- 1Department of Biomedical Sciences of Cells and Systems, University Medical Center Groningen/University of Groningen, Groningen, Netherlands
- 2Department of Medical Microbiology and Infection Prevention, University Medical Center Groningen/University of Groningen, Groningen, Netherlands
- 3Winclove Probiotics, Amsterdam, Netherlands
- 4Alzheimer Center Groningen, University Medical Center Groningen, Groningen, Netherlands
- 5Department of Neurology, University Medical Center Groningen, Groningen, Netherlands
- 6Department of Internal Medicine, University Medical Center Groningen, Groningen, Netherlands
Alzheimer's disease (AD) is a global public health priority as with aging populations, its prevalence is expected to rise even further in the future. The brain and gut are in close communication through immunological, nervous and hormonal routes, and therefore, probiotics are examined as an option to influence AD hallmarks, such as plaques, tangles, and low grade inflammation. This study aimed to provide an overview of the available animal evidence on the effect of different probiotics on gut microbiota composition, short chain fatty acids (SCFAs), inflammatory markers, Amyloid-β (Aβ), and cognitive functioning in AD animal models. A systematic literature search was performed in PubMed, SCOPUS, and APA PsychInfo. Articles were included up to May 2021. Inclusion criteria included a controlled animal study on probiotic supplementation and at least one of the abovementioned outcome variables. Of the eighteen studies, most were conducted in AD male mice models (n = 9). Probiotics of the genera Lactobacillus and Bifidobacterium were used most frequently. Probiotic administration increased species richness and/or bacterial richness in the gut microbiota, increased SCFAs levels, reduced inflammatory markers, and improved cognitive functioning in AD models in multiple studies. The effect of probiotic administration on Aβ remains ambiguous. B. longum (NK46), C. butyricum, and the mixture SLAB51 are the most promising probiotics, as positive improvements were found on almost all outcomes. The results of this animal review underline the potential of probiotic therapy as a treatment option in AD.
Introduction
Alzheimer's disease (AD) is the most common form of dementia and worldwide an estimated 44 million people suffer from AD (1). The global prevalence is expected to rise in the future due to aging populations. AD is therefore a global public health priority (2). Key symptoms of AD include cognitive decline, behavioral changes, and inability to perform ordinary tasks. AD is associated with decreased quality of life and limited daily functioning (3, 4). The cause of AD is still unknown, although insights into the molecular pathology do exist. One of the pathological hallmarks of AD is the formation of plaques in the brain, consisting of the peptide amyloid-beta (Aβ) (5). Aβ is also produced in a healthy brain. In the case of AD, however, the production or decreased removal of Aβ results in the formation of plaques which negatively affects the communication between brain cells (5). Also, oligomeric Aβ negatively affects the function and structure of synapses (6). Synaptic markers are found to predict cognitive functioning in AD (7). Another pathological hallmark of AD is the formation of neurofibrillary tangles in the brain, consisting of highly phosphorylated tau protein (8). These fibrillary inclusions are reported to be accountable for neuronal cell death (8).
The microbiome consists of a diverse ecosystem of microorganisms, including bacteria, fungi, protozoa, viruses, and all their genes and functions, in contrast to the microbiota which only comprises living microbes (9). The amount and diversity of the microbiota gradually decrease with age (10, 11). At all ages, variability and heterogeneity of the gut microbiota differs largely between persons due to extrinsic factors (e.g., diet, antibiotics, lifestyle, or disease) and intrinsic factors (e.g., genetics) (9, 10, 12). In general, high species diversity is interpreted as a sign of a healthy microbiome. The human microbiome has been linked to multiple aspects of human health and disease, including AD (13–15). There is a bidirectional relationship between the brain and the gut, which is also known as the “gut-brain axis” (GBA) (16). The GBA is bidirectional in the sense that the gut microbiota signals to the brain and the brain signals to the gut microbiota (17). The brain and gut communicate via neural processing of the central nervous system and the enteric nervous system (17). The GBA connects the emotional and cognitive parts of the brain with peripheral intestinal functions and mechanisms, such as immune activation, intestinal permeability, enteric reflex, and entero-endocrine signaling (17). One way by which the gut microbiota exerts its effects is through the production of metabolites, such as short chain fatty acids (SCFAs) and branched-chain amino acids, and bacterial fragments, such as peptidoglycans, that reach the brain via the circulation (10). Therefore, the GBA is sometimes also referred to as the microbiota-gut-brain axis (9, 18).
Recent studies have focused on the role of the gut microbiota in several brain disorders, including autism, anxiety, schizophrenia, Parkinson's disease, and AD (10). When focusing on AD, emerging evidence hypothesizes that gut dysbiosis is suggested to stimulate the aggregation of Aβ, neuroinflammation, oxidative stress, and insulin resistance (19). However, a causal relation between gut dysbiosis and neural dysfunction remains elusive until now (19). On a group level, the gut microbiome of people with AD was different compared to healthy age- and sex-matched individuals (14, 20, 21). For instance, Vogt et al. (14) found that the gut microbiome of people with AD was less diverse. Several studies comparing AD patients to healthy controls reported a reduction in the gut microbiome in the phylum Firmicutes and in the genus Bifidobacterium (B.); and an increase in Bacteroidetes and Proteobacteria, more specifically the phylum Enterobacteriaceae (14, 20). Additionally, a correlation was found for genera that were more abundant in AD compared to controls, with CSF markers of AD pathology (14). Liu et al. (20) also found a significant correlation between clinical severity scores of people with AD and altered microbiomes. For instance, a negative association was found between Mini-Mental State Examination (MMSE) and Montreal Cognitive Assessment (MoCA) scores and the phylum Proteobacteria, its class Gammaproteobacteria, and the family Enterobacteriaceae (P < 0.05) and between MoCA scores and Veillonellaceae (P < 0.05) (20). In contrast, a positive association was found between Clinical Dementia Rating and the family Enterobacteriaceae (P < 0.05), and between MMSE scores and Bacteroidetes and Ruminococcaceae (P < 0.05) (20). In addition, the intestinal barrier and the blood-brain barrier (BBB) may both play an important role in the pathogenesis of AD. The permeability of both barriers increases with age (22). This increased permeability, as well as damage to the intestinal barrier and BBB caused by gut dysbiosis, may facilitate the entry of pathogens into the blood and brain (23). These pathogens can theoretically enter the brain through the damaged BBB and can worsen neuroinflammation and induce amyloid aggregation, which is a primitive immune reaction (23). Consequently, increased intestinal permeability and microbial dysbiosis also trigger systemic inflammation within the body, for example, by increasing serum interleukin 6 (IL-6) levels (23). Elevated levels of IL-6 are also found in serum and brain tissue of people with AD (24). Systemic inflammation in AD is also argued to induce proinflammatory states of microglia and astrocytic phenotypes, which stimulate tau hyperphosphorylation, Aβ oligomerization, component activation, and the breakdown of neurotransmitters into potentially toxic metabolites (25).
Probiotics are products that deliver live microorganisms with a suitable viable count of well-defined strains with a reasonable expectation of delivering benefits for the host's wellbeing (26). Health benefits have been demonstrated for several probiotic strains, including Lactobacillus (L.), and Bifidobacterium (B.) (27). It should be noted that the efficacy of probiotics is strain- and disease-specific (28), hence many types of bacteria could be considered probiotics under the right conditions. The benefits of probiotics occur in the GI tract by influencing the intestinal microbiota and the introduction of beneficial functions to the microbiota, which could result in the prevention or amelioration of gut inflammation or other systemic disease phenotypes (29). Besides the positive influences on the human gut microbiota, probiotics can regulate neurotransmitters and growth factors, such as gamma-aminobutyric acid, serotonin, glutamate, and brain-derived neurotrophic factor (30, 31). Moreover, the gut microbiota is not only important for the intestinal permeability, but also for the production of SCFAs (32). SCFAs that are produced through probiotics are suggested to induce a decrease in pro-inflammatory cytokines, due to their immunomodulatory effects (32, 33). For example, SCFAs provide anti-inflammatory effects in the intestinal mucosa through the inhibition of histone deacetylases and the activation of cell surface G-protein coupled receptors in intestinal epithelial cells and immune cells (34). Two recent meta-analyses investigated the effect of probiotic supplementation in patients with AD or mild cognitive impairment. One study reported improvement in cognitive functioning with the use of probiotics, and it was hypothesized that this was due to the decrease in levels of inflammatory and oxidative biomarkers (35). The other study looked at the effectiveness of probiotic supplementation on cognitive functioning in people with dementia and found no beneficial effect of probiotic supplementation on cognitive functioning in patients with AD, with very low evidence certainty (36). Both analyses included only three RCTs on AD, of which one used co-supplementation with selenium, which precludes any firm conclusions on the potential benefits of probiotics for AD.
Several findings have emerged from animal studies that used different models of AD, which may provide information that could be translated to a clinical application. A systematic review on animal evidence can provide an overview, which may help to find an optimal (mix of) probiotic(s) to further test in AD patients in clinical trials. Moreover, more insights into the potential underlying mechanism can be provided by investigating the effect of probiotics on multiple outcome variables related to AD and/or the microbiota-gut-brain-axis. This systematic review aims to provide an overview of the available animal evidence on the effect of different strains of probiotics on gut microbiota composition, SCFAs, inflammatory markers, Aβ, and cognitive functioning in models of AD.
Methods
Search Strategy
This study was performed according to the Preferred Reporting for Systematic Reviews and Meta-analysis (PRISMA) (37). A systematic literature search was performed in PubMed, SCOPUS, and APA PsycInfo. Articles were included up to May 2021. Combinations of the following keywords were used: “probiotics” and “Alzheimer's disease.” These words were added to the search query together with synonyms or MeSH terms. Duplicates have been removed from the final study selection. After selecting relevant titles and abstracts selection, full-text articles were assessed. A PRISMA flow chart is used to graphically display the final selection of articles (see Figure 1).
Inclusion and Exclusion Criteria
Full-text articles were considered eligible if they met the following criteria: (1) the article is peer-reviewed, (2) the article is written in English, (3) a controlled study was conducted on probiotic supplementation, (4) the study uses an animal model of AD, and (5) at least one of the following outcome measures was addressed: cognitive functioning, gut microbiota composition, Aβ deposition, inflammatory markers, and SCFAs. Besides gut microbiota composition, gut permeability or other gut health markers were not included due to a lack of available data. The exclusion criterium was when a study uses mixtures of probiotics with other nutrients or interventions, so that the effect of probiotics cannot be distinguished.
Outcome Measures
The outcome measures of this study included gut microbiota composition, SCFAs, inflammatory markers, Aβ, and cognitive functioning. Gut microbiota composition encompassed species richness, microbial abundance, and microbiome diversity. Inflammatory markers comprised biomarkers that reflect the pro- or anti-inflammatory status, assessed either in blood or CSF. Finally, cognitive functioning was defined as mental abilities, including learning, thinking, reasoning, remembering, problem solving, decision making, and attention (38).
Data Extraction
Data extraction was done on the following items: publication year, country, study sample characteristics of the intervention and control group (e.g., animal model; control group; sample size; sex), intervention characteristics (e.g., type, duration, and dosage of probiotics; type and duration and dosage of control condition), the operationalization of the outcome measures, and results. The extracted data were compared and analyzed for similarities and differences.
Quality Assessment
The risk of bias was assessed with SYRCLE (39), because this tool was specifically designed to assess the methodological quality of animal intervention studies. SYRCLE is based on the widely used Cochrane Collaboration Risk of Bias tool and was adapted to aspects of bias that specifically play a role in animal research (39).
Ten entries of potential biases in the included studies were reviewed, which are related to selection bias, performance bias, detection bias, attrition bias, reporting bias and other biases. Each potential bias was evaluated by answering SYRCLE's signaling questions with “yes” indicating a low risk of bias, “no” indicating a high risk of bias, and “no information” indicating an unclear risk of bias (39). A summary score was calculated based on the number of yeses compared to the total number of SYRCLE items.
Results
Search Results
The systematic literature search resulted in 633 studies from which 65 duplicates were removed. The title and abstract of 568 articles were screened for in- and exclusion criteria. In total, 24 articles were read in full-text. Out of these, six articles were excluded (40–45), because they were inaccessible, animals were supplemented with mixtures of probiotics with other nutrients, or had a different focus (glucose uptake, rough eye phenotypes, DNA, oxidation and SIRT1), leaving eighteen articles for analysis. The final PRISMA flow chart can be found in Figure 1.
Quality Assessment
In total, 18 articles were assessed for methodological quality by means of the SYRCLE tool (see Table 1). Although the SYRCLE score does not have an overall quality score, most articles scored a four, five, or six out of ten, which reflects a moderate quality. Two remarkable results can be distinguished: Ou et al. (46) had a relatively high methodological quality with a score of eight out of ten, whereas Lee et al. (62) had a relatively low methodological quality with a score of three out of ten. Most studies had incomplete descriptions of the methodology, resulting in an unclear risk of bias. The high frequency of an unclear risk of bias was especially true for items that measure selection bias, performance bias, detection bias, and attrition bias.
Study Characteristics
An overview of the study characteristics can be found in Table 2. All articles were published between 2017 and 2021. Most studies were conducted in mice models of AD (n = 14; mostly APP/PS1), and four studies used AD models in Wistar rats. Only two studies used female animals, compared to thirteen studies using male animals and three studies did not provide a description on the sex of the animals.
Regarding the type of probiotics, lactobacilli and bifidobacteria were frequently used (i.e., L. plantarum, B. bifidum, and B. longum). Ten studies used a single-strain probiotic, compared to eight studies using a mixture. Two studies used the mixture VSL#3, which consists of L. plantarum, L. delbrueckii subsp. Bulgaricus, L. paracasei, L. acidophilus, B. breve B. longum, B. infantis, and Streptococcus salivarius subsp. thermophilus. One study used SLAB51, which is a probiotic mixture consisting of: Streptococcus thermophilus (DSM 32245), B. lactis (DSM 32246), B. lactis (DSM 32247), L. acidophilus (DSM 32241), L. helveticus (DSM 32242), L. paracasei (DSM 32243), L. plantarum (DSM 32244), and L. brevis (DSM 27961). Another study used the mixture Optibac, which consists of L. acidophilus and L. rhamnosus. An overview of the other mixtures can be seen in Table 2. Study durations ranged from 4 weeks to 6 months. In fifteen studies, probiotics were administered orally, while in three studies probiotics were administered intragastrically.
Overall Effects of Probiotics
The majority of studies investigated the effect of probiotics on multiple outcome variables. Two studies looked at all the included outcome variables, whereas one study only looked at one outcome variable (see Table 3). In all studies, probiotics influenced at least one outcome variable of AD. Probiotic administration affected gut microbiota composition (n = 13 studies), SCFAs (n = 4 studies), inflammatory markers (n = 7 studies), and cognitive functioning (n = 12 studies). For Aβ, conflicting results were found, as some (n = 10 studies) found positive effects, whereas others (n = 5 studies) found no effect or no significant effect compared to AD control animals.
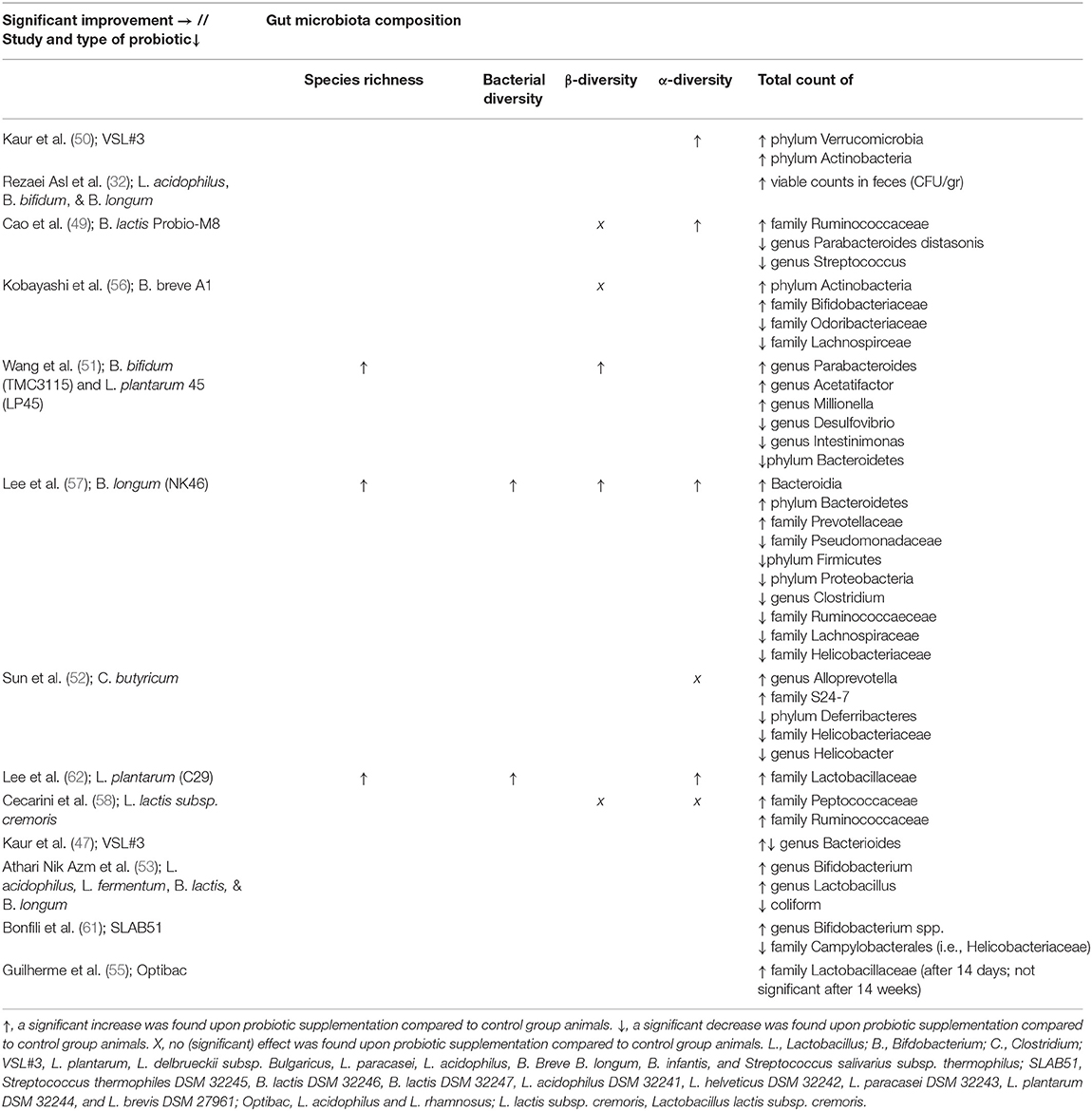
Table 3. Overview of results per study and probiotic strain on cognitive functioning, gut microbiota composition, Aβ, inflammatory markers, or SCFAs in AD animals compared to AD animals without probiotic administration.
Probiotics and Gut Microbiota Composition
Thirteen studies looked at the effect of probiotics on gut microbiota composition, of which eight looked at microbiome richness and/or diversity (see Tables 3, 4). An improvement in both species richness (51, 57, 62) and bacterial diversity (57, 62) was found compared to control group animals. More specifically, a significant difference in α-diversity was found in four studies (49, 50, 57, 62), which was not replicated by Sun et al. (52) and Cecarini et al. (58). Similarly, two studies (51, 57) found a significant increase in β-diversity compared to AD control animals, whereas three others did not (49, 56, 58).
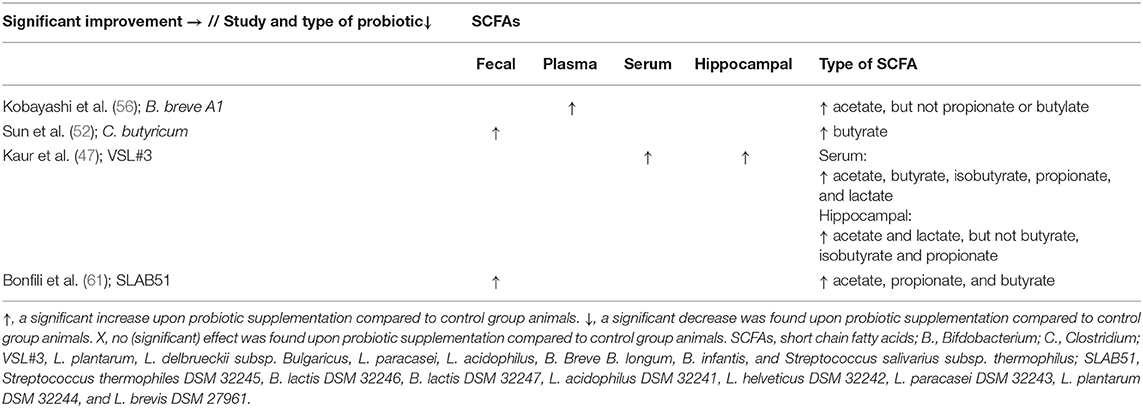
Table 4. Overview of results per study and probiotic strain on gut microbiota composition in AD animals compared to AD animals without probiotic administration.
An increase in certain bacterial phyla was observed upon probiotic administration (i.e., Actinobacteria, Verrucomicrobia, Bacteroidetes) (50, 56). At the family level, an increase in Peptococcaceae, Ruminococcaceae, Prevotellaceae, S24-7, and Lactobacillaceae was found compared to control group animals (49, 52, 55–58, 62). An increase in Acetatifactor, Millionella, Alloprevotella, Parabacteroides, Bifidobacterium, Lactobacillus and Bacteroidales was observed compared to AD controls at the genus level (47, 51–53, 61). In contrast in other studies, a decrease at the phylum level was found for Firmicutes, Bacteroidetes, Proteobacteria, and Deferribacteres compared to control animals (49, 51, 52, 57). Also, at the family level, a decrease was observed for Odorbacteraceae, Lachnospiraceae, Helicobacteraceae, Ruminococaceae, and Pseudomonadaceae compared to AD controls (52, 56, 57, 61). A decrease compared to AD control animals was also found for Parabacteroides, Streptococcus, Desulfovibrio, Bacteroidales, Intestinimonas, Clostridium, and Helicobacter at the genus level (47, 49, 51, 52, 57). At the phylum level, no change in the overall Firmicutes/Bacteroidetes ratio was found (50).
When investigating the different probiotics, all included probiotics showed at least one effect on the gut microbiota composition (see Tables 3, 4).
Probiotics and SCFAs
Only four out of 18 studies addressed the effect of probiotics on SCFA levels, in which an improvement in plasma, serum, fecal, and hippocampal SCFA levels was observed compared to AD control animals (see Tables 3, 5) (47, 52, 56, 61).
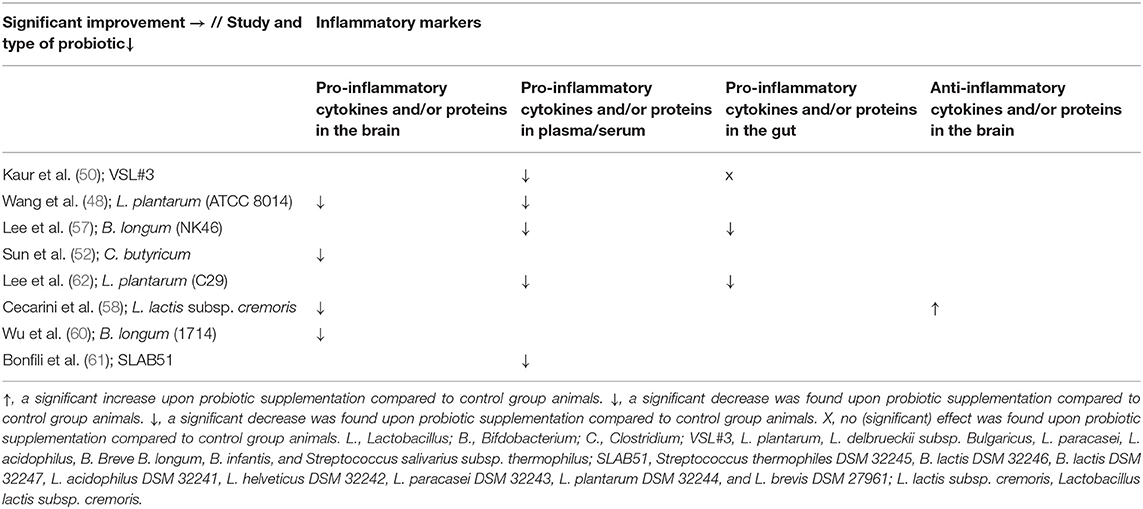
Table 5. Overview of results per study and probiotic strain on SCFAs in AD animals compared to AD animals without probiotic administration.
A study by Kaur et al. (47) reported increased serum and hippocampal SCFAs compared to AD control animals. An increase in plasma acetate levels was found (56), as well as an increase in total fecal butyrate levels compared to AD control animals (52). Furthermore, an increase in fecal levels of acetic, propionic, and butyric acid was observed (61).
When looking at the different strains of probiotics, a significant increase in SCFA levels was observed upon administration with B. breve A1, the mixture VSL#3, the mixture SLAB51, and C. butyricum (see Tables 3, 5).
Probiotics and Inflammatory Markers
Eight studies investigated the effect of probiotics on relevant inflammatory markers for AD (50–52, 57, 58, 60–62) (see Tables 3, 6). Seven studies found a reduction in proinflammatory markers compared to control group animals. One study found a partial effect: a significant reduction in levels of proinflammatory cytokines in the ileum was found, as well as a significant improvement in serum eicosanoid levels compared to control group animals (50). In contrast, the same study found no significant effect on proinflammatory cytokines or Lipocalin-2 levels in the brain and no significant effect on protein levels in the ileum compared to AD controls (50).
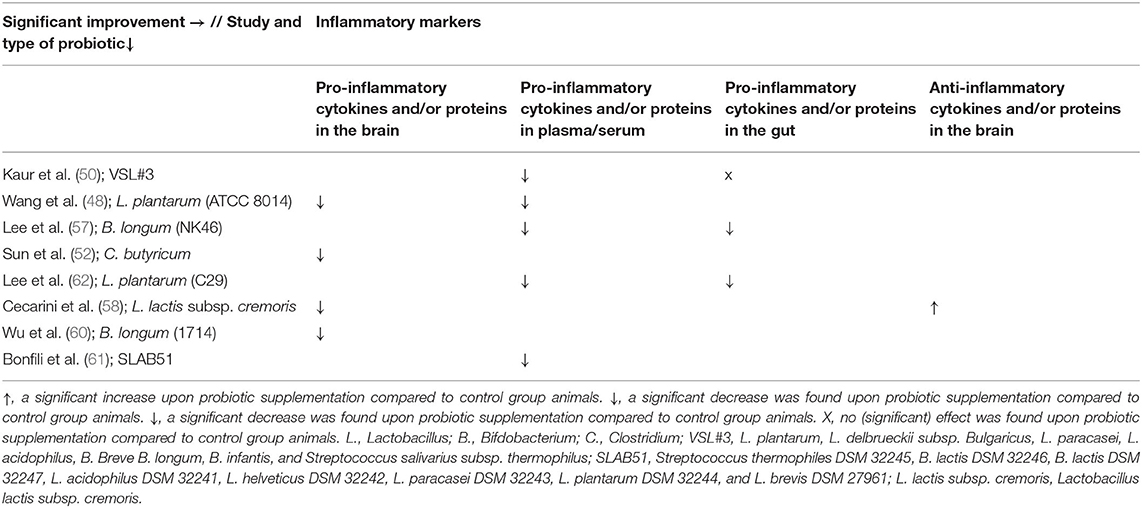
Table 6. Overview of results per study and probiotic strain on inflammatory markers in AD animals compared to AD animals without probiotic administration.
Several studies found a reduction in proinflammatory cytokines in the brain (hippocampus), and blood (plasma) compared to AD control animals, such as IL-1α, IL-2, IL-6, IL-4, IL-12, IL-17, IL-1β, INF-γ, and TNF-α (51, 52, 57, 58, 60–62). Likewise, a decrease in serum eicosanoids, plasma lipopolysaccharide (LPS) levels, and plasma clusterin concentrations was found (50, 51, 57). Also, an increase in the anti-inflammatory cytokine IL-10 was observed in the brain compared to control group animals (58). Kaur et al. (50) did not find a decrease in proinflammatory proteins or protein levels of proinflammatory cytokines in the brain and ileum compared to AD control animals. When looking more specifically at gut inflammation, a reduction in TNF-α in the colon was reported (62). Cyclo-oxygenase 2 expression in the colon, and nuclear factor kappa-light-chain-enhancer of activated B cells (NF-lb.) activation in the colon and in microglial BV-2 cells were found to be decreased compared to control group animals (57, 62).
Most included probiotics showed at least one significant effect on inflammatory markers (see Tables 3, 6). No significant results were found after administration with the mixture VSL#3. Significant improvements were found upon administration with: L. plantarum (ATCC8014), L. plantarum (C29), B. longum (NK46), B. longum (1714), the mixture SLAB51, L. lactis subsp. cremoris, and C. butyricum.
Probiotics and Aβ
A total of 15 studies assessed the effect of probiotics on Aβ parameters (see Tables 3, 7). A reduction in the amount of Aβ plaques in the brain (46, 49, 51, 53), as well as the size of Aβ plaques in the brain (53), Aβ deposition in the brain (46, 52, 60, 61) and the Aβ expression in the hippocampus (57, 62) was found compared to control group animals. More specifically, a decrease in brain levels of Aβ(1-42) (52, 58, 61) and Aβ(1-40) (58) was observed in four studies compared to control group animals. Contrastingly, three studies did not find any effect in the brain (50) and hippocampus (52, 55), or no significant effect in the brain (32, 59) upon probiotic administration on Aβ. Similarly, another study also found no differences in the brain after probiotic administration compared to control group animals for Aβ(1-40) (61).
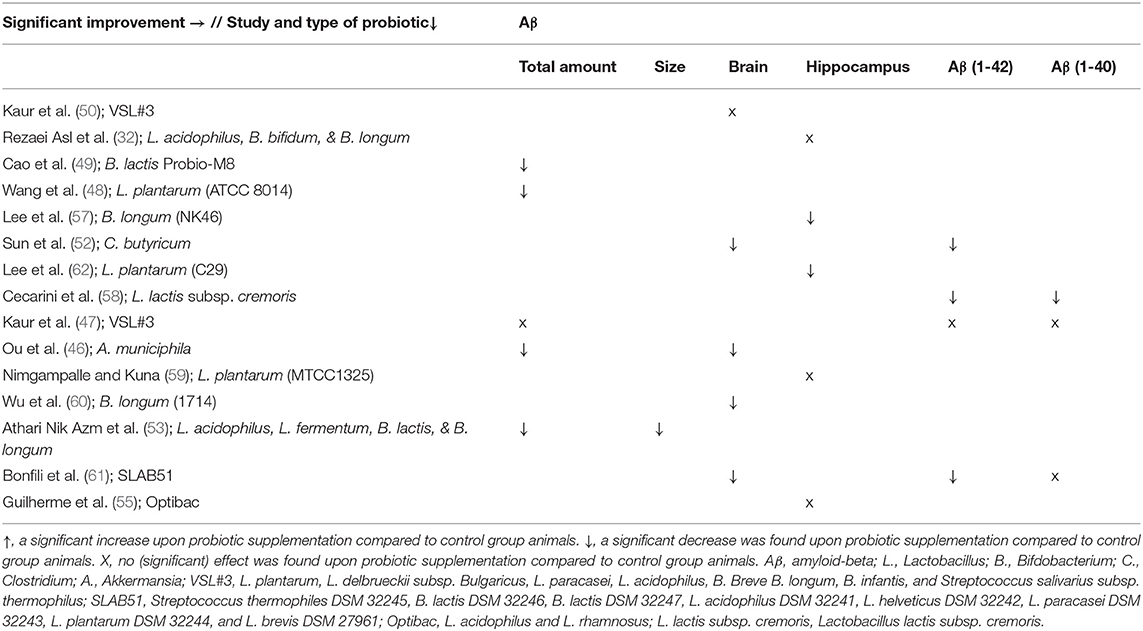
Table 7. Overview of results per study and probiotic strain on Aβ in AD animals compared to AD animals without probiotic administration.
When looking at the different strains of probiotics, no effect regarding Aβ was found upon administration with L. plantarum (MTCC1325); the mixture VSL#3; L. acidophilus, B. bifidum & B. longum; and Optibac (see Tables 3, 7). Significant effects were observed upon administration with L. plantarum (ATCC8014); L. plantarum (C29); B. longum (NK46); B. longum (1714); B. lactis Probio-M8; Akkermansia municiphila; the mixture SLAB51; L. lactis subsp. cremoris; C. butyricum; and L. acidophilus, L. fermentum, B. lactis & B. longum.
Probiotics and Cognitive Functioning
Fifteen studies investigated the effect of probiotics on cognitive functioning, of which twelve studies found at least one improvement in cognitive functioning compared to the control groups (see Tables 3, 8) (32, 46, 48–54, 56–59, 61, 62). As shown in Table 3, not all tests performed found an improvement (e.g., only in one out of three tests). Six studies found an improvement for all tests, three of which used more than one test.
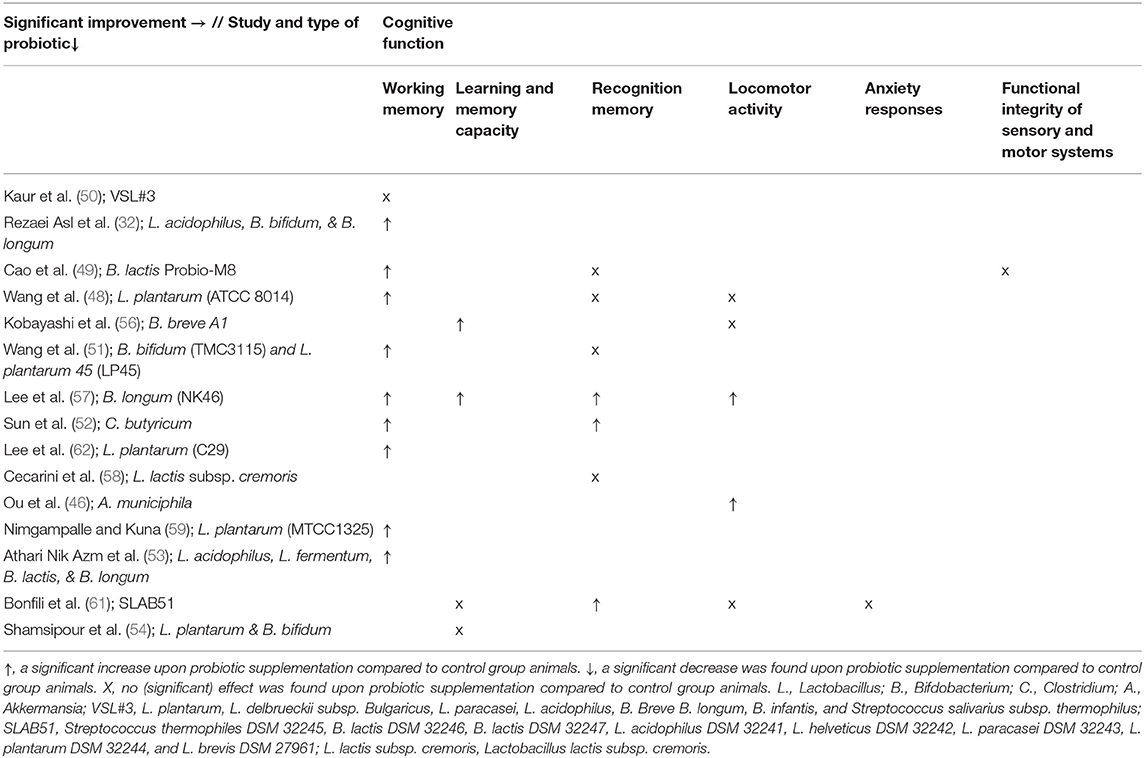
Table 8. Overview of results per study and probiotic strain on cognitive functioning in AD animals compared to AD animals without probiotic administration.
Improvements in spatial working memory, as assessed by the Morris Water Maze test, were found after probiotic supplementation compared to control group animals in all studies (32, 48, 51–53, 57, 59, 62). However, a probe trial test, part of the Morris Water Maze test that measures how long the test subject spends in the target quadrant, did not show significant differences between AD rats with probiotic supplementation and control rats (32). Learning and memory capacity, as measured by the Passive Avoidance Test, improved in two studies compared to control group animals (56, 57), whereas it did not improve in another study (61). Inconsistent results were also found for the Novel Object Recognition Test, which measures recognition memory: an improvement was observed in three studies (52, 57, 61), while four other studies did not find any differences compared to AD controls (48, 49, 51, 58). Improvements (46, 57), as well as no difference (56), were found for locomotor activity assessed via the Y-maze test compared to AD control animals. Similarly, the Open-Field test, which measures general locomotor activity, improved compared to AD controls in one study (46), which was not replicated in two other studies (48, 61). No differences compared to AD controls could be assessed for the Elevated Plus Maze Test, which measures anxiety responses (61).
When looking at different strains of probiotics, no effect on cognitive functioning was found upon administration with VSL#3, L. lactis subsp. cremoris, and L. plantarum & B. bifidum (see Tables 3, 8). Significant effects were observed upon administration with B. breve A1; L. plantarum (ATCC8014); L. plantarum (C29); L. plantarum (MTCC1325); B. longum (NK46); L. acidophilus, B. bifidum & B. longum; B. lactis Probio-M8; Akkermansia municiphila; SLAB51; Clostridium butyricum (C. butyricum); and L. acidophilus, L. fermentum, B. lactis & B. longum.
Discussion
Potential Mechanisms to Explain the Effects of Probiotics in AD
AD pathology has been associated with alterations in the gut microbiota composition and GI inflammation (13, 50, 63, 64). It can be argued that increased gut permeability allows increased concentrations of LPS in the gut and in the circulation, which in turn can trigger amyloid secretion in the gut, while amyloid secretion in the gut can exacerbate the intestinal permeability (46, 65, 66). This process results in increased production and translocation of cytokines and pro-inflammatory components of GI origin into the body (46, 65, 66). These inflammatory compounds could not only increase systemic inflammation, but could also cross the BBB and may induce neuroinflammation, amyloid secretion, neuronal injury, dysfunction of specific brain regions, the development of insulin resistance and ultimately lead to neuronal death in AD (46, 65, 67–70). Some animal studies reported a significant decrease in GI inflammation and attenuated intestinal permeability upon probiotic administration in AD model animals (50, 62). Another study argued that probiotic administration could ameliorate cognitive decline by means of a reduction of gut microbiota LPS production and the regulation of microbiota LPS-mediated NF-κB activation in BV-2 cells, a type of microglial cells (57). From a clinical perspective, a recent meta-analysis summarizing human RCTs found a significant improvement in cognition, as well as a significant reduction in high-sensitivity C-reactive protein levels in the probiotics group compared to the control group (35), supporting the idea that probiotics can reduce systemic inflammation. Overall, these findings suggest that probiotic supplementation suppresses the downward spiral of GI inflammation, altered gut microbiota composition, increased gut permeability, translocation of pro-inflammatory compounds through the BBB, and potential adverse inflammatory and metabolic processes in the brain.
Another underlying mechanism might be SCFA production of the metabolites SCFAs by the gut microbiome. Some metabolites, like SCFAs, can pass through the BBB directly, induce a decrease in pro-inflammatory cytokines in the brain, and modulate the maturation of microglia (32, 52, 56, 71). Additionally, SCFAs are found to interfere with protein-protein interactions, which are necessary for the formation of toxic soluble Aβ aggregates/converse Aβ peptides into Aβ neurotoxic aggregates (72). This interference capability is especially true for valeric acid, butyric acid, and propionic acid (72). This way, SCFAs may help to alleviate elements of the pathophysiological processes of AD.
This review found that probiotic administration in AD rodent models increased plasma, fecal, and hippocampal SCFA levels, reduced inflammatory markers in the blood and brain, and improved cognitive functioning in multiple studies, which is consistent with the hypotheses mentioned above.
B. longum (NK46), C. butyricum, and the Mixture SLAB51
B. longum (NK46), C. butyricum, and the mixture SLAB51 seem to be the most promising probiotics for AD, as they have shown the most positive outcomes. B. longum is an anaerobic, non-halophilic, gram-positive bacterium that is naturally present in the human GI tract (73). B. longum is considered safe by the EFSA (74). Although multiple health benefits have been found upon B. longum administration, such as diarrhea prevention in antibiotic treated patients, and immune stimulation (73), it is unclear why this probiotic is effective for AD hallmarks in animal studies. A possible rationale is that B. longum (NK46) may stimulate the production of butyrate. Another hypothesis is that B. longum is associated with reduced intestinal inflammation and improved epithelial barrier integrity, and therefore decreases the passage of pro-inflammatory compounds through the BBB and reduces other potential adverse metabolic processes (50). B. longum is able to decrease gut microbiota LPS production as well as regulate LPS-induced NF-κB activation in microglial BV-2 cells in AD mice (57).
C. butyricum is an anaerobic, gram-positive, spore-forming bacteria that is common in the human colon (75). It has various implications for human health, ranging from pathogenic to beneficial, such as inducing botulism in infants and helping to overcome antibiotic-associated diarrhea in children (76, 77). Furthermore, C. butyricum is known for its ability to produce large amounts of SCFAs, such as butyrate and acetate (75). It is hypothesized that this high production of SCFAs is the reason for the beneficial health effects of C. butyricum in rodent studies. In Asia, C. butyricum is frequently used as a probiotic (75). However, C. butyricum is not yet on the QPS safety list of the EFSA (74). A recent animal study showed a neuroprotective effect of C. butyricum in mouse models of traumatic brain injury, partially due to increased secretion of glucagon-like peptide 1 (GLP-1), a 30-amino acid peptide hormone, through the GBA (78). Stoeva et al. (79) hypothesize that C. butyricum stimulates the secretion of GLP-1, which protects the BBB, potentially via the modulation of tight junctions. Likewise, C. butyricum is found to prevent brain endothelial barrier dysfunction, as demonstrated by decreased brain water content and the restoration of normal levels of tight junction protein expression (79). Furthermore, significant improvements in neurological dysfunction, brain edema, neurodegeneration, and BBB impairment were observed after C. butyricum administration (78). Moreover, C. butyricum decreased plasma-d lactate and colonic IL-6, whilst protecting the intestinal barrier integrity and upregulating the expression of occludin (78). Another animal study, which looked at vascular dementia in mice, found that C. butyricum significantly ameliorated cognitive dysfunction and histopathological changes, increased brain-derived neurotrophic factor (BDNF) and Bcl-2 levels, which are cell survival proteins that inhibit apoptosis (80), decreased Bax levels, which is part of the Bcl-2 family (81), decreased p-Akt levels, which is phospholyrated protein kinase B, and reduced neuronal apoptosis (79, 82). C. butyricum was also found to restore butyrate levels in feces and brain and regulate the gut microbiota in mouse models of vascular dementia (82). When looking at mouse models of AD, administration of C. butyricum prevented cognitive impairment, Aβ deposits, microglia activation, and production of TNF-α and IL-1β in the brain of AD mice (52). Also, C. butyricum treatment reversed abnormal gut microbiota and butyrate (52). More specifically, butyrate treatment was found to reduce CD11b and Cyclo-oxygenase 2 levels, and suppress the phosphorylation of NF-κB p65 in the Aβ-induced BV2 microglia (52). However, in this research, only one study investigated the effect of C. butyricum on AD mice, meaning that no firm conclusions can be drawn upon it yet.
Like mentioned before, SLAB51 is a probiotic mixture consisting of: Streptococcus thermophilus (DSM 32245), B. lactis (DSM 32246), B. lactis (DSM 32247), L. acidophilus (DSM 32241), L. helveticus (DSM 32242), L. paracasei (DSM 32243), L. plantarum (DSM 32244), and L. brevis (DSM 27961). All probiotics, except B. lactis, are considered safe by the EFSA (74). An animal study in AD mice found that the SLAB51 mixture significantly decreased oxidative stress in AD mice brains by activating Sirtuin 1 (SIRT1)-dependent mechanisms (45). SIRT1 is a protein family that is used during the cellular response to inflammatory, metabolic, and oxidative stressors (83). These proteins play a role in NAD* dependent deacetylation of histones, as well as in neuronal plasticity, cognitive function, and neuronal degeneration (84). SIRT1 levels were found to be lower in serum samples of patients with AD or MCI compared to age matched controls (85). Additionally, beneficial antioxidant effects were found in the brain of AD mice after SLAB51 administration (45). Another study in AD mice found that the mixture SLAB51 ameliorated the impaired glucose metabolism in AD by restoring the brain levels of glucose transporters GLUT3 and GLUT1, ameliorating brain glucose homeostasis, reducing tau phosphorylation by modulating pAMPK and pAkt, and decreasing advanced glycation end products (43). Because of this, the authors argue that amelioration of the impaired glucose metabolism in AD is able to delay AD progression through gut microbiota manipulation with SLAB51 (43). From a broader perspective, the SLAB51 mixture is also considered a promising candidate for the prevention or (coadjuvant) treatment of Parkinson's disease, as the mixture was able to protect dopaminergic neurons and improve behavioral impairments in in-vivo studies (86). Also, the mixture SLAB51 counteracted neuroinflammation and oxidative stress in both in-vivo and in-vitro studies (86). Moreover, the mixture SLAB51 modulated the BDNF pathway, increased neuroprotective protein levels, and decreased neuronal death proteins in the in-vitro studies (86).
No clinical trials have been found that investigated the effect of B. longum (NK46), C. butyricum, or the mixture SLAB51 in AD or MCI patients yet. From a broader perspective, two clinical trials investigated a mixture containing bifidobacteria and lactobacilli in AD patients and found significant improvements in cognitive functioning and some metabolic parameters (87, 88). One of these clinical trials used co-supplementation with selenium. In contrast, a recent meta-analysis, that included these two studies and an RCT in patients with severe AD (89), found no effect on cognitive function in AD patients upon probiotic supplementation, which all consisted of lactobacilli and bifidobacteria (36).
Combined Interventions
Although in this review we looked exclusively to interventions with only probiotics, in the future it may be wise to look broader than probiotics alone, since the effects on AD hallmarks appear to be stronger when probiotic supplementation is combined with another intervention. This insight may imply that probiotics work synergistically with other interventions. For instance, a stronger effect was found upon probiotic administration together with memantine (1 mg/ml), which is an AD drug classified as an N-Methyl-D-aspartic acid receptor antagonist, exercise, L. plantarum-fermented soybean, and p62-transformed L. lactis compared to probiotics alone (51, 54, 58, 62). Taking this chain of thought further, this review focused exclusively on probiotics due to the lack of animal studies on prebiotics. Prebiotics are substrates (often carbohydrates) that are selectively utilized by host microorganisms that confer a health benefit. There are multiple types of prebiotics that are suggested to benefit the microbiota (90–92). Prebiotics metabolized by the gut microbiota to SCFAs are, like probiotics, suggested to slow down AD progression due to their effect on the intestinal microbiota (33, 72). One animal AD study, investigating the prebiotic effects of fructooligosaccharides, found that these specific fructooligosaccharides could, among other things, improve oxidative stress and inflammation, regulate the synthesis and secretion of neurotransmitters, positively affect the diversity and stability of the microbiome of AD rats, and down-regulate the expression of both tau and Aβ1-42 (93). From a broader perspective, healthy dietary patterns characterized by high levels of prebiotics and probiotics, in association with other nutrients, are found to delay cognitive decline and decrease the risk of AD (66, 94).
Translation to Clinical Randomized Controlled Trials
This review is conducted from an animal perspective. Therefore, results from this study cannot be one-on-one translated into the clinic. This is not only due to the dissimilarity of AD animal models compared to AD patients, but also due to confounders in animal experiments, such as environmental factors and host genetic background (95). This review is also based on studies that used a variety of AD models, which could have introduced some bias by potentially affecting the composition of the gut microbiota differently (95). In addition, both the human microbiome (96) and AD characteristics (97) are sex-specific, and thus, women and men may benefit differently from probiotic augmentation. Most animal studies used male rodents and separate studies are needed to investigate females.
In this review, four studies used AD rat models (32, 53, 54, 59). Three of these studies used a mixture of bifidobacteria and lactobacilli strains, while one study used L. plantarum. Interestingly, the findings are relatively in line with the results of mice studies in the sense that significant evidence can be found for improvement on both cognitive functioning and gut microbiota composition, whereas more ambiguous results can be found for Aβ levels.
To go from animal to clinical trials, information on preliminary efficacy, toxicity, safety, and pharmacokinetics is needed. Research should look into the optimal duration of probiotic supplementation in AD, as well as the ability of probiotics to survive passage through the human GI tract. For future clinical trials, it is recommended to use oral administration (i.e., in the form of a sachet or pill), as this is minimally invasive for patients.
Limitations, Strengths and Recommendations for Future Research
Some limitations of this review must be mentioned. First, the included studies used a variety of animal models, as well as a variety of cognitive tests. These cognitive tests assess a slightly different part of cognitive functioning, which negatively influences the internal validity of this review. Second, as assessed in Chapter 3.2, only one study (46) has a high methodological quality. Therefore, the general poor methodological quality may have negatively influenced the results of this study. These findings support the statement that the reporting of experimental details on animals, methods and materials in animal studies is often poor (98). Third, the included studies used relatively small intervention groups and mostly male mice, which both negatively affect the external validity of the results. This is unfortunate as sex and gender differences exist both in the human form of AD and in the human microbiome. Furthermore, most of the included studies were conducted over a period of 2 months. Therefore, long-term conclusions cannot be drawn. Also, specific probiotics were only tested in a single study, and no direct comparisons between probiotics have been made so far. Lastly, publication bias may have caused an underreporting of studies where no effects were found. A strength of this review is that this review provides an in-depth overview of all animal studies on AD on probiotic strain level, whilst taking multiple outcome variables into account.
Future research should investigate the clinical effects of probiotic supplementation on AD symptoms and hallmarks. B. longum (NK46), C. butyricum and the mixture SLAB51 are promising types of probiotics to test in clinical trials. B. longum is considered safe for human consumption by the EFSA (74). It is advised to use oral administration. It is also argued that AD drugs can potentially have a negative effect on the gut microbiota whilst temporarily alleviating the symptoms of AD (e.g., cognitive improvement, reduced inflammation, or a reduction of Aβ and tau proteins) (94). Others argue that the combined administration of prebiotics, probiotics, and treatment could prevent or alleviate gut problems, which may potentially strengthen the efficacy of AD drugs by eliminating a possible factor that sustains the disease (94). This combination may be especially relevant to investigate in AD patients who are currently unresponsive to pharmacological treatment (94).
Conclusion
In conclusion, this review shows that probiotic administration in AD rodent models increased species richness and/or bacterial diversity of the gut microbiota, increased SCFA levels, reduced inflammatory markers, and improved cognitive functioning in multiple studies. The effect of probiotic administration on Aβ remains ambiguous. B. longum (NK46), C. butyricum, and the mixture SLAB51 are the most promising probiotics, as positive improvements were found on almost all outcomes. B. longum is considered safe for human consumption by the EFSA. A drawback is that each of these probiotics was tested in only one study. It would be helpful when the findings can be repeated by other groups and/or in other models. Taking all studies into account, this animal review underscores the potential of probiotic therapy as a treatment option in AD and this topic warrants animal and clinical follow-up.
Data Availability Statement
The original contributions presented in the study are included in the article/supplementary material, further inquiries can be directed to the corresponding author/s.
Author Contributions
TR designed the study and performed the literature search, screening, data extraction, data analysis, and manuscript writing. SH, PD, BM, HH, MD, and IS contributed to the manuscript writing. All authors have approved the final version of this manuscript and contributed to the process of making arguments within the manuscript.
Funding
This research was part of the project No Guts No Glory. This project was supported via an anniversary grant of the Dutch Brain Foundation (Hersenstichting) Grant No. 94648.
Conflict of Interest
SH was employee of Winclove Probiotics. Winclove produces, markets and investigates probiotics. HH and Winclove receive collective research grants.
The remaining authors declare that the research was conducted in the absence of any commercial or financial relationships that could be construed as a potential conflict of interest.
Publisher's Note
All claims expressed in this article are solely those of the authors and do not necessarily represent those of their affiliated organizations, or those of the publisher, the editors and the reviewers. Any product that may be evaluated in this article, or claim that may be made by its manufacturer, is not guaranteed or endorsed by the publisher.
Acknowledgments
We would like to thank Astrid Doorduijn and Wiesje van der Flier for supervising the master thesis of TR, which formed the basis for this article. Moreover, we would like to thank Lianne Remie and Winni Schalkwijk for their valuable feedback.
Abbreviations
Aβ, amyloid beta; AD, Alzheimer's disease; BBB, blood-brain barrier; Bcl-2, B-cell lymphoma 2; BDNF, brain-derived neurotrophic factor; ELISA, enzyme-linked immunosorbent assay; GBA, gut-brain axis; GI, gastrointestinal; IL, interleukin; INF-γ, interferon gamma; LPS, lipopolysaccharides; MoCa, Montreal Cognitive Assessment; MMSE, Mini-Mental State Examination; NF-κB, nuclear factor kappa-light-chain-enhancer of activated B cells; SCFA, short-chain fatty acid; TNF-α, tumor necrosis factor alpha.
References
1. Prince, MJ, Wimo, A, Guerchet, MM, Wu, Y-T, Prina, M,. World Alzheimer Report 2015- The Global Impact of Dementia. Available online at: https://www.alzint.org/u/WorldAlzheimerReport2015.pdf (accessed May 6, 2021).
2. Hill E, Goodwill AM, Gorelik A, Szoeke C. Diet and biomarkers of Alzheimer's disease: a systematic review and meta-analysis. Neurobiol Aging. (2019) 76:45–52. doi: 10.1016/j.neurobiolaging.2018.12.008
3. Shin IS, Carter M, Masterman D, Fairbanks L, Cummings JL. Neuropsychiatric symptoms and quality of life in Alzheimer disease. Am J Geriatr Psychiatry. (2005) 13:469–74. doi: 10.1097/00019442-200506000-00005
4. Razani J, Bayan S, Funes C, Mahmoud N, Torrence N, Wong J, et al. Patterns of deficits in daily functioning and cognitive performance of patients with Alzheimer disease. J Geriatr Psychiatry Neurol. (2011) 24:23–32. doi: 10.1177/0891988710390812
5. Mroczko B, Groblewska M, Litman-Zawadzka A, Kornhuber J, Lewczuk P. Cellular receptors of amyloid β Oligomers (AβOs) in Alzheimer's disease. Int J Mol Sci. (2018) 19:1884. doi: 10.3390/ijms19071884
6. Sheng M, Sabatini BL, Südhof TC. Synapses and Alzheimer's disease. Cold Spring Harb Perspect Biol. (2012) 4:a005777. doi: 10.1101/cshperspect.a005777
7. Bereczki E, Francis PT, Howlett D, Pereira JB, Höglund K, Bogstedt A, et al. Synaptic proteins predict cognitive decline in Alzheimer's disease and lewy body dementia. Alzheimers Dement. (2016) 12:1149–58. doi: 10.1016/j.jalz.2016.04.005
8. Kolarova M, García-Sierra F, Bartos A, Ricny J, Ripova D. Structure and pathology of tau protein in Alzheimer disease. Int J Alzheimers Dis. (2012) 2012:731526. doi: 10.1155/2012/731526
9. Morais LH, Schreiber HL4th, Mazmanian SK. The gut microbiota-brain axis in behaviour and brain disorders. Nat Rev Microbiol. (2021) 19:241–55. doi: 10.1038/s41579-020-00460-0
10. Cryan JF, O'Riordan KJ, Cowan CSM, Sandhu KV, Bastiaanssen TFS, Boehme M, et al. The Microbiota-Gut-Brain Axis. Physiol Rev. (2019) 99:1877–2013. doi: 10.1152/physrev.00018.2018
11. Claesson MJ, Cusack S, O'Sullivan O, Greene-Diniz R, de Weerd H, Flannery E, et al. Composition, variability, and temporal stability of the intestinal microbiota of the elderly. Proc Natl Acad Sci U S A. (2011) 108:4586–91. doi: 10.1073/pnas.1000097107
12. Liu YH, Gao X, Na M, Kris-Etherton PM, Mitchell DC, Jensen GL. Dietary pattern, diet quality, and dementia: a systematic review and meta-analysis of prospective cohort studies. J Alzheimers Dis. (2020) 78:151–68. doi: 10.3233/JAD-200499
13. Sochocka M, Donskow-Łysoniewska K, Diniz BS, Kurpas D, Brzozowska E, Leszek J. The gut microbiome alterations and inflammation-driven pathogenesis of alzheimer's disease-a critical review. Mol Neurobiol. (2019) 56:1841–51. doi: 10.1007/s12035-018-1188-4
14. Vogt NM, Kerby RL, Dill-McFarland KA, Harding SJ, Merluzzi AP, Johnson SC, et al. Gut microbiome alterations in Alzheimer's disease. Sci Rep. (2017) 7:13537. doi: 10.1038/s41598-017-13601-y
15. Askarova S, Umbayev B, Masoud AR, Kaiyrlykyzy A, Safarova Y, Tsoy A, et al. The links between the gut microbiome, aging, modern lifestyle and alzheimer's disease. Front Cell Infect Microbiol. (2020) 10:104. doi: 10.3389/fcimb.2020.00104
16. Sound R. The gut microbiota–brain axis and role of probiotics. In: Ghosh D, editor. Nutraceuticals in Brain Health and Beyond. London: Academic Press (2021). p. 175–91. doi: 10.1016/B978-0-12-820593-8.00013-6
17. Carabotti M, Scirocco A, Maselli MA, Severi C. The gut-brain axis: interactions between enteric microbiota, central and enteric nervous systems. Ann Gastroenterol. (2015) 28:203–9.
18. Chidambaram SB, Tuladhar S, Bhat A, Mahalakshmi AM, Ray B, Essa MM, et al. Autism and gut-brain axis: role of probiotics. Adv Neurobiol. (2020) 24:587–600. doi: 10.1007/978-3-030-30402-7_21
19. Liu S, Gao J, Zhu M, Liu K, Zhang HL. Gut microbiota and dysbiosis in alzheimer's disease: implications for pathogenesis and treatment. Mol Neurobiol. (2020) 57:5026–43. doi: 10.1007/s12035-020-02073-3
20. Liu P, Wu L, Peng G, Han Y, Tang R, Ge J, et al. Altered microbiomes distinguish Alzheimer's disease from amnestic mild cognitive impairment and health in a Chinese cohort. Brain Behav Immun. (2019) 80:633–43. doi: 10.1016/j.bbi.2019.05.008
21. Zhuang ZQ, Shen LL, Li WW, Fu X, Zeng F, Gui L, et al. Gut microbiota is altered in patients with Alzheimer's disease. J Alzheimers Dis. (2018) 63:1337–46. doi: 10.3233/JAD-180176
22. Erdo F, Denes L, de Lange E. Age-associated physiological and pathological changes at the blood-brain barrier: a review. J Cereb Blood Flow Metab. (2017) 37:4–24. doi: 10.1177/0271678X16679420
23. Li Z, Zhu H, Zhang L, Qin C. The intestinal microbiome and Alzheimer's disease: a review. Animal Model Exp Med. (2018) 1:180–8. doi: 10.1002/ame2.12033
24. Calsolaro V, Edison P. Neuroinflammation in Alzheimer's disease: current evidence and future directions. Alzheimers Dement. (2016) 12:719–32. doi: 10.1016/j.jalz.2016.02.010
25. Walker KA, Ficek BN, Westbrook R. Understanding the role of systemic inflammation in Alzheimer's disease. ACS Chem Neurosci. (2019) 10:3340–2. doi: 10.1021/acschemneuro.9b00333
26. Hill C, Guarner F, Reid G, Gibson GR, Merenstein DJ, Pot B, et al. Expert consensus document. the international scientific association for probiotics and prebiotics consensus statement on the scope and appropriate use of the term probiotic. Nat Rev Gastroenterol Hepatol. (2014) 11:506–14. doi: 10.1038/nrgastro.2014.66
27. Fijan S. Microorganisms with claimed probiotic properties: an overview of recent literature. Int J Environ Res Public Health. (2014) 11:4745–67. doi: 10.3390/ijerph110504745
28. Sniffen JC, McFarland LV, Evans CT, Goldstein EJC. Choosing an appropriate probiotic product for your patient: an evidence-based practical guide. PLoS ONE. (2018) 13:e0209205. doi: 10.1371/journal.pone.0209205
29. Hemarajata P, Versalovic J. Effects of probiotics on gut microbiota: mechanisms of intestinal immunomodulation and neuromodulation. Therap Adv Gastroenterol. (2013) 6:39–51. doi: 10.1177/1756283X12459294
30. Reid G, Sanders ME, Gaskins HR, Gibson GR, Mercenier A, Rastall R, et al. New scientific paradigms for probiotics and prebiotics. J Clin Gastroenterol. (2003) 37:105–18. doi: 10.1097/00004836-200308000-00004
31. Lu Y, Christian K, Lu B. BDNF a key regulator for protein synthesis-dependent LTP and long-term memory? Neurobiol Learn Mem. (2008) 89:312–23. doi: 10.1016/j.nlm.2007.08.018
32. Rezaei Asl Z, Sepehri G, Salami M. Probiotic treatment improves the impaired spatial cognitive performance and restores synaptic plasticity in an animal model of Alzheimer's disease. Behav Brain Res. (2019) 376:112183. doi: 10.1016/j.bbr.2019.112183
33. Markowiak-Kopeć P, Slizewska K. The effect of probiotics on the production of short-chain fatty acids by human intestinal microbiome. Nutrients. (2020) 12:1107. doi: 10.3390/nu12041107
34. Parada Venegas D, De la Fuente MK, Landskron G, González MJ, Quera R, Dijkstra G, et al. Short chain fatty acids (SCFAs)-mediated gut epithelial and immune regulation and its relevance for inflammatory bowel diseases. Front Immunol. (2019) 10:277. doi: 10.3389/fimmu.2019.01486
35. Den H, Dong X, Chen M, Zou Z. Efficacy of probiotics on cognition, and biomarkers of inflammation and oxidative stress in adults with Alzheimer's disease or mild cognitive impairment - a meta-analysis of randomized controlled trials. Aging. (2020) 12:4010–39. doi: 10.18632/aging.102810
36. Krüger JF, Hillesheim E, Pereira ACSN, Camargo CQ, Rabito EI. Probiotics for dementia: a systematic review and meta-analysis of randomized controlled trials. Nutr Rev. (2021) 79:160–70. doi: 10.1093/nutrit/nuaa037
37. Moher D, Liberati A, Tetzlaff J, Altman DG, PRISMA Group. Preferred reporting items for systematic reviews and meta-analyses: the PRISMA statement. PLoS Med. (2009) 6:e1000097. doi: 10.1371/journal.pmed.1000097
38. Fisher GG, Chacon M, Chaffee DS. Theories of cognitive aging and work. In: Baltes B, Rudolph CW, Zacher H, editors. Work Across the Lifespan. London: Academic Press (2019). p. 17–45. doi: 10.1016/B978-0-12-812756-8.00002-5
39. Hooijmans CR, Rovers MM, de Vries RB, Leenaars M, Ritskes-Hoitinga M, Langendam MW. SYRCLE's risk of bias tool for animal studies. BMC Med Res Methodol. (2014) 14:43. doi: 10.1186/1471-2288-14-43
40. Abraham D, Feher J, Scuderi GL, Szabo D, Dobolyi A, Cservenak M, et al. Exercise and probiotics attenuate the development of Alzheimer's disease in transgenic mice: role of microbiome. Exp Gerontol. (2019) 115:122–31. doi: 10.1016/j.exger.2018.12.005
41. Téglás T, Ábrahám D, Jókai M, Kondo S, Mohammadi R, Fehér J, et al. Exercise combined with a probiotics treatment alters the microbiome, but moderately affects signalling pathways in the liver of male APP/PS1 transgenic mice. Biogerontology. (2020) 21:807–15. doi: 10.1007/s10522-020-09895-7
42. Tan FHP, Liu G, Lau SA, Jaafar MH, Park YH, Azzam G, et al. Lactobacillus probiotics improved the gut microbiota profile of a drosophila melanogaster Alzheimer's disease model and alleviated neurodegeneration in the eye. Benef Microbes. (2020) 11:79–89. doi: 10.3920/BM2019.0086
43. Bonfili L, Cecarini V, Gogoi O, Berardi S, Scarpona S, Angeletti M, et al. Gut microbiota manipulation through probiotics oral administration restores glucose homeostasis in a mouse model of Alzheimer's disease. Neurobiol Aging. (2020) 87:35–43. doi: 10.1016/j.neurobiolaging.2019.11.004
44. Liu M, Hu R, Guo Y, Sun W, Li J, Fan M, et al. [Influence of Lactobacillus reuteri SL001 on intestinal microbiota in AD model mice and C57BL/6 mice]. Sheng Wu Gong Cheng Xue Bao. (2020) 36:1887–98. doi: 10.13345/j.cjb.200024
45. Bonfili L, Cecarini V, Cuccioloni M, Angeletti M, Berardi S, Scarpona S, et al. SLAB51 Probiotic formulation activates SIRT1 pathway promoting antioxidant and neuroprotective effects in an AD mouse model. Mol Neurobiol. (2018) 55:7987–8000. doi: 10.1007/s12035-018-0973-4
46. Ou Z, Deng L, Lu Z, Wu F, Liu W, Huang D, et al. Protective effects of Akkermansia muciniphila on cognitive deficits and amyloid pathology in a mouse model of Alzheimer's disease. Nutr Diabetes. (2020) 10:12. doi: 10.1038/s41387-020-0115-8
47. Kaur H, Golovko S, Golovko MY, Singh S, Darland DC, Combs CK. Effects of probiotic supplementation on short chain fatty acids in the Appnl-g-f mouse model of Alzheimer's disease. J Alzheimers Dis. (2020) 76:1083–102. doi: 10.3233/JAD-200436
48. Wang F, Xu T, Zhang Y, Zheng T, He Y, He F, et al. Long-term combined administration of Bifidobacterium bifidum TMC3115 and Lactobacillus plantarum 45 alleviates spatial memory impairment and gut dysbiosis in APP/PS1 mice. FEMS Microbiol Lett. (2020) 367:fnaa048. doi: 10.1093/femsle/fnaa048
49. Cao J, Amakye WK, Qi C, Liu X, Ma J, Ren J. Bifidobacterium Lactis Probio-M8 regulates gut microbiota to alleviate Alzheimer's disease in the APP/PS1 mouse model. Eur J Nutr. (2021) 60:3757–69. doi: 10.1007/s00394-021-02543-x
50. Kaur H, Nagamoto-Combs K, Golovko S, Golovko MY, Klug MG, Combs CK. Probiotics ameliorate intestinal pathophysiology in a mouse model of Alzheimer's disease. Neurobiol Aging. (2020) 92:114–34. doi: 10.1016/j.neurobiolaging.2020.04.009
51. Wang QJ, Shen YE, Wang X, Fu S, Zhang X, Zhang YN, et al. Concomitant memantine and Lactobacillus plantarum treatment attenuates cognitive impairments in APP/PS1 mice. Aging. (2020) 12:628–49. doi: 10.18632/aging.102645
52. Sun J, Xu J, Yang B, Chen K, Kong Y, Fang N, et al. Effect of clostridium butyricum against microglia-mediated neuroinflammation in Alzheimer's disease via regulating gut microbiota and metabolites butyrate. Mol Nutr Food Res. (2020) 64:e1900636. doi: 10.1002/mnfr.201900636
53. Athari Nik Azm S, Djazayeri A, Safa M, Azami K, Ahmadvand B, Sabbaghziarani F, et al. Lactobacilli and bifidobacteria ameliorate memory and learning deficits and oxidative stress in β-amyloid (1-42) injected rats. Appl Physiol Nutr Metab. (2018) 43:718–26. doi: 10.1139/apnm-2017-0648
54. Shamsipour S, Sharifi G, Taghian F. Impact of interval training with probiotic (L. plantarum / Bifidobacterium bifidum) on passive avoidance test, ChAT and BDNF in the hippocampus of rats with Alzheimer's disease. Neurosci Lett. (2021) 756:135949. doi: 10.1016/j.neulet.2021.135949
55. Guilherme MDS, Nguyen VTT, Reinhardt C, Endres K. Impact of gut microbiome manipulation in 5xFAD mice on Alzheimer's disease-like pathology. Microorganisms. (2021) 9:815. doi: 10.3390/microorganisms9040815
56. Kobayashi Y, Sugahara H, Shimada K, Mitsuyama E, Kuhara T, Yasuoka A, et al. Therapeutic potential of Bifidobacterium breve strain A1 for preventing cognitive impairment in Alzheimer's disease. Sci Rep. (2017) 7:13510. doi: 10.1038/s41598-017-13368-2
57. Lee HJ, Lee KE, Kim JK, Kim DH. Suppression of gut dysbiosis by Bifidobacterium longum alleviates cognitive decline in 5XFAD transgenic and aged mice. Sci Rep. (2019) 9:11814. doi: 10.1038/s41598-019-48342-7
58. Cecarini V, Bonfili L, Gogoi O, Lawrence S, Venanzi FM, Azevedo V, et al. Neuroprotective effects of p62(SQSTM1)-engineered lactic acid bacteria in Alzheimer's disease: a pre-clinical study. Aging. (2020) 12:15995–6020. doi: 10.18632/aging.103900
59. Nimgampalle M, Kuna Y. Anti-Alzheimer properties of probiotic, lactobacillus plantarum MTCC 1325 in Alzheimer's disease induced albino rats. J Clin Diagn Res. (2017) 11:KC01–5. doi: 10.7860/JCDR/2017/26106.10428
60. Wu Q, Li Q, Zhang X, Ntim M, Wu X, Li M, et al. Treatment with Bifidobacteria can suppress Aβ accumulation and neuroinflammation in APP/PS1 mice. PeerJ. (2020) 8:e10262. doi: 10.7717/peerj.10262
61. Bonfili L, Cecarini V, Berardi S, Scarpona S, Suchodolski JS, Nasuti C, et al. Microbiota modulation counteracts Alzheimer's disease progression influencing neuronal proteolysis and gut hormones plasma levels. Sci Rep. (2017) 7:2426. doi: 10.1038/s41598-017-02587-2
62. Lee HJ, Hwang YH, Kim DH. Lactobacillus plantarum C29-Fermented soybean (DW2009) Alleviates memory impairment in 5XFAD transgenic mice by regulating microglia activation and gut microbiota composition. Mol Nutr Food Res. (2018) 62:e1800359. doi: 10.1002/mnfr.201800359
63. Zhu F, Li C, Chu F, Tian X, Zhu J. Target dysbiosis of gut microbes as a future therapeutic manipulation in Alzheimer's disease. Front Aging Neurosci. (2020) 12:544235. doi: 10.3389/fnagi.2020.544235
64. Chen C, Ahn EH, Kang SS, Liu X, Alam A, Ye K. Gut dysbiosis contributes to amyloid pathology, associated with C/EBPβ/AEP signaling activation in Alzheimer's disease mouse model. Sci Adv. (2020) 6:eaba0466. doi: 10.1126/sciadv.aba0466
65. Kesika P, Suganthy N, Sivamaruthi BS, Chaiyasut C. Role of gut-brain axis, gut microbial composition, and probiotic intervention in Alzheimer's disease. Life Sci. (2021) 264:118627. doi: 10.1016/j.lfs.2020.118627
66. Pistollato F, Sumalla Cano S, Elio I, Masias Vergara M, Giampieri F, Battino M. Role of gut microbiota and nutrients in amyloid formation and pathogenesis of Alzheimer disease. Nutr Rev. (2016) 74:624–34. doi: 10.1093/nutrit/nuw023
67. Bekkering P, Jafri I, van Overveld FJ, Rijkers GT. The intricate association between gut microbiota and development of type 1, type 2 and type 3 diabetes. Expert Rev Clin Immunol. (2013) 9:1031–41. doi: 10.1586/1744666X.2013.848793
68. Daulatzai MA. Role of stress, depression, and aging in cognitive decline and Alzheimer's disease. Curr Top Behav Neurosci. (2014) 18:265–96. doi: 10.1007/7854_2014_350
69. Naseer MI, Bibi F, Alqahtani MH, Chaudhary AG, Azhar EI, Kamal MA, et al. Role of gut microbiota in obesity, type 2 diabetes and Alzheimer's disease. CNS Neurol Disord Drug Targets. (2014) 13:305–11. doi: 10.2174/18715273113126660147
70. Alam MZ, Alam Q, Kamal MA, Abuzenadah AM, Haque A. A possible link of gut microbiota alteration in type 2 diabetes and Alzheimer's disease pathogenicity: an update. CNS Neurol Disord Drug Targets. (2014) 13:383–90. doi: 10.2174/18715273113126660151
71. Erny D, Hrabě de Angelis AL, Jaitin D, Wieghofer P, Staszewski O, David E, et al. Host microbiota constantly control maturation and function of microglia in the CNS. Nat Neurosci. (2015) 18:965–77. doi: 10.1038/nn.4030
72. Ho L, Ono K, Tsuji M, Mazzola P, Singh R, Pasinetti GM. Protective roles of intestinal microbiota derived short chain fatty acids in Alzheimer's disease-type beta-amyloid neuropathological mechanisms. Expert Rev Neurother. (2018) 18:83–90. doi: 10.1080/14737175.2018.1400909
73. https://www.uniprot.org/. Bifidobacterium longum (strain NCC 2705).(2021). Available online at: https://www.uniprot.org/proteomes/UP000000439 (accessed July 1, 2021).
74. EFSA EFSA Panel on Biological Hazards (BIOHAZ), Koutsoumanis K, Allende A, Alvarez-Ordóñez A, Bolton D, Bover-Cid S, et al. Update of the list of QPS-recommended biological agents intentionally added to food or feed as notified to EFSA 14: suitability of taxonomic units notified to EFSA until March 2021. EFSA J. (2021) 19:e06689. doi: 10.2903/j.efsa.2021.6689
75. Cassir N, Benamar S, La Scola B. Clostridium butyricum: from beneficial to a new emerging pathogen. Clin Microbiol Infect. (2016) 22:37–45. doi: 10.1016/j.cmi.2015.10.014
76. Dykes JK, Lúquez C, Raphael BH, McCroskey L, Maslanka SE. Laboratory investigation of the first case of botulism caused by clostridium butyricum type E toxin in the United States. J Clin Microbiol. (2015) 53:3363–5. doi: 10.1128/JCM.01351-15
77. Seki H, Shiohara M, Matsumura T, Miyagawa N, Tanaka M, Komiyama A, et al. Prevention of antibiotic-associated diarrhea in children by Clostridium butyricum MIYAIRI. Pediatr Int. (2003) 45:86–90. doi: 10.1046/j.1442-200X.2003.01671.x
78. Li H, Sun J, Du J, Wang F, Fang R, Yu C, et al. Clostridium butyricum exerts a neuroprotective effect in a mouse model of traumatic brain injury via the gut-brain axis. Neurogastroenterol Motil. (2018) 30:e13260. doi: 10.1111/nmo.13260
79. Stoeva MK, Garcia-So J, Justice N, Myers J, Tyagi S, Nemchek M, et al. Butyrate-producing human gut symbiont, clostridium butyricum, and its role in health and disease. Gut Microbes. (2021) 13:1–28. doi: 10.1080/19490976.2021.1907272
80. Nabar NR, Shi CS, Kehrl JH. Signaling by the Toll-Like Receptors Induces Autophagy Through Modification of Beclin 1: Molecular Mechanism. In Academic Press (2018). p. 75–84. doi: 10.1016/B978-0-12-809819-6.00006-X
81. Kale J, Osterlund EJ, Andrews DW. BCL-2 family proteins: changing partners in the dance towards death. Cell Death Differ. (2018) 25:65–80. doi: 10.1038/cdd.2017.186
82. Liu J, Sun J, Wang F, Yu X, Ling Z, Li H, et al. Neuroprotective effects of clostridium butyricum against vascular dementia in mice via metabolic butyrate. Biomed Res Int. (2015) 2015:412946. doi: 10.1155/2015/412946
83. Hadar A, Gozes I, Gurwitz D. RGS2 and SIRT1 link renin angiotensin aldosterone system to Alzheimer's disease. In: Gozes I, editor. Neuroprotection in Alzheimer's Disease. Elsevier (2017). p. 239–51. doi: 10.1016/B978-0-12-803690-7.00012-0
84. Ng F, Wijaya L, Tang BL. SIRT1 in the brain-connections with aging-associated disorders and lifespan. Front Cell Neurosci. (2015) 9:64. doi: 10.3389/fncel.2015.00064
85. Kumar R, Chaterjee P, Sharma PK, Singh AK, Gupta A, Gill K, et al. Sirtuin1: a promising serum protein marker for early detection of Alzheimer's disease. PLoS ONE. (2013) 8:e61560. doi: 10.1371/journal.pone.0061560
86. Castelli V, d'Angelo M, Lombardi F, Alfonsetti M, Antonosante A, Catanesi M, et al. Effects of the probiotic formulation SLAB51 in in vitro and in vivo Parkinson's disease models. Aging. (2020) 12:4641–59. doi: 10.18632/aging.102927
87. Akbari E, Asemi Z, Daneshvar Kakhaki R, Bahmani F, Kouchaki E, Tamtaji OR, et al. Effect of probiotic supplementation on cognitive function and metabolic status in alzheimer's disease: a randomized, double-blind and controlled trial. Front Aging Neurosci. (2016) 8:256. doi: 10.3389/fnagi.2016.00256
88. Tamtaji OR, Heidari-Soureshjani R, Mirhosseini N, Kouchaki E, Bahmani F, Aghadavod E, et al. Probiotic and selenium co-supplementation, and the effects on clinical, metabolic and genetic status in Alzheimer's disease: a randomized, double-blind, controlled trial. Clin Nutr. (2019) 38:2569–75. doi: 10.1016/j.clnu.2018.11.034
89. Agahi A, Hamidi GA, Daneshvar R, Hamdieh M, Soheili M, Alinaghipour A, et al. Does severity of Alzheimer's disease contribute to its responsiveness to modifying gut microbiota? a double blind clinical trial. Front Neurol. (2018) 9:662. doi: 10.3389/fneur.2018.00662
90. Egert M, Simmering R. The microbiota of the human skin. Adv Exp Med Biol. (2016) 902:61–81. doi: 10.1007/978-3-319-31248-4_5
91. Costabile A, Fava F, Röytiö H, Forssten SD, Olli K, Klievink J, et al. Impact of polydextrose on the faecal microbiota: a double-blind, crossover, placebo-controlled feeding study in healthy human subjects. Br J Nutr. (2012) 108:471–81. doi: 10.1017/S0007114511005782
92. Tzounis X, Rodriguez-Mateos A, Vulevic J, Gibson GR, Kwik-Uribe C, Spencer JP. Prebiotic evaluation of cocoa-derived flavanols in healthy humans by using a randomized, controlled, double-blind, crossover intervention study. Am J Clin Nutr. (2011) 93:62–72. doi: 10.3945/ajcn.110.000075
93. Chen D, Yang X, Yang J, Lai G, Yong T, Tang X, et al. Prebiotic effect of fructooligosaccharides from morinda officinalis on Alzheimer's disease in rodent models by targeting the microbiota-gut-brain axis. Front Aging Neurosci. (2017) 9:403. doi: 10.3389/fnagi.2017.00403
94. Hort J, Valis M, Angelucci F. Administration of pre/probiotics with conventional drug treatment in Alzheimer's disease. Neural Regen Res. (2020) 15:448–9. doi: 10.4103/1673-5374.266057
95. Nguyen TL, Vieira-Silva S, Liston A, Raes J. How informative is the mouse for human gut microbiota research? Dis Model Mech. (2015) 8:1–16. doi: 10.1242/dmm.017400
96. Razavi AC, Potts KS, Kelly TN, Bazzano LA. Sex, gut microbiome, and cardiovascular disease risk. Biol Sex Differ. (2019) 10:29. doi: 10.1186/s13293-019-0240-z
97. Nebel RA, Aggarwal NT, Barnes LL, Gallagher A, Goldstein JM, Kantarci K, et al. Understanding the impact of sex and gender in Alzheimer's disease: a call to action. Alzheimers Dement. (2018) 14:1171–83. doi: 10.1016/j.jalz.2018.04.008
Keywords: microbiota-gut-brain axis, gut microbiota composition, short chain fatty acids, inflammatory markers, amyloid-beta, cognitive functioning, Bifidobacterium longum, Clostridium butyricum
Citation: de Rijke TJ, Doting MHE, van Hemert S, De Deyn PP, van Munster BC, Harmsen HJM and Sommer IEC (2022) A Systematic Review on the Effects of Different Types of Probiotics in Animal Alzheimer's Disease Studies. Front. Psychiatry 13:879491. doi: 10.3389/fpsyt.2022.879491
Received: 19 February 2022; Accepted: 01 April 2022;
Published: 27 April 2022.
Edited by:
Błażej Misiak, Wroclaw Medical University, PolandReviewed by:
Claudia Perez-Cruz, Instituto Politécnico Nacional de México (CINVESTAV), MexicoTauqeerunnisa Syeda, Purdue University, United States
Copyright © 2022 de Rijke, Doting, van Hemert, De Deyn, van Munster, Harmsen and Sommer. This is an open-access article distributed under the terms of the Creative Commons Attribution License (CC BY). The use, distribution or reproduction in other forums is permitted, provided the original author(s) and the copyright owner(s) are credited and that the original publication in this journal is cited, in accordance with accepted academic practice. No use, distribution or reproduction is permitted which does not comply with these terms.
*Correspondence: Iris E. C. Sommer, aS5lLmMuc29tbWVyQHVtY2cubmw=