. (2013) 26:3–31. doi: 10.1177/0952695113484320
5. Harris J. Leo Kanner and autism: a 75-year perspective. Int Rev Psychiatry. (2018) 30:3–17. doi: 10.1080/09540261.2018.1455646
6. Fombonne E. Epidemiological controversies in autism. Swiss Arch Neurol Psychiatry Psychother. (2020) 171:w03084.
7. Montes G, Cianca M. Family burden of raising a child with ASD. In: Patel V, Preedy V, Martin C editors. Comprehensive Guide to Autism. New York, NY: Springer (2014).
8. Malik-Soni N, Shaker A, Luck H, Mullin AE, Wiley RE, Lewis MS, et al. Tackling healthcare access barriers for individuals with autism from diagnosis to adulthood. Pediatr Res. (2021) 91:1028–35. doi: 10.1038/s41390-021-01465-y
9. Politte LC, Howe Y, Nowinski L, Palumbo M, McDougle CJ. Evidence-based treatments for autism spectrum disorder. Curr Treat Options Psychiatry. (2015) 2:38–56.
10. Chadman KK, Fernandes S, DiLiberto E, Feingold R. Do animal models hold value in Autism spectrum disorder (ASD) drug discovery?. Expert Opin Drug Discov. (2019) 14:727–34. doi: 10.1080/17460441.2019.1621285
11. Larijani B, Goodarzi P, Payab M, Tayanloo-Beik A, Sarvari M, Gholami M, et al. The design and application of an appropriate Parkinson’s disease animal model in regenerative medicine. Cell Biol Transl Med. (2019) 13:89–105. doi: 10.1007/5584_2019_422
12. Goodarzi P, Aghayan HR, Larijani B, Soleimani M, Dehpour A-R, Sahebjam M, et al. Stem cell-based approach for the treatment of Parkinson’s disease. Med J Islam Repub Iran. (2015) 29:168.
13. Larijani B, Parhizkar Roudsari P, Hadavandkhani M, Alavi-Moghadam S, Rezaei-Tavirani M, Goodarzi P, et al. Stem cell-based models and therapies: a key approach into schizophrenia treatment. Cell Tissue Bank. (2021) 22:207–23.
14. Goodarzi P, Payab M, Alavi-Moghadam S, Larijani B, Rahim F, Bana N, et al. Development and validation of Alzheimer’s disease animal model for the purpose of regenerative medicine. Cell Tissue Bank. (2019) 20:141–51.
15. Geng Y, Peterson RT. The zebrafish subcortical social brain as a model for studying social behavior disorders. Dis Model Mech. (2019) 12:dmm039446. doi: 10.1242/dmm.039446
16. Deutsch SI, Urbano MR, Zemlin C. Mouse models have limitations for development of medications for autism spectrum disorders, but also show much promise. Future Neurol. (2012) 7:1–4.
17. Banerjee S, Bhat M, Riordan M. Genetic aspects of autism spectrum disorders: insights from animal models. Front Cell Neurosci. (2014) 8:58. doi: 10.3389/fncel.2014.00058
18. Watson KK, Platt ML. Of mice and monkeys: using non-human primate models to bridge mouse-and human-based investigations of autism spectrum disorders. J Neurodev Disord. (2012) 4:21. doi: 10.1186/1866-1955-4-21
19. Ijaz MS, Hoffman EJ. Zebrafish: a translational model system for studying neuropsychiatric disorders. J Am Acad Child Adolesc Psychiatry. (2016) 55:746.
20. Tropepe V, Sive HL. Can zebrafish be used as a model to study the neurodevelopmental causes of autism? GenesBrain Behav. (2003) 2:268–81. doi: 10.1034/j.1601-183x.2003.00038.x
21. Kalueff AV, Stewart AM, Gerlai R. Zebrafish as an emerging model for studying complex brain disorders. Trends Pharmacol Sci. (2014) 35:63–75.
22. Meshalkina DA, Kizlyk MN, Kysil EV, Collier AD, Echevarria DJ, Abreu MS, et al. Zebrafish models of autism spectrum disorder. Exp Neurol. (2018) 299:207–16.
24. Ristori MV, Mortera SL, Marzano V, Guerrera S, Vernocchi P, Ianiro G, et al. Proteomics and metabolomics approaches towards a functional insight onto AUTISM spectrum disorders: phenotype stratification and biomarker discovery. Int J Mol Sci. (2020) 21:6274. doi: 10.3390/ijms21176274
25. Larijani B, Foroughi Heravani N, Alavi-Moghadam S, Goodarzi P, Rezaei-Tavirani M, Payab M, et al. Cell therapy targets for autism spectrum disorders: hopes, challenges and future directions. Cell Biol Transl Med. (2020) 13:107–24. doi: 10.1007/5584_2020_491
26. Kumar S, Reynolds K, Ji Y, Gu R, Rai S, Zhou CJ. Impaired neurodevelopmental pathways in autism spectrum disorder: a review of signaling mechanisms and crosstalk. J Neurodev Disord. (2019) 11:10. doi: 10.1186/s11689-019-9268-y
27. Masini E, Loi E, Vega-Benedetti AF, Carta M, Doneddu G, Fadda R, et al. An overview of the main genetic, epigenetic and environmental factors involved in autism spectrum disorder focusing on synaptic activity. Int J Mol Sci. (2020) 21:8290. doi: 10.3390/ijms21218290
28. Tran KT, Le VS, Bui HTP, Do DH, Ly HTT, Nguyen HT, et al. Genetic landscape of autism spectrum disorder in Vietnamese children. Sci Rep. (2020) 10:5034.
29. Cardoso IL, Almeida S. Genes involved in the development of autism. Int Arch Commun Disord. (2019) 2:1–9.
31. Ornoy A, Weinstein-Fudim L, Ergaz Z. Genetic syndromes, maternal diseases and antenatal factors associated with autism spectrum disorders (ASD). Front Neurosci. (2016) 10:316. doi: 10.3389/fnins.2016.00316
32. Saxena R, Babadi M, Namvarhaghighi H, Roullet FI. Role of environmental factors and epigenetics in autism spectrum disorders. Prog Mol Biol Transl Sci. (2020) 173:35–60.
33. Emberti Gialloreti L, Mazzone L, Benvenuto A, Fasano A, Garcia Alcon A, Kraneveld A, et al. Risk and protective environmental factors associated with autism spectrum disorder: evidence-based principles and recommendations. J Clin Med. (2019) 8:217. doi: 10.3390/jcm8020217
34. Nisar S, Hashem S, Bhat AA, Syed N, Yadav S, Azeem MW, et al. Association of genes with phenotype in autism spectrum disorder. Aging. (2019) 11:10742.
35. O’Reilly C, Lewis JD, Elsabbagh M. Is functional brain connectivity atypical in autism? A systematic review of EEG and MEG studies. PLoS One. (2017) 12:e0175870. doi: 10.1371/journal.pone.0175870
36. Ha S, Sohn I-J, Kim N, Sim HJ, Cheon K-A. Characteristics of brains in autism spectrum disorder: structure, function and connectivity across the lifespan. Exp Neurobiol. (2015) 24:273. doi: 10.5607/en.2015.24.4.273
37. Weston CS. Four social brain regions, their dysfunctions, and sequelae, extensively explain autism spectrum disorder symptomatology. Brain Sci. (2019) 9:130. doi: 10.3390/brainsci9060130
38. Galvez-Contreras AY, Zarate-Lopez D, Torres-Chavez AL, Gonzalez-Perez O. Role of oligodendrocytes and myelin in the pathophysiology of autism spectrum disorder. Brain Sci. (2020) 10:951.
39. Thompson KK, Tsirka SE. The diverse roles of microglia in the neurodegenerative aspects of central nervous system (CNS) autoimmunity. Int J Mol Sci. (2017) 18:504. doi: 10.3390/ijms18030504
40. Matta SM, Hill-Yardin EL, Crack PJ. The influence of neuroinflammation in Autism Spectrum Disorder. Brain Behav Immun. (2019) 79:75–90.
41. Koyama R, Ikegaya Y. Microglia in the pathogenesis of autism spectrum disorders. Neurosci Res. (2015) 100:1–5.
42. Siracusa R, Fusco R, Cuzzocrea S. Astrocytes: role and functions in brain pathologies. Front Pharmacol. (2019) 10:1114. doi: 10.3389/fphar.2019.01114
43. Srikantha P, Mohajeri MH. The possible role of the microbiota-gut-brain-axis in autism spectrum disorder. Int J Mol Sci. (2019) 20:2115.
44. Sheffler ZM, Reddy V, Pillarisetty LS. Physiology, neurotransmitters. Treasure Island, FL: StatPearls Publishing (2019).
45. Cetin FH, Tunca H, Güney E, Iseri E. Neurotransmitter systems in autism spectrum disorder. In: Fitzgerald M editor. Autism Spectrum Disorder—Recent Advances. Croatia: IntechOpen (2015). p. 15–30.
46. Eissa N, Al-Houqani M, Sadeq A, Ojha SK, Sasse A, Sadek B. Current enlightenment about etiology and pharmacological treatment of autism spectrum disorder. Front Neurosci. (2018) 12:304. doi: 10.3389/fnins.2018.00304
48. Sharma SR, Gonda X, Tarazi FI. Autism spectrum disorder: classification, diagnosis and therapy. Pharmacol Ther. (2018) 190:91–104.
49. Ventura P, de Giambattista C, Spagnoletta L, Trerotoli P, Cavone M, Di Gioia A, et al. Methylphenidate in autism spectrum disorder: a long-term follow up naturalistic study. J Clin Med. (2020) 9:2566. doi: 10.3390/jcm9082566
50. Ghanizadeh A, Molla M, Olango GJ. The effect of stimulants on irritability in autism comorbid with ADHD: a systematic review. Neuropsychiatr Dis Treat. (2019) 15:1547.
51. Golubchik P, Sever J, Weizman A. Low-dose quetiapine for adolescents with autistic spectrum disorder and aggressive behavior: open-label trial. Clin Neuropharmacol. (2011) 34:216–9. doi: 10.1097/WNF.0b013e31823349ac
52. Cook EH Jr., Rowlett R, Jaselskis C, Leventhal BL. Fluoxetine treatment of children and adults with autistic disorder and mental retardation. J Am Acad Child Adoles Psychiatry. (1992) 31:739–45.
53. Banas K, Sawchuk B. Clonidine as a treatment of behavioural disturbances in autism spectrum disorder: a systematic literature review. J Can Acad Child Adolesc Psychiatry. (2020) 29:110.
54. LeClerc S, Easley D. Pharmacological therapies for autism spectrum disorder: a review. Pharm Ther. (2015) 40:389.
55. Hendaus MA, Jomha FA, Alhammadi AH. Vasopressin in the amelioration of social functioning in autism spectrum disorder. J Clin Med. (2019) 8:1061.
56. Scharoun SM, Wright KT, Robertson-Wilson JE, Fletcher PC, Bryden PJ. Physical activity in individuals with autism spectrum disorders (ASD): a review. In: M Fitzgerald, J Yip editors. Autism-Paradigms, Recent Research and Clinical Applications. London: InTech Open (2017).
57. Li Y-J, Ou J-J, Li Y-M, Xiang D-X. Dietary supplement for core symptoms of autism spectrum disorder: where are we now and where should we go?. Front Psychiatry. (2017) 8:155. doi: 10.3389/fpsyt.2017.00155
58. Hendren RL, James SJ, Widjaja F, Lawton B, Rosenblatt A, Bent S. Randomized, placebo-controlled trial of methyl B12 for children with autism. J Child Adolesc Psychopharmacol. (2016) 26:774–83.
59. Koren G, Moser SS. Does high-dose gestational folic acid increase the risk for autism? The birth order hypothesis. Med Hypotheses. (2019) 132:109350.
60. Baspinar B, Yardimci H. Gluten-free casein-free diet for autism spectrum disorders: can it be effective in solving behavioural and gastrointestinal problems? Eurasian J Med. (2020) 52:292. doi: 10.5152/eurasianjmed.2020.19230
61. McGuinness G, Kim Y. Sulforaphane treatment for autism spectrum disorder: a systematic review. EXCLI J. (2020) 19:892.
62. Gonzales ELT, Jang J-H, Mabunga DFN, Kim J-W, Ko MJ, Cho KS, et al. Supplementation of Korean Red Ginseng improves behavior deviations in animal models of autism. Food Nutr Res. (2016) 60:29245. doi: 10.3402/fnr.v60.29245
63. Lee SH, Shin S, Kim T-H, Kim SM, Do TY, Park S. Safety, effectiveness, and economic evaluation of an herbal medicine, Ukgansangajinpibanha granule, in children with autism spectrum disorder: a study protocol for a prospective, multicenter, randomized, double-blinded, placebo-controlled, parallel-group clinical trial. Trials. (2019) 20:434. doi: 10.1186/s13063-019-3537-7
64. Kalal BS, Pai VR, Bhat SS. Autism treatment challenges: need for accelerated research in pharmacological interventions. Clin Biotechnol Microbiol. (2016) 1:9–10.
65. Lenroot RK, Yeung PK. Heterogeneity within autism spectrum disorders: what have we learned from neuroimaging studies?. Front Hum Neurosci. (2013) 7:733. doi: 10.3389/fnhum.2013.00733
66. Leitner Y. The co-occurrence of autism and attention deficit hyperactivity disorder in children–what do we know?. Front Hum Neurosci. (2014) 8:268. doi: 10.3389/fnhum.2014.00268
67. Mills AS, Tablon-Modica P, Mazefksy CA, Weiss JA. Emotion dysregulation in children with autism: a multimethod investigation of the role of child and parent factors. Res Autism Spectr Disord. (2022) 91:101911.
68. Baribeau DA, Vigod S, Pullenayegum E, Kerns CM, Mirenda P, Smith IM, et al. Co-occurring trajectories of anxiety and insistence on sameness behaviour in autism spectrum disorder. Br J Psychiatry. (2021) 218:20–7. doi: 10.1192/bjp.2020.127
69. Ming X, Walters AS. Autism spectrum disorders, attention deficit/hyperactivity disorder, and sleep disorders. Curr Opin Pulm Med. (2009) 15:578–84.
70. McCracken JT, Anagnostou E, Arango C, Dawson G, Farchione T, Mantua V, et al. Drug development for autism spectrum disorder (ASD): progress, challenges, and future directions. Eur Neuropsychopharmacol. (2021) 48:3–31.
71. Chadman KK. Animal models for autism in 2017 and the consequential implications to drug discovery. Expert Opin Drug Discov. (2017) 12:1187–94. doi: 10.1080/17460441.2017.1383982
72. Nematizadeh M, Payab M, Gholami M, Arjmand B, Larijani B, Tayanloo-Beik A. Preclinical studies for development of biomedical products. In: Arjmand B, Payab M, Goodarzi P editors. Biomedical Product Development:Bench to Bedside. Cham: Springer (2020). p. 49–60.
73. Arjmand B, Tayanloo-Beik A, Foroughi Heravani N, Alaei S, Payab M, Alavi-Moghadam S, et al. Zebrafish for personalized regenerative medicine; a more predictive humanized model of endocrine disease. Front Endocrinol. (2020) 11:396. doi: 10.3389/fendo.2020.00396
74. Stewart AM, Nguyen M, Wong K, Poudel MK, Kalueff AV. Developing zebrafish models of autism spectrum disorder (ASD). Prog Neuropsychopharmacol Biol Psychiatry. (2014) 50:27–36.
75. Guo S. Using zebrafish to assess the impact of drugs on neural development and function. Expert Opin Drug Discov. (2009) 4:715–26. doi: 10.1517/17460440902988464
76. Panula P, Chen YC, Priyadarshini M, Kudo H, Semenova S, Sundvik M, et al. The comparative neuroanatomy and neurochemistry of zebrafish CNS systems of relevance to human neuropsychiatric diseases. Neurobiol Dis. (2010) 40:46–57. doi: 10.1016/j.nbd.2010.05.010
77. Panula P, Sallinen V, Sundvik M, Kolehmainen J, Torkko V, Tiittula A, et al. Modulatory neurotransmitter systems and behavior: towards zebrafish models of neurodegenerative diseases. Zebrafish. (2006) 3:235–47. doi: 10.1089/zeb.2006.3.235
78. Sakai C, Ijaz S, Hoffman EJ. Zebrafish models of neurodevelopmental disorders: past, present, and future. Front Mol Neurosci. (2018) 11:294. doi: 10.3389/fnmol.2018.00294
80. Renier C, Faraco JH, Bourgin P, Motley T, Bonaventure P, Rosa F, et al. Genomic and functional conservation of sedative-hypnotic targets in the zebrafish. Pharmacogenet Genomics. (2007) 17:237–53.
81. Rihel J, Prober DA, Arvanites A, Lam K, Zimmerman S, Jang S, et al. Zebrafish behavioral profiling links drugs to biological targets and rest/wake regulation. Science. (2010) 327:348–51. doi: 10.1126/science.1183090
82. Ghosh S, Hui SP. Regeneration of zebrafish CNS: adult neurogenesis. Neural Plast. (2016) 2016:5815439.
83. Won SY, Choi B-O, Chung KW, Lee JE. Zebrafish is a central model to dissect the peripheral neuropathy. Genes Genomics. (2019) 41:993–1000.
84. Dreosti E, Hoffman EJ, Rihel J. Modeling autism spectrum disorders in zebrafish. In: Gerlai RT editor. Behavioral and Neural Genetics of Zebrafish. Amsterdam: Elsevier (2020). p. 451–80.
85. Ogi A, Licitra R, Naef V, Marchese M, Fronte B, Gazzano A, et al. Social preference tests in zebrafish: a systematic review. Front Vet Sci. (2021) 7:590057. doi: 10.3389/fvets.2020.590057
86. Landin J, Hovey D, Xu B, Lagman D, Zettergren A, Larhammar D, et al. Oxytocin receptors regulate social preference in zebrafish. Sci Rep. (2020) 10:5435.
87. Nunes AR, Ruhl N, Winberg S, Oliveira RF. Social phenotypes in zebrafish. In: Kalueff AV editor. The Rights and Wrongs of Zebrafish: Behavioral Phenotyping of Zebrafish. Berlin: Springer (2017). p. 95–130.
88. Kurta A, Palestis BG. Effects of ethanol on the shoaling behavior of zebrafish (Danio rerio). Dose Response. (2010) 8:527–33. doi: 10.2203/dose-response.10-008.Palestis
89. Kyzar EJ, Collins C, Gaikwad S, Green J, Roth A, Monnig L, et al. Effects of hallucinogenic agents mescaline and phencyclidine on zebrafish behavior and physiology. Prog Neuropsychopharmacol Biol Psychiatry. (2012) 37:194–202. doi: 10.1016/j.pnpbp.2012.01.003
90. Rosa LV, Costa FV, Canzian J, Borba JV, Quadros VA, Rosemberg DB. Three-and bi-dimensional analyses of the shoaling behavior in zebrafish: influence of modulators of anxiety-like responses. Prog Neuropsychopharmacol Biol Psychiatry. (2020) 102:109957. doi: 10.1016/j.pnpbp.2020.109957
91. Snekser JL, Ruhl N, Bauer K, McRobert SP. The influence of sex and phenotype on shoaling decisions in zebrafish. Int J Comp Psychol. (2010) 23:70–81.
92. Buske C, Gerlai R. Shoaling develops with age in Zebrafish (Danio rerio). Prog Neuropsychopharmacol Biol Psychiatry. (2011) 35:1409–15. doi: 10.1016/j.pnpbp.2010.09.003
93. Shelton DS, Price BC, Ocasio KM, Martins EP. Density and group size influence shoal cohesion, but not coordination in zebrafish (Danio rerio). J Comp Psychol. (2015) 129:72. doi: 10.1037/a0038382
94. Suriyampola PS, Sykes DJ, Khemka A, Shelton DS, Bhat A, Martins EP. Water flow impacts group behavior in zebrafish (Danio rerio). Behav Ecol. (2016) 28:94–100.
95. Manuel R, Gorissen M, Piza Roca C, Zethof J, van de Vis H, Flik G. Inhibitory avoidance learning in zebrafish (Danio rerio): effects of shock intensity and unraveling differences in task performance. Zebrafish. (2014) 11:341–52. doi: 10.1089/zeb.2013.0970
96. Amorim RR, Silva PF, Luchiari AC. Effects of alcohol on inhibitory avoidance learning in zebrafish (Danio rerio). Zebrafish. (2017) 14:430–7.
97. Manuel R, Gorissen M, Stokkermans M, Zethof J, Ebbesson LO, van de Vis H. The effects of environmental enrichment and age-related differences on inhibitory avoidance in zebrafish (Danio rerio Hamilton). Zebrafish. (2015) 12:152–65. doi: 10.1089/zeb.2014.1045
98. Manuel R, Zethof J, Flik G, van den Bos R. Providing a food reward reduces inhibitory avoidance learning in zebrafish. Behav Process. (2015) 120:69–72. doi: 10.1016/j.beproc.2015.08.013
99. Kalueff AV, Gebhardt M, Stewart AM, Cachat JM, Brimmer M, Chawla JS, et al. Towards a comprehensive catalog of zebrafish behavior 1.0 and beyond. Zebrafish. (2013) 10:70–86. doi: 10.1089/zeb.2012.0861
100. Way GP, Ruhl N, Snekser JL, Kiesel AL, McRobert SP. A comparison of methodologies to test aggression in zebrafish. Zebrafish. (2015) 12:144–51.
101. Woodward MA, Winder LA, Watt PJ. Enrichment increases aggression in zebrafish. Fishes. (2019) 4:22.
102. Liu C-X, Li C-Y, Hu C-C, Wang Y, Lin J, Jiang Y-H. CRISPR/Cas9-induced shank3b mutant zebrafish display autism-like behaviors. Mol Autism. (2018) 9:23. doi: 10.1186/s13229-018-0204-x
103. Nabinger DD, Altenhofen S, Peixoto JV, da Silva JMK, Bonan CD. Long-lasting behavioral effects of quinpirole exposure on zebrafish. Neurotoxicol Teratol. (2021) 88:107034. doi: 10.1016/j.ntt.2021.107034
104. Riehl R, Kyzar E, Allain A, Green J, Hook M, Monnig L, et al. Behavioral and physiological effects of acute ketamine exposure in adult zebrafish. Neurotoxicol Teratol. (2011) 33:658–67. doi: 10.1016/j.ntt.2011.05.011
105. Cachat J, Kyzar EJ, Collins C, Gaikwad S, Green J, Roth A, et al. Unique and potent effects of acute ibogaine on zebrafish: the developing utility of novel aquatic models for hallucinogenic drug research. Behav Brain Res. (2013) 236:258–69. doi: 10.1016/j.bbr.2012.08.041
106. Cleal M, Parker MO. Moderate developmental alcohol exposure reduces repetitive alternation in a zebrafish model of fetal alcohol spectrum disorders. Neurotoxicol Teratol. (2018) 70:1–9. doi: 10.1016/j.ntt.2018.09.001
107. Howe K, Clark MD, Torroja CF, Torrance J, Berthelot C, Muffato M, et al. The zebrafish reference genome sequence and its relationship to the human genome. Nature. (2013) 496:498–503. doi: 10.1038/nature12111
108. Bradford YM, Toro S, Ramachandran S, Ruzicka L, Howe DG, Eagle A, et al. Zebrafish models of human disease: gaining insight into human disease at ZFIN. ILAR J. (2017) 58:4–16. doi: 10.1093/ilar/ilw040
109. Rea V, Van Raay TJ. Using zebrafish to model autism spectrum disorder: a comparison of ASD risk genes between zebrafish and their mammalian counterparts. Front Mol Neurosci. (2020) 13:575575. doi: 10.3389/fnmol.2020.575575
110. Vasileiou G, Ekici AB, Uebe S, Zweier C, Hoyer J, Engels H, et al. Chromatin-remodeling-factor ARID1B represses Wnt/β-catenin signaling. Am J Hum Genet. (2015) 97:445–56. doi: 10.1016/j.ajhg.2015.08.002
111. Liu X, Hu G, Ye J, Ye B, Shen N, Tao Y, et al. De Novo ARID1B mutations cause growth delay associated with aberrant Wnt/β–catenin signaling. Hum Mutat. (2020) 41:1012–24. doi: 10.1002/humu.23990
112. Nishiyama M, Skoultchi AI, Nakayama KI. Histone H1 recruitment by CHD8 is essential for suppression of the Wnt–β-catenin signaling pathway. Mol Cell Biol. (2012) 32:501–12. doi: 10.1128/MCB.06409-11
113. Sugathan A, Biagioli M, Golzio C, Erdin S, Blumenthal I, Manavalan P, et al. CHD8 regulates neurodevelopmental pathways associated with autism spectrum disorder in neural progenitors. Proc Natl Acad Sci. (2014) 111:E4468–77.
114. Bernier R, Golzio C, Xiong B, Stessman HA, Coe BP, Penn O, et al. Disruptive CHD8 mutations define a subtype of autism early in development. Cell. (2014) 158:263–76.
115. Yang T, Zhao H, Lu C, Li X, Xie Y, Fu H, et al. Synaptic plasticity, a prominent contributor to the anxiety in fragile X syndrome. Neural Plast. (2016) 2016:9353929. doi: 10.1155/2016/9353929
116. Tucker B, Richards RI, Lardelli M. Contribution of mGluR and Fmr1 functional pathways to neurite morphogenesis, craniofacial development and fragile X syndrome. Hum Mol Genet. (2006) 15:3446–58. doi: 10.1093/hmg/ddl422
117. Ng M-C, Yang Y-L, Lu K-T. Behavioral and synaptic circuit features in a zebrafish model of fragile X syndrome. PLoS One. (2013) 8:e51456. doi: 10.1371/journal.pone.0051456
118. Sharifi O, Yasui DH. The molecular functions of MeCP2 in rett syndrome pathology. Front Genet. (2021) 12:624290. doi: 10.3389/fgene.2021.624290
119. Pietri T, Roman A-C, Guyon N, Romano SA, Washbourne P, Moens CB, et al. The first mecp2-null zebrafish model shows altered motor behaviors. Front Neural Circuits. (2013) 7:118. doi: 10.3389/fncir.2013.00118
120. Chen C-Y, Chen J, He L, Stiles BL. PTEN: tumor suppressor and metabolic regulator. Front Endocrinol. (2018) 9:338. doi: 10.3389/fendo.2018.00338
121. Backman SA, Stambolic V, Mak TW. PTEN function in mammalian cell size regulation. Curr Opin Neurobiol. (2002) 12:516–22.
122. Croushore JA, Blasiole B, Riddle RC, Thisse C, Thisse B, Canfield VA, et al. Ptena and ptenb genes play distinct roles in zebrafish embryogenesis. Dev Dyn. (2005) 234:911–21. doi: 10.1002/dvdy.20576
123. Choorapoikayil S, Weijts B, Kers R, de Bruin A, den Hertog J. Loss of Pten promotes angiogenesis and enhanced vegfaa expression in zebrafish. Dis Model Mech. (2013) 6:1159–66. doi: 10.1242/dmm.012377
124. Hoffman EJ, Turner KJ, Fernandez JM, Cifuentes D, Ghosh M, Ijaz S, et al. Estrogens suppress a behavioral phenotype in zebrafish mutants of the autism risk gene, CNTNAP2. Neuron. (2016) 89:725–33. doi: 10.1016/j.neuron.2015.12.039
125. Martin-de-Saavedra MD, dos Santos M, Varea O, Spielman BP, Gao R, Forrest M, et al. CNTNAP2 ectodomain, detected in neuronal and CSF sheddomes, modulates Ca2+ dynamics and network synchrony. bioRxiv. [Preprint]. (2019). doi: 10.1101/605378
126. Duchon A, Herault Y. DYRK1A, a dosage-sensitive gene involved in neurodevelopmental disorders, is a target for drug development in Down syndrome. Front Behav Neurosci. (2016) 10:104. doi: 10.3389/fnbeh.2016.00104
127. Ricceri L, Michetti C, Scattoni ML. Mouse behavior and models for autism spectrum disorders. In: Sala C, Verpelli C editors. Neuronal and Synaptic Dysfunction in Autism Spectrum Disorder and Intellectual Disability. Amsterdam: Elsevier (2016). p. 269–93.
128. Rissone A, Foglia E, Sangiorgio L, Cermenati S, Nicoli S, Cimbro S, et al. The synaptic proteins β-neurexin and neuroligin synergize with extracellular matrix-binding vascular endothelial growth factor a during zebrafish vascular development. Arterioscler Thromb Vasc Biol. (2012) 32:1563–72. doi: 10.1161/ATVBAHA.111.243006
129. Sanders SJ, Campbell AJ, Cottrell JR, Moller RS, Wagner FF, Auldridge AL, et al. Progress in understanding and treating SCN2A-mediated disorders. Trends Neurosci. (2018) 41:442–56. doi: 10.1016/j.tins.2018.03.011
130. Baraban SC, Dinday MT, Hortopan GA. Drug screening in Scn1a zebrafish mutant identifies clemizole as a potential Dravet syndrome treatment. Nat Commun. (2013) 4:2410.
131. Uchino S, Waga C. SHANK3 as an autism spectrum disorder-associated gene. Brain Dev. (2013) 35:106–10.
132. James DM, Kozol RA, Kajiwara Y, Wahl AL, Storrs EC, Buxbaum JD, et al. Intestinal dysmotility in a zebrafish (Danio rerio) shank3a; shank3b mutant model of autism. Mol Autism. (2019) 10:3. doi: 10.1186/s13229-018-0250-4
133. Gamache TR, Araki Y, Huganir RL. Twenty years of SynGAP research: from synapses to cognition. J Neurosci. (2020) 40:1596–605. doi: 10.1523/JNEUROSCI.0420-19.2020
134. Kozol RA, Cukier HN, Zou B, Mayo V, De Rubeis S, Cai G, et al. Two knockdown models of the autism genes SYNGAP1 and SHANK3 in zebrafish produce similar behavioral phenotypes associated with embryonic disruptions of brain morphogenesis. Hum Mol Genet. (2015) 24:4006–23. doi: 10.1093/hmg/ddv138
135. de Bruijn E, Cuppen E, Feitsma H. Highly efficient ENU mutagenesis in zebrafish. Methods Mol Biol. (2009) 546:3–12.
136. den Broeder MJ, van der Linde H, Brouwer JR, Oostra BA, Willemsen R, Ketting RF. Generation and characterization of FMR1 knockout zebrafish. PLoS One. (2009) 4:e7910. doi: 10.1371/journal.pone.0007910
137. Miller AC, Voelker LH, Shah AN, Moens CB. Neurobeachin is required postsynaptically for electrical and chemical synapse formation. Curr Biol. (2015) 25:16–28. doi: 10.1016/j.cub.2014.10.071
138. Kim L, He L, Maaswinkel H, Zhu L, Sirotkin H, Weng W. Anxiety, hyperactivity and stereotypy in a zebrafish model of fragile X syndrome and autism spectrum disorder. Prog Neuropsychopharmacol Biol Psychiatry. (2014) 55:40–9. doi: 10.1016/j.pnpbp.2014.03.007
139. Wu Y-J, Hsu M-T, Ng M-C, Amstislavskaya TG, Tikhonova MA, Yang Y-L, et al. Fragile X mental retardation-1 knockout zebrafish shows precocious development in social behavior. Zebrafish. (2017) 14:438–43. doi: 10.1089/zeb.2017.1446
140. Hu J, Chen L, Yin J, Yin H, Huang Y, Tian J. Hyperactivity, memory defects, and craniofacial abnormalities in zebrafish fmr1 mutant larvae. Behav Genet. (2020) 50:152–60. doi: 10.1007/s10519-020-09995-7
141. Leong WY, Lim ZH, Korzh V, Pietri T, Goh EL. Methyl-CpG binding protein 2 (Mecp2) regulates sensory function through Sema5b and Robo2. Front Cell Neurosci. (2015) 9:481. doi: 10.3389/fncel.2015.00481
142. Wienholds E, Van Eeden F, Kosters M, Mudde J, Plasterk RH, Cuppen E. Efficient target-selected mutagenesis in zebrafish. Genome Res. (2003) 13:2700–7.
143. Moens CB, Donn TM, Wolf-Saxon ER, Ma TP. Reverse genetics in zebrafish by TILLING. Brief Funct Genomics Proteomic. (2008) 7:454–9.
144. Dahlem TJ, Hoshijima K, Jurynec MJ, Gunther D, Starker CG, Locke AS, et al. Simple methods for generating and detecting locus-specific mutations induced with TALENs in the zebrafish genome. PLoS Genet. (2012) 8:e1002861. doi: 10.1371/journal.pgen.1002861
145. Doyon Y, McCammon JM, Miller JC, Faraji F, Ngo C, Katibah GE, et al. Heritable targeted gene disruption in zebrafish using designed zinc-finger nucleases. Nat Biotechnol. (2008) 26:702–8.
146. Meng X, Noyes MB, Zhu LJ, Lawson ND, Wolfe SA. Targeted gene inactivation in zebrafish using engineered zinc-finger nucleases. Nat Biotechnol. (2008) 26:695–701.
147. Sander JD, Cade L, Khayter C, Reyon D, Peterson RT, Joung JK, et al. Targeted gene disruption in somatic zebrafish cells using engineered TALENs. Nat Biotechnol. (2011) 29:697–8. doi: 10.1038/nbt.1934
148. Gupta RM, Musunuru K. Expanding the genetic editing tool kit: ZFNs, TALENs, and CRISPR-Cas9. J Clin Invest. (2014) 124:4154–61. doi: 10.1172/JCI72992
149. Hwang WY, Peterson RT, Yeh J-RJ. Methods for targeted mutagenesis in zebrafish using TALENs. Methods. (2014) 69:76–84.
150. Kim O-H, Cho H-J, Han E, Hong TI, Ariyasiri K, Choi J-H, et al. Zebrafish knockout of Down syndrome gene, DYRK1A, shows social impairments relevant to autism. Mol Autism. (2017) 8:50. doi: 10.1186/s13229-017-0168-2
151. Ribeiro D, Nunes AR, Gliksberg M, Anbalagan S, Levkowitz G, Oliveira RF. Oxytocin receptor signalling modulates novelty recognition but not social preference in zebrafish. J Neuroendocrinol. (2020) 32:e12834. doi: 10.1111/jne.12834
152. Cornet C, Di Donato V, Terriente J. Combining zebrafish and CRISPR/Cas9: toward a more efficient drug discovery pipeline. Front Pharmacol. (2018) 9:703. doi: 10.3389/fphar.2018.00703
153. Irion U, Krauss J, Nüsslein-Volhard C. Precise and efficient genome editing in zebrafish using the CRISPR/Cas9 system. Development. (2014) 141:4827–30.
154. Ruzzo EK, Pérez-Cano L, Jung J-Y, Wang L-K, Kashef-Haghighi D, Hartl C, et al. Inherited and de novo genetic risk for autism impacts shared networks. Cell. (2019) 178:850–866.e26.
155. Bill BR, Petzold AM, Clark KJ, Schimmenti LA, Ekker SC. A primer for morpholino use in zebrafish. Zebrafish. (2009) 6:69–77.
156. Draper BW, Morcos PA, Kimmel CB. Inhibition of zebrafish fgf8 pre-mRNA splicing with morpholino oligos: a quantifiable method for gene knockdown. Genesis. (2001) 30:154–6. doi: 10.1002/gene.1053
157. Nasevicius A, Ekker SC. Effective targeted gene ‘knockdown’ in zebrafish. Nat Genet. (2000) 26:216–20.
158. Ishibashi M, Manning E, Shoubridge C, Krecsmarik M, Hawkins TA, Giacomotto J, et al. Copy number variants in patients with intellectual disability affect the regulation of ARX transcription factor gene. Hum Genet. (2015) 134:1163–82. doi: 10.1007/s00439-015-1594-x
159. Oksenberg N, Stevison L, Wall JD, Ahituv N. Function and regulation of AUTS2, a gene implicated in autism and human evolution. PLoS Genet. (2013) 9:e1003221. doi: 10.1371/journal.pgen.1003221
160. Sicca F, Ambrosini E, Marchese M, Sforna L, Servettini I, Valvo G, et al. Gain-of-function defects of astrocytic Kir4.1 channels in children with autism spectrum disorders and epilepsy. Sci Rep. (2016) 6:34325.
161. Patowary A, Won SY, Oh SJ, Nesbitt RR, Archer M, Nickerson D, et al. Family-based exome sequencing and case-control analysis implicate CEP41 as an ASD gene. Transl Psychiatry. (2019) 9:4. doi: 10.1038/s41398-018-0343-z
162. Turner TN, Sharma K, Oh EC, Liu YP, Collins RL, Sosa MX, et al. Loss of δ-catenin function in severe autism. Nature. (2015) 520:51–6. doi: 10.1038/nature14186
163. Kok Fatma O, Shin M, Ni C-W, Gupta A, Grosse Ann S, van Impel A, et al. Reverse genetic screening reveals poor correlation between morpholino-induced and mutant phenotypes in zebrafish. Dev Cell. (2015) 32:97–108. doi: 10.1016/j.devcel.2014.11.018
164. Lawson ND. Reverse genetics in Zebrafish: mutants, morphants, and moving forward. Trends Cell Biol. (2016) 26:77–9. doi: 10.1016/j.tcb.2015.11.005
165. Eisen JS, Smith JC. Controlling morpholino experiments: don’t stop making antisense. Development. (2008) 135:1735–43. doi: 10.1242/dev.001115
166. Robu ME, Larson JD, Nasevicius A, Beiraghi S, Brenner C, Farber SA, et al. p53 activation by knockdown technologies. PLoS Genet. (2007) 3:e78. doi: 10.1371/journal.pgen.0030078
167. Stainier DYR, Raz E, Lawson ND, Ekker SC, Burdine RD, Eisen JS, et al. Guidelines for morpholino use in zebrafish. PLoS Genet. (2017) 13:e1007000. doi: 10.1371/journal.pgen.1007000
168. Joseph TP, Zhou F, Sai LY, Chen H, Lin SL, Schachner M. Duloxetine ameliorates valproic acid−induced hyperactivity, anxiety−like behavior, and social interaction deficits in zebrafish. Autism Res. (2021) 15:27–41. doi: 10.1002/aur.2620
169. Torres-Hernández BA, Valle-Mojica D, Lisa M, Ortíz JG. Valerenic acid and Valeriana officinalis extracts delay onset of Pentylenetetrazole (PTZ)-Induced seizures in adult Danio rerio (Zebrafish). BMC Comp Altern Med. (2015) 15:228. doi: 10.1186/s12906-015-0731-3
170. Cachat JM, Canavello PR, Elegante MF, Bartels BK, Elkhayat SI, Hart PC, et al. Modeling stress and anxiety in zebrafish. In: Kalueff AV, Cachat J editors. Zebrafish Models in Neurobehavioral Research. Berlin: Springer (2011). p. 73–88.
171. Stewart A, Wu N, Cachat J, Hart P, Gaikwad S, Wong K, et al. Pharmacological modulation of anxiety-like phenotypes in adult zebrafish behavioral models. Prog Neuropsychopharmacol Biol Psychiatry. (2011) 35:1421–31.
172. Audira G, Sampurna BP, Juniardi S, Liang S-T, Lai Y-H, Hsiao C-D. A versatile setup for measuring multiple behavior endpoints in zebrafish. Inventions. (2018) 3:75.
173. Fontana BD, Alnassar N, Parker MO. The zebrafish (Danio rerio) anxiety test battery: comparison of behavioral responses in the novel tank diving and light–dark tasks following exposure to anxiogenic and anxiolytic compounds. Psychopharmacology. (2021) 239:287–96. doi: 10.1007/s00213-021-05990-w
174. Haghani S, Karia M, Cheng R-K, Mathuru AS. An automated assay system to study novel tank induced anxiety. Front Behav Neurosci. (2019) 13:180. doi: 10.3389/fnbeh.2019.00180
175. Levin ED, Bencan Z, Cerutti DT. Anxiolytic effects of nicotine in zebrafish. Physiol Behav. (2007) 90:54–8.
176. James DM, Davidson EA, Yanes J, Moshiree B, Dallman JE. The gut-brain-microbiome axis and its link to autism: emerging insights and the potential of zebrafish models. Front Cell Dev Biol. (2021) 9:662916. doi: 10.3389/fcell.2021.662916
177. Chernikova MA, Flores GD, Kilroy E, Labus JS, Mayer EA, Aziz-Zadeh L. The brain-gut-microbiome system: pathways and implications for autism spectrum disorder. Nutrients. (2021) 13:4497.
178. Vuong HE, Hsiao EY. Gut microbes join the social network. Neuron. (2019) 101:196–8. doi: 10.1016/j.neuron.2018.12.035
179. Li Q, Han Y, Dy ABC, Hagerman RJ. The gut microbiota and autism spectrum disorders. Front Cell Neurosci. (2017) 11:120. doi: 10.3389/fncel.2017.00120
180. Lord C, Elsabbagh M, Baird G, Veenstra-Vanderweele J. Autism spectrum disorder. Lancet. (2018) 392:508–20.
181. Tayanloo-Beik A, Rabbani Z, Soveyzi F, Alavi-Moghadam S, Rezaei-Tavirani M, Goodarzi P, et al. Cellular therapy for treatment of spinal cord injury in Zebrafish model. Mol Biol Rep. (2021) 48:1787–800.
182. Pasupuleti MK, Molahally SS, Salwaji S. Ethical guidelines, animal profile, various animal models used in periodontal research with alternatives and future perspectives. J Indian Soc Periodontol. (2016) 20:360. doi: 10.4103/0972-124X.186931
183. Larijani B, Hamidpour SK, Tayanloo-Beik A, Shahbazbadr A, Yavari H, Namazi N, et al. An overview of zebrafish modeling methods in drug discovery and development. Adv Exp Med Biol. (2021). [Epub ahead of print]. doi: 10.1007/5584_2021_684
184. Aghayan HR, Hosseini MS, Gholami M, Mohamadi-Jahani F, Tayanloo-Beik A, Alavi-Moghadam S, et al. Mesenchymal stem cells’ seeded amniotic membrane as a tissue-engineered dressing for wound healing. Drug Deliv Transl Res. (2022) 12:538–49.
185. Song D-Y, Kim SY, Bong G, Kim JM, Yoo HJ. The use of artificial intelligence in screening and diagnosis of autism spectrum disorder: a literature review. J Korean Acad Child Adolesc Psychiatry. (2019) 30:145.
186. Chen T, Chen Y, Yuan M, Gerstein M, Li T, Liang H, et al. The development of a practical artificial intelligence tool for diagnosing and evaluating autism spectrum disorder: multicenter study. JMIR Med Inform. (2020) 8:e15767. doi: 10.2196/15767
187. Shahamiri SR, Thabtah F. Autism AI: a new autism screening system based on Artificial Intelligence. Cogn Comput. (2020) 12:766–77.
188. Simeoli R, Milano N, Rega A, Marocco D. Using technology to identify children with autism through motor abnormalities. Front Psychol. (2021) 12:635696. doi: 10.3389/fpsyg.2021.635696
189. Oliveira JS, Franco FO, Revers MC, Silva AF, Portolese J, Brentani H, et al. Computer-aided autism diagnosis based on visual attention models using eye tracking. Sci Rep. (2021) 11:10131. doi: 10.1038/s41598-021-89023-8
Keywords: autism spectrum disorders, animal modeling, Danio rerio, drug development, drug discovery, zebrafish
Citation: Tayanloo-Beik A, Hamidpour SK, Abedi M, Shojaei H, Tavirani MR, Namazi N, Larijani B and Arjmand B (2022) Zebrafish Modeling of Autism Spectrum Disorders, Current Status and Future Prospective. Front. Psychiatry 13:911770. doi: 10.3389/fpsyt.2022.911770
Received: 06 April 2022; Accepted: 22 June 2022;
Published: 14 July 2022.
Edited by:
Roberto Canitano, Siena University Hospital, ItalyReviewed by:
Melanie P. Leussis, Emmanuel College, United StatesStefano Musardo, Université de Genève, Switzerland
Copyright © 2022 Tayanloo-Beik, Hamidpour, Abedi, Shojaei, Tavirani, Namazi, Larijani and Arjmand. This is an open-access article distributed under the terms of the Creative Commons Attribution License (CC BY). The use, distribution or reproduction in other forums is permitted, provided the original author(s) and the copyright owner(s) are credited and that the original publication in this journal is cited, in accordance with accepted academic practice. No use, distribution or reproduction is permitted which does not comply with these terms.
*Correspondence: Babak Arjmand, barjmand@sina.tums.ac.ir
†ORCID: Akram Tayanloo-Beik, orcid.org/0000-0001-8370-9557; Mostafa Rezaei Tavirani, orcid.org/0000-0003-1767-7475; Nazli Namazi, orcid.org/0000-0002-3119-2805; Bagher Larijani, orcid.org/0000-0001-5386-7597; Babak Arjmand, orcid.org/0000-0001-5001-5006