- 1Laboratory of Translational Physiology and Pharmacology, Pharmaceutical Sciences Graduate Program, Vila Velha University, Vila Velha, Brazil
- 2Federal Institute of Education, Science and Technology (IFES), Vila Velha, Brazil
- 3Physiology and Pharmacology of Chronic Diseases (FIFAEC), Center for Research in Molecular Medicine and Chronic Diseases (CIMUS), University of Santiago de Compostela, Santiago de Compostela, Spain
Alzheimer's disease (AD) is a progressive and incurable neurodegenerative disorder of integrative areas of the brain, characterized by cognitive decline and disability resulting in negative impacts on the family of the patients and the health care services worldwide. AD involves oxidative stress, neuroinflammation and accelerated apoptosis, accompanied by deposition of amyloid-β peptide plaques and tau protein-based neurofibrillary tangles in the central nervous system. Among the multiple factors that contribute to the onset and evolution of this disease, aging stands out. That is why the prevalence of this disease has increased due to the constant increase in life expectancy. In the hope of finding new, more effective methods to slow the progression of this disease, over the last two decades, researchers have promoted “omics”-based approaches that include the gut microbiota and their reciprocal interactions with different targets in the body. This scientific advance has also led to a better understanding of brain compartments and the mechanisms that affect the integrity of the blood-brain barrier. This review aims to discuss recent advances related to the gut-brain-microbiota axis in AD. Furthermore, considering that AD involves psychiatric symptoms, this review also focuses on the psychiatric factors that interact with this axis (an issue that has not yet been sufficiently addressed in the literature).
Introduction
With the aging of the population, as a result of the change in the age pyramid, neurodegenerative diseases are increasing their percentage as a cause of death and disability, besides representing a large and growing economic impact on health care (1, 2). Among neurodegenerative diseases, Alzheimer's disease (AD) is the most important, since it corresponds to 60% of dementias, being the most prevalent etiology worldwide (3). The costs related to the treatment and medical care of people with AD are considerably high and dementia is one of the most expensive conditions for society (4). Thus, AD stands out in the development of public policies to increase survival, reduce the socioeconomic impact, as well as improve the quality of life of people diagnosed with AD and the caregivers and family members (3).
AD affects the various cognitive domains of an individual, such as memory, executive functions, language, behavioral habits, as well as visuospatial skills and attention (5). Symptoms are explained by progressive synaptic loss, cholinergic dysfunction, and neuronal death observed in brain regions responsible for cognitive functions, especially in the cerebral cortex, hippocampus, and striatum (6–8). Such neuropathological findings appear before the manifestation of cognitive deterioration and neuropsychic dysfunctions (9).
In this context, intestinal dysbiosis has been strongly associated with the etiopathogenic mechanisms of cognitive and neuropsychiatric dysfunctions of AD and may be closely related to the promotion of neuroinflammation, oxidative stress, hyperphosphorylation of tau protein, and aggregation of amyloid-β protein (Aβ) (10, 11).
Aging, a main risk factor for AD, involves a process of changes in the gastrointestinal system: hypochlorhydria, difficulties in transit and intestinal motility, degenerative changes in enteric nerve cells, reduced absorption capacity as well as changes in the quality and quantity of the intestinal microbiota (12–14). Therefore, scientific evidence indicates the importance of understanding the underlying mechanisms involved in the brain microbiota-gut axis as a promising pathway for new therapeutic strategies for the prevention and treatment of AD.
Gut-brain cross-talking is crucial in the regulation of the central nervous system (CNS) and the gastrointestinal system, and several studies have characterized its importance in the homeostatic health-disease process (15–18). In recent years, there has been a growing interest in the characterization of the gut microbiota as a main regulator of this bidirectional communication that impacts the neurophysiological bases of psychiatric and neurodegenerative disorders (19–21). Thus, the development of technologies for the study of microbiota-brain communication has gained prominence, as well as the investigation of new therapies for neuropsychiatric symptoms through intestinal modulation. This review aims to update knowledge about the role of the gut-brain-microbiota axis in the development and prevention of neuropsychiatric symptoms in Alzheimer's disease (AD).
Gut Microbiota, Aging and Alzheimer's Disease
The gut microbiota comprises a range of microorganisms of around 1,000–5,000 different non-redundant species. The vast majority correspond to bacteria belonging to Phyla Firmicutes, Bacteroidetes, Actinobacteria, Proteobacteria, Fusobacteria and Verrucomicrobia, and the existence of 150 times more genes than in the human genome has been demonstrated (22, 23). It consists of more than 100 trillion microbial cells that interfere not only in intestinal and absorptive functions, but also in a wide network of neuronal, mental, immunological, endocrine, and metabolic actions (24).
The intestinal microbiota plays important functions in the body, including the maintenance of a mucous barrier of enterocytes responsible for a complex system of defense against pathogens in the host, the control over intestinal permeability and the regulation of absorption, immunomodulation, and anti-inflammatory mechanisms (25). In addition, it is responsible for metabolic pathways of production of vitamins, hormones, amino acids, and the biotransformation of short-chain fatty acids (26).
Gut-brain communication is based on signals generated in the gut microbiota that send and receive information from distant organs. This axis of information includes neural pathways through autonomic nervous system with branches of the vagus nerve, endocrine transmission through hormones (mainly the hypothalamus-pituitary-adrenal system) and immunological propagation through chemokines and cytokines (16, 27, 28) (Figure 1).
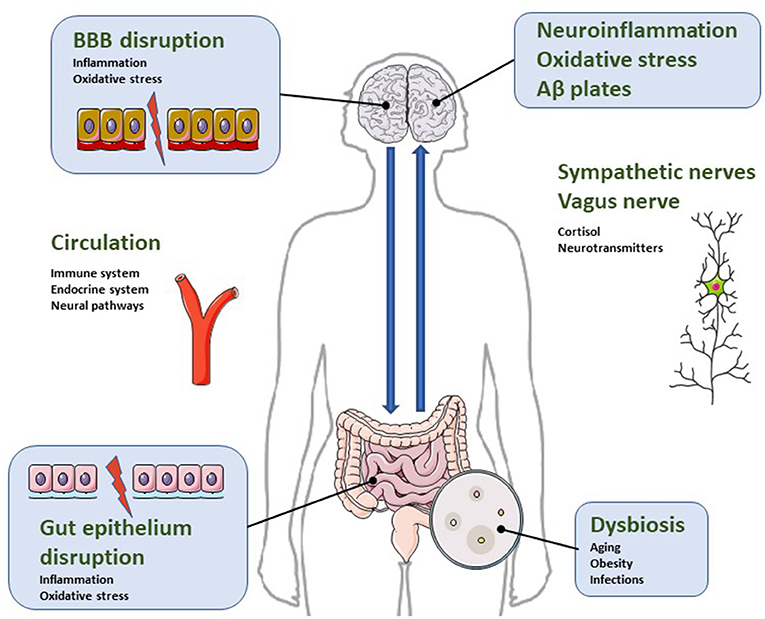
Figure 1. Illustrative diagram regarding the bidirectional relationship of the microbiota-gut-brain axis in the pathophysiology of neuroinflammatory parameters characteristic of AD. Dysbiosis is a condition characterized by increased intestinal permeability due to gut epithelial disruption. It impacts microglial and astrocytic activation and causes blood brain barrier disruption. Consequently, dysbiosis activates neuroinflammatory pathways, oxidative stress and neuronal dysregulation, leading to apoptosis and accumulation of β-amyloid protein. For more details, see text.
This symbiotic community of non-pathogenic microorganisms has wide variability and changes due to exposure to intrinsic and extrinsic factors throughout life, such as use of antibiotics, sedentary lifestyle, infections, diet, aging, cesarean sections, genetics, chronic stress and lack of breastfeeding (29–32). Some of the factors that regulate communication through the gut-brain axis are represented in Figure 2, although their review is beyond the scope of this article.
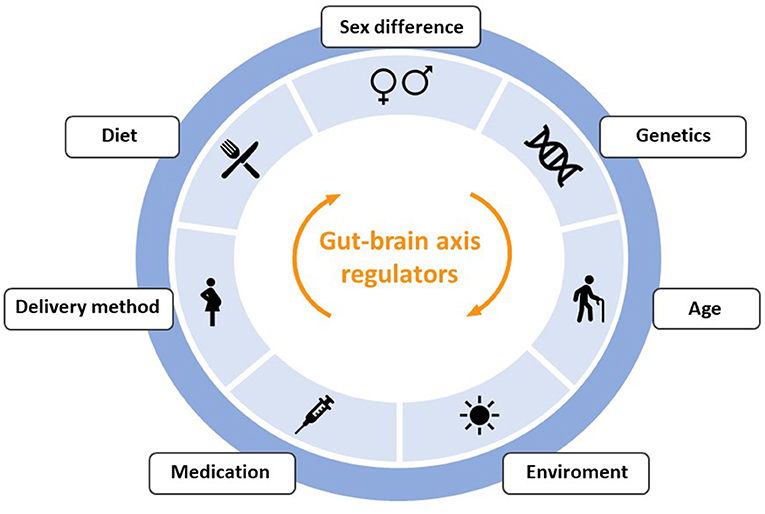
Figure 2. Outline of the main factors that can produce alterations in the communication between the intestine and the brain through the gut-brain-axis.
The imbalance in the composition of the intestinal microbiota is called intestinal dysbiosis and triggers a cascade of dysfunctional processes, including neuropsychiatric symptoms of neurodegenerative diseases such as AD (33–36) (Figure 1).
With age, microbiota suffers a reduction in the amount of Lactobacillus, Bifidobacteria, Bacteroidetes, Firmicutes, Lachnospiraceae, Ruminococcaceae, Coprococcus, Roseburia and Faecalibacterium, and an increase in the proportion of Odoribacter and Butyricimonas (37–39). Such changes are associated with gastrointestinal disorders that favor increased intestinal permeability, dysbiosis, and the development of a neuroinflammatory cascade (Figure 1) (40, 41).
Microbiota and Neurotransmitters
Neurotransmitters play an important role in the etiopathogenic mechanisms involved in the neurodegeneration of Alzheimer's disease since they are responsible for the satellite transmission and interneuron communication (Table 1) (42). According to this, the main agents approved for the treatment of AD are acetylcholinesterase inhibitors (donepezil, tacrine, galantamine and rivastigmine), which prevent the decrease in acetylcholine, a neurotransmitter related to cognitive functions such as learning and memory, in the synaptic cleft (43).
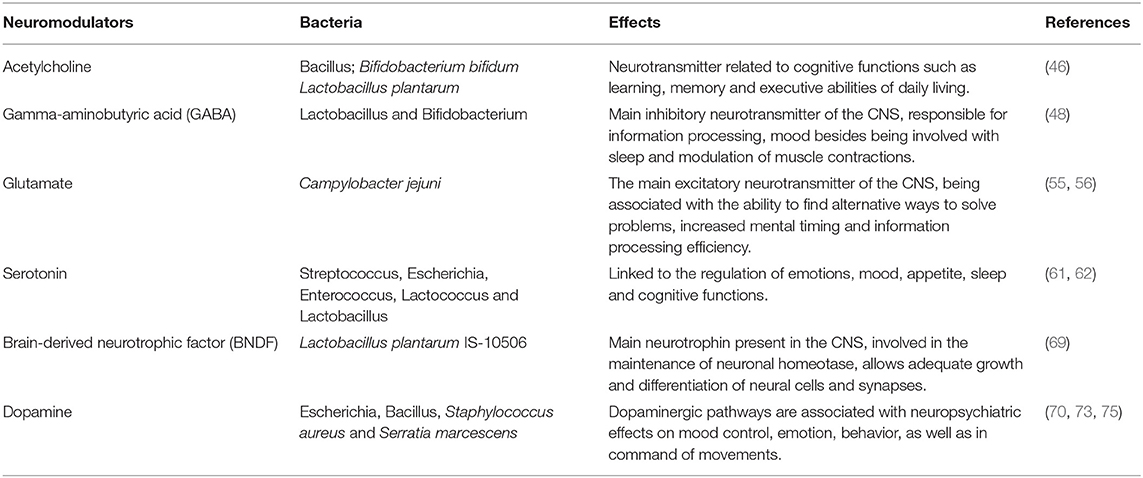
Table 1. Scientific evidence of the association of bacteria, neurotransmitters, and neuropsychiatric effects.
Gut microbiota participates in the production and release of neurotransmitters through enteroendocrine cells, which consequently interfere with CNS functions (44). Strains of Lactobacillus have been shown to be capable of producing acetylcholine (45). Furthermore, in an animal model of AD, the administration of Bifidobacterium bifidum and Lactobacillus plantarum for 8 weeks, associated with physical training, relieved amyloid-β protein neurotoxicity and improved spatial learning by an acetylcholine-mediated mechanism (46).
The main CNS inhibitory neurotransmitter, γ-aminobutyric acid (GABA), important for maintaining neuronal homeostasis, is produced by strains of Lactobacillus and Bifidobacterium (47, 48). Enteric dysbiosis reduces GABA in the gastrointestinal tract and brain, unbalancing the GABA/glutamate ratio, increasing the excitotoxic potential and leading to AD progression (49). In fact, GABA levels are reduced in the cortex of AD patients (50). Also, in experiments with diet-induced obese and metabolically dysfunctional mice, GABA production by lactobacilli has been shown to reduce metabolic and depressive behavior disturbances (51).
Glutamate, the main excitatory neurotransmitter in the CNS, is also a key part of balanced synaptic transmission and neuronal plasticity, since it acts directly through N-methyl-D-aspartate (NMDA) receptors in the acquisition of new knowledge, as well as storage capacity (52, 53). An overactivation of glutamate may be involved in neurotoxicity, leading to neuronal damage/death, which justifies the introduction of memantine (NMDA antagonist) in the pharmacotherapy of AD. Research in humans has associated glutamate metabolism by the gut microbiota with neurocognitive functions, such as the ability to find alternative ways to solve problems, increased mental timing, and efficiency in information processing (54). Finally, Campylobacter jejuni was associated with a stimulus to glutamate synthesis through direct stimulation of the enzyme γ-glutamyltranspeptidase (GGT) (55, 56).
Other authors have shown an association between intestinal diseases and neuropsychiatric disorders. One study identified reduced anxiety-like behavior associated with the absence of gut microbiota in mice, related to lower expression of NMDA receptor messenger RNA in the amygdala (57). Also, colitis induction in mice generated anxious behaviors and cognitive loss associated with the reduction of intestinal bacteria due to acute local inflammation, mainly lactobacilli and segmented filamentous bacteria. These effects were prevented by administration of probiotics containing Lactobacillus rhamnosus R0011 and Lactobacillus helveticus R0052 (58).
The serotonergic system is also associated with brain dysfunctions such as mood swings, cognition, and the circadian cycle (59, 60). Serotonin is a product of tryptophan metabolism and about 90% of its synthesis occurs through bacteria of the gastrointestinal tract, such as Streptococcus, Escherichia, Enterococcus, Lactococcus and Lactobacillus (61, 62). Tryptophan concentrations are increased in the plasma of germ-free male animals and anxiety normalized after restoration of the gut microbiota, reinforcing the importance of bidirectional communication of the gut-brain axis in serotonergic neurotransmission (63).
Eubiosis (a healthy and balanced bacterial ecosystem) was also related to the regulation of brain-derived neurotrophic factor (BNDF) levels in brain regions associated with memory, learning, cognition, and visuospatial abilities (64, 65). BDNF mediates important beneficial effects of exercise, including various neuronal processes of development and neuroplasticity (66), and a reduction in its expression is considered a reliable marker for AD, as it can exacerbate pathophysiological tau protein and β-amyloid deposition (67). BDNF has been shown to decrease Aβ production by enhancing α-secretase processing of amyloid precursor protein (APP) (68). Upregulation of brain BDNF expression was shown in rats after probiotic treatment containing Lactobacillus plantarum IS-10506, suggesting its prophylactic value against AD (69).
In addition, studies increasingly demonstrate the interaction of the dopaminergic system with the neuropsychiatric symptoms of AD (70). Neuronal death and age-dependent dopaminergic degeneration were observed exclusively in the ventral tegmental area in pre-plaque stages in a mouse model of AD (71). Dopaminergic neurons are located mainly in the ventral tegmental area, which is responsible for the motivation and reward system, and in the substantia nigra pars compact, mainly involved in movement control. Dopamine may be directly related to neuropsychiatric symptoms common in AD, such as anhedonia and apathy (72). Also, the cholinergic deficit in AD was transiently restored by the administration of a dopaminergic receptor agonist under transcranial magnetic stimulation (73, 74). Finally, the in vitro production of dopamine by several bacteria, including Bacillus cereus, Bacillus mycoides, Bacillus subtilis, Escherichia coli, Serratia marcescens, and Staphylococcus aureus, has been reported (75, 76).
This experimental and clinical evidence suggests the relevance of the gut microbiota not only for host intestinal health, but also for neurocognitive protection and improvement of clinical outcomes.
Microbiota and Neuroinflammation in AD
Neuroinflammation has been reported to play an important role in the pathophysiological mechanisms of AD (77, 78). It may have a peripheral origin, since increased peripheral cytokine production may lead to endogenous cerebral cytokine production (79). Thus, intestinal inflammatory processes can affect brain function, and have been postulated as a prominent factor in the progression of neurodegenerative diseases (80). Symptoms associated with cognitive and functional impairment of Alzheimer's, such as apathy (81) or agitation (82) have been related to an increase in peripheral proinflammatory mediators. In fact, there is considerable experimental evidence indicating that systemic inflammation causes disruptive effects on the blood brain barrier BBB, facilitating the entry of inflammatory factors into the brain, a process implicated in the pathogenesis of neurodegeneration (83, 84) (Figure 1).
The disruptive processes of the BBB that allow the entry of inflammatory factors into the CNS have been related to altered states of the intestinal microbiota. In 2014, a study showed that germ-free mice showed increased permeability of their BBB, compared to mice with normal gut flora, and a lower permeability of the barrier could be restored by a recolonization of the normal microbiota (85). In fact, eubiosis helps keep intestinal intercellular junctions intact, favoring a correct @ control of intestinal permeability, which prevents the entry of proinflammatory substances into the bloodstream (86). However, dysbiosis increases permeability, giving rise to a dysfunctional intestinal barrier and culminating in an inflammatory process that compromises the integrity of the BBB (87), and, therefore, facilitates the onset of neurodegenerative processes.
Other studies have also suggested a relationship between alterations in the intestinal microbiota and neuroinflammation. Inflammatory neurodegeneration could be triggered by the activation of the pro-inflammatory nuclear factor κB (NF-kB) by lipopolysaccharides produced by Bacteroidetes species (88). Also, the induction of cerebral amyloidosis in patients with AD has been related to an inflammatory process due to an imbalance between proinflammatory bacteria (Escherichia and Shigella) to the detriment of beneficial species, mainly Escherichia rectale. Likewise, the reduction of proinflammatory cytokines has been related to the presence of anti-inflammatory intestinal bacteria in patients with dementia and amyloidosis (89).
Kim et al. (90) showed that the gut microbiota of mice with amyloid disease and neurofibrillary tangles had a loss of epithelial barrier integrity in addition to gut inflammation. Interestingly, transplantation of fecal microbiota from healthy wild-type mice to diseased mice reduced the formation of β-amyloid plaques and neurofibrillary tangles, glial reactivity, and cognitive impairment and also reversed abnormalities in colonic gene expression related to the activity of intestinal macrophages and circulating inflammatory monocytes in the blood (90).
Using transgenic animals, other researchers have shown that fecal microbiota transplantation reduces amyloid plaques, inflammatory markers, and improves neuroplasticity and cognitive aspects (91). Therefore, modulation of the gut microbiota by probiotics and fecal microbiota transplantation interventions is relevant to protect the host's cognitive behavior, an effect in which the reduction of neuroinflammation is involved (92).
For a recent review on the role of the microbiota in neuroinflammation and synaptic dysfunction in AD see, e.g., Bairamian et al. (41).
Microbiota and Oxidative Stress in AD
Oxidative stress leads to increased inflammation through mitochondrial destruction and activation of astrocytes by reactive oxygen species (ROS) (93). According to the oxidative stress theory, neuronal death in AD is due to an imbalance between ROS production and clearance. Thus, the excessive concentration of ROS acts on the membranes of neuronal cells, causing a change in permeability and, consequently, an alteration in cell communication and neuronal signal transduction through a deregulation of calcium influx (94, 95).
In addition to this loss of neuronal synapses, oxidative stress contributes to the pathogenesis of AD through participation in the process of abnormal proteolytic cleavage of APP with production and deposition of the β-amyloid substance, formation of senile plaques, and hyperphosphorylation of tau protein (96, 97). In this way, oxidative stress contributes not only to the onset of AD, but also to the progression and severity of the disease (98). Recently, the importance of oxidative stress in the pathogenesis, diagnosis and monitoring of various neurodegenerative diseases, including AD, has been reviewed (99, 100).
Thus, the regulation of oxidative stress could play an important role in the control of these diseases. In this sense, the maintenance of eubiosis can play a prominent role. In fact, intestinal microbiota plays an important role in regulating oxidative stress. Gastrointestinal bacteria such as lactobacilli and bifidobacteria can convert nitrate and nitrite into nitric oxide (NO) (101). Moreover, species such as streptomycetes and bacilli synthesize NO from L-arginine through the enzyme NO synthase (NOS) (102). NO is essential in the transmission of information from the noradrenergic enteric nervous system, besides being excreted by the glutamatergic stimulus via activation of NMDA receptors. Although it has an essential role in neuroprotection, exacerbated NO levels can also cause oxidative stress, culminating in apoptotic cell damage and axonal degeneration (103). In this sense, intestinal eubiosis is important, since its ability to stimulate microglia leads to increased production of inducible NOS (iNOS), increasing NO production and helping to maintain immune balance (104, 105).
Recently, we have demonstrated that synbiotic kefir supplementation in elderly AD patients improves cognitive dysfunction on specific tests, with benefits related to memory, language, executive functions, visuospatial function, conceptualization, and abstraction skills. This outcome was observed with an increased NO and a reduction in protein oxidation and ROS levels (106).
Microbiota and Hyperactivation of the Hypothalamic-Pituitary- Adrenal Axis by Stress
Chronic stress is a physiological adaptive response of the organism to external events that can cause some harm to the individual and a risk factor for the development of neurodegenerative diseases, including AD. In fact, a neuroadaptive response to sustained and chronic stress causes immunological and neuroendocrine imbalance, increases inflammatory activity, and generates behavioral and psychological changes (107–109).
The hypothalamic-pituitary-adrenal (HPA) axis regulates the response to acute and chronic stressors, such as sedentary lifestyle, altered circadian rhythm, alcohol abuse, and exposure to noisy environments (110–112). The HPA axis responds to stress with the production of corticotrophin-releasing hormone (CRH) by the hypothalamus. CRH stimulates the pituitary gland to produce adrenocorticotrophic hormone (ACTH), which, in turn, acts on the adrenal glands to release cortisol (113). Persistent overactivation of the HPA axis leads to neuroinflammation, neuroendocrine dysfunction, hippocampal atrophy, and consequently cognitive deficits and neuropsychiatric symptoms (114, 115).
While chronically elevated cortisol levels negatively affect brain function, activation of the HPA axis also modifies the composition of the gut microbiota, causing dysbiosis (116). Treatment with prebiotics containing fructooligosaccharides and galactooligosaccharides had antidepressant and anxiolytic effects associated with reduced levels of corticosteroids caused by stress (117). Accordingly, recent studies have shown that a treatment with fructooligosaccharides could ameliorate the cognitive impairment and neuropathology change, as well as alleviate Aβ accumulation in the brain of the APP/PS1 double transgenic mice model of AD (118, 119). Similarly, galactooligosaccharides have been reported to reverse cognitive behavioral impairment in APP/PS1 mice, as well as reduce their levels of depression (120). In addition, the intake of lactobacilli was related to the improvement of cognitive parameters and the decrease in serum corticosterone levels (121).
A probiotic formulation consisting of Bifidobacterium longum 1714 given to 22 healthy male volunteers was able to mitigate increases in cortisol levels and subjective anxiety in response to an acute stressor. In addition, there was also an improvement in hippocampal-dependent visuospatial memory performance and changes in brain activity, as assessed by electroencephalography (122).
In another clinical study, the consumption of a probiotic formulation composed of Lactobacillus helveticus R0052 and Bifidobacterium longum R0175 in combination mitigated psychological distress without showing adverse effects, demonstrating the role of intestinal flora in stress, anxiety and depression (123).
Finally, germ-free mice exhibited higher levels of ACTH and corticosterone compared to the control group in response to restraint stress. This effect was fully reversed after reconstitution with Bifidobacterium infantis (124). Thus, the interaction of the intestinal microbiota was verified as an important factor in the stress response of the HPA axis.
Conclusion and Expected Forthcoming Advances
The gut microbiota is associated with functions that extend to the local gastrointestinal context, but are related to the modulation of important endocrine, metabolic, immunological, and neural pathways in the body. The existence of the microbiota-brain-gut axis demonstrates how crucial the maintenance of eubiosis is for brain homeostasis. In the context of AD, the intestinal microbiota plays a key role in microglial activation, neurogenesis, and BBB permeability.
Scientific evidence indicates that intestinal dysbiosis causes a neuroinflammatory cascade, changes in membrane permeability, in addition to worsening β-amyloid protein aggregation and tau protein hyperphosphorylation.
This article aimed at a narrative review of new knowledge and more recent evidence on the relationship between the brain-microbiota axis and the neuropsychiatric symptoms of AD. Greater efforts should be made to obtain scientific data for the development of therapeutic interventions consisting of the modulation of the intestinal microbiota that provide satisfactory clinical results and an improvement in the quality of life of patients with AD.
Author Contributions
SQ, AT, and BC: manuscript concept and initial draft. LM and AP: figures and bibliographic research. TP, EV, and MC-T: final version. EV and MC-T: funding and coordination. All authors contributed to the article and approved the submitted version.
Funding
The authors gratefully acknowledge the State Agency for Development of Science and Innovation of Espírito Santo (FAPES), The National Council for the Development of Science and Technology (CNPq), Agencia Estatal de Investigación. Ministerio de Ciencia e Innovación (Spain) and Xunta de Galicia (Spain), for their contribution to this scientific production with the following Grants: (a) PRONEX (FAPES/CNPq), EV, Edital 24/2018, Termo Outorga 569/2018; (b) PPSUS (FAPES/CNPq/Decit-MS/SESA), EV, Edital 03/2018; Termo Outorga 225/2018; (c) Universal (FAPES), BC, Edital 21/2018, Termo Outorga 120/2019; (d) Agencia Estatal de Investigación, MC-T, (PID2020-119178GB-I00); (e) Xunta de Galicia: Plan Galego IDT, 2021-2022 (Grant Number Code: ED431B 2020/26. Research group GPC GI-18629; (f) Grant FAPES, BC: Processo 552/2018; (g) Grant CNPq, EV: Processo 305740/2019-9; (h) Grant CNPq, TP: Processo 309277/2019-1.
Conflict of Interest
The authors declare that the research was conducted in the absence of any commercial or financial relationships that could be construed as a potential conflict of interest.
Publisher's Note
All claims expressed in this article are solely those of the authors and do not necessarily represent those of their affiliated organizations, or those of the publisher, the editors and the reviewers. Any product that may be evaluated in this article, or claim that may be made by its manufacturer, is not guaranteed or endorsed by the publisher.
References
1. Vossius C, Rongve A, Testad I, Wimo A, Aarsland D. The use and costs of formal care in newly diagnosed dementia: a three-year prospective follow-up study. Am J Geriatr Psychiatry. (2014) 22:381–8. doi: 10.1016/j.jagp.2012.08.014
2. GBD 2016 Neurology Collaborators. Global, regional, and national burden of neurological disorders, 1990-2016: a systematic analysis for the Global Burden of Disease Study 2016. Lancet Neurol. (2019) 18:459–80. doi: 10.1016/S1474-4422(18)30499-X
3. Alzheimer's Association 2020. Alzheimer's disease facts and figures. Alzheimers Dement. (2020) 16:391–460. doi: 10.1002/alz.12068
4. Ferretti C, Sarti FM, Nitrini R, Ferreira FF, Brucki SMD. An assessment of direct and indirect costs of dementia in Brazil. PLoS ONE. (2018) 13:e0193209. doi: 10.1371/journal.pone.0193209
5. Atri A. The Alzheimer's disease clinical spectrum: diagnosis and management. Med Clin North Am. (2019) 103:263–93. doi: 10.1016/j.mcna.2018.10.009
6. Bakkour A, Morris JC, Wolk DA, Dickerson BC. The effects of aging and Alzheimer's disease on cerebral cortical anatomy: specificity and differential relationships with cognition. Neuroimage. (2013) 76:332–44. doi: 10.1016/j.neuroimage.2013.02.059
7. Nie X, Sun Y, Wan S, Zhao H, Liu R, Li X, et al. Subregional structural alterations in hippocampus and nucleus accumbens correlate with the clinical impairment in patients with Alzheimer's disease clinical spectrum: parallel combining volume and vertex-based approach. Front Neurol. (2017) 8:399. doi: 10.3389/fneur.2017.00399
8. de Jong LW, Ferrarini L, van der Grond J, Milles JR, Reiber JH, Westendorp RG, et al. Shape abnormalities of the striatum in Alzheimer's disease. J Alzheimers Dis. (2011) 23:49–59. doi: 10.3233/JAD-2010-101026
9. Blennow K, De Leon MJ, Zetterberg H. Alzheimer's disease. Lancet. (2006) 368:387–403. doi: 10.1016/S0140-6736(06)69113-7
10. Kowalski K, Mulak A. Brain-gut-microbiota axis in Alzheimer's disease. J Neurogastroenterol Motil. (2019) 25:48–60. doi: 10.5056/jnm18087
11. Sochocka M, Donskow-Łysoniewska K, Diniz BS, Kurpas D, Brzozowska E, Leszek J. The gut microbiome alterations and inflammation-driven pathogenesis of Alzheimer's disease-a critical review. Mol Neurobiol. (2019) 56:1841–51. doi: 10.1007/s12035-018-1188-4
12. Dumic I, Nordin T, Jecmenica M, Stojkovic Lalosevic M, Milosavljevic T, Milovanovic T. Gastrointestinal tract disorders in older age. Can J Gastroenterol Hepatol. (2019) 2019:6757524. doi: 10.1155/2019/6757524
13. Soenen S, Rayner CK, Jones KL, Horowitz M. The ageing gastrointestinal tract. Curr Opin Clin Nutr Metab Care. (2016) 19:12–8. doi: 10.1097/MCO.0000000000000238
14. Kesika P, Suganthy N, Sivamaruthi BS, Chaiyasut C. Role of gut-brain axis, gut microbial composition, and probiotic intervention in Alzheimer's disease. Life Sci. (2021) 264:118627. doi: 10.1016/j.lfs.2020.118627
15. Bonaz B, Sinniger V, Pellissier S. The vagus nerve in the neuro-immune axis: implications in the pathology of the gastrointestinal tract. Front Immunol. (2017) 8:1452. doi: 10.3389/fimmu.2017.01452
16. Vasquez EC, Aires R, Ton AMM, Amorim FG. New insights on the beneficial effects of the probiotic Kefir on vascular dysfunction in cardiovascular and neurodegenerative diseases. Curr Pharm Des. (2020) 26:3700–10. doi: 10.2174/1381612826666200304145224
17. Yue Q, Cai M, Xiao B, Zhan Q, Zeng C. The microbiota-gut-brain axis and epilepsy. Cell Mol Neurobiol. (2022) 42:439–53. doi: 10.1007/s10571-021-01130-2
18. Pereira TMC, Côco LZ, Ton AMM, Meyrelles SS, Campos-Toimil M, Campagnaro BP, et al. The emerging scenario of the gut-brain axis: the therapeutic actions of the new actor Kefir against neurodegenerative diseases. Antioxidants. (2021) 10:1845. doi: 10.3390/antiox10111845
19. Foster JA, Lyte M, Meyer E, Cryan JF. Gut microbiota and brain function: an evolving field in neuroscience. Int J Neuropsychopharmacol. (2016) 19:pyv114. doi: 10.1093/ijnp/pyv114
20. Fung TC. The microbiota-immune axis as a central mediator of gut-brain communication. Neurobiol Dis. (2020) 136:104714. doi: 10.1016/j.nbd.2019.104714
21. Cerdó T, Diéguez E, Campoy C. Impact of gut microbiota on neurogenesis and neurological diseases during infancy. Curr Opin Pharmacol. (2020) 50:33–7. doi: 10.1016/j.coph.2019.11.006
22. Costea PI, Hildebrand F, Arumugam M, Bäckhed F, Blaser MJ, Bushman FD, et al. Enterotypes in the landscape of gut microbial community composition. Nat Microbiol. (2018) 3:8–16. doi: 10.1038/s41564-017-0072-8
23. Qin J, Li R, Raes J, Arumugam M, Burgdorf KS, Manichanh C, et al. A human gut microbial gene catalogue established by metagenomic sequencing. Nature. (2010) 464:59–65. doi: 10.1038/nature08821
24. Gebrayel P, Nicco C, Al Khodor S, Bilinski J, Caselli E, Comelli EM, et al. Microbiota medicine: towards clinical revolution. J Transl Med. (2022) 20:111. doi: 10.1186/s12967-022-03296-9
25. Di Tommaso N, Gasbarrini A, Ponziani FR. Intestinal barrier in human health and disease. Int J Environ Res Public Health. (2021) 18:12836. doi: 10.3390/ijerph182312836
26. Usami M, Miyoshi M, Yamashita H. Gut microbiota and host metabolism in liver cirrhosis. World J Gastroenterol. (2015) 21:11597–608. doi: 10.3748/wjg.v21.i41.11597
27. Petra AI, Panagiotidou S, Hatziagelaki E, Stewart JM, Conti P, Theoharides TC. Gut-microbiota-brain axis and its effect on neuropsychiatric disorders with suspected immune dysregulation. Clin Ther. (2015) 37:984–95. doi: 10.1016/j.clinthera.2015.04.002
28. Chen W, Liu D, Ren C, Su X, Wong CK, Yang R, et al. Special network comprised of macrophages, epithelial cells, and gut microbiota for gut homeostasis. Cells. (2022) 11:307. doi: 10.3390/cells11020307
29. Burokas A, Moloney RD, Dinan TG, Cryan JF. Microbiota regulation of the Mammalian gut-brain axis. Adv Appl Microbiol. (2015) 91:1–62. doi: 10.1016/bs.aambs.2015.02.001
30. Ton AMM, Arpini CM, Campagnaro BP, Pereira T, Vasquez EC. Alzheimer's disease: a brief update on the influence of gut microbiota and the impact of functional food. J Food Microbiol. (2018) 2:11–5. Available online at: https://www.alliedacademies.org/articles/alzheimers-disease-a-brief-update-on-the-influence-of-gut-microbiota-andthe-impact-of-functional-food.pdf
31. Angelucci F, Cechova K, Amlerova J, Hort J. Antibiotics, gut microbiota, and Alzheimer's disease. J Neuroinflammation. (2019) 16:108. doi: 10.1186/s12974-019-1494-4
32. Pushpanathan P, Mathew GS, Selvarajan S, Seshadri KG, Srikanth P. Gut microbiota and its mysteries. Indian J Med Microbiol. (2019) 37:268–77. doi: 10.4103/ijmm.IJMM_19_373
33. Weiss GA, Hennet T. Mechanisms, and consequences of intestinal dysbiosis. Cell Mol Life Sci. (2017) 74:2959–77. doi: 10.1007/s00018-017-2509-x
34. Vasquez EC, Pereira TMC, Campos-Toimil M, Baldo MP, Peotta VA. Gut microbiota, diet, and chronic diseases: the role played by oxidative stress. Oxid Med Cell Longev. (2019) 2019:7092032. doi: 10.1155/2019/7092032
35. Varesi A, Pierella E, Romeo M, Piccini GB, Alfano C, Bjørklund G, et al. The potential role of gut microbiota in Alzheimer's disease: from diagnosis to treatment. Nutrients. (2022) 14:668. doi: 10.3390/nu14030668
36. Zhang H, Chen Y, Wang Z, Xie G, Liu M, Yuan B, et al. Implications of gut microbiota in neurodegenerative diseases. Front Immunol. (2022) 13:785644. doi: 10.3389/fimmu.2022.785644
37. Hébuterne X. Gut changes attributed to ageing: effects on intestinal microflora. Curr Opin Clin Nutr Metab Care. (2003) 6:49–54. doi: 10.1097/00075197-200301000-00008
38. Mariat D, Firmesse O, Levenez F, Guimarăes VD, Sokol H, Doré J, et al. The firmicutes/bacteroidetes ratio of the human microbiota changes with age. BMC Microbiol. (2009) 9:123. doi: 10.1186/1471-2180-9-123
39. Zapata HJ, Quagliarello VJ. The microbiota and microbiome in aging: potential implications in health and age-related diseases. J Am Geriatr Soc. (2015) 63:776–81. doi: 10.1111/jgs.13310
40. Pimenta FS, Luaces-Regueira M, Ton AM, Campagnaro BP, Campos-Toimil M, Pereira TM, et al. Mechanisms of action of Kefir in chronic cardiovascular and metabolic diseases. Cell Physiol Biochem. (2018) 48:1901–14. doi: 10.1159/000492511
41. Bairamian D, Sha S, Rolhion N, Sokol H, Dorothée G, Lemere CA, et al. Microbiota in neuroinflammation and synaptic dysfunction: a focus on Alzheimer's disease. Mol Neurodegener. (2022) 17:19. doi: 10.1186/s13024-022-00522-2
42. Ferreira-Vieira TH, Guimaraes IM, Silva FR, Ribeiro FM. Alzheimer's disease: targeting the cholinergic system. Curr Neuropharmacol. (2016) 14:101–15. doi: 10.2174/1570159X13666150716165726
43. Kandimalla R, Reddy PH. Therapeutics of Neurotransmitters in Alzheimer's Disease. J Alzheimers Dis. (2017) 57:1049–69. doi: 10.3233/JAD-161118
44. Alkasir R, Li J, Li X, Jin M, Zhu B. Human gut microbiota: the links with dementia development. Protein Cell. (2017) 8:90–102. doi: 10.1007/s13238-016-0338-6
45. Stanaszek PM, Snell JF, O'Neill JJ. Isolation, extraction, and measurement of acetylcholine from Lactobacillus plantarum. Appl Environ Microbiol. (1977) 34:237–9. doi: 10.1128/aem.34.2.237-239.1977
46. Shamsipour S, Sharifi G, Taghian F. An 8-week administration of Bifidobacterium bifidum and Lactobacillus plantarum combined with exercise training alleviates neurotoxicity of Aβ and spatial learning via acetylcholine in Alzheimer rat model. J Mol Neurosci. (2021) 71:1495–505. doi: 10.1007/s12031-021-01812-y
47. Siragusa S, De Angelis M, Di Cagno R, Rizzello CG, Coda R, Gobbetti M. Synthesis of gamma-aminobutyric acid by lactic acid bacteria isolated from a variety of Italian cheeses. Appl Environ Microbiol. (2007) 73:7283–90. doi: 10.1128/AEM.01064-07
48. Barrett E, Ross RP, O'Toole PW, Fitzgerald GF, Stanton C. γ-Aminobutyric acid production by culturable bacteria from the human intestine. J Appl Microbiol. (2012) 113:411–7. doi: 10.1111/j.1365-2672.2012.05344.x
49. Hu X, Wang T, Jin F. Alzheimer's disease and gut microbiota. Sci China Life Sci. (2016) 59:1006–23. doi: 10.1007/s11427-016-5083-9
50. Solas M, Puerta E, Ramirez MJ. Treatment options in Alzheimer's disease: the GABA story. Curr Pharm Des. (2015) 21:4960–71. doi: 10.2174/1381612821666150914121149
51. Patterson E, Ryan PM, Wiley N, Carafa I, Sherwin E, Moloney G, et al. Gamma-aminobutyric acid-producing lactobacilli positively affect metabolism and depressive-like behaviour in a mouse model of metabolic syndrome. Sci Rep. (2019) 9:16323. doi: 10.1038/s41598-019-51781-x
52. Hynd MR, Scott HL, Dodd PR. Glutamate-mediated excitotoxicity, and neurodegeneration in Alzheimer's disease. Neurochem Int. (2004) 45:583–95. doi: 10.1016/j.neuint.2004.03.007
53. Chang CH, Lin CH, Lane HY. d-glutamate and gut microbiota in Alzheimer's disease. Int J Mol Sci. (2020) 21:2676. doi: 10.3390/ijms21082676
54. Palomo-Buitrago ME, Sabater-Masdeu M, Moreno-Navarrete JM, Caballano-Infantes E, Arnoriaga-Rodríguez M, Coll C, et al. Glutamate interactions with obesity, insulin resistance, cognition, and gut microbiota composition. Acta Diabetol. (2019) 56:569–79. doi: 10.1007/s00592-019-01313-w
55. Van der Stel AX, van Mourik A, Łaniewski P, van Putten JP, Jagusztyn-Krynicka EK, Wösten MM. The Campylobacter jejuni RacRS two-component system activates the glutamate synthesis by directly upregulating γ-glutamyltranspeptidase (GGT). Front Microbiol. (2015) 6:567. doi: 10.3389/fmicb.2015.00567
56. Baj A, Moro E, Bistoletti M, Orlandi V, Crema F, Giaroni C. Glutamatergic signaling along the microbiota-gut-brain axis. Int J Mol Sci. (2019) 20:1482. doi: 10.3390/ijms20061482
57. Neufeld KM, Kang N, Bienenstock J, Foster JA. Reduced anxiety-like behavior and central neurochemical change in germ-free mice. Neurogastroenterol Motil. (2011) 23:255–64, e119. doi: 10.1111/j.1365-2982.2010.01620.x
58. Emge JR, Huynh K, Miller EN, Kaur M, Reardon C, Barrett KE, et al. Modulation of the microbiota-gut-brain axis by probiotics in a murine model of inflammatory bowel disease. Am J Physiol Gastrointest Liver Physiol. (2016) 310:G989–98. doi: 10.1152/ajpgi.00086.2016
59. Cools R, Roberts AC, Robbins TW. Serotoninergic regulation of emotional and behavioural control processes. Trends Cogn Sci. (2008) 12:31–40. doi: 10.1016/j.tics.2007.10.011
60. Zarros, Ach., Kalopita KS, Tsakiris ST. Serotoninergic impairment and aggressive behavior in Alzheimer's disease. Acta Neurobiol Exp. (2005) 65:277–86.
61. Yano JM Yu K, Donaldson GP, Shastri GG, Ann P, Ma L, Nagler CR, et al. Indigenous bacteria from the gut microbiota regulate host serotonin biosynthesis. Cell. (2015) 161:264–76. doi: 10.1016/j.cell.2015.02.047
62. Legan TB, Lavoie B, Mawe GM. Direct and indirect mechanisms by which the gut microbiota influence host serotonin systems. Neurogastroenterol Motil. (2022) 18:666–73. doi: 10.1111/nmo.14346
63. Clarke G, Grenham S, Scully P, Fitzgerald P, Moloney RD, Shanahan F, et al. The microbiome-gut-brain axis during early life regulates the hippocampal serotonergic system in a sex-dependent manner. Mol Psychiatry. (2013) 18:666–73. doi: 10.1038/mp.2012.77
64. Nakamura-Palacios EM, Caldas CK, Fiorini A, Chagas KD, Chagas KN, Vasquez EC. Deficits of spatial learning and working memory in spontaneously hypertensive rats. Behav Brain Res. (1996) 74:217–27. doi: 10.1016/0166-4328(95)00165-4
65. Maqsood R, Stone TW. The gut-brain axis, BDNF, NMDA and CNS disorders. Neurochem Res. (2016) 41:2819–35. doi: 10.1007/s11064-016-2039-1
66. Fumagalli F, Racagni G. Riva MA. The expanding role of BDNF: a therapeutic target for Alzheimer's disease? Pharmacogenom J. (2006) 6:8–15. doi: 10.1038/sj.tpj.6500337
67. Caffino L, Mottarlini F, Fumagalli F. Born to protect: leveraging BDNF against cognitive deficit in Alzheimer's disease. CNS Drugs. (2020) 34:281–97. doi: 10.1007/s40263-020-00705-9
68. Nigam SM, Xu S, Kritikou JS, Marosi K, Brodin L, Mattson MP. Exercise and BDNF reduce Aβ production by enhancing α-secretase processing of APP. J Neurochem. (2017) 142:286–96. doi: 10.1111/jnc.14034
69. Ranuh R, Athiyyah AF, Darma A, Risky VP, Riawan W, Surono IS, et al. Effect of the probiotic Lactobacillus plantarum IS-10506 on BDNF and 5HT stimulation: role of intestinal microbiota on the gut-brain axis. Iran J Microbiol. (2019) 11:145–50.
70. Martorana A. Koch G. “Is dopamine involved in Alzheimer's disease?” Front Aging Neurosci. (2014) 6:252. doi: 10.3389/fnagi.2014.00252
71. Nobili A, Latagliata EC, Viscomi MT, Cavallucci V, Cutuli D, Giacovazzo G, et al. Dopamine neuronal loss contributes to memory and reward dysfunction in a model of Alzheimer's disease. Nat Commun. (2017) 8:14727. doi: 10.1038/ncomms14727
72. D'Amelio M, Nisticò R. Unlocking the secrets of dopamine in Alzheimer's Disease. Pharmacol Res. (2018) 128:399. doi: 10.1016/j.phrs.2017.06.018
73. Martorana A, Stefani A, Palmieri MG, Esposito Z, Bernardi G, Sancesario G, et al. L-dopa modulates motor cortex excitability in Alzheimer's disease patients. J Neural Transm. (2008) 115:1313–9. doi: 10.1007/s00702-008-0082-z
74. Ni Z, Chen R. Transcranial magnetic stimulation to understand pathophysiology and as potential treatment for neurodegenerative diseases. Transl Neurodegener. (2015) 4:22. doi: 10.1186/s40035-015-0045-x
75. Tsavkelova EA, Botvinko IV, Kudrin VS, Oleskin AV. Detection of neurotransmitter amines in microorganisms with the use of high-performance liquid chromatography. Dokl Biochem. (2000) 372:115–7.
76. Holzer P, Farzi A. Neuropeptides and the microbiota-gut-brain axis. Adv Exp Med Biol. (2014) 817:195–219. doi: 10.1007/978-1-4939-0897-4_9
77. Sato C, Barthélemy NR, Mawuenyega KG, Patterson BW, Gordon BA, Jockel-Balsarotti J, et al. Tau kinetics in neurons and the human central nervous system. Neuron. (2018) 97:1284–98.e7. doi: 10.1016/j.neuron.2018.02.015
78. Liu P, Wang Y, Sun Y, Peng G. Neuroinflammation as a potential therapeutic target in Alzheimer's disease. Clin Interv Aging. (2022) 17:665–74. doi: 10.2147/CIA.S357558
79. Engelhardt B, Vajkoczy P, Weller RO. The movers and shapers in immune privilege of the CNS. Nat Immunol. (2017) 18:123–31. doi: 10.1038/ni.3666
80. Xie J, Van Hoecke L, Vandenbroucke RE. The impact of systemic inflammation on Alzheimer's disease pathology. Front Immunol. (2022) 12:796867. doi: 10.3389/fimmu.2021.796867
81. Teixeira AL, Salem H, Martins LB, Gonzales MM, Seshadri S, Suchting R. Factors associated with apathy in Alzheimer's disease: a cross-sectional analysis of the Texas Alzheimer's Research and Care Consortium (TARCC) Study. J Alzheimers Dis. (2022) 86:403–11. doi: 10.3233/JAD-215314
82. Tumati S, Herrmann N, Marotta G, Li A, Lanctôt KL. Blood-based biomarkers of agitation in Alzheimer's disease: Advances and future prospects. Neurochem Int. (2022) 152:105250. doi: 10.1016/j.neuint.2021.105250
83. Varatharaj A, Galea I. The blood-brain barrier in systemic inflammation. Brain Behav Immun. (2017) 60:1–12. doi: 10.1016/j.bbi.2016.03.010
84. Viña D, Seoane N, Vasquez EC, Campos-Toimil M. cAMP Compartmentalization in cerebrovascular endothelial cells: new therapeutic opportunities in Alzheimer's disease. Cells. (2021) 10:1951. doi: 10.3390/cells10081951
85. Braniste V, Al-Asmakh M, Kowal C, Anuar F, Abbaspour A, Tóth M, et al. The gut microbiota influences blood-brain barrier permeability in mice. Sci Transl Med. (2014) 6:263ra158. doi: 10.1126/scitranslmed.3009759
86. Kelly JR, Kennedy PJ, Cryan JF, Dinan TG, Clarke G, Hyland NP. Breaking down the barriers: the gut microbiome, intestinal permeability, and stress-related psychiatric disorders. Front Cell Neurosci. (2015) 9:392. doi: 10.3389/fncel.2015.00392
87. Spielman LJ, Gibson DL, Klegeris A. Unhealthy gut, unhealthy brain: The role of the intestinal microbiota in neurodegenerative diseases. Neurochem Int. (2018) 120:149–63. doi: 10.1016/j.neuint.2018.08.005
88. Lukiw WJ. Bacteroides fragilis lipopolysaccharide and inflammatory signaling in Alzheimer's Disease. Front Microbiol. (2016) 7:1544. doi: 10.3389/fmicb.2016.01544
89. Cattaneo A, Cattane N, Galluzzi S, Provasi S, Lopizzo N, Festari C, et al. Association of brain amyloidosis with pro-inflammatory gut bacterial taxa and peripheral inflammation markers in cognitively impaired elderly. Neurobiol Aging. (2017) 49:60–8. doi: 10.1016/j.neurobiolaging.2016.08.019
90. Kim MS, Kim Y, Choi H, Kim W, Park S, Lee D, et al. Transfer of a healthy microbiota reduces amyloid and tau pathology in an Alzheimer's disease animal model. Gut. (2020) 69:283–94. doi: 10.1136/gutjnl-2018-317431
91. Sun J, Xu J, Ling Y, Wang F, Gong T, Yang C, et al. Fecal microbiota transplantation alleviated Alzheimer's disease-like pathogenesis in APP/PS1 transgenic mice. Transl Psychiatry. (2019) 9:189. doi: 10.1038/s41398-019-0525-3
92. Friedland RP, Chapman MR. The role of microbial amyloid in neurodegeneration. PLoS Pathog. (2017) 13:e1006654. doi: 10.1371/journal.ppat.1006654
93. Oliver DMA, Reddy PH. Small molecules as therapeutic drugs for Alzheimer's disease. Mol Cell Neurosci. (2019) 96:47–62. doi: 10.1016/j.mcn.2019.03.001
94. Benzi G, Moretti A. Are reactive oxygen species involved in Alzheimer's disease? Neurobiol Aging. (1995) 16:661–74. doi: 10.1016/0197-4580(95)00066-N
95. Praticò D. Oxidative stress hypothesis in Alzheimer's disease: a reappraisal. Trends Pharmacol Sci. (2008) 29:609–15. doi: 10.1016/j.tips.2008.09.001
96. Braak H, Thal DR, Ghebremedhin E, Del Tredici K. Stages of the pathologic process in Alzheimer disease: age categories from 1 to 100 years. J Neuropathol Exp Neurol. (2011) 70:960–9. doi: 10.1097/NEN.0b013e318232a379
97. Hanseeuw BJ, Betensky RA, Jacobs HIL, Schultz AP, Sepulcre J, Becker JA, et al. Association of amyloid and tau with cognition in preclinical Alzheimer disease: a longitudinal study. JAMA Neurol. (2019) 76:915–24. doi: 10.1001/jamaneurol.2019.1424
98. Goshtasbi H, Pakchin PS, Movafeghi A, Barar J, Castejon AM, Omidian H, et al. Impacts of oxidants and antioxidants on the emergence and progression of Alzheimer's disease. Neurochem Int. (2022) 153:105268. doi: 10.1016/j.neuint.2021.105268
99. Cecerska-Heryć E, Polikowska A, Serwin N, Roszak M, Grygorcewicz B, Heryć R, et al. Importance of oxidative stress in the pathogenesis, diagnosis, and monitoring of patients with neuropsychiatric disorders, a review. Neurochem Int. (2022) 153:105269. doi: 10.1016/j.neuint.2021.105269
100. Collins AE, Saleh TM, Kalisch BE. Naturally occurring antioxidant therapy in alzheimer's disease. Antioxidants. (2022) 11:213. doi: 10.3390/antiox11020213
101. Sobko T, Reinders CI, Jansson E, Norin E, Midtvedt T, Lundberg JO. Gastrointestinal bacteria generate nitric oxide from nitrate and nitrite. Nitric Oxide. (2005) 13:272–8. doi: 10.1016/j.niox.2005.08.002
102. Bostanciklioglu M. An update on the interactions between Alzheimer's disease, autophagy and inflammation. Gene. (2019) 705:157–66. doi: 10.1016/j.gene.2019.04.040
103. Vermeiren J, Van de Wiele T, Verstraete W, Boeckx P, Boon N. Nitric oxide production by the human intestinal microbiota by dissimilatory nitrate reduction to ammonium. J Biomed Biotechnol. (2009) 2009:284718. doi: 10.1155/2009/284718
104. Tse JKY. Gut microbiota, nitric oxide, and microglia as prerequisites for neurodegenerative disorders. ACS Chem Neurosci. (2017) 8:1438–47. doi: 10.1021/acschemneuro.7b00176
105. Steinert JR, Chernova T, Forsythe ID. Nitric oxide signaling in brain function, dysfunction, and dementia. Neuroscientist. (2010) 16:435–52. doi: 10.1177/1073858410366481
106. Ton AMM, Campagnaro BP, Alves GA, Aires R, Côco LZ, Arpini CM, et al. Oxidative stress and dementia in Alzheimer's patients: effects of synbiotic supplementation. Oxid Med Cell Longev. (2020) 2020:2638703. doi: 10.1155/2020/2638703
107. Bisht K, Sharma K, Tremblay MÈ. Chronic stress as a risk factor for Alzheimer's disease: roles of microglia-mediated synaptic remodeling, inflammation, and oxidative stress. Neurobiol Stress. (2018) 9:9–21. doi: 10.1016/j.ynstr.2018.05.003
108. Marin MF, Lord C, Andrews J, Juster RP, Sindi S, Arsenault-Lapierre G, et al. Chronic stress, cognitive functioning and mental health. Neurobiol Learn Mem. (2011) 96:583–95. doi: 10.1016/j.nlm.2011.02.016
109. Saeedi M, Rashidy-Pour A. Association between chronic stress and Alzheimer's disease: therapeutic effects of Saffron. Biomed Pharmacother. (2021) 133:110995. doi: 10.1016/j.biopha.2020.110995
110. Kennedy PJ, Cryan JF, Quigley EM, Dinan TG, Clarke G A sustained hypothalamic-pituitary-adrenal axis response to acute psychosocial stress in irritable bowel syndrome. Psychol Med. (2014) 44:3123–34. doi: 10.1017/S003329171400052X
111. Tsigos C, Chrousos GP. Hypothalamic-pituitary-adrenal axis, neuroendocrine factors, and stress. J Psychosom Res. (2002) 53:865–71. doi: 10.1016/S0022-3999(02)00429-4
112. Kazakou P, Nicolaides NC, Chrousos GP. Basic concepts and hormonal regulators of the stress system. Horm Res Paediatr. (2022) 4:715–38. doi: 10.1159/000523975
113. Chen XQ. Functional diversity of corticotropin-releasing hormone. Nat Rev Endocrinol. (2022) 18:333. doi: 10.1038/s41574-022-00663-w
114. Jacobson L. Hypothalamic-pituitary-adrenocortical axis: neuropsychiatric aspects. Compr Physiol. (2014) 4:715–38. doi: 10.1002/cphy.c130036
115. Mikulska J, Juszczyk G, Gawrońska-Grzywacz M, Herbet M. HPA Axis in the pathomechanism of depression and schizophrenia: new therapeutic strategies based on its participation. Brain Sci. (2021) 11:1298. doi: 10.3390/brainsci11101298
116. Farzi A, Fröhlich EE, Holzer P. Gut microbiota, and the neuroendocrine system. Neurotherapeutics. (2018) 15:5–22. doi: 10.1007/s13311-017-0600-5
117. Burokas A, Arboleya S, Moloney RD, Peterson VL, Murphy K, Clarke G, et al. Targeting the microbiota-gut-brain axis: prebiotics have anxiolytic and antidepressant-like effects and reverse the impact of chronic stress in mice. Biol Psychiatry. (2017) 82:472–87. doi: 10.1016/j.biopsych.2016.12.031
118. Sun J, Liu S, Ling Z, Wang F, Ling Y, Gong T, et al. Fructooligosaccharides ameliorating cognitive deficits and neurodegeneration in APP/PS1 transgenic mice through modulating gut microbiota. J Agric Food Chem. (2019) 67:3006–17. doi: 10.1021/acs.jafc.8b07313
119. Wu S, Zhang J, Jiang C, Wang S, Que R, An L. Up-regulation of neprilysin mediates the protection of fructo-oligosaccharides against Alzheimer's disease. Food Funct. (2020) 11:6565–72. doi: 10.1039/D0FO00161A
120. Yang D, Qiao J, Wang JX, Wei WY, Zhao ZX, Cai HY. [Effects of B-GOS on cognitive behavior and depression of transgenic mice with Alzheimer's disease]. Zhongguo Ying Yong Sheng Li Xue Za Zhi. (2021) 37:240–6. doi: 10.12047/j.cjap.6105.2021.028
121. Galley JD, Bailey MT. Impact of stressor exposure on the interplay between commensal microbiota and host inflammation. Gut Microbes. (2014) 5:390–6. doi: 10.4161/gmic.28683
122. Allen AP, Hutch W, Borre YE, Kennedy PJ, Temko A, Boylan G, et al. Bifidobacterium longum 1714 as a translational psychobiotic: modulation of stress, electrophysiology and neurocognition in healthy volunteers. Transl Psychiatry. (2016) 6:e939. doi: 10.1038/tp.2016.191
123. Messaoudi M, Lalonde R, Violle N, Javelot H, Desor D, Nejdi A, et al. Assessment of psychotropic-like properties of a probiotic formulation (Lactobacillus helveticus R0052 and Bifidobacterium longum R0175) in rats and human subjects. Br J Nutr. 105:755–64. doi: 10.1017/S0007114510004319
Keywords: Alzheimer's disease, microbiota-gut-brain axis, dysbiosis, probiotic, neuropsychiatric symptoms
Citation: Queiroz SAL, Ton AMM, Pereira TMC, Campagnaro BP, Martinelli L, Picos A, Campos-Toimil M and Vasquez EC (2022) The Gut Microbiota-Brain Axis: A New Frontier on Neuropsychiatric Disorders. Front. Psychiatry 13:872594. doi: 10.3389/fpsyt.2022.872594
Received: 09 February 2022; Accepted: 12 May 2022;
Published: 01 June 2022.
Edited by:
Hanna Karakula-Juchnowicz, Medical University of Lublin, PolandReviewed by:
Santosh Jadhav, Institute of Neuroimmunology (SAS), SlovakiaPeng Liu, Shenyang Pharmaceutical University, China
Copyright © 2022 Queiroz, Ton, Pereira, Campagnaro, Martinelli, Picos, Campos-Toimil and Vasquez. This is an open-access article distributed under the terms of the Creative Commons Attribution License (CC BY). The use, distribution or reproduction in other forums is permitted, provided the original author(s) and the copyright owner(s) are credited and that the original publication in this journal is cited, in accordance with accepted academic practice. No use, distribution or reproduction is permitted which does not comply with these terms.
*Correspondence: Manuel Campos-Toimil, bWFudWVsLmNhbXBvcyYjeDAwMDQwO3VzYy5lcw==