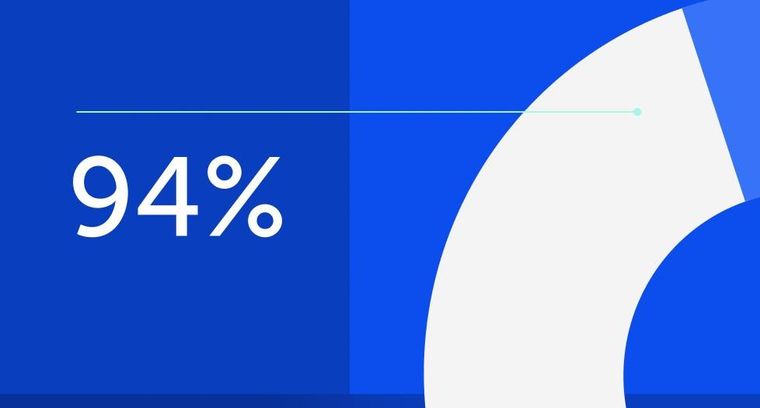
94% of researchers rate our articles as excellent or good
Learn more about the work of our research integrity team to safeguard the quality of each article we publish.
Find out more
SYSTEMATIC REVIEW article
Front. Psychiatry, 10 September 2021
Sec. Psychopathology
Volume 12 - 2021 | https://doi.org/10.3389/fpsyt.2021.724606
This article is part of the Research TopicCan Psychedelic Therapies open a New Frontier in Mental Healthcare (Or Will the Bubble Burst?)View all 22 articles
Clinical studies suggest the therapeutic potential of psychedelics, including ayahuasca, DMT, psilocybin, and LSD, in stress-related disorders. These substances induce cognitive, antidepressant, anxiolytic, and antiaddictive effects suggested to arise from biological changes similar to conventional antidepressants or the rapid-acting substance ketamine. The proposed route is by inducing brain neuroplasticity. This review attempts to summarize the evidence that psychedelics induce neuroplasticity by focusing on psychedelics' cellular and molecular neuroplasticity effects after single and repeated administration. When behavioral parameters are encountered in the selected studies, the biological pathways will be linked to the behavioral effects. Additionally, knowledge gaps in the underlying biology of clinical outcomes of psychedelics are highlighted. The literature searched yielded 344 results. Title and abstract screening reduced the sample to 35; eight were included from other sources, and full-text screening resulted in the final selection of 16 preclinical and four clinical studies. Studies (n = 20) show that a single administration of a psychedelic produces rapid changes in plasticity mechanisms on a molecular, neuronal, synaptic, and dendritic level. The expression of plasticity-related genes and proteins, including Brain-Derived Neurotrophic Factor (BDNF), is changed after a single administration of psychedelics, resulting in changed neuroplasticity. The latter included more dendritic complexity, which outlasted the acute effects of the psychedelic. Repeated administration of a psychedelic directly stimulated neurogenesis and increased BDNF mRNA levels up to a month after treatment. Findings from the current review demonstrate that psychedelics induce molecular and cellular adaptations related to neuroplasticity and suggest those run parallel to the clinical effects of psychedelics, potentially underlying them. Future (pre)clinical research might focus on deciphering the specific cellular mechanism activated by different psychedelics and related to long-term clinical and biological effects to increase our understanding of the therapeutic potential of these compounds.
Classic serotonergic hallucinogens (psychedelics) are a class of psychoactive compounds that produce mind-altering effects through agonism of the serotonergic receptors (5-HT), especially the 5-HT2A receptor (1). Psilocybin, lysergic acid diethylamide (LSD), N, N-dimethyltryptamine (DMT), and the DMT-containing brew ayahuasca are prototypical examples of recreationally used psychedelics that have been shown to influence humans' physiological, cognitive, and emotional state, including mood changes and increased conscious processing of emotions (2). Psychedelics are considered physiologically safe as they do not provoke physical toxicity (3–5). Effects depend on the dose, type of substance, route of administration, body weight, tolerance, age, species, and metabolism, where high doses frequently intensify subjective effects compared to lower doses (5–7). Other significant predictors of psychedelic effects are the mental state (set) and environment (setting), mood, and personality (8, 9).
When looking closer, these psychedelics differ slightly in their pharmacologic characteristics. Psilocybin, found in specific fungi like the Psilocybe Cubensis, is degraded quickly into its active metabolite psilocin after ingestion. Both psilocin and psilocybin exhibit affinity for a range of serotonin receptors (5-HT1A/B/D/E, 2B, 5, 6, 7) with high affinity for the 5-HT2A receptor. Psychological effects start around 10–40 min after ingestion and last for 2–6 h. Linear pharmacokinetics over the 0.3–0.6 mg/kg oral dose range were demonstrated (10). LSD exhibits affinity for 5-HT1A/D, 2A/B/C, and 5-HT6, the dopamine D1 and D2, and α-adrenergic receptors. It displays a shared agonism for 5-HT2A and dopamine D2 receptors (11, 12). The acute physiological effect of a moderate dose of LSD, 75–150 μg p.o. for humans, shows dose-proportional pharmacokinetic effects that last 6–12 h, with the maximum plasma concentration after 1.5 h (13, 14). DMT and its analog 5-MeO-DMT are agonists of 5-HT1A/D, 2A, and 5-HT6 receptors, and 5-HT1A, and 2A/B/C receptors, respectively. Ayahuasca contains next to DMT non-psychedelic β-alkaloids that act as inhibitors of monoamine oxidase A. These compounds allow DMT to pass through the digestive tract and reach the brain unmetabolized. When DMT is administered without the other ayahuasca components, effects arise within minutes after ingestion when inhaled or injected, and last for 15 min (15). After intake of ayahuasca, effects are noticeable 30 min after ingestion, lasting for 3 h, with a peak at 1.5–2 h, corresponding with the peak in DMT plasma concentration, indicating a significant role for DMT in the pharmacology of ayahuasca (16).
Next to their acute effects, studies have demonstrated that psychedelics also induce changes in processes, as mentioned above, beyond their expected blood plasma lifetime. Naturalistic research, for example, has shown enhancement of emotional and cognitive processes after oral self-administration of psilocybin and ayahuasca, in a social setting, lasting up to 4 weeks after the experience, compared to baseline (17, 18). In placebo-controlled experimental studies, LSD, ayahuasca, and psilocybin improved depressive, anxiolytic, and addictive symptoms in patients after one to two doses, measurable 3 weeks to 6 months after administration [for a review, see: (19)]. Given the persisting nature of the psychological effects beyond the presence of the substance in the blood, a biological adaptation is suggested.
Biological adaptations that can underlie psychedelics' persisting behavioral and cognitive changes include changes in neuroplasticity. Neuroplasticity is the brain's ability to change throughout life and consists of changes in cell structure, structural plasticity, and changes in the efficacy of synaptic transmission, also called functional plasticity (20). Structural and functional plasticity are interconnected processes at a molecular and (sub)cellular level (Figure 1).
Figure 1. Mechanisms of neuroplasticity. Schematic representation of the different mechanisms of neuroplasticity at a molecular and (sub)cellular level. Neuroplasticity at a molecular level affects intracellular signaling pathways, gene transcription, and protein synthesis. At a cellular level, neuroplasticity occurs at the subcellular levels of neurogenesis, dendritogenesis, and synaptogenesis. The neuronal changes of neurogenesis and dendritic changes alter the structural characteristics of the neuron: structural plasticity. Synaptogenesis involves functional changes: functional plasticity. LTP, long-term potentiation; LTD, long-term depression.
To fully understand the extent of psychedelics' effects on these levels, more detail is given first about the levels at which neuroplasticity can occur and the signaling substances involved.
At a molecular level, neuroplastic changes occur via signaling pathways, that is, cascades of intracellular proteins transmitting signals from receptors to the DNA (21). Signaling pathways are activated by Ca2+ influx through depolarization or N-methyl-D-aspartate receptor (NMDAR) activation. They include the Ca2+/calmodulin-dependent protein kinase (CaMK2), extracellular regulated kinase 1/2 (ERK1/2) mitogen-activated protein kinase (MAP)/ERK, and the brain-derived neurotrophic factor/tropomyosin receptor kinase B (BDNF/TrkB) pathway. In the nucleus, the cyclic AMP-responsive element-binding protein (CREB) or the nuclear factor kappa B protein complex (NF-kB) is activated, allowing modulation of gene transcription and protein synthesis of plasticity processes. For example, immediate early genes (IEGs), such as c-Fos, Arc, Egr1/2, C/EBP-β, Fosb, Junb, Sgk1, Nr4a1, and Dusp1, are rapidly expressed upon neuronal activity and are essential for synaptic plasticity. These changes in the expression of plasticity-related genes can influence neuroplasticity at the cellular level.
At a cellular level, changes can be structural or functional, and both types have different levels that will be listed here. Structural plasticity includes neuronal plasticity, dendritic plasticity, and synaptic plasticity. Neuronal plasticity consists of neurogenesis, the generation of neurons, and occurs in distinctive phases (22). First, proliferating progenitor cells are generated in the hippocampal subgranular zone and differentiate into dentate granule neurons (23). The proliferating cells that survive the elimination via apoptotic cell death migrate and mature into newborn granule cells and fully integrate into the hippocampal network (23–25). Dendritic plasticity includes changes in the number or the complexity of dendritic spines, where a high number of spines and complex dendritic branches reflect more synaptic strength (26, 27). Of note, the extensive release of γ-aminobutyric acid (GABA) or glutamate causes dendritic spine formation (27).
At the synapse, the strength of synapses is related to learning and memory formation. It can change in two directions, either increasing, known as long-term potentiation (LTP), and decreasing, called long-term depression (LTD). This type of synaptic plasticity alters the neuron's structure and its functional properties. Synaptic plasticity is regulated by various factors, with the protein BDNF as the primary regulator; BDNF is expressed highly throughout the central nervous system, particularly in the hippocampus (28).
BDNF is involved in multiple levels of neuroplasticity like synaptic modulation, adult neurogenesis, and dendritic growth (29–32). Interestingly, studies have shown that BDNF levels are diminished in pathological populations suffering from anxiety, depression, and addiction (33). Preclinical and clinical research has shown that these markers are increased and enhanced by selective serotonin reuptake inhibitors (SSRIs) (34, 35) used to manage the symptoms of these disorders (36). The rapid-acting dissociative agent ketamine, which has shown its efficacy in treating depression, is known to increase BDNF levels (37, 38). It has been suggested that the persisting therapeutic effects of psychedelics are attributable to a similar biological mechanism (39, 40).
Psychedelics' influence on neuroplasticity is investigated in preclinical (in vitro/in vivo) and clinical studies (Figure 2). In vitro studies using rodent cell lines include neuronal stem cells (NSCs) derived from the subgranular zone of the hippocampus of embryonic mice (41). Human cell lines include induced pluripotent stem cells (iPSCs), cerebral organoids that consist of artificially grown cells of synthesized tissues resembling the cortex, and the neuroblastoma cell line SH-SY5Y (42–44). The latter can be used to model neuronal function and differentiation, and neurodegeneration by inducing chemical damage (oxygen deprivation) via in vitro administration of the dopamine analog and neurotoxin 6-hydroxydopamine (6-OHDA) (44). In vivo studies in rodents use electrophysiology, the measurement of gene transcription and protein levels, and receptor knockout models to test the contribution of—for example—a specific receptor in the drug effects. Moreover, a well-established in vivo technique to identify neurogenesis is immunostaining [“immunohistochemistry” (IHC)] with the mitotic marker 5-bromo-2′-deoxyuridine (BrdU) or Ki-67 to determine progenitor cell growth and division (proliferation) (45). These techniques can be used in healthy, intact animals or after surgical damage to the brain using suturing of an internal carotid artery to test neuroplasticity changes after drug administration and brain damage (46).
Figure 2. Evaluation of psychedelics' influence on cellular and molecular neuroplasticity in preclinical and clinical studies. Schematic overview of the current methods to study the neuroplastic effects of psychedelics at a molecular and cellular level. NSCs, Neural stem cells; iPSCs, induced pluripotent stem cells.
In clinical studies, biological samples are collected from healthy volunteers and patients suffering from a psychopathology like treatment-resistant depression (TRD) to determine BDNF levels (47–50). Clinical symptoms are assessed with, for example, the Montgomery-Åsberg Depression Rating Scale (MADRS) to test for depression severity (51).
To summarize, it is hypothesized that neurobiological changes, specifically enhanced neuroplasticity, underlie psychedelics' therapeutic effects. The techniques mentioned above can be used to assess changes in plasticity after the administration of psychedelics compared to baseline, a placebo, or a control group. Understanding the biological pathways of psychedelics' acute and persisting effects is essential to grasp these compounds' full therapeutic potential. Although psychedelics do not have an established therapeutic use in psychiatry yet, promising preliminary findings of their therapeutic potential support further investigation and give insight into psychiatric disorders' biological underpinnings. To address this knowledge gap and answer the question of what effects (serotonergic) psychedelics have on molecular and cellular neuroplasticity, a systematic review was performed focusing exclusively on classical serotonergic psychedelics (including psilocybin, LSD, ayahuasca, DMT, and its closely related analogue 5-methoxy-N,N-dimethyltryptamine, 5-MeO-DMT). The listed substances were chosen because of their shared agonism at 5-HT2A receptors. In line with SSRIs and ketamine, it was hypothesized that psychedelics enhance molecular and cellular neuroplasticity.
According to PRISMA guidelines, a literature search was performed using the database PubMed in October 2020 (52). Two search strings were combined with the Boolean command “AND.” The first string included MeSH terms referring to neuroplasticity: neuronal plasticity, functional neuroplasticity, structural neuroplasticity, spine density, receptor density, axonal arbor, neuritogenesis, synaptogenesis, synapse formation, neurogenesis, BDNF, proliferation, maturation, survival, migration, neuronal migration; the second string included terms to describe the psychedelics that were focal in this review: classical psychedelics, psychedelics, hallucinogens, psilocybin, 4-phosphoryloxy-N,N-dimethyltryptamine, psilocin, 4-hydroxy-N,N-dimethyltryptamine, LSD, lysergic acid diethylamide, DMT, N,N dimethyltryptamine, 5-MeO-DMT, 5-methoxy-N,N dimethyltryptamine.
The literature search targeting the title and abstract gave 344 hits in total. This sample underwent de-duplication (n = 0) and a selection process using the following inclusion criteria: published in a peer-reviewed journal in the English language, including one of the target psychedelics, and assessing neurobiological parameters (e.g., cellular or molecular). This led to a sample of 35 articles, from which 23 were excluded because no molecular or cellular parameters of neuroplasticity were assessed. Additionally, eight articles were identified through other sources (cross-references), eventually resulting in a final dataset of 16 experimental studies in animals and four in humans (Figure 3).
The preclinical and clinical research findings are discussed in two separate sections; the methodological details of reviewed studies are presented in Tables 1, 2. A distinction is made between single and repeated dose administration and between acute, subacute, and long-term effects. Acute effects are measured within 24 h after administration of the psychedelic, subacute effects are measured between 24 h and 1 week after administration, and effects are considered long-term when they are observed after more than 1-week post-treatment.
Table 1. Preclinical studies investigating psychedelics' effects on molecular and (sub)cellular neuroplasticity.
Table 2. Clinical studies investigating cellular and molecular effects of serotonergic psychedelics.
The effects of psychedelics on molecular and cellular neuroplasticity in preclinical studies are presented from a molecular to a subcellular level. They are separated by in vitro and in vivo studies. Evidence from preclinical studies (n = 15 out of 16) suggests that psychedelics induce structural and functional synaptic modulations at a molecular and cellular level (Table 1).
Evidence from in vitro studies (n = 5) suggests that psychedelics stimulate molecular and cellular neuroplasticity (41–44, 53). The acute effect of a single dose of (5-MeO-)DMT on neuroplasticity was investigated by three in vitro studies (42, 43, 53). Administration of DMT (10 μM) and LSD (90 μM) to cortical rat neurons (n = 39–41) for 24 h resulted in increased dendritic complexity, expressed by an increased number and total length of dendrites compared to vehicle-treated controls (53). The dendritic spine-promoting properties were found to be 5-HT2AR-mediated, as they were blocked by ketanserin. LSD was the most potent psychedelic regarding neuritogenesis compared to the tested psychedelics (53). In human cerebral organoids (n = 4–5), 5-MeO-DMT (13 μM) administered for 24 h directly resulted in the stimulated synthesis of proteins involved in plasticity-related intracellular signaling pathways such as NMDAR, alpha-amino-3-hydroxy-methyl-5-4-isoxazolpropionic receptor (AMPAR), and Eprhin B2 (43). These findings show acute changes in molecular processes related to structural and functional neuroplasticity induced by 5-MeO-DMT.
Besides the stimulation of neuroplasticity in “optimal” (healthy) conditions, evidence shows that a single dose of DMT (1, 10, 50, 200 μM) exhibits acute neuroprotective properties in cultured human iPSCs cells that were differentiated into cortical neurons (n = 3) and exposed to severe neuronal stress (42). DMT stimulated neurogenesis by increasing the neuronal survival rate from 19% (untreated cells) to 31% (10 μM) and 64% (50 μM), 6 h after exposure to severe hypoxic stress. Moreover, selective silencing of the plasticity-promoting intracellular sigma-1 receptor (S1R) (64) decreased the survival of iPSCs by 93%, indicating that the S1R mediated the DMT-induced survival (42). Together, these findings show that a single dose of 5-MeO-DMT, DMT, and LSD in vitro directly stimulate dendritic and neuronal plasticity that resulted from intracellular changes. Subacute and long-term effects of a single dose of a psychedelic on molecular and cellular plasticity were not investigated in vitro.
Acute effects of repeated administration of DMT and ayahuasca were investigated in vitro, and suggest stimulation of neuroplasticity at a (sub)cellular level (41, 44). Repeated daily administration of DMT (1 μM, 7 days) to neural stem cells (n = 6) from adult mice's subgranular zone of the dentate gyrus showed stimulation of proliferation, and differentiation to neurons, astrocytes, and oligodendrocytes (41). In cultured human neuroblastoma (SH-SY5Y) cells (n = 3) exposed to neurotoxic stress using 6-OHDA, cell viability was differentially changed after treatment with ayahuasca (1, 1.5, 2.5, or 10 μg/mL) every 24 h, and incubation for 48 and 72 h (44). Low doses of ayahuasca (1.5 and 2.5 μg/mL) increased cell viability (±70%) compared to non-stressed controls after 48 h, whereas cell viability was decreased after a high dose (10.5μg/mL) at 72 h post-administration (44). These findings suggest acute stimulative effects of neural plasticity by DMT, dose-dependent neuroprotective properties, and possibly proliferative effects of ayahuasca in stressed cell cultures at low doses. Together, the findings from in vitro studies support plasticity-promoting characteristics of DMT, ayahuasca, and LSD when administered once or multiple times. Subacute and long-term effects of repeated administration of psychedelics on molecular and cellular plasticity were not investigated in vitro.
Evidence from in vivo studies (n = 13 out of 16) also indicates neuroplastic effects of psychedelics. Findings from in vivo studies investigating acute effects of a single dose of 5-MeO-DMT, DMT, psilocybin, and LSD (37%) suggest altered cellular plasticity of structural and functional nature (54–60). A single dose of 5-MeO-DMT (100 μg, i.c.v.) stimulated neurogenesis and spinogenesis in mice (n = 5), 12 h post-treatment (54). The proliferation of neuronal progenitor cells, and the survival of newborn granule cells were increased in the ventral hippocampus, a brain area involved in emotion and stress regulation (65), compared to vehicle-treated controls (54). 5-MeO-DMT in this study also stimulated spinogenesis of granule cells in the hippocampus. The dendritic spines grew more quickly toward the complex morphology of a mature neuron. Electrophysiological analysis showed a lower action potential threshold of synapses in the hippocampus, indicating that the synapses are more prone to receive synaptic input and suggesting stimulated functional plasticity (54). A single dose of psilocybin (0.5, 1, 2, 4, 8, 14, 20 mg/kg, i.p.) administered to rats (n = 10) altered the expression of plasticity-promoting genes in the prefrontal cortex (PFC) and hippocampus, 90 min after treatment (55). Psilocybin stimulated the expression of IEGs in the PFC, and induced stimulating and inhibiting effects in the hippocampus (55). Because more target genes were regulated in the PFC, the authors suggested a stronger stimulation in the PFC over the hippocampus by psilocybin (55). Moreover, in both areas, most IEGs were affected in a dose-dependent manner, with higher doses inducing more stimulation of gene expression (55). A single dose of LSD (0.20, 0.24, 0.5, 1.0 mg/kg i.p.) stimulated the mRNA expression of plasticity-promoting genes in the cortex of rats and mice, within 1–2 h after administration and in a time- and dose-dependent manner (56–59). Conversely, in a different study with rats (n = 5) that were administered LSD (0.5 mg/kg, i.p.), no changes were found in hippocampal proliferation 2.5 h after administration compared to vehicle-treated controls indicated by BrdU+ cells (60). Taken together, it is shown that a single treatment with psychedelics acutely regulates molecular processes of plasticity-promoting gene expression, neuroplasticity at the cellular level of neurogenesis, and dendritic plasticity. Psychedelics' subacute effects of a single dose of DMT (10 mg/kg, i.p.) were investigated in rat cortical pyramidal neurons (n = 11–37 neurons from three animals) (53). Spontaneous excitatory postsynaptic currents (EPSCs) and dendritic spine density were stimulated 24 h after administration, indicating stimulated structural and functional plasticity (53). Psychedelics' long-term effects of a single dose were not assessed on molecular and cellular plasticity in vivo.
Evidence from in vivo studies investigating subacute and long-term, but not acute, effects of repeated administration of psychedelics (n = 3) show that DMT and LSD stimulate neurogenesis (41, 60, 61). The subacute effects of repeated (4 consecutive days) or prolonged (21 days, every other day) DMT (2 mg/kg, i.p.) treatment were assessed on neurogenesis in mice, 24 h after the administration stopped (41). Short-term treatment (n = 5) resulted in BrdU+ cells in the hippocampus, indicating enhanced proliferation and migration of neuronal precursors, and long-term (n = 12) increased neuronal survival in the subgranular zone (41). Repeated LSD (0.5 mg/kg, i.p.) administration, daily for seven consecutive days to rats (n = 3–5) did not result in changes in BrdU+ cells compared to vehicle-treated controls, 26 h after administration, indicating that repeated LSD administration did not affect neurogenesis (60). Together, these findings show that repeated administration of DMT, but not LSD, subacutely resulted in stimulated hippocampal neurogenesis. The long-term effects of repeated doses of psychedelics were examined for LSD, at a molecular level (61). Chronic treatment with LSD (0.16 mg/kg i.p., every other day for 90 days) increased the expression of plasticity-related genes in the mPFC of rats (n = 10), 4 weeks after treatment cessation (61). Among the upregulated genes were Bdnf, Egr2, Nor-1, Nr2a, and Npy. Nr2a encodes for NMDA receptor subunits, and the NPY protein stimulates neurogenesis and has anxiolytic effects (66, 67). These findings show that repeated LSD administration stimulates the expression of plasticity-related genes 4 weeks after treatment. Taken together, the findings from in vivo studies support plasticity-promoting properties of psychedelics at a molecular and cellular level after a single or multiple dose administration.
Four studies (out of 16) investigated the relationship between biological effects of psychedelics (5-MeO-DMT, DMT, ayahuasca, LSD, and psilocybin), and behavioral changes (41, 46, 62, 63). While the acute effects of a single dose of a psychedelic on biological markers and behavior were not assessed, the long-term effects of a single dose of psilocybin were investigated (62). Mice (n = 6) that were administered a single, low dose of psilocybin (0.1 mg/kg, i.p) showed a non-significant increase in proliferation of hippocampal progenitor cells 14 days later. In contrast, higher doses (1.0 mg/kg, i.p.) led to a significant decrease in proliferation, 14 days post-treatment (62). To investigate if the hippocampus mediates behavioral changes 48 h after treatment with psilocybin mice (n = 9–10) underwent fear conditioning (62, 68). Psilocybin-treated mice exhibited increased extinction compared to saline-treated controls for all doses, indicating a quicker learning response to fear (62). At a biological level, psilocybin induced a dose-dependent effect on neurogenesis, with a low dose increasing, and a high dose decreasing neurogenesis (62). Behavioral effects of psilocybin demonstrated a dose-independent stimulation of fear extinction, suggesting that alterations to hippocampal neurogenesis are not related to fear extinction after psilocybin administration.
The relationship between psychedelics' effect on biological markers and behavior was also investigated after repeated psychedelic administration. Three studies examined the immediate and long-term effects of repeated administration of ayahuasca, LSD, and DMT (41, 46, 63). Ayahuasca was administered daily to rats (n = 7–10) for 28 days, in a dose that was 0.5, 1, or 2 times the human, ritualistic oral dose, which is 0.26 mg/kg DMT p.o., 2.58 mg/kg harmine, 0.171 mg/kg harmaline, and 0.33 mg/kg tetrahydroharmine. These administration patterns did not change hippocampal BDNF protein levels and resulted in increased anxiety behavior in male rats treated with the middle dose, 1 h after the last treatment (63). After 3 h, female rats treated with the high dose exhibited increased hippocampal BDNF protein levels but did not show changed anxiety behavior (63). These findings indicate direct sex- and dose-specific effects of repeated ayahuasca on molecular neuroplasticity and anxiety behavior.
While subacute effects of repeated doses of psychedelics were not investigated, long-term effects of chronic DMT (2 mg/kg, i.p.) administration (21 days, every other day) to mice (n = 10–12) were tested. Findings showed enhanced neurogenesis that corresponded with improved spatial learning and memory tasks for 10 days post-treatment (41). In a study in rats (n = 10) that had received surgical brain damage and were treated with DMT (1 mg/kg, i.p., followed by a maintenance dose of 2 mg/kg/h for 24 h) afterwards, DMT led to stimulated cortical (mRNA) and plasma BDNF (protein) levels 1 h after DMT treatment cessation (46). On a behavioral level, rats (n = 8) showed an increased motor function that lasted up to 30 days after treatment (46). Moreover, animals (n = 10) treated with DMT combined with an S1R antagonist (BD-1,063) exhibited a higher lesion volume than DMT-treated animals 24 h after brain damage was inflicted, suggesting that the effects of DMT are S1R-mediated (46). These findings show that DMT has an immediate stimulative impact on molecular plasticity processes and promotes recovery behavior up to a month after induced brain damage. Taken together, the findings from behavior studies support the plasticity-promoting characteristics of ayahuasca and DMT at a molecular and cellular level, accompanied by plasticity-related changes in behavior.
Evidence from four randomized, placebo-controlled studies investigating the acute and subacute, but not long-term effects, of a single dose of psychedelics on a molecular level show that a single treatment with ayahuasca or LSD can, but does not always, increase circulating BDNF in healthy volunteers and TRD patients (47–50) (Table 2). Clinical research investigating psychedelics' effect on cellular neuroplasticity is lacking.
A single, low dose of LSD (5, 10, and 20 μg) administered to healthy volunteers (n = 24) resulted in increased serum BDNF levels compared to placebo, 6 h after treatment (50). Blood samples taken every 2 h, until 6 h after administration, showed elevated plasma BDNF levels at 4 h after administration for the 5 μg, and at 6 h for the 20 μg dose. BDNF levels were highest at 4 h after treatment for the 5 μg dose and at 6 h after treatment for the 10 μg and 20 μg doses, suggesting dose-specific stimulation of BDNF (50). In a cross-over study in healthy participants (n = 18) that were treated with single doses of LSD (25, 50, 100, and 200 μg) over six sessions, with 10 days in-between administrations, findings showed that blood plasma BDNF levels were dose-dependently elevated compared with placebo (49). Six hours after administering 200 μg, participants reported ego dissolution and anxiety, in parallel with increased plasma BDNF (49). The subjective response was partially prevented by administering a 5-HT2A/C receptor antagonist (ketanserin) 1 h before LSD treatment, as demonstrated by a “25 μg-dose response” after administration of 200 μg of LSD plus ketanserin (49).
The subacute neuroplastic properties of a single, oral dose of ayahuasca (1 mL/kg, p.o., ayahuasca composition not reported) were assessed in patients suffering from TRD (n = 28), and in healthy controls (n = 45) naïve to ayahuasca, in a controlled environment (47). Blood serum BDNF levels were increased at 48 h after administration compared to baseline in both groups, and they correlated negatively with the MADRS scores in TRD patients treated with ayahuasca (47). These findings suggest that lower depressive symptomatology was associated with higher BDNF levels. It was suggested that patients with the most persistent depression benefited the most from ayahuasca treatment (47). Since the specific composition of the ayahuasca brew and thus the DMT dose was not reported in the study, it is difficult to compare the outcomes of this experiment with similar studies. Conversely, in a follow-up study, a single oral dose of ayahuasca (1 mL/kg, p.o., 0.36 mg/mL DMT, 1.86 mg/mL harmine, 0.24 mg/mL harmaline) administered to TRD patients (n = 28) and healthy controls (n = 45) in a controlled setting did not affect serum BDNF levels at 48 h after administration (48).
The limited number of studies investigating molecular biological and behavioral correlates of psychedelics' effects shows that psychedelics acutely and subacutely stimulate molecular plasticity and decrease depressive symptoms in healthy and TRD patients, with effects lasting up to 48 h after administration. The acute biological and behavioral effects of repeated administration on molecular and cellular plasticity were not investigated in a clinical setting.
To understand the acute, subacute (24 h−1 week post-treatment), and longer-term effects of (serotonergic) psychedelics on molecular and cellular neuroplasticity, preclinical and clinical studies were evaluated. Evidence from preclinical studies shows that psychedelics acutely stimulate structural neuroplasticity processes at a molecular and (sub)cellular level after a single dose. Subacute effects of a single dose of a psychedelic on molecular and cellular neuroplasticity have not yet been investigated, and one study investigating the long-term effects of psilocybin showed decreased neurogenesis weeks after a single dose. Repeated administration of psychedelics is shown to stimulate neurogenesis acutely and molecular plasticity, subacutely. Moreover, a limited number of (pre)clinical studies that investigated the relationship between biological and behavioral adaptations showed that the stimulation of molecular and neuronal was accompanied by increased learning behavior. Under stressful conditions, neuronal plasticity and molecular plasticity processes were found to be stimulated in rodents, and ayahuasca-induced increases in plasma BDNF levels correlated with diminished depressive symptoms in clinical populations, subacutely. Similarly, findings from clinical studies showed that blood BDNF levels were directly elevated in healthy participants that were treated with a single dose of LSD. Long-term and repeated administration effects on molecular and cellular plasticity were not investigated. Overall, the limited evidence that is presented is consistent with our hypothesis that psychedelics stimulate molecular and cellular structural neuroplasticity.
Of note, the antidepressant effects of ayahuasca may also be produced by its non-psychedelic β-alkaloids harmine, tetrahydroharmine, and harmaline present in the ayahuasca brew (69). Findings from in vitro and in vivo studies show that these compounds stimulate neurogenesis, BDNF, and have antidepressant effects (70–73). Neuroplastic changes induced by ayahuasca may result from DMT, β-alkaloids, or an interaction between these compounds, something that should be taken into consideration when interpreting findings from biological studies using ayahuasca.
Four main findings stand out from our review. The first concerns dose differences between preclinical and clinical studies and their translation from animal to human. Clinically, LSD doses varied between 5 and 200 μg. To compare preclinical and clinical doses, the conversion formula for the animal dose = human dose × (37/3) was used (74). For example, a high dose of 200 μg p.o. LSD for a human with an average weight of 70 kg equals 0.00285 mg/kg, and converts to a dose of 0.021 mg/kg LSD for rats, and 0.041 mg/kg LSD for mice (75). Using the molarity formula (M = m/MW * 1/V where m = mass in grams, MW = molecular weight of LSD and V = volume of the diluent in liters), assuming a mouse weight of 25 g and making 0.025 L solution, an approximation of the in vitro LSD dosage would be 0.126 μM based on a human dose of 200 μg per 70 kg. This is remarkably lower than the 10 μM LSD used by Ly et al. (53), and presumably even lower for the other, lower, clinical doses. Conversion from in vitro to in vivo doses and vice versa is more complicated than these calculations. Animal doses of LSD varied between 0.16 and 1.0 mg/kg i.p. in rats and mice, indicating that LSD doses were higher in animal studies than in clinical studies. This difference is even larger due to the first-pass metabolism reducing the systemic exposure of LSD after oral administration used in clinical studies. With intraperitoneal administration in rodents, this degradation is avoided. These findings suggest that the highest doses given in clinical studies resemble the lowest doses of LSD in preclinical studies, highlighting an important factor that should be considered in the translation of preclinical findings to humans. Further research into the neuropharmacokinetics of psychedelics could bridge this gap between optimal preclinical and clinical doses.
The second significant finding concerns sex-differences in response to psychedelics, which were shown in a preclinical study where male, but not female rats showed increased anxiety behavior directly after prolonged ayahuasca administration (63). This could be related to sex-specific changes in neuroplasticity (76). The female sex hormone estrogen exhibits antidepressant effects through stimulation of BDNF and synaptic plasticity, in a manner that is distinct for males and females (77, 78). In that line, female rats showed greater sensitivity to the antidepressant effects of ketamine than male rats, and effects were abolished in rats whose ovaries had been removed and restored when estrogen and progesterone were supplemented (79). The antidepressive effects of ketamine and psychedelics are both suggested to result from changes in neuroplasticity, and these findings indicate a potential role for gonadal hormones in the sex-specific response to these substances. Neurobiological research in animal models is biased toward males (80). These facts highlight the importance of investigating both sexes in preclinical research to further elucidate sex differences in psychopathologies and improve translation to clinical populations.
The third finding concerns the measurement of BDNF in clinical studies. All clinical studies reported peripheral BDNF levels, an indirect measure of BDNF levels in the brain. It would be more precise to examine cerebrospinal fluid (CSF) BDNF levels as this directly reflects brain activity (81). While the collection of CSF is invasive, only a limited number of studies have investigated BDNF CSF levels; two studies found a positive correlation between CSF and plasma BDNF levels in first-episode psychotic and depressed patients (82, 83). Furthermore, while previously it was not clear whether clinical response was related with plasma BDNF levels, evidence suggests that there is a positive relation with clinical improvement being linked with improved neuroplasticity (33). Nonetheless, further research is recommended to investigate the effect of psychedelics on CSF BDNF levels in clinical populations and its relation with BDNF plasma levels.
The fourth finding of this review concerns the sample size of some in vivo studies, which was low. A sample size of six animals per group is considered an adequate sample size in animal research by many researchers but reduces the statistical power (84). This is a well-known problem in (neuro)biological research (85). Researchers are to justify the number of animals used in their experiments, which should be designed to minimalize the number of animals used. This could explain the low sample size in in vivo studies reviewed here, and is essential to consider because it reduces the statistical power and limiting the reliability of conclusions.
The observed psychedelics-induced changes in neuroplasticity are suggested to result from the neurobiological pathways activated by 5-HT2AR upon activation by psychedelics, affecting the serotonergic and glutamatergic system (Figure 4). Psychedelics primarily act on 5-HT2ARs expressed on glutamatergic pyramidal cells in cortical and deep cortical layers (V and VI) (86, 87). Via activation of 5-HT2AR, psychedelics activate intracellular signaling pathways such as PLC, PLA, and Src (88–90). Activation of Src is suggested to be essential for psychedelics' hallucinogenic effects, as its inhibition prevented hallucinogenic effects of LSD (90). Activation of these pathways' intracellular signaling leads to Ca2+ and glutamate release that stimulates synaptic plasticity. Increased glutamate in the cortex release can further stimulate synaptic plasticity via AMPAR on pyramidal neurons in cortical layer V and subsequent transportation (trafficking) of AMPAR to the postsynaptic cell membrane (43). This increases AMPAR density, resulting in more extracellular glutamate and BDNF release in the cortex (86, 91). The potential of classic psychedelics to alter glutamate in the human cortex, albeit in a region-dependent manner, has been demonstrated (92). Indirectly, psychedelics influence plasticity via the expression of BDNF and other plasticity-related genes and proteins, including IEGs (91, 93). Cortical bdnf mRNA was upregulated by LSD and ayahuasca (61, 63). IEGs are implicated in synaptic plasticity and synaptogenesis and many IEGs encode for proteins involved in specific signaling cascades (94). For instance, Arc is localized at dendrites and involved in cytoskeletal rearrangements (95), Egr2 has coupled activity with the NMDAR (96), Sgk promotes cell survival (97) and the Neuron-derived orphan receptor 1 (Nor1; NR4A3) is important for LTP (98).
Figure 4. Proposed mechanism of action of cellular and molecular effects of serotonergic psychedelics. Schematic and simplified overview of the intracellular transduction cascades in the PFC induced by 5-HT2AR activation by psychedelics. When activated by psychedelics, the 5-HT2AR can activate multiple signaling cascades by coupling to G-proteins. These can activate PLC or PLA2 signaling, both leading to modulations in synaptic plasticity. In addition, glutamate and TrkB activation stimulate AMPAR trafficking. BDNF also activates mTOR through TrkB, resulting in stimulated synaptic plasticity. 5-HT, Serotonin; 5-HT2AR, Serotonergic 2A receptor; Akt, Protein kinase B; AMPAR, α-amino-3-hydroxy-5-methyl-4-isozalopropionic acid receptor; BDNF, Brain-derived neurotropic factor; C/EBP-β, CCAAT enhancer binding protein; CAMK2, Ca2+/calmodulin-dependent protein kinase; CREB, cyclic AMP-responsive element-binding protein; DAG, Diaglycerol; ERK 1/2, extracellular regulated kinase 1/2; Iκβ-α, inhibitor of nuclear factor kappa B alpha; IP3, Inositol triphosphate; MAPK, mitogen-activated protein kinase; mTOR, Mammalian target of rapamycin; NFκβ-α, nuclear factor kappa B protein complex alpha; NMDAR, N-methyl-D-asparate receptor; PI3K, Phosphatidylinositol-4,5-bisphosphate 3-kinase; PKA, Protein Kinase A; PLA2, Phospholipase A2; PLC, Phospholipase C; TrkB, Tropomyosin receptor kinase B.
Alternatively, enhanced neuroplasticity can be attributed to differences in receptor affinity, given that psychedelics are not pure 5-HT2AR agonists, which could explain the different effects on neuroplasticity between psychedelics. DMT exhibits, besides the 5-HT2AR, also high affinity for the S1R, which is highly expressed in the hippocampus and is a stimulator of synaptic plasticity (99–101). Activation of S1R by DMT has been suggested to stimulate synaptic plasticity in addition to 5-HT2AR-induced modulation and is likely responsible for ayahuasca's antidepressant effects (64, 102). Moreover, LSD was shown to stimulate S1R indirectly via activation of the neurosteroid dehydroepiandrosterone (DHEA), which stimulated synaptic plasticity and neurogenesis (103, 104). In like manner, activation of S1R by SSRIs enhances BDNF expression (105, 106). These findings support the hypothesis that the psychedelics'-induced stimulation of neuroplasticity underlies a mechanism similar to SSRIs.
The dissociative ketamine is another substance whose antidepressant effects are suggested to result from enhanced BDNF and synaptic plasticity (107). As an NMDAR antagonist, ketamine blocks postsynaptic NMDAR located on glutamatergic neurons in the cortex. It deactivates the eukaryotic Elongation Factor-2 (eEF2) kinase, which subsequently alleviates its block on BDNF translation, resulting in heightened BDNF levels (108). In addition, ketamine is hypothesized to block NMDAR on GABA interneurons, releasing the inhibition of glutamate release (109). This activates AMPAR on glutamatergic cells and subsequently increases BDNF and glutamate in the cortex (110). Ketamine and psychedelics activate cortical AMPAR and subsequently stimulate BDNF and synaptic efficacy (111). This could explain the (rapid) antidepressant and anxiolytic effect of psychedelics and ketamine, and provides insight into the biological underpinnings of these substances and their therapeutic potential.
This systematic review is the first to explain psychedelics' rapid antidepressant and cognitive effects, by investigating molecular and cellular changes related to neuroplasticity. The data reviewed here contributes to a clearer understanding of the underlying biological mechanisms of serotonergic psychedelics and emphasizes the need for scientific research in this field, because psychedelics are not only beneficial in populations suffering from psychopathologies, but also for those without, enhancing social and cognitive skills such as empathy and creativity, but also general well-being (18, 112). Further research is essential to establish the specific (intra)cellular mechanism activated by different psychedelics, their long-term effects, and their relation with altered behavior. The current findings support research exploring psychedelics' potential in the treatment of psychopathologies.
The original contributions presented in the study are included in the article/supplementary material, further inquiries can be directed to the corresponding author.
CV performed the research and designed the figures. CV, NM, and KK wrote the manuscript. All authors contributed to the article and approved the submitted version.
The authors declare that the research was conducted in the absence of any commercial or financial relationships that could be construed as a potential conflict of interest.
All claims expressed in this article are solely those of the authors and do not necessarily represent those of their affiliated organizations, or those of the publisher, the editors and the reviewers. Any product that may be evaluated in this article, or claim that may be made by its manufacturer, is not guaranteed or endorsed by the publisher.
Figures 1–4 were created with BioRender.com.
5-HT, Serotonin; 5-HT2AR, Serotonergic 2A receptor; 5-HT2CR, Serotonergic 2C receptor; 5-MeO-DMT, 5-methoxy-N,N-dimethyltryptamine; 6-OHDA, 6-hydroxydopamine; AC1/8, Ca2+-stimulated type 1 and type 8 adenylyl cyclases; Akt, Protein kinase B; AMPAR, α-amino-3-hydroxy-5-methyl-4-isozalopropionic acid receptor; AP, Action potential; Arc, Activity-regulated cytoskeleton-associated protein; Aya, Ayahuasca; BD-1063, 1-[2-(3,4-dichlorophenyl)ethyl]-4-methylpiperazine; BDNF, Brain-derived neurotropic factor; BrdU, Bromodeoxyuridine; C/EBP-β, CCAAT enhancer binding protein; CaM, Calmodulin; CAMK2, Ca2+/calmodulin-dependent protein kinase; Clk1, CDC2-like kinase isoforms 1; Arrdc2, Arrestin domain containing 2 or induced by lysergic acid diethylamide 1 (ilad1); CREB, Cyclic AMP-responsive element-binding protein; CSF, Cerebrospinal fluid; DAG, Diaglycerol; DCX, Doublecortin; DG, Dentate gyrus; DHEA, dehydroepiandrosterone; DMT, N,N-dimethyltryptamine; DOI, 2,5-Dimethoxy-4-iodoamphetamine; Drd1, Dopamine receptor D1; Drd2, Dopamine receptor D2; Dups1, dual specificity phosphatases; eEF2, Eukaryotic elongation factor-2; Egr1, Early growth response protein 1; Egr2 or Krox20, Early Growth Response Protein 2; ELISA, Enzyme-linked immunosorbent assay; EPAC1, Exchange factor directly activated by cAMP 1; EPM, Elevated Plus Maze; EPSCs, Excitatory postsynaptic currents; ERK 1/2, Extracellular regulated kinase 1/2; F, Female; FDA, Food and Drug Administration; FACS, Fluorescence-activated cell sorting; GABA, Gamma aminobutyric acid; GPCR, G-protein coupled receptor; HC, Hippocampus; i. c.v., Intracerebroventricular; i.p., Intraperitoneal; i.v., Intravenous; ICC, Immunocytochemistry; IEGs, Immediate Early Genes; IHC, Immuohistochemistry; Iκβ-α, Inhibitor of nuclear factor kappa B alpha; IL-6, Interleukin 6; Ilad-1, Induced by lysergic acid diethylamide 1 or named Arrestin domain containing 2 (arrdc2); IP3, Inositol triphosphate; iPSCs, Induced pluripotent stem cells; KO, Knocked out; LSD, Lysergic acid diethylamide; LTD, Long-term depression; LTP, Long-term potentiation; M, Male; MADRS, Montgomery-Åsberg Depression Rating Scale; MAP, Mitogen-activated protein kinase; MCAO, Middle cerebral occlusion; MDD, Major Depression Disorder; mGluR5, Metabotropic glutamate receptor 5; MKP1, Map Kinase Protein 1; mPFC, Medial prefrontal cortex; MRI, Magnetic Resonance Imaging; mRNA, Messenger ribonucleic acid; mTOR, Mammalian target of rapamycin; MTT, 3-(4,5-dimethylthiazol-2-yl)-2,5-diphenyl-2H-tetrazolium bromide; MWM, Morris Water Maze; NFκβ-α, Nuclear factor kappa B protein complex alpha; NMDAR, N-methyl-D-asparate receptor; Nor1, Neuron-derived orphan receptor 1; Npy, Neuropeptide Y; Nr4a1, Nuclear receptor 4A1; NSCs, Neuronal stem cells; OR, Object recognition task; p.o., Oral administration; P11, S100 calcium-binding protein A10; PCR, Polymerase chain reaction; PFC, Prefrontal cortex; PI3K, Phosphatidylinositol-4,5-bisphosphate 3-kinase; PKA, Protein Kinase A; PLA2, Phospholipase A2; PLC, Phospholipase C; PRISMA, Preferred Reporting Items for Systematic Reviews and Meta-Analyses; Psd95, Postsynaptic density 95; Psi, Psilocybin; Ptgs2, prostaglandin-endoperoxide synthase 2; RNA, Ribonucleic acid; Nor-1, Neuron-derived orphan receptor 1; S1R, Sigma-1-receptor; Sgk1, Serum glucocorticoid kinase 1; SGZ, Subgranular zone; siRNA, Small interfering RNA; SSRIs, Selective-serotonin reuptake inhibitors; TRD, Treatment-resistant depression; TrkB, Tropomyosin receptor kinase B.
2. Vollenweider FX, Preller KH. Psychedelic drugs: neurobiology and potential for treatment of psychiatric disorders. Nat Rev Neurosci. (2020) 21:611–24. doi: 10.1038/s41583-020-0367-2
3. Bogenschutz MP, Johnson MW. Classic hallucinogens in the treatment of addictions. Progress Neuro-Psychopharmacol Biol Psychiatry. (2016) 64:250–8. doi: 10.1016/j.pnpbp.2015.03.002
4. Halpern JH, Pope HG. Do hallucinogens cause residual neuropsychological toxicity? Drug Alcohol Depend. (1999) 53:247–56. doi: 10.1016/S0376-8716(98)00129-X
5. Studerus E, Kometer M, Hasler F, Vollenweider FX. Acute, subacute and long-term subjective effects of psilocybin in healthy humans: a pooled analysis of experimental studies. J Psychopharmacol. (2011) 25:1434–52. doi: 10.1177/0269881110382466
6. Griffiths RR, Johnson MW, Richards WA, Richards BD, McCann U, Jesse R. Psilocybin occasioned mystical-type experiences: immediate and persisting dose-related effects. Psychopharmacology. (2011) 218:649–65. doi: 10.1007/s00213-011-2358-5
7. Liechti ME. Modern clinical research on LSD. Neuropsychopharmacology. (2017) 42:2114–27. doi: 10.1038/npp.2017.86
8. Carhart-Harris RL, Nutt DJ. Serotonin and brain function: a tale of two receptors. J Psychopharmacol. (2017) 31:1091–120. doi: 10.1177/0269881117725915
9. Swanson LR. Unifying theories of psychedelic drug effects. Front Pharmacol. (2018) 9:172. doi: 10.3389/fphar.2018.00172
10. Brown RT, Nicholas CR, Cozzi NV, Gassman MC, Cooper KM, Muller D, et al. Pharmacokinetics of escalating doses of oral psilocybin in healthy adults. Clin Pharmacokinet. (2017) 56:1543–54. doi: 10.1007/s40262-017-0540-6
11. Marona-Lewicka D, Thisted RA, Nichols DE. Distinct temporal phases in the behavioral pharmacology of LSD: dopamine D2receptor-mediated effects in the rat and implications for psychosis. Psychopharmacology. (2005) 180:427–35. doi: 10.1007/s00213-005-2183-9
12. Mucke HAM. From psychiatry to flower power and back again: the amazing story of lysergic acid diethylamide. Assay Drug Dev Technol. (2016) 14:276–81. doi: 10.1089/adt.2016.747
13. Passie T, Halpern JH, Stichtenoth DO, Emrich HM, Hintzen A. The pharmacology of lysergic acid diethylamide: a review. CNS Neurosci Ther. (2008) 14:295–314. doi: 10.1111/j.1755-5949.2008.00059.x
14. Dolder PC, Schmid Y, Steuer AE, Kraemer T, Rentsch KM, Hammann F, et al. Pharmacokinetics and pharmacodynamics of lysergic acid diethylamide in healthy subjects. Clin Pharmacokinet. (2017) 56:1219–30. doi: 10.1007/s40262-017-0513-9
15. Domínguez-Clavé E, Soler J, Elices M, Pascual JC, Álvarez E, de la Fuente Revenga M, et al. Ayahuasca: pharmacology, neuroscience and therapeutic potential. Brain Res Bull. (2016) 126:89–101. doi: 10.1016/j.brainresbull.2016.03.002
16. Ruffell S, Netzband N, Bird C, Young AH, Juruena MF. The pharmacological interaction of compounds in ayahuasca: a systematic review. Braz J Psychiatry. (2020) 42:646–56. doi: 10.1590/1516-4446-2020-0884
17. Uthaug MV, van Oorsouw K, Kuypers KPC, van Boxtel M, Broers NJ, Mason NL, et al. Sub-acute and long-term effects of ayahuasca on affect and cognitive thinking style and their association with ego dissolution. Psychopharmacology. (2018) 235:2979–89. doi: 10.1007/s00213-018-4988-3
18. Mason NL, Mischler E, Uthaug MV, Kuypers KPC. Sub-acute effects of psilocybin on empathy, creative thinking, subjective well-being. J Psychoactive Drugs. (2019) 51:123–34. doi: 10.1080/02791072.2019.1580804
19. Andersen KAA, Carhart-Harris R, Nutt DJ, Erritzoe D. Therapeutic effects of classic serotonergic psychedelics: a systematic review of modern-era clinical studies. Acta Psychiatr Scand. (2021) 143:101–18. doi: 10.1111/acps.13249
20. Fuchs E, Flügge G. Adult neuroplasticity: more than 40 years of research. Neural Plasticity. (2014) 541870. doi: 10.1155/2014/541870
21. Gulyaeva NV. Molecular mechanisms of neuroplasticity: an expanding universe. Biochemistry. (2017) 82:237–42. doi: 10.1134/S0006297917030014
22. Pathania M, Yan LD, Bordey A. A symphony of signals conducts early and late stages of adult neurogenesis. Neuropharmacology. (2010) 58:865–76. doi: 10.1016/j.neuropharm.2010.01.010
23. Gonçalves JT, Schafer ST, Gage FH. Adult neurogenesis in the hippocampus: from stem cells to behavior. Cell. (2016) 167:897–914. doi: 10.1016/j.cell.2016.10.021
24. Lucassen PJ, Meerlo P, Naylor AS, van Dam AM, Dayer AG, Fuchs E, et al. Regulation of adult neurogenesis by stress, sleep disruption, exercise and inflammation: implications for depression and antidepressant action. Euro Neuropsychopharmacol. (2010) 20:1–17. doi: 10.1016/j.euroneuro.2009.08.003
25. Kempermann G, Song H, Gage FH. Neurogenesis in the Adult Hippocampus. Cold Spring Harb Perspect Biol. (2015) 7:199–207. doi: 10.1101/cshperspect.a018812
26. Ribak CE, Shapiro LA. Dendritic development of newly generated neurons in the adult brain. Brain Res Rev. (2007) 55:390–4. doi: 10.1016/j.brainresrev.2006.12.005
27. Runge K, Cardoso C, de Chevigny A. Dendritic spine plasticity: function and mechanisms. Front Synaptic Neurosci. (2020) 12:36. doi: 10.3389/fnsyn.2020.00036
28. Leal G, Afonso PM, Salazar IL, Duarte CB. Regulation of hippocampal synaptic plasticity by BDNF. Brain Res. (2015) 1621:82–101. doi: 10.1016/j.brainres.2014.10.019
29. Conner JM, Lauterborn JC, Yan Q, Gall CM, Varon S. Distribution of brain-derived neurotrophic factor (BDNF) protein and mRNA in the normal adult rat CNS: evidence for anterograde axonal transport. J Neurosci. (1997) 17:2295–313. doi: 10.1523/JNEUROSCI.17-07-02295.1997
30. Lu B. BDNF and activity-dependent synaptic modulation. Learn Memory. (2003) 10:86–98. doi: 10.1101/lm.54603
31. Binder DK, Scharfman HE. Brain-derived neurotrophic factor. Growth Factors. (2004) 22:123–31. doi: 10.1080/08977190410001723308
32. Kowiański P, Lietzau G, Czuba E, Waśkow M, Steliga A, Moryś J. BDNF: a key factor with multipotent impact on brain signaling and synaptic plasticity. Cell Mol Neurobiol. (2018) 38:579–93. doi: 10.1007/s10571-017-0510-4
33. Brunoni AR, Lopes M, Fregni F. A systematic review and meta-analysis of clinical studies on major depression and BDNF levels: implications for the role of neuroplasticity in depression. Int J Neuropsychopharmacol. (2008) 11:1169–80. doi: 10.1017/S1461145708009309
34. Björkholm C, Monteggia LM. BDNF - a key transducer of antidepressant effects. Neuropharmacology. (2016) 102:72–9. doi: 10.1016/j.neuropharm.2015.10.034
35. Zhou C, Zhong J, Zou B, Fang L, Chen J, Deng X, et al. Meta-analyses of comparative efficacy of antidepressant medications on peripheral BDNF concentration in patients with depression. PLoS ONE. (2017) 12:e172270. doi: 10.1371/journal.pone.0172270
36. Martinowich K, Lu B. Interaction between BDNF and serotonin: role in mood disorders. Neuropsychopharmacology. (2008) 33:73–83. doi: 10.1038/sj.npp.1301571
37. Zarate CA, Jaskaran BS, Carlson PJ, Brutsche NE, Rezvan A, Luckenbaugh DA, et al. A randomized trial of an N-methyl-D-aspartate antagonist in treatment-resistant major depression. Arch Gen Psychiatry. (2006) 63:856–64. doi: 10.1001/archpsyc.63.8.856
38. DiazGranados N, Ibrahim LA, Brutsche NE, Ameli R, Henter ID, Luckenbaugh DA, et al. Rapid resolution of suicidal ideation after a single infusion of an N-methyl-D-aspartate antagonist in patients with treatment-resistant major depressive disorder. J Clin Psychiatry. (2010) 71:1605–11. doi: 10.4088/JCP.09m05327blu
39. Vollenweider FX, Kometer M. The neurobiology of psychedelic drugs: implications for the treatment of mood disorders. Nat Publish Group. (2010) 11:642–51. doi: 10.1038/nrn2884
40. Inserra A, Gregorio D, De, Gobbi G. Psychedelics in psychiatry: neuroplastic, immunomodulatory, neurotransmitter mechanisms. Pharmacol Rev. (2021) 73:202–77. doi: 10.1124/pharmrev.120.000056
41. Morales-Garcia JA, Calleja-Conde J, Lopez-Moreno JA, Alonso-Gil S, Sanz-SanCristobal M, Riba J, et al. N,N-dimethyltryptamine compound found in the hallucinogenic tea ayahuasca, regulates adult neurogenesis in vitro and in vivo. Transl Psychiatry. (2020) 10:331. doi: 10.1038/s41398-020-01011-0
42. Szabo A, Kovacs A, Riba J, Djurovic S, Rajnavolgyi E, Frecska E. The endogenous hallucinogen and trace amine N.N-dimethyltryptamine (DMT) displays potent protective effects against hypoxia via sigma-1 receptor activation in human primary iPSC-derived cortical neurons and microglia-like immune cells. Front Neurosci. (2016) 10:423. doi: 10.3389/fnins.2016.00423
43. Dakic V, Minardi Nascimento J, Costa Sartore R, MacIel RDM, De Araujo DB, Ribeiro S, et al. Short term changes in the proteome of human cerebral organoids induced by 5-MeO-DMT. Sci Rep. (2017) 7:1–13. doi: 10.1038/s41598-017-12779-5
44. Katchborian-Neto A, Santos WT, Nicácio KJ, Corrêa JOA, Murgu M, Martins TMM, et al. Neuroprotective potential of Ayahuasca and untargeted metabolomics analyses: applicability to Parkinson's disease. J Ethnopharmacol. (2020) 255:112743. doi: 10.1016/j.jep.2020.112743
45. Wojtowicz JM, Kee N. BrdU assay for neurogenesis in rodents. Nat Protoc. (2006) 1:1399–405. doi: 10.1038/nprot.2006.224
46. Nardai S, László M, Szabó A, Alpár A, Hanics J, Zahola P, et al. N,N-dimethyltryptamine reduces infarct size and improves functional recovery following transient focal brain ischemia in rats. Exp Neurol. (2020) 327:113245. doi: 10.1016/j.expneurol.2020.113245
47. de Almeida RN, Galvão ACdM, da Silva FS, Silva EAdS, Palhano-Fontes F, Maia-de-Oliveira JP, et al. Modulation of serum brain-derived neurotrophic factor by a single dose of ayahuasca: observation from a randomized controlled trial. Front Psychol. (2019) 10:1234. doi: 10.3389/fpsyg.2019.01234
48. Galvão-Coelho NL, Cecília A, Galvão DM, De Almeida RN, Palhano-fontes F, Braga IC, et al. Changes in inflammatory biomarkers are related to the antidepressant effects of Ayahuasca. J Psychopharmacol. (2020) 34:1–9. doi: 10.1177/0269881120936486
49. Holze F, Vizeli P, Ley L, Müller F, Dolder P, Stocker M, et al. Acute dose-dependent effects of lysergic acid diethylamide in a double-blind placebo-controlled study in healthy subjects. Neuropsychopharmacology. (2020) 46:537–44. doi: 10.1038/s41386-020-00883-6
50. Hutten NR, Mason NL, Dolder PC, Theunissen EL, Holze F, Liechti ME, et al. Low doses of LSD acutely increase BDNF blood plasma levels in healthy volunteers. ACS Pharmacol Transl Sci. (2020) 4:461–6. doi: 10.1021/acsptsci.0c00099
51. Montgomery SA, Asberg M. A new depression scale designed to be sensitive to change. Br J Psychiatry. (1979) 134:382–9. doi: 10.1192/bjp.134.4.382
52. Moher D, Liberati A, Tetzlaff J, Altman DG, Altman D, Antes G, et al. Preferred reporting items for systematic reviews and meta-analyses: the PRISMA statement. PLoS Med. (2009) 6:e1000097. doi: 10.1371/journal.pmed.1000097
53. Ly C, Greb AC, Cameron LP, Wong JM, Barragan EV, Wilson PC, et al. Psychedelics promote structural and functional neural plasticity. Cell Rep. (2018) 23:3170–82. doi: 10.1016/j.celrep.2018.05.022
54. Lima da Cruz RV, Moulin TC, Petiz LL, Leão RN. A single dose of 5-MeO-DMT stimulates cell proliferation, neuronal survivability, morphological and functional changes in adult mice ventral dentate gyrus. Front Mol Neurosci. (2018) 11:312. doi: 10.3389/fnmol.2018.00312
55. Jefsen OH, Elfving B, Wegener G, Müller HK. Transcriptional regulation in the rat prefrontal cortex and hippocampus after a single administration of psilocybin. J Psychopharmacol. (2020) 35:1–11. doi: 10.1177/0269881120959614
56. González-Maeso J, Yuen T, Ebersole BJ, Wurmbach E, Lira A, Zhou M, et al. Transcriptome fingerprints distinguish hallucinogenic and nonhallucinogenic 5-hydroxytryptamine 2A receptor agonist effects in mouse somatosensory cortex. J Neurosci. (2003) 23:8836–43. doi: 10.1523/JNEUROSCI.23-26-08836.2003
57. Nichols CD, Sanders-Bush E. A single dose of lysergic acid diethylamide influences gene expression patterns within the mammalian brain. Neuropsychopharmacology. (2002) 26:634–42. doi: 10.1016/S0893-133X(01)00405-5
58. Nichols CD, Garcia EE, Sanders-Bush E. Dynamic changes in prefrontal cortex gene expression following lysergic acid diethylamide administration. Mol Brain Res. (2003) 111:182–8. doi: 10.1016/S0169-328X(03)00029-9
59. Nichols CD, Sanders-Bush E. Molecular genetic responses to lysergic acid diethylamide include transcriptional activation of MAP kinase phosphatase-1, C/EBP-β and ILAD-1, a novel gene with homology to arrestins. J Neurochem. (2004) 90:576–84. doi: 10.1111/j.1471-4159.2004.02515.x
60. Jha S, Rajendran R, Fernandes KA, Vaidya VA. 5-HT2A/2Creceptor blockade regulates progenitor cell proliferation in the adult rat hippocampus. Neurosci Lett. (2008) 441:210–4. doi: 10.1016/j.neulet.2008.06.028
61. Martin DA, Marona-Lewicka D, Nichols DE, Nichols CD. Chronic LSD alters gene expression profiles in the mPFC relevant to schizophrenia. Neuropharmacology. (2014) 83:1–8. doi: 10.1016/j.neuropharm.2014.03.013
62. Catlow BJ, Song S, Paredes DA, Kirstein CL, Sanchez-Ramos J. Effects of psilocybin on hippocampal neurogenesis and extinction of trace fear conditioning. Exp Brain Res. (2013) 228:481–91. doi: 10.1007/s00221-013-3579-0
63. Colaço CS, Alves SS, Nolli LM, Pinheiro WO, de Oliveira DGR, Santos BWL, et al. Toxicity of ayahuasca after 28 days daily exposure and effects on monoamines and brain-derived neurotrophic factor (BDNF) in brain of Wistar rats. Metab Brain Dis. (2020) 35:739–51. doi: 10.1007/s11011-020-00547-w
64. Kourrich S, Su TP, Fujimoto M, Bonci A. The sigma-1 receptor: roles in neuronal plasticity and disease. Trends Neurosci. (2012) 35:762–71. doi: 10.1016/j.tins.2012.09.007
65. Wingenfeld K, Wolf OT. Stress, memory, the hippocampus. Hippocampus Clin Neurosci. (2014) 34:109–20. doi: 10.1159/000356423
66. Zaben MJ, Gray WP. Neuropeptides and hippocampal neurogenesis. Neuropeptides. (2013) 47:431–8. doi: 10.1016/j.npep.2013.10.002
67. Reichmann F, Holzer P. Neuropeptide Y: a stressful review. Neuropeptides. (2016) 55:99–109. doi: 10.1016/j.npep.2015.09.008
68. Maren S. Neurobiology of pavlovian fear conditioning. Annu Rev Neurosci. (2001) 24:897–931. doi: 10.1146/annurev.neuro.24.1.897
69. Osorio F, de L, Sanches RF, Macedo LR, dos Santos RG, Maia-de-Oliveira JP, et al. Antidepressant effects of a single dose of ayahuasca in patients with recurrent depression: a preliminary report. Rev Brasil Psiquiatria. (2015) 37:13–20. doi: 10.1590/1516-4446-2014-1496
70. Farzin D, Mansouri N. Antidepressant-like effect of harmane and other β-carbolines in the mouse forced swim test. Euro Neuropsychopharmacol. (2006) 16:324–8. doi: 10.1016/j.euroneuro.2005.08.005
71. Fortunato JJ, Réus GZ, Kirsch TR, Stringari RB, Fries GR, Kapczinski F, et al. Chronic administration of harmine elicits antidepressant-like effects and increases BDNF levels in rat hippocampus. J. Neural Transm. (2010) 117:1131–7. doi: 10.1007/s00702-010-0451-2
72. Fortunato JJ, Réus GZ, Kirsch TR, Stringari RB, Stertz L, Kapczinski F, et al. Acute harmine administration induces antidepressive-like effects and increases BDNF levels in the rat hippocampus. Progress Neuro-Psychopharmacol Biol Psychiatry. (2009) 33:1425–30. doi: 10.1016/j.pnpbp.2009.07.021
73. Morales-García JA, De La Fuente Revenga M, Alonso-Gil S, Rodríguez-Franco MI, Feilding A, Perez-Castillo A, et al. The alkaloids of Banisteriopsis caapi, the plant source of the Amazonian hallucinogen Ayahuasca, stimulate adult neurogenesis in vitro. Sci Rep. (2017) 7:5309. doi: 10.1038/s41598-017-05407-9
74. Pandy V. A simple method for animal dose calculation in preclinical research. EC Pharmacol Toxicol. (2020) 8:1–2.
75. Nair A, Jacob S. A simple practice guide for dose conversion between animals and human. J Basic Clin Pharmacy. (2016) 7:27. doi: 10.4103/0976-0105.177703
76. Marques AA, Bevilaqua MC, da Fonseca AM, Nardi AE, Thuret S, Dias GP. Gender differences in the neurobiology of anxiety: focus on adult hippocampal neurogenesis. Neural Plast. (2016) 2016:14. doi: 10.1155/2016/5026713
77. Bath KG, Schilit A, Lee FS. Stress effects on BDNF expression: effects of age, sex, and form of stress. Neuroscience. (2013) 239:149–56. doi: 10.1016/j.neuroscience.2013.01.074
78. Oberlander XJG, Woolley CS. Erratum: 17β-Estradiol acutely potentiates glutamatergic synaptic transmission in the hippocampus through distinct mechanisms in males and females (The journal of neuroscience, (2016) 36, 9, (2677-2690), 10.1523/JNEUROSCI.4437-15.2016). J Neurosci. (2017) 37:12314–27. doi: 10.1523/JNEUROSCI.3011-17.2017
79. Carrier N, Kabbaj M. Sex differences in the antidepressant-like effects of ketamine. Neuropharmacology. (2013) 70:27–34. doi: 10.1016/j.neuropharm.2012.12.009
80. Kokras N, Dalla C. Preclinical sex differences in depression and antidepressant response: implications for clinical research. J Neurosci Res. (2017) 95:731–6. doi: 10.1002/jnr.23861
81. Castrén E, Kojima M. Brain-derived neurotrophic factor in mood disorders and antidepressant treatments. Neurobiol Dis. (2017) 97:119–26. doi: 10.1016/j.nbd.2016.07.010
82. Pillai A, Kale A, Joshi S, Naphade N, Raju MSVK, Nasrallah H, et al. Decreased BDNF levels in CSF of drug-naive first-episode psychotic subjects: correlation with plasma BDNF and psychopathology. Int J Neuropsychopharmacol. (2010) 13:535–9. doi: 10.1017/S1461145709991015
83. Pillai A, Bruno D, Sarreal AS, Hernando RT, Saint-Louis LA, Nierenberg J, et al. Plasma BDNF levels vary in relation to body weight in females. PLoS ONE. (2012) 7:1–6. doi: 10.1371/journal.pone.0039358
84. Charan J, Kantharia N. How to calculate sample size in animal studies? J Pharmacol Pharmacother. (2013) 4:303–6. doi: 10.4103/0976-500X.119726
85. Button KS, Ioannidis JPA, Mokrysz C, Nosek BA, Flint J, Robinson ESJ, et al. Power failure: why small sample size undermines the reliability of neuroscience. Nat Rev Neurosci. (2013) 14:365–76. doi: 10.1038/nrn3475
86. Beique J.-C, Imad M, Mladenovic L, Gingrich JA, Andrade R. Mechanism of the 5-hydroxytryptamine 2A receptor-mediated facilitation of synaptic activity in prefrontal cortex. Proc Natl Acad Sci. (2007) 104:9870–5. doi: 10.1073/pnas.0700436104
87. Andrade R. Serotonergic regulation of neuronal excitability in the prefrontal cortex. Neuropharmacology. (2011) 61:382–6. doi: 10.1016/j.neuropharm.2011.01.015
88. Kurrasch-Orbaugh DM, Parrish JC, Watts VJ, Nichols DE. A complex signaling cascade links the serotonin2A receptor to phospholipase A2 activation: the involvement of MAP kinases. J Neurochem. (2003) 86:980–91. doi: 10.1046/j.1471-4159.2003.01921.x
89. Qu Y, Chang L, Klaff J, Balbo A, Rapoport SI. Imaging brain phospholipase A2 activation in awake rats in response to the 5-HT2A/2C agonist (±)2,5-dimethoxy-4-iodophenyl-2-aminopropane (DOI). Neuropsychopharmacology. (2003) 28:244–52. doi: 10.1038/sj.npp.1300022
90. González-Maeso J, Weisstaub NV, Zhou M, Chan P, Ivic L, Ang R, et al. Hallucinogens recruit specific cortical 5-HT2AReceptor-mediated signaling pathways to affect behavior. Neuron. (2007) 53:439–52. doi: 10.1016/j.neuron.2007.01.008
91. Aghajanian GK, Marek GJ. Serotonin and hallucinogens. Neuropsychopharmacology. (1999) 21:16S–23S. doi: 10.1016/S0893-133X(98)00135-3
92. Mason NL, Kuypers KPC, Müller F, Reckweg J, Tse DHY, Toennes SW, et al. Me, myself, bye: regional alterations in glutamate and the experience of ego dissolution with psilocybin. Neuropsychopharmacology. (2020) 45:2003–11. doi: 10.1038/s41386-020-0718-8
93. Sheldon PW, Aghajanian GK. Serotonin (5-HT) induces IPSPs in pyramidal layer cells of rat piriform cortex: evidence for the involvement of a 5-HT2 -activated interneuron. Brain Res. (1990) 506:62–9. doi: 10.1016/0006-8993(90)91199-Q
94. Pérez-Cadahía B, Drobic B, Davie JR. Activation and function of immediate-early genes in the nervous system. Biochem Cell Biol. (2011) 89:61–73. doi: 10.1139/O10-138
95. Plath N, Ohana O, Dammermann B, Errington ML, Schmitz D, Gross C, et al. Arc/Arg3.1 is essential for the consolidation of synaptic plasticity and memories. Neuron. (2006) 52:437–44. doi: 10.1016/j.neuron.2006.08.024
96. Lin L, Carter J, Gao X, Whitehead J, Tourtellotte WG. The neuroplasticity-associated arc gene is a direct transcriptional target of early growth response (Egr) transcription factors. Mol Cell Biol. (2005) 25:10286–300. doi: 10.1128/MCB.25.23.10286-10300.2005
97. Brunet A, Park J, Tran H, Hu LS, Hemmings BA, Greenberg ME. Protein kinase SGK mediates survival signals by phosphorylating the forkhead transcription Factor FKHRL1 (FOXO3a). Mol Cell Biol. (2001) 21:952–65. doi: 10.1128/MCB.21.3.952-965.2001
98. Bridi MS, Abel T. The NR4A orphan nuclear receptors mediate transcription-dependent hippocampal synaptic plasticity. Neurobiol Learn Mem. (2013) 105:151–8. doi: 10.1016/j.nlm.2013.06.020
99. Hayashi T, Su TP. Sigma-1 receptor chaperones at the ER- mitochondrion interface regulate Ca2+ signaling and cell survival. Cell. (2007) 131:596–610. doi: 10.1016/j.cell.2007.08.036
100. Carbonaro TM, Gatch MB. Neuropharmacology of N.N-dimethyltryptamine. Brain Res Bull. (2016) 126:74–88. doi: 10.1016/j.brainresbull.2016.04.016
101. Ryskamp DA, Zhemkov V, Bezprozvanny I. Mutational analysis of sigma-1 receptor's role in synaptic stability. Front Neurosci. (2019) 13:1012. doi: 10.3389/fnins.2019.01012
102. Inserra A. Hypothesis: the psychedelic ayahuasca heals traumatic memories via a sigma 1 receptor-mediated epigenetic-mnemonic process. Front Pharmacol. (2018) 9:330. doi: 10.3389/fphar.2018.00330
103. Moriguchi S, Shinoda Y, Yamamoto Y, Sasaki Y, Miyajima K, Tagashira H, et al. Stimulation of the sigma-1 receptor by DHEA enhances synaptic efficacy and neurogenesis in the hippocampal dentate gyrus of olfactory bulbectomized mice. PLoS ONE. (2013) 8:e60863. doi: 10.1371/journal.pone.0060863
104. Strajhar P, Schmid Y, Liakoni E, Dolder PC, Rentsch KM, Kratschmar DV, et al. Acute effects of lysergic acid diethylamide on circulating steroid levels in healthy subjects. J Neuroendocrinol. (2016) 28:12374. doi: 10.1111/jne.12374
105. Yagasaki Y, Numakawa T, Kumamaru E, Hayashi T, Su TP, Kunugi H. Chronic antidepressants potentiate via sigma-1 receptors the brain-derived neurotrophic factor-induced signaling for glutamate release. J Biol Chem. (2006) 281:12941–9. doi: 10.1074/jbc.M508157200
106. Narita N, Hashimoto K, Tomitaka SI, Minabe Y. Interactions of selective serotonin reuptake inhibitors with subtypes of σ receptors in rat brain. Eur J Pharmacol. (1996) 307:117–9. doi: 10.1016/0014-2999(96)00254-3
107. Lepack AE, Bang E, Lee B, Dwyer JM, Duman RS. Fast-acting antidepressants rapidly stimulate ERK signaling and BDNF release in primary neuronal cultures. Neuropharmacology. (2016) 111:242–52. doi: 10.1016/j.neuropharm.2016.09.011
108. Zhou W, Wang N, Yang C, Li XM, Zhou ZQ, Yang JJ. Ketamine-induced antidepressant effects are associated with AMPA receptors-mediated upregulation of mTOR and BDNF in rat hippocampus and prefrontal cortex. Euro Psychiatry. (2014) 29:419–23. doi: 10.1016/j.eurpsy.2013.10.005
109. Savalia NK, Shao L, Kwan AC. Neurosciences opinion a dendrite-focused framework for understanding the actions of ketamine and psychedelics. Trends Neurosci. (2020) 44:1–16. doi: 10.1016/j.tins.2020.11.008
110. Hasler G. Toward specific ways to combine ketamine and psychotherapy in treating depression. CNS Spectr. (2020) 25:445–7. doi: 10.1017/S1092852919001007
111. Aleksandrova LR, Phillips AG, Wang YT. Antidepressant effects of ketamine and the roles of AMPA glutamate receptors and other mechanisms beyond NMDA receptor antagonism. J Psychiatry Neurosci. (2017) 42:222–9. doi: 10.1503/jpn.160175
Keywords: psychedelics, structural neuroplasticity, functional neuroplasticity, molecular neuroplasticity, cellular neuroplasticity
Citation: de Vos CMH, Mason NL and Kuypers KPC (2021) Psychedelics and Neuroplasticity: A Systematic Review Unraveling the Biological Underpinnings of Psychedelics. Front. Psychiatry 12:724606. doi: 10.3389/fpsyt.2021.724606
Received: 13 June 2021; Accepted: 19 August 2021;
Published: 10 September 2021.
Edited by:
Joanna Caroline Neill, The University of Manchester, United KingdomReviewed by:
Ahmad Adam Khundakar, Teesside University, United KingdomCopyright © 2021 de Vos, Mason and Kuypers. This is an open-access article distributed under the terms of the Creative Commons Attribution License (CC BY). The use, distribution or reproduction in other forums is permitted, provided the original author(s) and the copyright owner(s) are credited and that the original publication in this journal is cited, in accordance with accepted academic practice. No use, distribution or reproduction is permitted which does not comply with these terms.
*Correspondence: Kim P. C. Kuypers, ay5rdXlwZXJzQG1hYXN0cmljaHR1bml2ZXJzaXR5Lm5s
Disclaimer: All claims expressed in this article are solely those of the authors and do not necessarily represent those of their affiliated organizations, or those of the publisher, the editors and the reviewers. Any product that may be evaluated in this article or claim that may be made by its manufacturer is not guaranteed or endorsed by the publisher.
Research integrity at Frontiers
Learn more about the work of our research integrity team to safeguard the quality of each article we publish.