- 1Institute of Translational Biomedicine, Saint Petersburg State University, Saint Petersburg, Russia
- 2Biological Faculty, Saint Petersburg State University, Saint Petersburg, Russia
- 3Saint Petersburg University Hospital, Saint Petersburg, Russia
Attention deficit hyperactivity disorder (ADHD) is manifested by a specific set of behavioral deficits such as hyperactivity, impulsivity, and inattention. The dopamine neurotransmitter system is postulated to be involved in the pathogenesis of ADHD. Guanfacine, a selective α2A-adrenoceptor agonist, is prescribed for ADHD treatment. ADHD also is known to be associated with impairment of multiple aspects of cognition, including spatial memory, however, it remains unclear how modulation of the norepinephrine system can affect these deficits. Hyperdopaminergic dopamine transporter knockout (DAT-KO) rats are a valuable model for investigating ADHD. The DAT-KO rats are hyperactive and deficient in spatial working memory. This work aimed to evaluate the effects of noradrenergic drugs on the fulfillment of spatial cognitive tasks by DAT-KO rats. The rats were tested in the Hebb – Williams maze during training and following noradrenergic drugs administration. The efficiency of spatial orientation was assessed as to how fast the animal finds an optimal way to the goal box. Testing in a new maze configuration allowed us to evaluate the effects of drug administration after the acquisition of the task rules. The behavioral variables such as the distance traveled, the time to reach the goal box, and the time spent in the error zones were analyzed. It has been observed that α2A-adrenoceptor agonist Guanfacine (0.25 mg/kg) had only a minimal inhibitory effect on hyperactivity of DAT-KO rats in the maze but significantly ameliorated their perseverative pattern of activity and reduced the time spent in the error zones. In contrast, α2A-adrenoceptor antagonist Yohimbine, at the dose of 1 mg/kg, increased the distance traveled by DAT-KO rats and elevated the number of perseverative reactions and the time spent in the error zones. Guanfacine caused minimal effects in wild-type rats, while Yohimbine altered several parameters reflecting a detrimental effect on the performance in the maze. These data indicate that modulation of α2A-adrenoceptor activity potently affects both dopamine-dependent hyperactivity and cognitive dysfunctions. Similar mechanisms may be involved in the beneficial effects of Guanfacine on cognitive deficits in ADHD patients. This study further supports the translational potential of DAT-KO rats for testing new pharmacological drugs.
Introduction
Attention deficit hyperactivity disorder (ADHD), a neurodevelopmental mental disorder, is characterized by inattention, hyperactivity, and impulsive behavior (1, 2). The ADHD arises in childhood and may persist in adulthood (3, 4). The abnormalities in memory processes were also found in patients with ADHD (5–11). The disruption of spatial working memory in ADHD cases and different approaches to reveal these abnormalities were described in several investigations, including studies performed with the use of a virtual Hebb-Williams maze. In some studies, deficits of attention and spatial working memory are discussed together because they are difficult to separate (12–14). Several hypotheses explain mechanisms underlying this disorder that have not yet been fully validated. However, the key role of abnormalities in the dopamine and the norepinephrine systems in ADHD development was proposed (15, 16).
The dopamine (DA) system is involved in complex behaviors such as decision making (17), motivation (18, 19), reward evaluation (20), social behavior (21), and goal-directed behavior (22, 23). Among several genes associated with the pathogenesis of ADHD, the most noticeable is the gene encoding the dopamine transporter (DAT) (24, 25). It is assumed that norepinephrine (NE) also plays an important role in the pathophysiology of ADHD (26–28). Recent investigations indicated the role of norepinephrine transporter (NET) in ADHD development (29). The NE system is involved in attention processes related to prefrontal cortex (PFC) functions. The PFC receives inputs both from noradrenergic and dopaminergic systems (30–32) and interaction between them seem to be critical for alterations of the PFC functions observed in ADHD (31, 33, 34). It is suggested that the DA role is mainly associated with reward expectancy, whereas NE is involved in the evaluation of the goal and rules of the task (35). Thus, both DA and NE are crucial for PFC cognitive function and probably cannot act independently of each other.
Animal model studies can be helpful for understanding the ADHD mechanisms and elaboration of new diagnostic and therapeutic strategies (22, 36–38). These rodent studies revealed that DA and NE play critical roles in working memory, top-down control, and cognitive flexibility. Low and medium levels of NE and DA in the PFC were found to improve cognitive functions, whereas high concentrations of catecholamines in the PFC negatively affected its functions, indicating that the optimal levels of NE and DA in the PFC are necessary for its proper functioning (39). The blockade of α2A-adrenoceptors in the PFC leads to the ADHD–like behaviors in rodents, including heightened impulsivity and locomotor hyperactivity. In contrast, stimulation of alpha-2-adrenoceptors in the PFC ameliorates these behavioral abnormalities. Thus, effective ADHD treatments should aim at facilitating and optimizing catecholamine transmission in the PFC (40).
The strain of rats with deletion of the DAT gene (DAT knockout rats, DAT-KO rats) was developed in order to investigate the cognitive abnormalities arising from dopamine system dysfunction (37). DAT-KO rats have no dopamine re-uptake and thus have spontaneously elevated extracellular dopamine levels. As a result, they demonstrate novelty-driven hyperactivity and increased stereotypy as well as impaired sensorimotor gating, decreased Y-maze spontaneous alternation, and poor performance of operant tasks (37, 41). Furthermore, they demonstrate deficient spatial working memory and perseverations in various tasks (37, 38, 41–43). Numerous investigations described the dependence of spatial tasks solution on DA transmission (35, 44–46). Accordingly, spatial task fulfillment was impaired in DAT-KO rats (43).
Some drugs prescribed for ADHD treatment in patients affect the NE system. Guanfacine (GF), an α2A-adrenoceptor agonist, was approved by FDA for ADHD treatment in 2009. It directly stimulates postsynaptic α2A-adrenoceptor in the central nervous system and thereby enhances NE neurotransmission (47, 48). The α2A-adrenoceptors are most densely represented in the PFC (30, 49). Guanfacine acts directly on postsynaptic alpha-2A adrenoceptors in the PFC and has beneficial effects on regulating PFC functions (50, 51). The blockade of these receptors by α2-adrenoceptor antagonist Yohimbine (YOH) in the PFC impairs working memory, weakens go-no-go responding, increases locomotor activity in animals. YOH is also used to investigate psychomotor task performance and probe working memory in humans (52–56).
Modulation of DAT-KO rat cognitive processes might result from tenable interrelationships between the noradrenergic and the dopaminergic systems. Several brain areas are critical for complex behaviors in which the noradrenergic and dopamine systems interact closely (16, 51). However, the major interactions between these systems occur in the cortical areas that receive dense NE innervation from the L (57, 58) and dopamine projections from the Ventral Tegmental Area and are responsible for memory and attention (32, 35).
This study aimed to evaluate the effects of α2A-adrenergic drugs on the fulfillment of spatial cognitive tasks in DAT-KO rats.
Materials and Methods
Animals
In 10 DAT-KO and 10 control wild-type (WT) rats, males of the same age (3–4 months), were used in the experiments. All experimental procedures were conducted in compliance with The Regulations on Research Using Experimental Animals (Order of Ministry of Health #742), FELASA and Rus-LASA requirements regarding the care and treatment of laboratory animals, and the Ethics committee of Saint Petersburg State University, St. Petersburg, Russia No. 131-03-4 of 24 September 2018.
Before the experiments, rats were maintained in IVC cages (RAIR IsoSystem World Cage 500; Lab Products, Inc.) with free access to food and water, at a temperature of 22 ± 1°C, 50–70% relative humidity and a 12 h light/dark cycle (light from 9 am). Experiments were carried out between 2 pm and 6 pm. For 5 days before the training, rats received food at a ratio of 90% of their regular diet (BioPro, Russia). Each animal was weighed daily prior to the experiment during all the experiments’ duration. At the start of the experiments, the bodyweight of DAT-KO rats was 234.8 ± 1.6 g and was lower than that of WT rats (313.4 ± 2.2 g). At the end of the experiments, the bodyweight of DAT-KO rats was 224.3 ± 1.9 g, and in WT rats, body weight was 269.7 ± 4.8 g.
Apparatus
We used the Hebb-Williams maze to study animal spatial working memory (59, 60). The Hebb – Williams maze consists of a square area, 75 × 75 cm, with 25 cm walls. One corner is designated as the start of the maze, and the opposing corner contains a goal box with a food well for reward (Figure 1). After testing each animal, the maze surfaces were rubbed with peroxide solution to prevent odor influence.
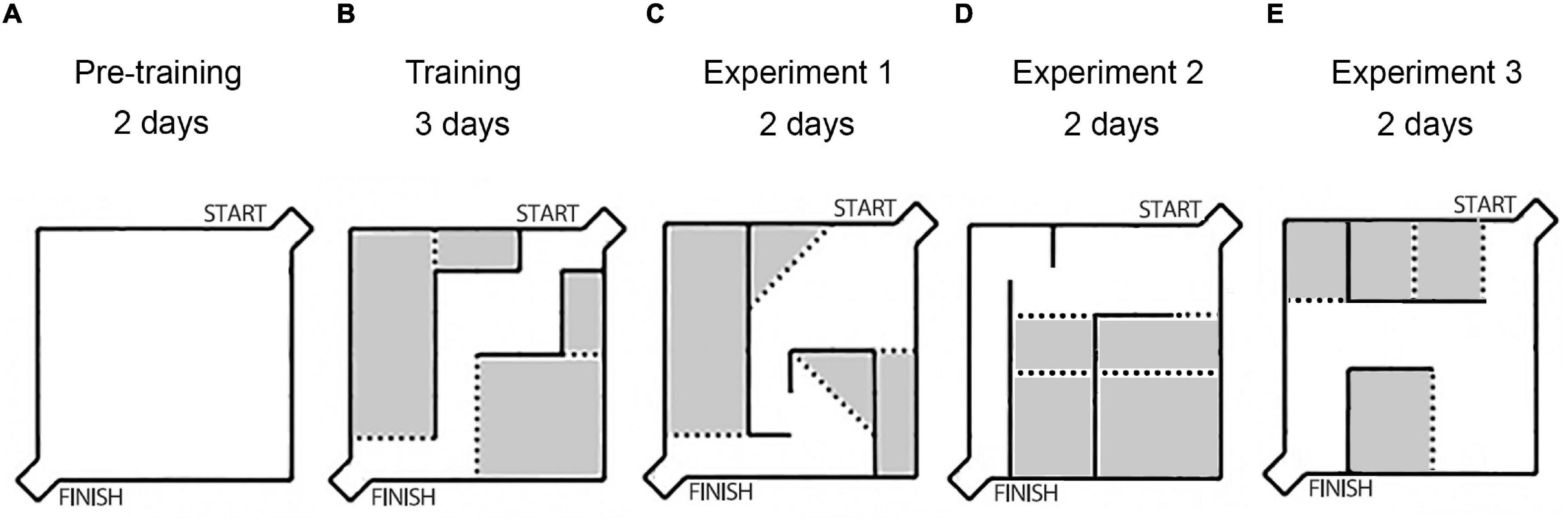
Figure 1. The scheme of the experiments: layouts of the Hebb-Williams maze arenas used in experiments. (A) The wall-less maze (pre-training period). (B) The unchanging walls arena, training period, without any injections. (C) The arena after saline injections. (D) The arena after Guanfacine (GF) injections. (E) The arena after Yohimbine (YOH) injections. The gray color indicates the error zones of the maze.
The behavioral task consisted of searching the path from start to finish to obtain food reinforcement. The interior walls of the maze are moveable, which allows the creation of new arena layouts and different routes through the maze (Figures 1B–E). Different arena layouts were used to diminish habituation’s influence on learning behavioral task rules. Each new arena was coupled with saline or drug injections.
Task Procedure
Pre-training and Training Periods
The pre-training period included a familiarization procedure in a wall-less maze during the first 2 days (Figure 1A), 10 pieces of popcorn breakfast loops (produced by Nestle S.A.) were spread out on the floor surface of the maze, and rats were allowed to individually explore the maze during 10 min. Then rats were trained in the unchanging maze arena (Figure 1B) during 3 days (three trials for each animal per day) with reward only in a goal box food well for habituation to the maze and task rules acquisition.
The Experimental Design
Experiment 1 – All rats received saline injections (0.9% NaCl, i.p.), 30 min before each testing and were trained in the new arena configuration for 2 days (Figure 1C). After it the following drugs were used: Experiment 2 (Figure 1D) – Guanfacine (GF; 0.25 mg/kg, i.p., 60 min before testing, Sigma, United States), and Experiment 3 (Figure 1E) – Yohimbine (YOH; 1 mg/kg, i.p., 10 min before testing, Sigma, United States). Each drug was used for two consecutive days. During drug administration the maze configuration was modified every 2 days. Each drug application followed a 2-day drug-free interval.
The behavioral variables such as the distance traveled, the time to reach the goal box, the number of entries into the error zones, time spent in the error zones and number of return were measured and analyzed by a video tracking system (EthoVision XT, Noldus Information Technology, VA) with the video camera being placed above the maze.
Statistical Analysis
All values were averaged over all trials for 2 days per animal, and then groups of rats were compared. The data were presented as mean ± SEM; p < 0.05 was considered statistically significant for all tests. Preliminary estimation of the data distribution normality (Gaussian distribution) was performed using the Kolmogorov–Smirnov test. The paired Mann-Whitney tests and one-way Kruskal–Wallis test combined with Dunn’s multiple-comparison post hoc test were used for statistical analysis.
Results
The spontaneous hyperactivity of DAT-KO rats was clearly revealed during the pre-training period, when their activity was significantly higher than in WT rats (Figure 2). The distance traveled (Figure 2A) was significantly higher in DAT-KO rats in comparison to WT rats (280.9 ± 36.9 (DAT-KO) vs. 89.5 ± 5.1 (WT), p < 0.001; one-way Kruskal–Wallis test combined with Dunn’s multiple-comparison post hoc test).
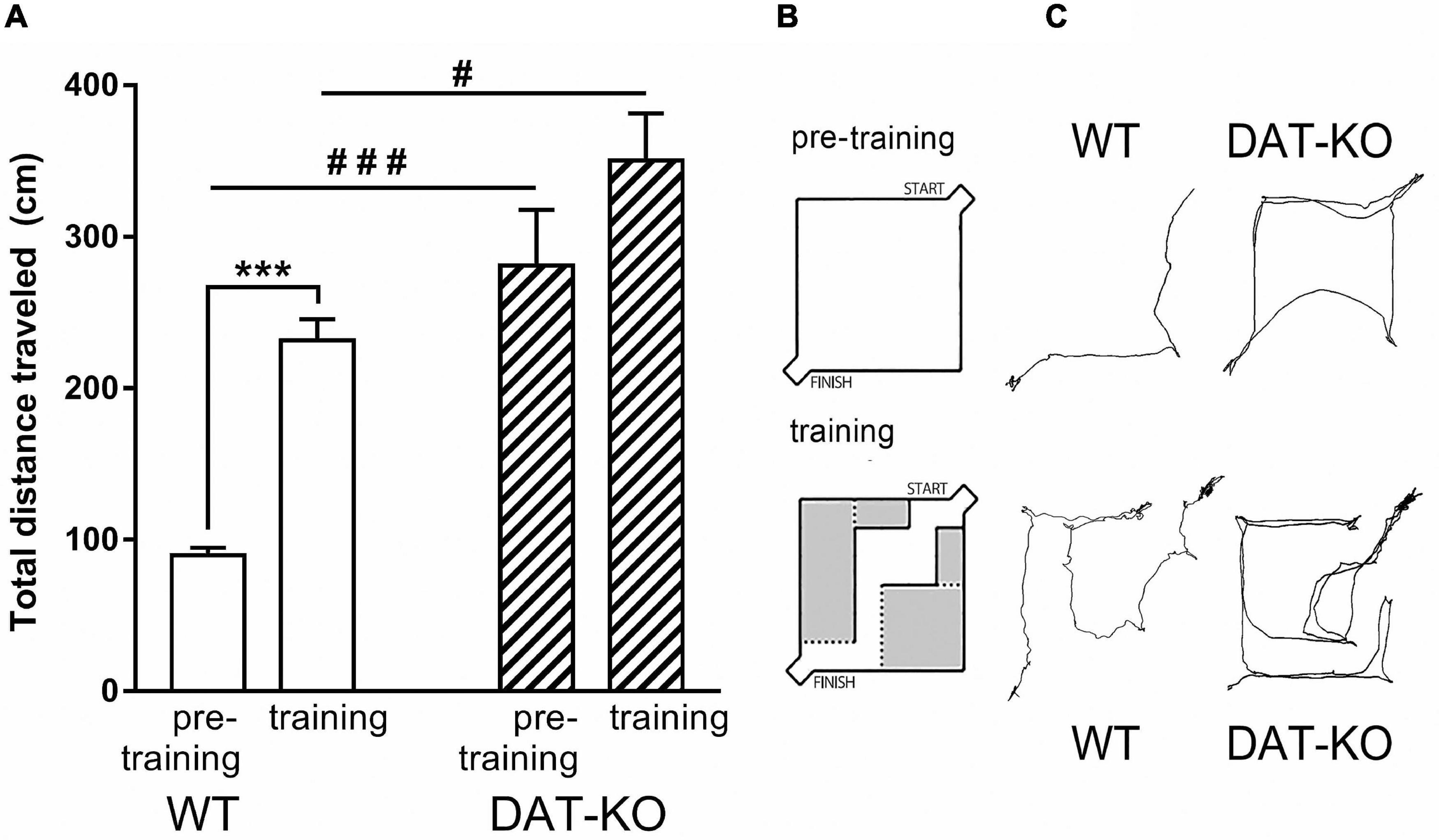
Figure 2. The distances traveled during pre-training and training periods in WT and DAT-KO rats. (A) The distances traveled during 30c, #p < 0.05; ###p < 0.001; one-way Kruskal–Wallis test combined with Dunn’s multiple-comparison post hoc test; ***p < 0.001; Mann Whitney paired test. (B) The wall-less arena in pre-training period and arena in training period. (C) Comparison of visual tracking samples; WT, wild-type; DAT-KO, dopamine transporter knockout rats.
We compared behavioral parameters of DAT-KO and WT rats during pre-training and training periods (Figure 2A). In both cases DAT-KO rats traveled significantly longer distances than WT rats. The pre-training in the wall-less arena reflects the habituation to the new environment, while the arena with internal walls allows investigation of the spatial memory and the acquisition of the task rules. WT rats probably try to remember the optimal route to the finish box and carefully investigate the arena hence their motor activity and distance traveling increase, while hyperactive DAT-KO rats are not able to do it due to their inattentiveness and decreased spatial memory (Figures 2B,C).
Following training (Figure 2A), distances traveled was significantly increased in WT rats (from 89.5 ± 5.1 to 231.5 ± 13.8; p < 0.001; Mann Whitney paired test) up to levels observed in untrained DAT-KO rats. In contrast, no significant increase was found during training in DAT-KO rats (280.9 ± 36.0 vs. 350.4 ± 31.0). It should be noted that a substantial change of the internal context of the maze has markedly modified the rat’s behavior. These changes may likely be connected with an interaction of two processes – habituation and learning.
Furthermore, in three experimental sessions (Experiment 1 – vehicle administration; Experiment 2 – GF, 0.25 mg/kg administration, and Experiment 3 – YOH, 1 mg/kg administration), we compared behavioral parameters of DAT-KO and WT rats in the Hebb-Williams maze. The data obtained were compared between two groups of rats and two drugs used.
During all experimental sessions, DAT-KO rats traveled significantly longer distances (Figure 3A) in comparison with WT rats irrespective of the drugs administered (924.1 ± 134.8 vs. 367.1 ± 21.6 for saline, p < 0.05; 763 ± 112 vs. 351.9 ± 26.9 for GF, p < 0.05; 2062.1 ± 472.2 vs. 268.8 ± 21.2 for YOH, p < 0.001; one-way Kruskal–Wallis test combined with Dunn’s multiple-comparison post hoc test). The DAT-KO rats showed no differences in distances traveled after GF compared to saline administration, whereas YOH administration increased distances covered (924.1 ± 134.8 (saline) vs. 2062.1 ± 472.2 (YOH); p < 0.05; Mann Whitney paired test). After GF injections, the distances traveled by WT rats, just as in DAT-KO rats, did not differ compared to saline injections but slightly decreased after YOH administration (367.1 ± 21.6 (saline) vs. 268.8 ± 21.2 (YOH); p < 0.05; Mann Whitney paired test).
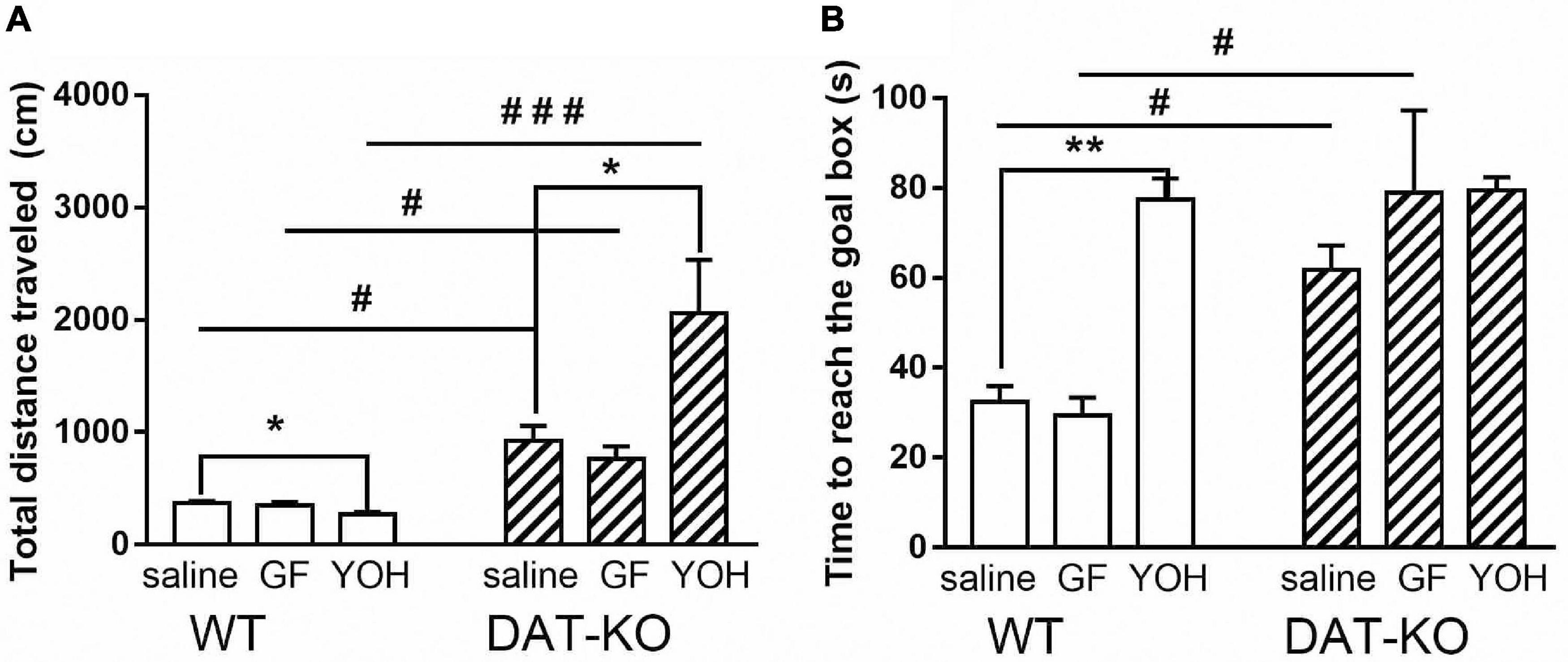
Figure 3. Comparison of distances traveled (A) and time to reach the goal box (B) by DAT-KO and WT rats during three experimental sessions; #p < 0.05; ###p < 0.001; one-way Kruskal–Wallis test combined with Dunn’s multiple-comparison post hoc test; *p < 0.05; **p < 0.01; Mann-Whitney test; WT, wild-type; DAT-KO, dopamine transporter knockout rats.
The time to reach the goal box (Figure 3B) also significantly increased (p < 0.05; one-way Kruskal–Wallis test combined with Dunn’s multiple-comparison post hoc test) in DAT-KO rats in comparison with their WT counterparts both after saline (61.8 ± 5.4 vs. 32.5 ± 3.5; p = 0.034) and GF (78.9 ± 18.3 vs. 29.5 ± 3.9; p = 0.01) injections, and did not differ after YOH administration (Figure 3B). In both groups of rats, no differences in task fulfillment duration after rats’ GF administrations were found. YOH did not significantly change the time to reach the goal box in DAT-KO rats, although it significantly increased this parameter in WT rats (77.5 ± 4.7) compared to vehicle controls (32.5 ± 3.5; p < 0.001, Mann Whitney paired test).
One of the core features of hyperactive DAT-KO animals is the perseverative (stereotypical) pattern of locomotor activity in locomotor activity boxes and increased level of perseverative errors in mazes assessing cognitive performances (43, 61). The analysis of the number of return runs showed (Figure 4A) that the perseverative activity is significantly higher in DAT-KO rats than in WT rats (p < 0.001; one-way Kruskal–Wallis test combined with Dunn’s multiple-comparison post hoc test). Comparison of stereotypical activity in DAT-KO rats after drugs administration showed that GF injections significantly decreased the occurrence of the perseverative reactions [2.4 ± 0.5 (saline) vs. 1.2 ± 0.03 (GF); p = 0.003; Mann Whitney paired test], whereas YOH injections markedly increased perseverative activity [2.4 ± 0.5 (saline) vs. 4.6 ± 0.6 (YOH); p < 0.05; Mann Whitney paired test]. WT rats showed a very low level of perseverative activity (Figure 4A), and no drugs affected it.
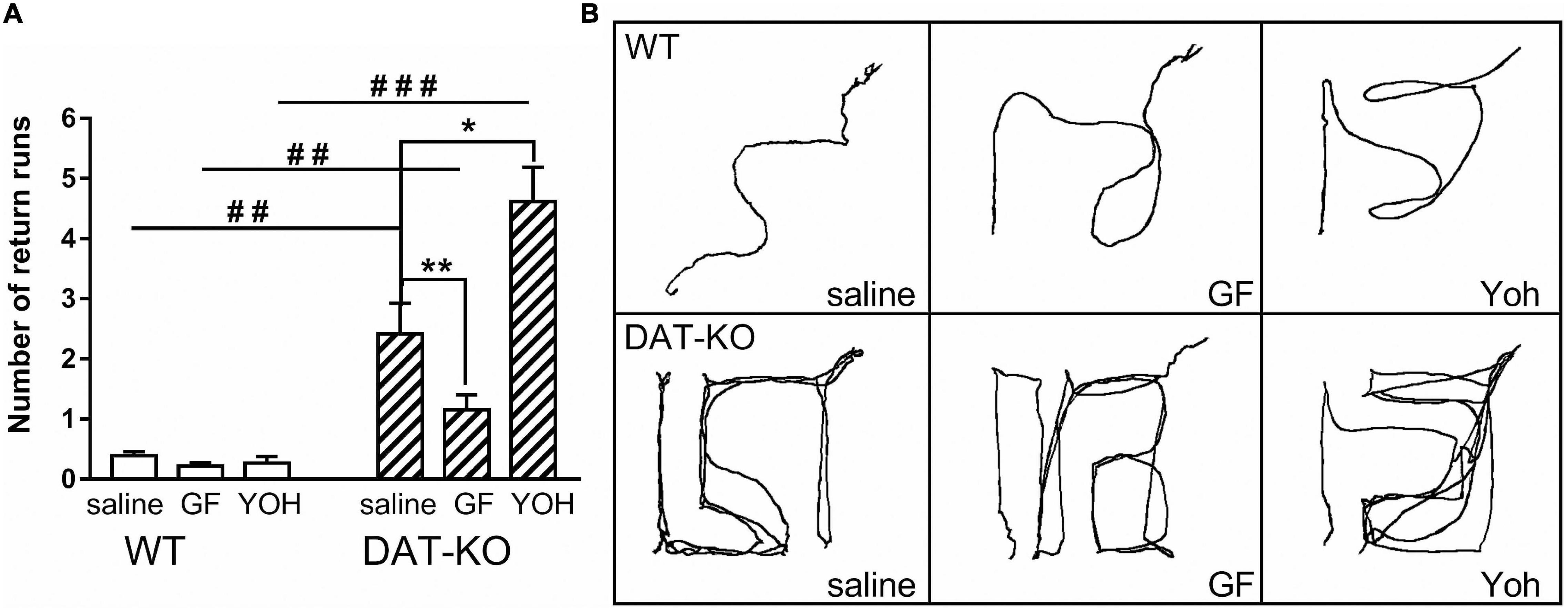
Figure 4. Comparison of the number of return runs reflecting stereotypical (perseverative) patterns of activity (A) and examples of movement patterns (B) in WT and DAT-KO rats in Experiment 1 (saline injections), Experiment 2 (GF injections), and Experiment 3 (YOH injections); ###p < 0.001; ##p < 0.01; one-way Kruskal–Wallis test combined with Dunn’s multiple-comparison post hoc test; *p < 0.05; **p < 0.01; Mann Whitney paired test; WT, wild-type; DAT-KO, dopamine transporter knockout rats.
The perseverative patterns of locomotor activity of DAT-KO animals are also evident when comparing video tracks of the maze exploration during reinforcement searching (Figure 4B). The wild-type animals chose an optimal path to the goal box, while the knockout rats made numerous returns to the start zone, also repeatedly visited the error zones.
As described previously (43), the DAT-KO rats have deficient spatial memory. In the present study, spatial navigation in DAT-KO rats was less efficient than in WT rats. Saline-treated DAT-KO rats spent more time in error zones [28.7 ± 1.7 (DAT-KO) vs. 14.7 ± 1.8 (WT); p < 0.001; one-way Kruskal–Wallis test combined with Dunn’s multiple-comparison post hoc test] and visited them more often [6.1 ± 1.1 (DAT-KO) vs. 1.4 ± 0.2 (WT); p = 0.0004; one-way Kruskal–Wallis test combined with Dunn’s multiple-comparison post hoc test] in comparison to WT rats (Figures 5A,B). The decrease in the time spent in the error zones after GF administration reflects the improvement of task fulfillment by DAT-KO rats [19.7 ± 2.2 (GF) vs. 28.7 ± 1.7 (saline); p = 0.003; Mann Whitney paired test] (Figure 5A). In contrast, the injection of YOH produced a significant increase of this parameter [40.9 ± 2.5 (YOH) vs. 28.7 ± 1.7 (saline); p = 0.016; Mann-Whitney paired test]. It was found (Figure 5A) that after YOH injections, DAT-KO rats spent a significantly longer time in the error zone than WT rats [40.9 ± 2.5 (DAT-KO) vs. 24.7 ± 2.6; p = 0.0005; one-way Kruskal–Wallis test combined with Dunn’s multiple-comparison post hoc test]. Both drugs tested in WT rats led to an increase in the time spent in the error zones, but only after YOH they remained in these zones significantly longer (19.2 ± 2.4) in comparison with vehicle conditions (14.7 ± 1.8; p = 0.008; Mann Whitney paired test). It should be noted that time spent in the error zones by WT rats was less than in DAT-KO rats after saline and YOH injections.
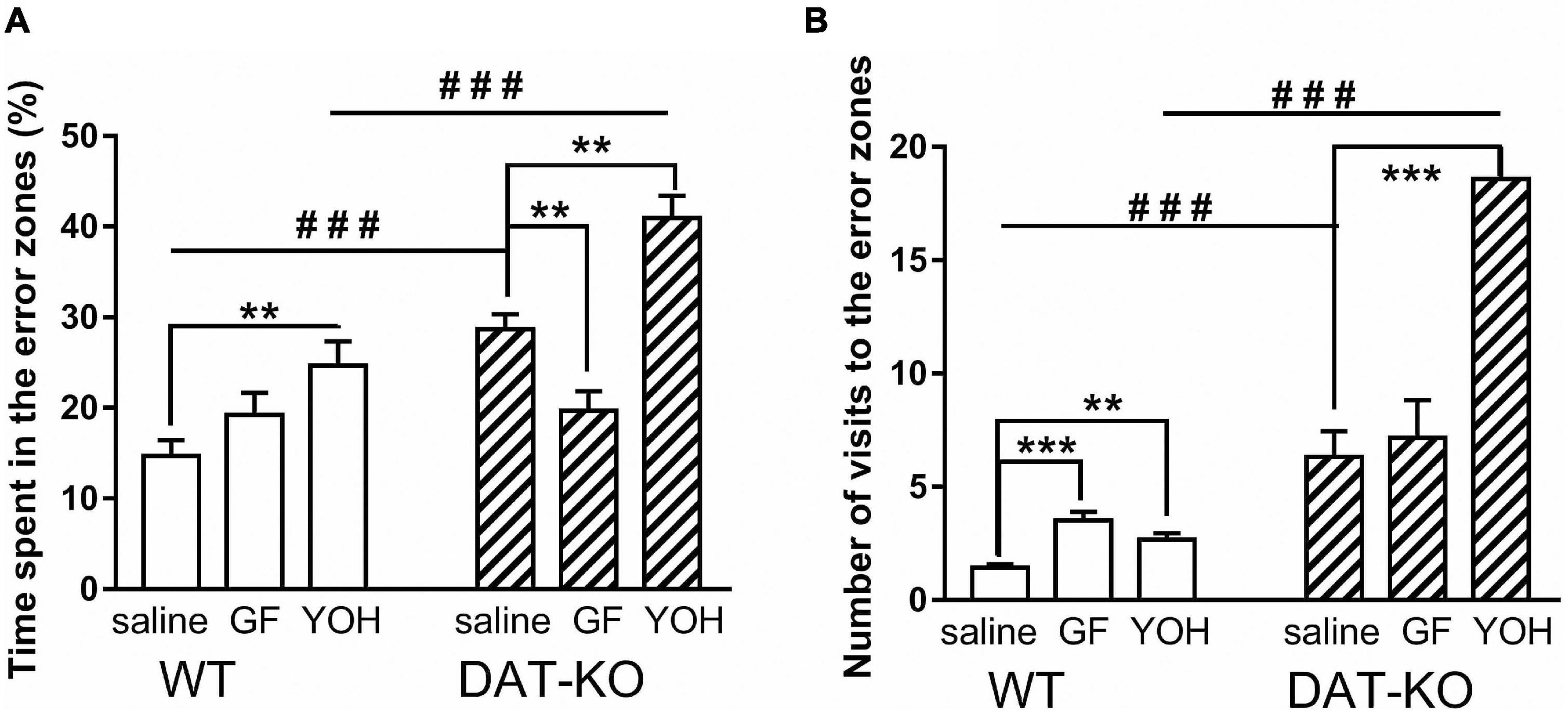
Figure 5. Comparison of the time spent in the error zones of the maze (A) and number of visits to the error zones (B) of WT and DAT-KO rats; ###p < 0.001; one-way Kruskal–Wallis test combined with Dunn’s multiple-comparison post hoc test; **p < 0.01; ***p < 0.001; Mann Whitney paired test; WT, wild-type; DAT-KO, dopamine transporter knockout rats.
Similar results were obtained following analysis of the number of error zone visits (Figure 5B). The number of visits to the error zones by WT rats also was significantly lower than in DAT-KO rats for saline injection [1.4 ± 0.2 (WT) vs. 6.3 ± 1.1 (DAT-KO); p = 0.0002; one-way Kruskal–Wallis test combined with Dunn’s multiple-comparison post hoc test] and for YOH injection [2.7 ± 0.3 (WT) vs. 18.6 ± 2.3; p = 0.0006; one-way Kruskal–Wallis test combined with Dunn’s multiple-comparison post hoc test]. Interesting, that the injection of YOH produced a significant increase the number of error zone visits as in WT [1.4 ± 0.2 (saline) vs. 2.7 ± 0.3 (YOH); p = 0.057; Mann Whitney paired test], as in DAT-KO rats [6.3 ± 1.1 (saline) vs. 18.6 ± 2.3; p < 0.001; Mann Whitney paired test] (Figure 5B).
Discussion
This study evaluated the effects of noradrenergic drugs on DAT-KO rats’ cognitive functions during spatial memory task performance. DAT plays a critical role in regulating both the intraneuronal and extracellular DA homeostasis by controlling the DA re-uptake (62). DAT-KO rats with the deleted DAT gene demonstrate elevated extracellular dopamine levels, spontaneous hyperactivity, perseverations, and cognitive deficits (37). Numerous investigations have documented hyperactivity, abnormal impulsivity, and inattentiveness in DAT-KO rats in various experimental settings (38, 41, 42, 63). Furthermore, our previous studies showed that DAT-KO rats, when performing spatial tasks in the 8-arm radial maze, had a high level of perseverative errors and fulfilled tasks less effectively than their WT counterparts indicative of deficit in working memory (43). We also found that DAT-KO rats can learn non-spatial object recognition tasks under the conditions of novel object presentations and can store this motor task in memory for at least 3 months. Thus, they have a deficiency in learning the cognitive task but can keep in memory the learned task for 3 months and fulfill it even better than during training (64). These data indicate that disruption of the dopamine re-uptake affects cognitive task learning in DAT-KO rats, and proves their translational value to investigate memory and attention deficits related to ADHD.
The role of DA and NE in terms of their differential participation in memory processes is well described. The role of DA in the modulation of the PFC functions was demonstrated in multiple studies in various animal species and humans. It is believed that the dopamine system participates in memory processes through its involvement in reward evaluation. At the same time, the Locus Coeruleus noradrenergic system may exert modulatory effects on the PFC functions through its role in task rule acquisition (35). The DA and NE levels were found to be phasically increased in the rat PFC during cognitive task performance (65). It is speculated that convergence of the DA and NE signaling pathways might have parallel neurophysiological effects. There are data that extracellular DA in the cortex is co-released with NE from noradrenergic terminals, and this co-release is mainly controlled by the α2A-adrenoceptors (66, 67). The α2A-adrenoceptors are essential for the PFC regulation (68, 69). D1 receptors can regulate the sustained firing of the PFC neurons in a delay period in the delayed response task (70). It is thus generally believed that the optimal PFC functions are likely dependent on NE stimulation of the postsynaptic α2A-adrenoceptors and dopaminergic stimulation of D1 receptors (51, 71). Nevertheless, it remains unclear how DA and NE systems coordinate their action to optimize the PFC functions (32).
Here, we investigated the influence of the α2A-adrenoceptor agonist and antagonist (Guanfacine and Yohimbine, respectively) on spatial memory of DAT-KO rats. It is known that α2A-adrenoceptor agonist GF can improve working memory in aged monkeys and rats and shows therapeutic efficacy in ADHD patients (50). It is believed that GF acts within the PFC via postsynaptic α2A-adrenoceptor on the dendritic spines inhibiting cAMP-PKA opening K+ channel signaling and causing activation of PFC neurons leading to the improvement of cognitive functions (51). In spontaneously hypertensive rats (SHR), GF caused a decrease in DA release in the prefrontal cortex, but NE concentrations were increased, and thus the NE system appears to be hyperactive as a result of α2A-adrenoceptor activation. These results indicate that GF can improve deficient NE modulation of neuronal circuits in the PFC in this animal model of ADHD (72). At the same time, the blockade of the α2A-adrenoceptors with YOH leads to a deficiency in impulsive decision making in delayed response tasks in rats (56, 73–75). Furthermore, the acute administration of YOH induces ADHD–like behaviors by increasing locomotor hyperactivity and impulsivity of rats (76). Infusion of YOH into the PFC in monkeys impaired working memory control of attention (77).
As with other experimental settings, DAT-KO rats demonstrated pronounced hyperactivity in the maze compared to WT animals. However, their activity level has not been significantly altered during training to the task compared to the pre-training period in wall-less maze. In contrast, WT animals significantly increased the distance traveled during training in comparison with the pre-training period. It is likely that in WT rats, the level of exploratory activity and attention was higher than in DAT-KO rats, and thus, the distances traveled in the maze increased as they were learning the task.
The level of motivation is also essential for task performance. In our study, we compared the preference of reward to regular food in DAT-KO and WT rats. While the reward preference seems to be similar in both groups of rats, the difference in body weight decreases during experimental tasks may suggest that potential minor alterations in the motivation of DAT-KO rats can not be fully excluded. Furthermore, potential contribution of transient anxiety phenotype in DAT-KO rats (38) to the findings observed can not be ruled out.
Despite their prominent hyperactivity, trained drug-naive DAT-KO rats took longer to reach the goal box than their WT counterparts. In addition, WT rats seem to acquire task rules faster than DAT-KO rats, and the drugs used influenced them differently than spontaneously hyperactive DAT-KO rats. It appears likely that due to inattentiveness and the deficient spatial working memory, DAT-KO rats showed poor performance in solving tasks and searching for a correct path to the goal. Furthermore, the DAT-KO rats also demonstrated an increased number of returned runs indicating the perseverative pattern of activity in task solving.
Guanfacine administration had only a minimal inhibitory effect on the distances traveled by DAT-KO rats in the maze. In contrast, YOH administration significantly increased the distance traveled. Likely, the potential inhibitory action of GF on hyperactivity was not revealed to a full extent due to experimental conditions in the maze. Therefore, further studies in locomotor activity monitors are necessary to evaluate the inhibitory action of GF in hyperactive DAT-KO rats and compare it to that of amphetamine and methylphenidate in DAT-KO rats (37) and mice (61).
Both GF and YOH did not affect the time necessary to reach the goal box compared to untreated DAT-KO rats but exerted opposite effects on the number of abnormal return runs, reflecting a perseverative pattern of locomotor activity of mutants. It is well known that hyperactivity in DAT-KO rats is often accompanied by stereotypical (perseverative) patterns of activity in various settings (38, 41, 43). In hyperdopaminergic DAT-KO rats, GF decreased, and YOH increased this type of activity, indicating that GF may optimize the PFC functions and thus diminish the ADHD-like behavior while YOH is aggravating it.
Similar opposite effects of these treatments were observed when the time spent in the maze error zones was analyzed. The DAT-KO rats are known to have deficient working memory (43). The mutants indeed spent more time in the error zones in the Hebb-Williams maze time, indicating an impaired level of task acquisition. Administration of GF ameliorated this deficit, while YOH exacerbated it. These data strongly indicate that GF can improve the consolidation of spatial information under the condition of hyperdopaminergia and consequently can ameliorate their spatial memory deficit. The role played by the noradrenergic modulation in memory consolidation processes was described in numerous investigations (57, 78, 79).
The effects of GF in WT rats were not so conclusive due to the lack of hyperdopaminergia to reveal its beneficial actions. At the same time, YOH caused locomotor activation, increased the duration of task fulfillment, time spent in the error zones of the maze, and the number of visits to the error zones in WT rats indicating impaired cognitive function following α2A-adrenoceptor blockade. These data support previous observations showing that YOH at low doses (0.5–2 mg/kg, i.p.) can induce locomotor activation in rats (73, 80) and increase impulsivity in the five-choice serial reaction time task (74). It was also shown that Guanfacine induces activation in the PFC leading to the decline of hyperactivity in DAT-KO rats, whereas Yohimbine may provoke hyperactivity by increasing dopamine synthesis and intraneuronal dopamine stores (81).
Our experiments found the opposite effects of agonist and antagonist of α2A-adrenoceptors on the spatial working memory of hyperdopaminergic DAT-KO rats. This might result from different influences exerted by the PFC on the subcortical structures via the noradrenergic and dopaminergic systems. In addition to working memory, Guanfacine, the agonist of α2A-adrenoceptor improves many PFC functions (51). It has been suggested that in SHR rats, abnormal behaviors may result from a disbalance between increased noradrenergic and decreased dopaminergic systems regulation in PFC (69). The complementary role of DA and NE in the PFC and their interactions for memory facilitation was discussed by other investigators (32, 67). It was proposed that beneficial effects of GF arise via strengthening PFC network connectivity as a consequence of NE actions on postsynaptic α2A-adrenoceptors dendrite spines in PFC (51, 82).
Conclusion
These results indicate that Guanfacine may positively affect ADHD-like abnormal behaviors of DAT-KO rats. This drug improves spatial memory deficit in DAT-KO rats, thus likely contributing to attention improvement. The treatment of DAT-KO rats with Yohimbine, an antagonist of α2A-adrenoceptors, exacerbates ADHD-like behaviors. These data further support the translational value of DAT-KO animals as a useful animal model to investigate pathological processes and discover new ADHD treatments.
Data Availability Statement
The raw data supporting the conclusions of this article will be made available by the authors, without undue reservation.
Ethics Statement
The animal study was reviewed and approved by the Ethics Committee of Saint Petersburg State University, St. Petersburg, Russia.
Author Contributions
NK, AV, and RG designed the study. NK, AB, and AG performed the experiments and data analysis. NK, AI, and RG writing and editing of the manuscript. RG and AV contributed to the conceptualization and funding acquisition for the project. All authors contributed to the article and approved the submitted version.
Funding
This work was supported by the Russian Science Foundation grant number 21-75-20069. RG was supported by the project ID: 73022475 of the Saint Petersburg State University.
Conflict of Interest
The authors declare that the research was conducted in the absence of any commercial or financial relationships that could be construed as a potential conflict of interest.
Publisher’s Note
All claims expressed in this article are solely those of the authors and do not necessarily represent those of their affiliated organizations, or those of the publisher, the editors and the reviewers. Any product that may be evaluated in this article, or claim that may be made by its manufacturer, is not guaranteed or endorsed by the publisher.
References
1. Grace AA. Dysregulation of the dopamine system in the pathophysiology of schizophrenia and depression. Nat Rev Neurosci. (2016) 17:524–32. doi: 10.1038/nrn.2016.57
2. Galéra C, Cortese S, Orri M, Collet O, van der Waerden J, Melchior M, et al. Medical conditions and attention-deficit/hyperactivity disorder symptoms from early childhood to adolescence. Mol Psychiatry. (2021):1–9. doi: 10.1038/s41380-021-01357-x
3. Bonvicini C, Faraone SV, Scassellati C. Attention-deficit hyperactivity disorder in adults: a systematic review and meta-analysis of genetic, pharmacogenetic and biochemical studies. Mol Psychiatry. (2016) 21:872–84. doi: 10.1038/mp.2016.74
4. Mucci F, Carpita B, Pagni G, Vecchia AD, Bjedov S, Pozza A, et al. Lifetime evolution of ADHD treatment. J Neural Transm. (2021) 128:1085–98. doi: 10.1007/s00702-021-02336-w
5. Dowson JH, McLean A, Bazanis E, Toone B, Young S, Robbins TW, et al. Impaired spatial working memory in adults with attention-deficit/hyperactivity disorder: comparisons with performance in adults with borderline personality disorder and in control subjects. Acta Psychiatr Scand. (2004) 110:45–54. doi: 10.1111/j.1600-0447.2004.00292.x
6. Kalanthroff E, Naparstek S, Henik A. Spatial processing in adults with attention deficit hyperactivity disorder. Neuropsychology. (2013) 27:546–55. doi: 10.1037/a0033655
7. Skodzik T, Holling H, Pedersen A. Long-term memory performance in adult ADHD: a meta-analysis. J Atten Disord. (2017) 21:267–83. doi: 10.1177/1087054713510561
8. Luo X, Guo J, Liu L, Zhao X, Li D, Li H, et al. The neural correlations of spatial attention and working memory deficits in adults with ADHD. Neuroimage Clin. (2019) 22:101728. doi: 10.1016/j.nicl.2019.101728
9. Arjona Valladares A, Gómez CM, Rodríguez-Martínez EI, Barriga-Paulino CI, Gómez-González J, Diaz-Sánchez JA. Attention-deficit/hyperactivity disorder in children and adolescents: an event-related potential study of working memory. Eur J Neurosci. (2020) 52:4356–69. doi: 10.1111/ejn.14767
10. Jang KM, Kim MS, Kim DW. The dynamic properties of a brain network during spatial working memory tasks in college students with ADHD traits. Front Hum Neurosci. (2020) 14:371. doi: 10.3389/fnhum.2020.580813
11. Ortega R, López V, Carrasco X, Escobar MJ, García AM, Parra MA, et al. Neurocognitive mechanisms underlying working memory encoding and retrieval in attention-deficit/hyperactivity disorder. Sci Rep. (2020) 10:7771. doi: 10.1038/s41598-020-64678-x
12. Shore DI, Stanford L, MacInnes WJ, Klein RM, Brown RE. Of mice and men: virtual Hebb-Williams mazes permit comparison of spatial learning across species. Cogn Affect Behav Neurosci. (2001) 1:83–9. doi: 10.3758/CABN.1.1.83
13. Mattfeld AT, Whitfield-Gabrieli S, Biederman J, Spencer T, Brown A, Fried R, et al. Dissociation of working memory impairments and attention-deficit/hyperactivity disorder in the brain. Neuroimage Clin. (2016) 10:274–82. doi: 10.1016/J.NICL.2015.12.003
14. Bollmann S, Ghisleni C, Poil SS, Martin E, Ball J, Eich-Höchli D, et al. Age-dependent and –independent changes in attention-deficit/hyperactivity disorder (ADHD) during spatial working memory performance. World J Biol Psychiatry. (2017) 18:279–90. doi: 10.3109/15622975.2015.1112034
15. Nikolaus S, Antke C, Beu M, Müller HW, Nikolaus S. Cortical GABA, striatal dopamine and midbrain serotonin as the key players in compulsiveand anxiety disorders – results from in vivo imaging studies. Rev Neurosci. (2010) 21:119–40. doi: 10.1515/REVNEURO.2010.21.2.119
16. Del Campo N, Chamberlain SR, Sahakian BJ, Robbins TW. The roles of dopamine and noradrenaline in the pathophysiology and treatment of attention-deficit/hyperactivity disorder. Biol Psychiatry. (2011) 69:e145–57. doi: 10.1016/j.biopsych.2011.02.036
17. Bardgett ME, Depenbrock M, Downs N, Points M, Green L. Dopamine modulates effort-based decision making in rats. Behav Neurosci. (2009) 123:242–51. doi: 10.1037/a0014625
18. Cagniard B, Balsam PD, Brunner D, Zhuang X. Mice with chronically elevated dopamine exhibit enhanced motivation, but not learning, for a food reward. Neuropsychopharmacology. (2006) 31:1362–70. doi: 10.1038/sj.npp.1300966
19. Tripp G, Wickens JR. Neurobiology of ADHD. Neuropharmacology. (2009) 57:579–89. doi: 10.1016/j.neuropharm.2009.07.026
20. Volkow ND, Wang GJ, Newcorn JH, Kollins SH, Wigal TL, Telang F, et al. Motivation deficit in ADHD is associated with dysfunction of the dopamine reward pathway. Mol Psychiatry. (2011) 16:1147–54. doi: 10.1038/mp.2010.97
21. Homberg JR, Olivier JDA, VandenBroeke M, Youn J, Ellenbroek AK, Karel P, et al. The role of the dopamine D1 receptor in social cognition: studies using a novel genetic rat model. Dis Model Mech. (2016) 9:1147–58. doi: 10.1242/dmm.024752
22. Sagvolden T, Russell VA, Aase H, Johansen EB, Farshbaf M. Rodent models of attention-deficit/hyperactivity disorder. Biol Psychiatry. (2005) 57:1239–47. doi: 10.1016/j.biopsych.2005.02.002
23. Natsheh JY, Shiflett MW. Dopaminergic modulation of goal-directed behavior in a rodent model of attention-deficit/hyperactivity disorder. Front Integr Neurosci. (2018) 12:45. doi: 10.3389/fnint.2018.00045
24. Mick E, Faraone SV. Genetics of attention deficit hyperactivity disorder. Child Adolesc Psychiatr Clin N Am. (2008) 17:261–84. doi: 10.1016/j.chc.2007.11.011
25. Sharp SI, McQuillin A, Gurling HMD. Genetics of attention-deficit hyperactivity disorder (ADHD). Neuropharmacology. (2009) 57:590–600. doi: 10.1016/j.neuropharm.2009.08.011
26. Zametkin AJ, Rapoport JL. Neurobiology of attention deficit disorder with hyperactivity: where have we come in 50 years? J Am Acad Child Adolesc Psychiatry. (1987) 26:676–86. doi: 10.1097/00004583-198709000-00011
27. Pliszka SR, McCracken JT, Maas JW. Catecholamines in attention-deficit hyperactivity disorder: current perspectives. J Am Acad Child Adolesc Psychiatry. (1996) 35:264–72. doi: 10.1097/00004583-199603000-00006
28. Biederman J, Spencer T. Attention-deficit/hyperactivity disorder (ADHD) as a noradrenergic disorder. Biol Psychiatry. (1999) 46:1234–42. doi: 10.1016/S0006-3223(99)00192-4
29. Ulke C, Rullmann M, Huang J, Luthardt J, Becker GA, Patt M, et al. Adult attention-deficit/hyperactivity disorder is associated with reduced norepinephrine transporter availability in right attention networks: a (S,S)-O-[11C]methylreboxetine positron emission tomography study. Transl Psychiatry. (2019) 9:301. doi: 10.1038/s41398-019-0619-y
30. Ramos BP, Arnsten AFT. Adrenergic pharmacology and cognition: focus on the prefrontal cortex. Pharmacol Ther. (2007) 113:523–36. doi: 10.1016/j.pharmthera.2006.11.006
31. Gao W-J, Wang H-X, Snyder AM, Li Y-C. The unique properties of the prefrontal cortex and mental illness. In: Mantamadiotis T editor. When Things Go Wrong – Diseases and Disorders of the Human Brain. (London: InTech) (2012). doi: 10.5772/35868
32. Xing B, Li YC, Gao WJ. Norepinephrine versus dopamine and their interaction in modulating synaptic function in the prefrontal cortex. Brain Res. (2016) 1641:217–33. doi: 10.1016/j.brainres.2016.01.005
33. Levy F. Pharmacological and therapeutic directions in ADHD: specificity in the PFC. Behav Brain Funct. (2008) 4:12. doi: 10.1186/1744-9081-4-12
34. Bokor G, Anderson PD. Attention-deficit/hyperactivity disorder. J Pharm Pract. (2014) 27:336–49. doi: 10.1177/0897190014543628
35. Rossetti ZL, Carboni S. Noradrenaline and dopamine elevations in the rat prefrontal cortex in spatial working memory. J Neurosci. (2005) 25:2322–9. doi: 10.1523/JNEUROSCI.3038-04.2005
36. Russell VA. Overview of animal models of attention deficit hyperactivity disorder (ADHD). Curr Protoc Neurosci. (2011) Chapter 9:Unit9.35. doi: 10.1002/0471142301.ns0935s54
37. Leo D, Sukhanov I, Zoratto F, Illiano P, Caffino L, Sanna F, et al. Pronounced hyperactivity, cognitive dysfunctions, and BDNF dysregulation in dopamine transporter knock-out rats. J Neurosci. (2018) 38:1959–72. doi: 10.1523/jneurosci.1931-17.2018
38. Adinolfi A, Zelli S, Leo D, Carbone C, Mus L, Illiano P, et al. Behavioral characterization of DAT-KO rats and evidence of asocial-like phenotypes in DAT-HET rats: the potential involvement of norepinephrine system. Behav Brain Res. (2019) 359:516–27. doi: 10.1016/J.BBR.2018.11.028
39. Cools R, Arnsten AFT. Neuromodulation of prefrontal cortex cognitive function in primates: the powerful roles of monoamines and acetylcholine. Neuropsychopharmacology. (2021) 47:309–28. doi: 10.1038/s41386-021-01100-8
40. Arnsten AFT, Li BM. Neurobiology of executive functions: catecholamine influences on prefrontal cortical functions. Biol Psychiatry. (2005) 57:1377–84. doi: 10.1016/j.biopsych.2004.08.019
41. Cinque S, Zoratto F, Poleggi A, Leo D, Cerniglia L, Cimino S, et al. behavioral phenotyping of dopamine transporter knockout rats: compulsive traits, motor stereotypies, and anhedonia. Front Psychiatry. (2018) 9:43. doi: 10.3389/fpsyt.2018.00043
42. Vengeliene V, Bespalov A, Robmanith M, Horschitz S, Berger S, Relo AL, et al. Towards trans-diagnostic mechanisms in psychiatry: neurobehavioral profile of rats with a loss-of-function point mutation in the dopamine transporter gene. Dis Model Mech. (2017) 10:451–61. doi: 10.1242/dmm.027623
43. Kurzina NP, Aristova IY, Volnova AB, Gainetdinov RR. Deficit in working memory and abnormal behavioral tactics in dopamine transporter knockout rats during training in the 8-arm maze. Behav Brain Res. (2020) 390:112642. doi: 10.1016/j.bbr.2020.112642
44. Whishaw IQ, Dunnett SB. Dopamine depletion, stimulation or blockade in the rat disrupts spatial navigation and locomotion dependent upon beacon or distal cues. Behav Brain Res. (1985) 18:11–29. doi: 10.1016/0166-4328(85)90165-2
45. Bezu M, Maliković J, Kristofova M, Engidawork E, Höger H, Lubec G, et al. Spatial working memory in male rats: pre-experience and task dependent roles of dopamine D1– and D2-like receptors. Front Behav Neurosci. (2017) 11:196. doi: 10.3389/fnbeh.2017.00196
46. Bernhardt N, Lieser MK, Hlusicka EB, Habelt B, Wieske F, Edemann-Callesen H, et al. Learning deficits in rats overexpressing the dopamine transporter. Sci Rep. (2018) 8:1–10. doi: 10.1038/s41598-018-32608-7
47. Huss M, Chen W, Ludolph AG. Guanfacine extended release: a new pharmacological treatment option in Europe. Clin Drug Investig. (2016) 36:1–25. doi: 10.1007/s40261-015-0336-0
48. Fukuyama K, Nakano T, Shiroyama T, Okada M. Chronic administrations of guanfacine on mesocortical catecholaminergic and thalamocortical glutamatergic transmissions. Int J Mol Sci. (2021) 22:4122. doi: 10.3390/ijms22084122
49. Lee M, Mueller A, Moore T. Differences in noradrenaline receptor expression across different neuronal subtypes in macaque frontal eye field. Front Neuroanat. (2020) 14:574130. doi: 10.3389/fnana.2020.574130
50. Arnsten AFT, Cai JX, Goldman-Rakic PS. The alpha-2 adrenergic agonist guanfacine improves memory in aged monkeys without sedative or hypotensive side effects: evidence for alpha-2 receptor subtypes. J Neurosci. (1988) 8:4287–98. doi: 10.1523/jneurosci.08-11-04287.1988
51. Arnsten AFT. Guanfacine’s mechanism of action in treating prefrontal cortical disorders: successful translation across species. Neurobiol Learn Mem. (2020) 176:107327. doi: 10.1016/j.nlm.2020.107327
52. Sallee FR, Sethuraman G, Sine L, Liu H. Yohimbine challenge in children with anxiety disorders. Am J Psychiatry. (2000) 157:1236–42. doi: 10.1176/appi.ajp.157.8.1236
53. Plewnia C, Bartels M, Cohen L, Gerloff C. Noradrenergic modulation of human cortex excitability by the presynaptic α2-antagonist yohimbine. Neurosci Lett. (2001) 307:41–4. doi: 10.1016/S0304-3940(01)01921-8
54. LeBlanc-Duchin D, Taukulis HK. Behavioral reactivity to a noradrenergic challenge after chronic oral methylphenidate (Ritalin®) in rats. Pharmacol Biochem Behav. (2004) 79:641–9. doi: 10.1016/j.pbb.2004.09.021
55. Al-Kuraishy H, Al-Gareeb A. Advanced central effects of yohimbine on the cognitive function, psychomotor performance task and working memory: a randomized controlled clinical trial study. Br J Pharm Res. (2015) 5:328–35. doi: 10.9734/bjpr/2015/15255
56. Schippers MC, Schetters D, De Vries TJ, Pattij T. Differential effects of the pharmacological stressor yohimbine on impulsive decision making and response inhibition. Psychopharmacology (Berl). (2016) 233:2775–85. doi: 10.1007/s00213-016-4337-3
57. Poe GR, Foote S, Eschenko O, Johansen JP, Bouret S, Aston-Jones G, et al. Locus coeruleus: a new look at the blue spot. Nat Rev Neurosci. (2020) 21:644–59. doi: 10.1038/s41583-020-0360-9
58. Breton-Provencher V, Drummond GT, Sur M. Locus coeruleus norepinephrine in learned behavior: anatomical modularity and spatiotemporal integration in targets. Front Neural Circuits. (2021) 15:638007. doi: 10.3389/FNCIR.2021.638007
60. Raut SB, Jadhav KS, Marathe PA. Role of dopamine – D2 receptor in spatial memory retention and retrieval determined using Hebb-Williams complex maze. Indian J Physiol Pharmacol. (2014) 58:191–5.
61. Gainetdinov RR, Wetsel WC, Jones SR, Levin ED, Jaber M, Caron MC. Role of serotonin in the paradoxical calming effect of psychostimulants on hyperactivity. Science. (1999) 283:397–401. doi: 10.1126/SCIENCE.283.5400.397
62. Vaughan RA, Foster JD. Mechanisms of dopamine transporter regulation in normal and disease states. Trends Pharmacol Sci. (2013) 34:489–96. doi: 10.1016/j.tips.2013.07.005
63. Adinolfi A, Carbone C, Leo D, Gainetdinov RR, Laviola G, Adriani W. Novelty-related behavior of young and adult dopamine transporter knockout rats: implication for cognitive and emotional phenotypic patterns. Genes Brain Behav. (2018) 17:e12463. doi: 10.1111/gbb.12463
64. Kurzina NP, Volnova AB, Aristova IY, Gainetdinov RR. A new paradigm for training hyperactive dopamine transporter knockout rats: influence of novel stimuli on object recognition. Front Behav Neurosci. (2021) 15:654469. doi: 10.3389/fnbeh.2021.654469
65. Puig MV, Rose J, Schmidt R, Freund N. Dopamine modulation of learning and memory in the prefrontal cortex: insights from studies in primates, rodents, and birds. Front Neural Circuits. (2014) 8:93. doi: 10.3389/fncir.2014.00093
66. Devoto P, Flore G, Pira L, Longu G, Gessa GL. Alpha2-adrenoceptor mediated co-release of dopamine and noradrenaline from noradrenergic neurons in the cerebral cortex. J Neurochem. (2004) 88:1003–9. doi: 10.1046/J.1471-4159.2003.02239.x
67. Ranjbar-Slamloo Y, Fazlali Z. Dopamine and noradrenaline in the brain; overlapping or dissociate functions? Front Mol Neurosci. (2020) 12:334. doi: 10.3389/fnmol.2019.00334
68. Solanto MV. Neuropsychopharmacological mechanisms of stimulant drug action in attention-deficit hyperactivity disorder: a review and integration. Behav Brain Res. (1998) 94:127–52. doi: 10.1016/S0166-4328(97)00175-7
69. Russell VA. Hypodopaminergic and hypernoradrenergic activity in prefrontal cortex slices of an animal model for attention-deficit hyperactivity disorder – the spontaneously hypertensive rat. Behav Brain Res. (2002) 130:191–6. doi: 10.1016/S0166-4328(01)00425-9
70. González-Burgos G, Kröner S, Krimer LS, Seamans JK, Urban NN, Henze DA, et al. Dopamine modulation of neuronal function in the monkey prefrontal cortex. Physiol Behav. (2002) 77:537–43. doi: 10.1016/S0031-9384(02)00940-x
71. Wang M, Ramos BP, Paspalas CD, Shu Y, Simen A, Duque A, et al. α2A-adrenoceptors strengthen working memory networks by inhibiting cAMP-HCN channel signaling in prefrontal cortex. Cell. (2007) 129:397–410. doi: 10.1016/j.cell.2007.03.015
72. Sagvolden T. The alpha-2A adrenoceptor agonist guanfacine improves sustained attention and reduces overactivity and impulsiveness in an animal model of attention-deficit/hyperactivity disorder (ADHD). Behav Brain Funct. (2006) 2:41. doi: 10.1186/1744-9081-2-41
73. Andén NE, Pauksens K, Svensson K. Selective blockade of brain α2-autoreceptors by yohimbine: effects on motor activity and on turnover of noradrenaline and dopamine. J Neural Transm. (1982) 55:111–20. doi: 10.1007/BF01243754
74. Sun HS, Green TA, Theobald DEH, Birnbaum SG, Graham DL, Zeeb FD, et al. Yohimbine increases impulsivity through activation of cAMP response element binding in the orbitofrontal cortex. Biol Psychiatry. (2010) 67:649–56. doi: 10.1016/j.biopsych.2009.11.030
75. Adams WK, Barrus MM, Zeeb FD, Cocker PJ, Benoit J, Winstanley CA. Dissociable effects of systemic and orbitofrontal administration of adrenoceptor antagonists on yohimbine-induced motor impulsivity. Behav Brain Res. (2017) 328:19–27. doi: 10.1016/j.bbr.2017.03.034
76. Ma CL, Arnsten AFT, Li BM. Locomotor hyperactivity induced by blockade of prefrontal cortical α 2-adrenoceptors in monkeys. Biol Psychiatry. (2005) 57:192–5. doi: 10.1016/j.biopsych.2004.11.004
77. Li BM, Mei ZT. Delayed-response deficit induced by local injection of the α2-adrenergic antagonist yohimbine into the dorsolateral prefrontal cortex in young adult monkeys. Behav Neural Biol. (1994) 62:134–9. doi: 10.1016/S0163-1047(05)80034-2
78. Marzo A, Totah NK, Neves RM, Logothetis NK, Eschenko O. Unilateral electrical stimulation of rat locus coeruleus elicits bilateral response of norepinephrine neurons and sustained activation of medial prefrontal cortex. J Neurophysiol. (2014) 111:2570–88. doi: 10.1152/JN.00920.2013
79. Totah NK, Neves RM, Panzeri S, Logothetis NK, Eschenko O. The locus coeruleus is a complex and differentiated neuromodulatory system. Neuron. (2018) 99:1055.e–68.e. doi: 10.1016/J.NEURON.2018.07.037
80. Zaretsky DV, Zaretskaia MV, DiMicco JA, Rusyniak DE. Yohimbine is a 5-HT1A agonist in rats in doses exceeding 1mg/kg. Neurosci Lett. (2015) 606:215–9. doi: 10.1016/j.neulet.2015.09.008
81. Bende MM, Bapat TR, Balsara JJ, Chandorkar AG. Effects of yohimbine on dopamine dependent behaviours in rats and mice. Indian J Physiol Pharmacol. (1990) 34:195–200. doi: 10.1016/0014-2999(95)00246-h
Keywords: dopamine transporter knockout (DAT-KO) rats, ADHD model, norepinephrine (NE), dopamine (DA), α2A-adrenoceptors, Guanfacine (GF), Yohimbine (YOH), spatial working memory
Citation: Kurzina N, Belskaya A, Gromova A, Ignashchenkova A, Gainetdinov RR and Volnova A (2022) Modulation of Spatial Memory Deficit and Hyperactivity in Dopamine Transporter Knockout Rats via α2A-Adrenoceptors. Front. Psychiatry 13:851296. doi: 10.3389/fpsyt.2022.851296
Received: 09 January 2022; Accepted: 28 February 2022;
Published: 25 March 2022.
Edited by:
Anushree N. Karkhanis, Binghamton University, United StatesReviewed by:
Sara Raulerson Jones, Wake Forest School of Medicine, United StatesYukiori Goto, Kyoto University, Japan
Copyright © 2022 Kurzina, Belskaya, Gromova, Ignashchenkova, Gainetdinov and Volnova. This is an open-access article distributed under the terms of the Creative Commons Attribution License (CC BY). The use, distribution or reproduction in other forums is permitted, provided the original author(s) and the copyright owner(s) are credited and that the original publication in this journal is cited, in accordance with accepted academic practice. No use, distribution or reproduction is permitted which does not comply with these terms.
*Correspondence: Anna Volnova, YS52b2xub3ZhQHNwYnUucnU=