- 1Laboratory of Psychiatric Neuroscience, Centre for Molecular Therapeutics, James Cook University, Townsville, QLD, Australia
- 2Australian Institute of Tropical Health and Medicine, James Cook University, Townsville, QLD, Australia
- 3Faculty of Health and Life Sciences, Psychology, Northumbria University, Newcastle upon Tyne, United Kingdom
The gut microbiome is rapidly becoming the focus of interest as a possible factor involved in the pathophysiology of neuropsychiatric disorders. Recent understanding of the pathophysiology of schizophrenia emphasizes the role of systemic components, including immune/inflammatory and metabolic processes, which are influenced by and interacting with the gut microbiome. Here we systematically review the current literature on the gut microbiome in schizophrenia-spectrum disorders and in their animal models. We found that the gut microbiome is altered in psychosis compared to healthy controls. Furthermore, we identified potential factors related to psychosis, which may contribute to the gut microbiome alterations. However, further research is needed to establish the disease-specificity and potential causal relationships between changes of the microbiome and disease pathophysiology. This can open up the possibility of. manipulating the gut microbiome for improved symptom control and for the development of novel therapeutic approaches in schizophrenia and related psychotic disorders.
Introduction
The gut microbiome has recently received considerable interest, due to its potential role in maintaining health and in the pathophysiology of chronic diseases (1). Our understanding of the involvement of the gut microbiome in diseases is fast increasing due to the emergence of new molecular biological techniques (2). In healthy individuals, the gut microbiome, which consists of more than 100 trillion bacteria (3, 4), has a symbiotic relationship with enteric cells to influence physiological function (5). The gut microbiome is highly variable between healthy individuals (3), with twins only sharing 50% of their species-level bacterial taxa (6). This interindividual difference is shaped through host-extrinsic, host-intrinsic, and environmental factors (2, 3). Host-extrinsic factors include lifestyles, such as physical activity, cultural habits, medication, and diet (2). Environmental factors altering the microbiome include the local environment and maternal transmission (2). Host-intrinsic factors, which might shape the gut microbiota, are genetics, sex, innate, and adaptive immunity, as well as metabolic factors, i.e., body mass index (2, 7). Although interindividual differences exist in healthy individuals, studies have demonstrated clear separations of the gut microbiome in chronic diseases such as individuals with allergies (8–10), celiac disease (11), gastric cancer (12), inflammatory bowel disease (13, 14) including Crohn’s disease (15) and ulcerative colitis (3, 16), obesity (3, 17, 18), anorexia (17, 19), and type 2 diabetes mellitus (20) compared to healthy controls. The functional importance of the gut microbiome was demonstrated by the transfer of the gut microbiome from obese to germ-free mice resulting in obesity (21).
Bidirectional communication has been well established between the gut and the brain and its importance for maintaining neuronal, hormonal and immunological homeostasis has been recently demonstrated (22). A damage to the integrity of the gut-brain communication results in altered brain function and behavior (23). More recently, the importance of the gut-brain axis has been highlighted as a possible contributing factor, among many others, such as genes, early environment and nutrition, in the development of neuropsychiatric disorders (5, 22).
Schizophrenia is a heterogeneous, chronic neurodevelopmental psychiatric spectrum disorder influenced by a hitherto poorly understood interaction between genetic and environmental factors (24). It affects about 1 in 100 people (1%) worldwide (25). A variety of different pathophysiological mechanisms have been proposed, such as the dopamine hyperactivity in certain brain systems (26, 27), impaired glutamate neurotransmission (28), and a disruption of the brain glucose and energy metabolism (29–32). It has been conceptualized that multiple environmental “hits” on the background of a genetic predisposition are required for its development (33, 34). Genome-wide association studies have shown that schizophrenia is a polygenic disorder with a complex array of contributing risk loci across the allelic frequency spectrum (35, 36). Environmental events throughout development and adulthood, such as viruses before birth, method of delivery, birth complications, and psychosocial traumas, are important in the pathophysiology of schizophrenia (27) and the shaping of the gut microbiome (37–39). Most recently, a study demonstrated that mice receiving feces from individuals with schizophrenia showed a behavioral phenotype that is consistent with that have been seen in animal models of schizophrenia and depression (40). This finding demonstrates that a constituent of the fecal matter have effect of brain function and behavior of the host and strengthen the suggestion that the microbiome might contribute to behavioral symptoms in psychosis (41).
In this systematic review, we summarize the most recent findings on the gut microbiome in psychosis, including animal models and clinical data. Furthermore, we identified potential factors particular to psychosis, which may contribute to the altered gut microbiome. The methodology of the studies covered was not described in details as these were extensively reviewed elsewhere (42). Compared to previous reviews (43) we provide detailed discussion of factors such as antipsychotic use, lifestyle and environmental factors as well as the potential pathological role of the microbiome in psychosis relevant to microbial changes.
Methods
Eligibility and Inclusion Criteria
We included original articles investigating preclinical and clinical studies exploring the fecal microbiome in animal models of schizophrenia and individuals of all ages with psychosis or at high risk and schizophrenia along with respective controls. Only studies published in English were included without a date restriction throughout the database search.
Database Search Strategy
This study followed the Preferred Items for Reporting Systematic Reviews and Meta-analysis Protocols (PRISM-P) (44). One author (AKK) conducted a Scopus, Web of Science, and PubMed database searches until the cut-off date of 14/02/2019. In all databases, free-text terms included (microbiota OR gastrointestinal microbiome OR microbiome OR microbio*) AND (schizophreni* OR “Dementia Praecox” OR psychotic OR schizoaffective OR psychoses OR psychosis). The search was limited to original articles, and therefore we excluded reviews, meta-analyses, and systematic reviews. The reference lists of eligible papers were manually screened for further relevant articles.
Report Selection
One of the authors (A-KK) determined the eligibility of papers by screening titles and abstracts for relevance. Eligible documents were then read as a whole to analyze if the articles matched the inclusion criteria. Excluded articles were documented, and reasons were given for exclusion.
Data Extraction
A-KK extracted information from relevant publications such as animal and patient characteristics. Study characteristics for animal experiments included strain, sex, number, age, and weight of animals and “schizophrenia” induction method, length of study, the timing of fecal sample collection, microbiota, and other findings of the study. Human studies were characterized by the number of participants, gender, age, exclusion and inclusion criteria, microbiota findings, and other findings within the study.
SYRCLE’s Risk of Bias Analysis
The overall risk of bias (RoB) was assessed in animal studies using an adapted SYRCLE’s risk of bias tool (45). All ten entries of the SYRCLE’s RoB tool were assessed by the authors (A-KK, RP) relating to selection, performance, detection, attrition, reporting, and other biases (45). All individual entries were assigned as “low RoB,” “high RoB,” “unclear,” or “not feasible.” A parameter was determined “unclear” if the item was not mentioned in the publication. The only exception to this was item 8, where ‘not mentioned’ was scored “high RoB.” For one article, it was not feasible to assess housing conditions (items 3, 4, and 5) due to the nature of that particular animal model of schizophrenia (46). Furthermore, studies were assessed for quality by answering the categories: (1) conflict of interest stated, (2) power analysis or sample size calculation, (3) experiment blinding at any level, and (4) randomization at any level.
STROBE Risk of Bias Analysis
Human studies were assessed using adapted STROBE assessment criteria, including 32 subsections, which were scored for all six studies. A-KK and RP assessed the completeness of reporting (CoR) score (CoR (%)=(yes/(yes+no))*100) by answering each recommendation in the STROBE statement with “yes” or “no.”
Microbiome Methodological Consideration
All studies were investigate for their CoR for microbiome relevant methodology. Categories range from sample preparation, handling to analysis of samples. The CoR score was calculated as described in the previous section. The overall CoR score was calculated for all studies, animal studies alone and human studies alone.
Results
Database Search
The initial search yielded 763 documents, including one additional record identified through other sources. After exclusion of duplicates, 673 articles remained and were included for evaluation of titles or abstracts, which resulted in 69 records for full-text article review. During full-text article review, 60 articles were excluded because they were not original research articles (reviews, n=43; letter to the editor, n=1; book chapter, n=1), referred to microbiomes other than the gut microbiome (oropharyngeal, n=4; blood, n=2), was not a mouse model of schizophrenia (n=4), or reported no control (n=5). One additional article was found during scanning of the references. This search resulted in nine articles included in this systematic review (Figure 1).
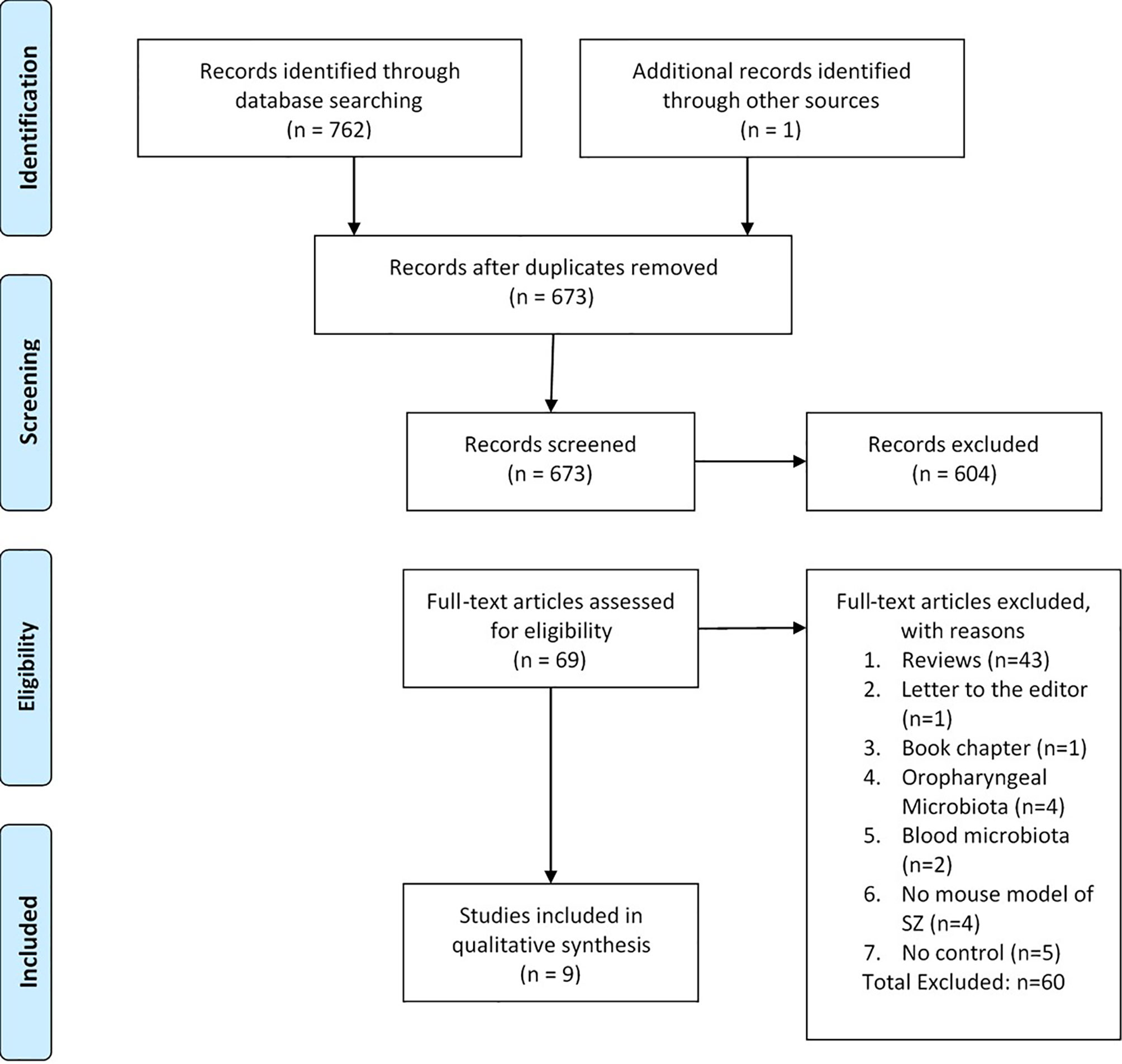
Figure 1 Flow diagram (47).
Study Characteristics
Animals
Preclinical publications included within this review used three different types of translationally validated animal models. A neurodevelopmental model, the maternal immune activation (MIA), which takes advantage of the finding that maternal infection during pregnancy increases the risk of developing schizophrenia in the offspring, by injecting pregnant females with viral mimic polyriboinosinic–polyribocytidilic acid (Poly I:C), which disrupts prenatal and early postnatal development (48). A pharmacological model, the N-methyl-D-aspartate (NMDA) receptor hypofunction model induced by administration of the NMDA receptor antagonist phencyclidine, is based on the findings that the administration of NMDA antagonists [phencyclidine (PCP) and ketamine] induce schizophrenia-like behaviors (49, 50). Social isolation is a major “psychological” stressor that has been used over the years to induce a behavioral and neurochemical phenotype corresponding to schizophrenia (51). This alteration has long-lasting effects on the brain and behavior (48). Articles describing preclinical results included 144 male Lister-Hooded rats investigated the microbiome in a pharmacological, phencyclidine (52), and a developmental model (social isolation) (46) (Table 1). In the neurodevelopmental model, the gut microbiome was assessed in ten C57/Bl6 offspring in the MIA group and ten respective controls with unknown sex (53). The two studies using rats had similar size rats of 100-130 g (46, 52); only one study reported that rats were 24 days old (46). C57BL/6N mice were six weeks of age (53). All studies analyzed microbiome using 16S rRNA gene MiSeq-based high throughput sequencing (46, 52, 53). All studies were supported by various sources of funding (46, 52, 53).
Human
Six eligible studies were identified, which investigated high-risk and ultrahigh-risk (UHR) individuals (4), first-episode psychosis (54), first-episode schizophrenia (55), and individuals with chronic schizophrenia (40, 56, 57) all compared to healthy controls (Table 2). High-risk (or at risk) state is the clinical presentation of those considered at risk of developing psychosis or schizophrenia. Such states were formerly considered as prodromes, emerging symptoms of psychosis, but this view is no longer maintained as a prodromal period can not be confirmed unless the emergence of the condition has occurred. Individuals are considered UHR for psychosis if they meet a set of standardized criteria including presumed genetic vulnerability (Trait), or a recent history of Attenuated Psychotic Symptoms (APS) or Brief Limited Intermittent Psychotic Symptoms (BLIPS) Yung, McGorry (58, 59). First-episode and chronic schizophrenia are defined in Table 2. A total of 321 patients and 273 healthy controls were investigated. All studies reported no significant differences between the experimental and control group for age, sex, and weight. Age varied widely between studies due to different stages of the disorder from 20.47 ± 4.57 to 54.7 ± 10.7. Common exclusion criteria included factors potentially influencing the gut microbiome such as gastrointestinal and endocrine disorders, previous antibiotic or probiotic treatment, alcohol and substance abuse. Inclusion criteria varied greatly between studies due to different baseline diagnostic criteria.
Risk of Bias
Animals
Of the 30 SYRCLE entries of all three studies, 15 (50%) were low RoB, 2 (6.6%) were high RoB, 10 (33.3%) were unclear, and 3 (10%) were considered not feasible (Figure 2).
Quality Assessment
All studies were randomized and acknowledged their funding source (Figure 3). However, none of the studies reported how the sample size was estimated during the design of the experiment (Figure 3). One study failed to report if the experimenter was blinded at any level.
Human Studies
The analysis included a total of 192 entries (32 STROBE entries per study). Of the 192 STROBE entries, 139 (72.4%) entries were scores “Yes” for CoR, and 53 (27.6%) were scored “No” for CoR (Figure 4).
Microbiome Methodological Consideration
The analysis included a total of 63 entries (7 entries per study). Of the 63 entries, 47 (74.6%) entries were scores “Yes” for CoR, and 16 (25.4%) were scored “No” for CoR (Figure 5). One study within this review did not use caecum samples rather than fecal samples (46). None of the animal studies reported the amount used for microbial analysis (46, 52, 53). Reporting for the amount of fecal sample varied within human studies with two studies failing to report amount used (40, 55). Similarly, mixed results were found for storage information given with two animal studies (52, 53) and one human study (55) not reporting the storage of samples between collection and analysis. Three studies did not report target regions (53–55). All studies reported the sequencing platform used (4, 40, 46, 52–57) and all human studies reported the DNA extraction protocol and PCR primers used (4, 40, 54–57). However, none of the animal studies reported the DNA extraction protocol (46, 52, 53) and one animal study failed to report PCR primers used. The major of publications within this review used 16S rRNA sequencing (4, 40, 46, 52, 53, 56, 57). Two studies used alternative techniques such as RT-qPCR for 16 s primers (54) or qPCR for 16 s primers (55). Studies, which reported target region investigated V3 or V4 regions (4, 46, 52, 56, 57). The preferred sequencing platform was the Illumina Miseq platform (4, 46, 52, 54, 56, 57).
Overall, we found a CoR score of 74.6% for all studies, which a large divide between CoR of animal (CoR: 47.6%) and human studies (CoR: 88.1%).
Microbiome Analysis and Its Relationship to Behavior
In the following sections, we summarize the taxonomic changes in validated animal models of schizophrenia (46, 52, 53) and high-risk and UHR individuals (4), first-episode psychosis (54), first-episode schizophrenia (55), and individuals with chronic schizophrenia (40, 56, 57), all compared to healthy controls. The reviewed publications consistently report OTU (operational taxonomic unit) values, alpha and beta diversity, terms not frequently used outside the field of microbiome research. OTU is used to cluster sequences based on their similarities (60). Alpha diversity (within-sample) is the species number (richness) and distribution (evenness) within a host organism or habitat, showing “how many different species were found,” i.e., how many different bacteria are in a healthy individual, which can be measured using Shannon diversity index and Faith’s Phylogenetic Diversity (56). Beta diversity (between-samples) answers the question “How different is the microbial composition in one environment compared to another?”, calculated using Bray-Curtis dissimilarity and unweighted UniFrac and ordinated using principal coordinate analysis (PCoA).
Within the Results section we will be reporting changes according to phylum levels, this structure will remain for the discussion, however we will be discussion changes at lower taxonomic units within the phylum sections of the discussion. Details of the taxonomic changes are described in Tables 1 and 2 and Figure 6 showing reduced abundance in orange, increased abundance in purple with lighter shades of orange and purple to signify that only preclinical evidence is available.
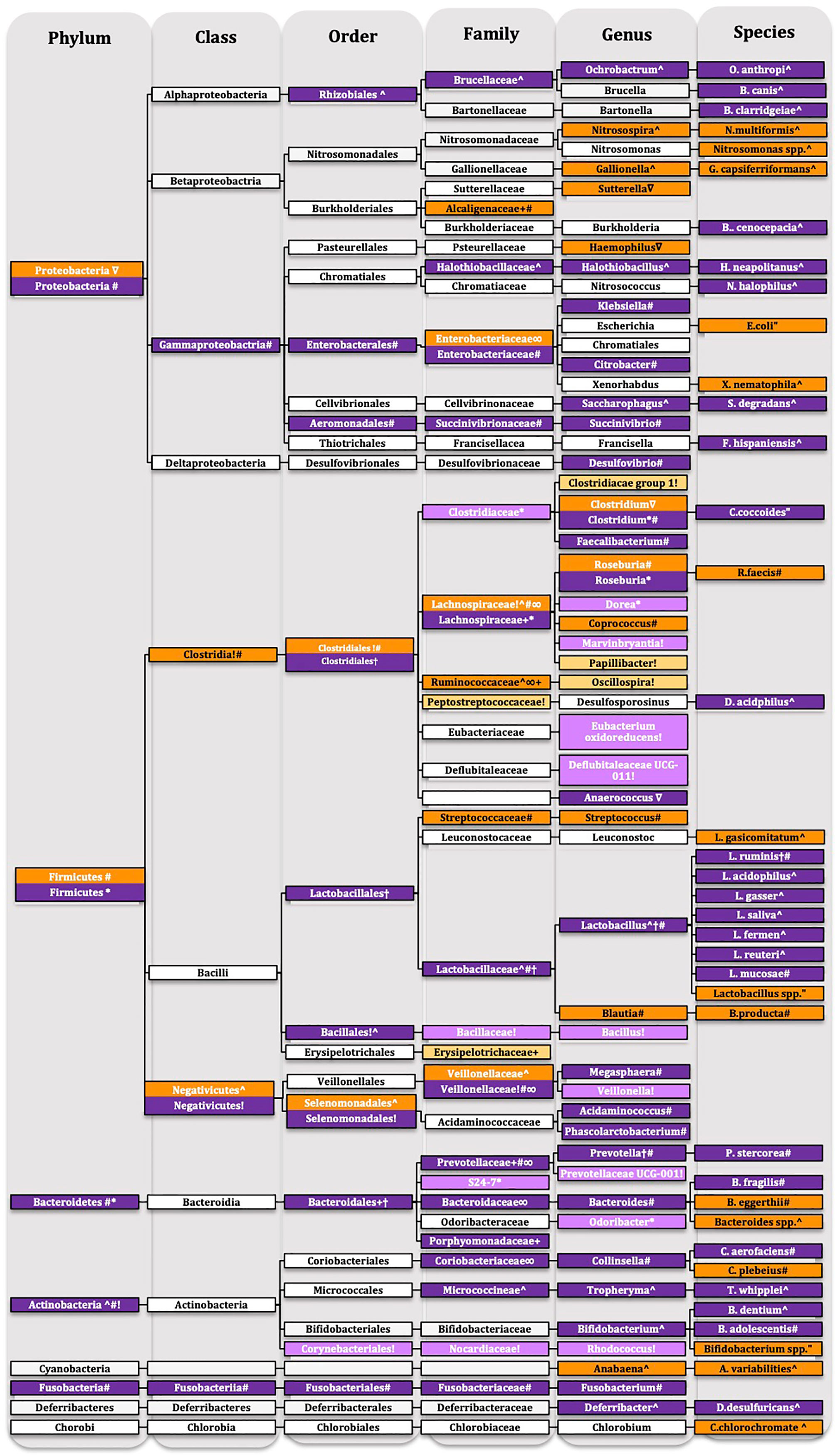
Figure 6 Taxonomic tree of schizophrenia. Showing reduced abundance in orange, increased abundance in purple with lighter shades of orange and purple to signify that only preclinical evidence is available. White-no change only for representative purposes. Ultrahigh-risk individuals †(4), first episode psychosis ^ (54), first episode schizophrenia “(55), chronic schizophrenia # (57) ∇(56) ∞(40), maternal immune activation model + (53), pharmacological model * (52), social isolation! (46).
Animals
One study investigated the gut microbiota of C57BL/6N offspring in the neurodevelopmental MIA model (53). MIA mice, from as early as three weeks, showed increased intestinal permeability, which was shown through increased translocation of fluorescein isothiocyanate-dextran across the intestinal epithelium (53). Alpha diversity is the species richness within a host organism or habitat, showing “how many different species were found,” i.e., how many different bacteria are in a healthy individual. This remains unaltered in MIA mice. Beta diversity, which reflects the species diversity to contribute to species evenness between microbial communities, i.e., how different was the diversity of bacteria between healthy controls compared to diseased individuals, was significantly altered by the MIA. PCoA (index of beta-diversity) showed that MIA samples clustered significantly differently to control samples, indicating different gut microbiome composition compared to control animals. The primary drivers of the gut microbiome changes concerning diversity were the classes Clostridia and Bacteroidia. MIA significantly altered families in the phyla Bacteroidetes, Firmicutes, and Proteobacteria compared to controls (53) (Figure 6 and Table 1).
Subchronic administration of phencyclidine for seven days, a pharmacological model of schizophrenia, significantly separated the microbiota population compared to controls (beta-diversity) (52). Locomotor activity was increased in phencyclidine treated animals with seven days and four weeks wash-out period compared to controls, which indicates a schizophrenia-like behavioral phenotype (52) (Table 1). Seven days after treatment with phencyclidine, no change was found in alpha-diversity. However, a weak but significant alteration was found in beta-diversity compared to controls using the PCoA-analysis, which indicates the separation of the microbial communities. Phencyclidine-treated animals showed increased abundance in genera belonging to the phyla Firmicutes and Bacteroidetes (52) (Figure 6 and Table 1). Four weeks after phencyclidine treatment, no changes in alpha-diversity and beta-diversity were found between groups. However, the abundance of genera within the phyla Firmicutes and Bacteroidetes were significantly increased in phencyclidine-treated animals (52) (Figure 6 and Table 1).
Social isolation resulted in hyperactivity, anxiety-like behavior, and impaired contextual learning and memory, as well as reduced IL-6 and IL-10 levels in the hippocampus (46). Although no significant changes for alpha-diversity and beta-diversity were found, socially isolated animals showed a trend toward a decrease in alpha diversity and a trend towards differential clustering of microbial communities (beta diversity) (46) (Figure 6 and Table 1). Social isolation increased the abundance of Actinobacteria at phylum level. At class, order, family, and genus level social isolation altered the abundance to both directions of the phyla Firmicutes, Actinobacteria, and Bacteroidetes (46) (Figure 6 and Table 1).
In summary, the preclinical studies using translationally valid models for schizophrenia show somewhat inconsistent findings with the decreased abundance of the phylum Proteobacteria emerging as a partially shared feature (53). At the same time, Actinobacteria (46) and Bacteroidetes (46, 52, 53) were increased, whereas bacteria within the phylum Firmicutes show altered expression toward both directions.
Human Studies
High-risk and UHR individuals who have a higher likelihood of developing psychosis in the future did not differ in microbial richness, alpha-diversity. However, beta-diversity was altered in high-risk and UHR individual’s analysis compared to controls. UHR individuals had increased bacterial abundance at order, genus, and species levels in the phyla Firmicutes and Bacteroidetes compared to the other groups (4) (Figure 6 and Table 2). It is important to note that clinically, UHR individuals showed more severe symptoms and functional impairments on the Scale of Prodromal Symptoms for screening of schizophrenic symptoms and the Global Assessment of Function Scale, Modified Version, respectively, than the other two groups (4). Functional profile analysis using Phylogenetic Investigation of Communities by Reconstruction of Unobserved States (PICRUSt), which is a bioinformatics software for metagenomic functional predictions, predicted that short-chain fatty acids (SCFA) related to pyruvate synthesis, acetyl-CoA synthesis, and fatty acid biosynthesis initiation pathways were increased in UHR individuals compared to high-risk and controls, however only the acetyl-CoA synthesis pathway was significantly predicted (4) (Table 2). This profile corresponds with altered glucose metabolites, which is particularly interesting in the context of energy metabolism abnormalities that have been recently identified in schizophrenia [27-30].
Patients with first-episode psychosis, who received a relatively short antipsychotic treatment (median length of 20 days), showed no difference in alpha diversity (54) (Figure 6 and Table 2). Individuals with first-episode psychosis had enrichment in the phylum Actinobacteria. At class, order, family, genus and species levels the overall abundance of bacteria were altered in the phyla Firmicutes, Actinobacteria, Proteobacteria, “Deferribacteres,” Euryarchaeota, Cyanobacteria, and Chlorobi in patients with first-episode psychosis compared to healthy controls (54) (Figure 6 and Table 2). Physically active patients had a reduced abundance of Firmicutes at family level compared to active, healthy controls.
At baseline, multiple bacteria were decreased in drug naïve first-episode schizophrenia at species level belonging to the phyla Actinobacteria, Proteobacteria, and Firmicutes compared to controls (55) (Figure 6 and Table 2).
Individuals with chronic schizophrenia with over ten years of antipsychotic medication were investigated and compared to healthy controls (57). Gut microbiota samples did not differ in alpha diversity, microbial richness, and diversity, from healthy controls. However, they showed differential clustering of microbial communities of chronic schizophrenic patients compared to respective controls (beta diversity). Furthermore, healthy controls showed more similar bacterial communities, tighter clustering, than patients with schizophrenia (57) (Figure 6 and Table 2). At phylum level, Proteobacteria and Fusobacteria were significantly increased, and Firmicutes were less abundant in schizophrenia patients compared to controls. At class, order, family, genus, and species levels bacteria belonging to the phyla Proteobacteria, Fusobacteria, Firmicutes, Bacteroidetes, Actinobacteria, and Euryarchaeota were altered in chronic schizophrenia patients (57) (Figure 6 and Table 2). Furthermore, using PICRUSt analysis, functional pathways were identified to be altered in individuals with schizophrenia, such as pathways responsible for the synthesis of vitamin B6, fatty acid, starch, sucrose, tryptophan, cysteine, methionine, and linoleic acid metabolism and the degradation of some xenobiotics (57).
Similar to the aforementioned study (57), no changes in alpha diversity were found in chronic, medicated patients with schizophrenia and schizoaffective disorder (56). However, beta diversity showed a clear separation between the patient and control populations and showed a wider distribution of schizophrenia samples (56) (Figure 6 and Table 2). At phylum level, Proteobacteria were decreased in patients with schizophrenia. At genus level, bacterial abundance was altered bidirectional in the phyla Proteobacteria, Proteobacteria, and Firmicutes (56) (Figure 6 and Table 2).
Another recent study has gone beyond just determining the bacterial abundance in the microbiome in people with schizophrenia and evaluated if behavioral phenotypes could be transferred through fecal microbial transplant from patients with schizophrenia to germ-free mice (40). The gut microbiome of patients with schizophrenia had reduced alpha diversity compared to healthy controls, suggesting a lower within-sample diversity. Furthermore, beta-diversity was also significantly altered in schizophrenia patients (40) (Figure 6 and Table 2). Bacterial abundance was altered at family level belonging to the phyla Firmicutes, Bacteroidetes, Actinobacteria, and Proteobacteria (40) (Figure 6 and Table 2). Animals, which received elements of the gut microbiome from patients with schizophrenia via fecal microbial transplants, showed a behavioral phenotype that had some overlaps with schizophrenia-like behaviors, including locomotor hyperactivity, reduced anxiety, and decreases depression-like behavior, attributed to increased activity. However, no difference was found in cognitive behaviors and sociability. Mice, which received fecal matter from individuals with schizophrenia, showed an increased startle response at high-decibel tones; however, no difference was found in pre-pulse inhibition of startle, which has been used extensively as a translational behavioral biomarker of psychotic states. Investigators further verified that gut composition was altered through the fecal microbial transplant and found that the gut microbiome significantly differed compared to that of control mice. The most changed bacterial families were Aerococcaceae (Phylum: Firmicutes), and Rikenellaceae (Phylum: Bacteroidetes), which was similar to changes found in patients with schizophrenia [58].
Overall, the studies highlighted in this review demonstrated differential changes for all major phyla, including Proteobacteria, Firmicutes, Bacteroidetes, Actinobacteria, and Fusobacteria in patients with schizophrenia compared to healthy controls (4, 40, 54–57).
Discussion
Diversity of the Gut Microbiome in Schizophrenia
The diversity of the gut microbiome is unique to each individual. The majority of publications reviewed here identified no change in alpha diversity across animal and human studies (4, 46, 52–54, 56, 57). However, one study demonstrated an overall decrease in alpha diversity in individuals with chronic schizophrenia (40). The majority of patients were medicated with antipsychotics, which may have resulted in reduced microbial community diversity (40), as treatment with an atypical antipsychotic reduced microbiome community diversity in patients with bipolar disorder (alpha diversity), which was more profound in females (61). On the contrary, individuals on antipsychotic medications had no change in microbial community diversity (57). Due to conflicting results, we can only speculate that antipsychotic treatment may have resulted in a decrease in alpha diversity. Therefore, future studies should investigate the potential impact of antipsychotic treatment on the gut microbiome in schizophrenia.
The gut microbiome of patients with psychosis and animal models of schizophrenia are separated from the microbiota of healthy control individuals and control animals (beta diversity) (4, 40, 52–54, 56, 57). This clearly demonstrates that the gut microbiome in psychosis differs from that of the healthy controls. However, as there is a lack of studies comparing the microbiome between psychosis and other psychiatric and chronic, noncommunicable disorders, this does not necessary mean that the microbiome profile identified in psychosis is diagnostically specific. Nevertheless, these results, together with data showing that high-risk and UHR individuals have an altered microbiome compared to that of healthy controls (4), raise the possibility of developing the gut microbiome profiling further to be used as part of a biomarker battery to identify individuals at risk for later development of psychosis.
The studies outlined in this systematic review revealed that the phyla Proteobacteria (57), Firmicutes (52, 57), Bacteroidetes (52, 57) Fusobacteria (57), and Actinobacteria (46, 54, 57) were altered in patients at risk to develop schizophrenia, with chronic schizophrenia or in animal models of the disease at all different taxonomic units (Figure 6). First, we will individually discuss each of these phyla, within each phyla section we are discussing lower taxonomic units, and consider the functional implications that might be associated with their change.
Proteobacteria
Altered abundance, in both directions, of Proteobacteria has been associated with obesity, inflammation, and altered gut permeability (62–65). Proteobacteria were increased (57), as well decreased (56) in patients with chronic schizophrenia (Figure 6 and Table 2) in the gut microbiome, but not in the oropharynx microbiome (66), which suggests that the imbalance of Proteobacteria abundance are specific to the gut microbiome. While an increased abundance of Proteobacteria is associated with the neonatal period (67), gastric bypass surgery (62, 68), and disease states such as metabolic disorders (69) and intestinal inflammation (70), decreased abundance of Proteobacteria have been found in overweight individuals (62). One study identified no difference in body mass index (57), whereas, in another study, individuals with schizophrenia had a significantly higher body mass index compared to healthy controls (56) (Table 2). Weight may have been a contributing factor influencing the abundance of Proteobacteria. Possibly, the decrease in Proteobacteria is more representative of patients with psychosis, because they tend to be more overweight or obese compared to mentally healthy controls (71). Therefore, future studies need to include lifestyle factors, such as diet, as potential covariates in the data analysis. At family level, both increased (57) and decreased (40) abundance of Enterobacteriaceae have been found (Figure 6 and Table 2). An increase in Enterobacteriaceae have been associated with obesity (63), inflammation (64) and a “leaky gut” (65); however, obesity could not have been the driver for the change of this bacterial family as individuals with schizophrenia in both studies were in the healthy weight range based on their body mass index (40, 57). Increased gut permeability has been suggested to be related to schizophrenia (72) and may have been a contributing factor for the increase in Enterobacteriaceae (57). Besides, patients with schizophrenia show higher levels of proinflammatory cytokines (73). It is unclear, however, if the increased abundance of Enterobacteriaceae (57) was driven by proinflammatory cytokines or increased gut permeability in schizophrenia.
Firmicutes
An increased abundance of Firmicutes has been associated with an unhealthy dietary pattern, such as western diet and obesity (5, 74). A higher abundance of Firmicutes was found in the oropharynx microbiome of patients with schizophrenia (66). Animals injected with phencyclidine, a pharmacological model of schizophrenia, showed an increase in Firmicutes in their gut microbiome (74). In contrast, Firmicutes were less abundant in patients with chronic schizophrenia (57) (Figure 6 and Table 2). At class, order and family levels Negativicutes, Selenomondales, and Veillonellaceae were reduced in first-episode psychosis (54) but were increased in an animal model of social isolation (46). Veillonellaceae were also increased in chronic schizophrenia (40, 57) (Figure 6 and Table 2). Patients with chronic schizophrenia showed both an increase and a decrease in the genus Clostridium (56) in different studies (Figure 6 and Table 2). Interestingly, an increase in this genus is associated with risperidone treatment (75). At this point, we are unable to conclude about the potential role of risperidone treatment as both studies (56, 57) report antipsychotic use, without specifying the type of medication used. Several species of Clostridium are precursors of 4-ethylphenylsulfate (76), which may contribute to the pathophysiology of schizophrenia, as it is important in pheromonal communication in mice under the control of testosterone levels (77). Serum 4-ethylphenylsulfate was elevated in MIA animals, a neurodevelopmental model of schizophrenia and 4-ethylphenylsulfate induced an anxiety-like behavioral phenotype, which may suggest behavioral abnormalities may be related to 4-ethylphenylsulfate produced by Clostridium (53). At species level, first-episode psychosis (54) and chronic schizophrenia patients (57), show similar to a study in oropharyngeal samples of schizophrenia patients, a significantly increased abundance of Lactobacillus phage phiadh (78) (Figure 6 and Table 2).
Bacteroidetes
Bacteroidetes were increased in the pharmacological model of schizophrenia (52) and patients with chronic schizophrenia (57) (Figure 6 and Table 2). Stress is involved in the pathophysiology of psychotic disorders, such as schizophrenia (79). In a mouse model of stress, mice were subjected to an aggressive male mouse within their home cage to induce stress. Investigators found elevated levels of the genus Bacteroides (80), which was demonstrated in chronic schizophrenia (57) (Figure 6 and Table 2). Treatment with the probiotic Bacteroides fragilis was shown to improve anxiety-like, repetitive and communicative behaviors, and sensorimotor gating in the MIA model (53) (Table 2). Bacteroides fragilis improved anxiety-like (57), repetitive (57) and communicative behaviors (57), sensorimotor gating (57); however, Bacteroides fragilis do not influence social behaviors (53) (Figure 6 and Table 2). Depressive-like symptoms in chronic schizophrenia patients were associated with an increased abundance of Bacteroides (56).
Actinobacteria
Actinobacteria are increased in first-episode psychosis, chronic schizophrenia, and socially isolated animals (46, 54, 57) (Figure 6 and Table 2). Tropheryma, which belong to the phyla Actinobacteria, most studied species is Tropheryma whipplei, has been associated with intestinal malabsorption (81). Tropheryma whipplei was significantly increased in patients with first-episode psychosis (54) (Figure 6 and Table 2). At genus level, Collinsella was elevated, which produces proinflammatory cytokines such as interleukin-17a and altered intestinal permeability in arthritis (82). Patients with schizophrenia show alter gut permeability (83). Additionally, multiple studies have found increases IL-17a plasma concentrations in naïve first-episode schizophrenia patients (84, 85).
In conclusion, the published literature indicates that schizophrenia, both first-episode and chronic, is associated with microbiota changes, as shown by beta diversity, that will distinguish them from healthy controls. In this systematic review we included studies in which healthy controls were compared to either individuals at risk to develop psychosis or with diagnosed psychosis (either first episode or chronic). Such cross-sectional design, together with a variety of cofounding factors, which were controlled for in some, but not in all cases, precludes us from concluding about causality. However, our review of the available human literature clearly indicates the existence of an association between different stages of psychosis and the gut microbiome. Future studies will be required to identify the primary drivers of the microbiome alterations in psychosis and the potential direction of causality between gut microbiome changes in psychosis.
Methodological Considerations
Cofounding Factors
Within the reviewed publications gender was not addressed as a potential cofounding varible, which can potentially affect the gut microbiome. A review investigated gender differences in the gut microbiome and concluded that gender effects are inconsistent and identified that differences in geography, life style, diet, age, genetics, and potential other factors contribute more extensively to alterations in the gut microbiome (86). Within the reviewed articles, we reviewed populations from different geographical locations, which may have contributed to observed differences in microbiome. However, all studies demonstrated a clear separation between matched controls and psychotic individuals controlling for geographical location. Another major cofounding variable is age. We observed a broad age range in the reviewed studies, which may be expected considering that we included studies reporting on at-risk, UHR as well as on chronic schizophrenia. It has been suggested that age might be a major contributor to alterations in the gut microbiome (87). Gut microbiome changes within the elderly are associated with physiological changes within the gastrointestinal tract and has been demonstrated to reduce over time in diversity, shifts in dominant species, a decline in beneficial bacteria and decreased availability of beneficial metabolites such as short chain fatty acids (88). Older individuals have lower levels of Firmicutes and increased abundance of Proteobacteria (88). It is difficult to establish the potential cofounding effect of age on the results presented in the review. It should be noted, however, that each study contained age-matched controls, just like in the case of geographical location.
Fecal Sample Methodology Cofounders
We demonstrated that the CoR was greater in human studies allowing for greater comparability between studies. However, animal studies lacked reporting and should therefore be interpretate carefully. Overall, alterations and lack of reporting can be potential cofounding factors [further extensive review elsewhere (42)]. Methodological difference should be standardised in the future to improve translatability between animal and human studies and would allow for improved interpretation of data.
Factors Potentially Contributing to the Altered Microbiome in Psychosis
In the following section we address potential factors influencing the gut microbiome in individuals with psychosis. Due to the heterogeneous nature of psychotic disorders no specific and unique factors to leading to psychosis are known. Therefore, we considered common life-style and environmental factors, genetic susceptibility and medication use that can potentially influence the gut microbiome in psychosis, as well as in other serious mental illness.
Environmental Factors
Early Life Events
Children will receive the first inoculum from their mothers (89). Mode of delivery can influence the gut microbiome (37). Offspring will receive during vaginal birth microbes found in the maternal vagina and feces (89). Whereas, during Cesarean delivery (C-section), most microbes colonizing the gut are from external body surfaces (89). C-section significantly decreases Bifidobacteria spp. (90) and increases Staphylococcus, Streptococcus or Propionibacteria (91, 92). However, the differences in microbiota between C-section and vaginal birth gradually disappear over time (37). Preterm birth will result in an increased abundance of Proteobacteria and a lack of Bifidobacterium and Lactobacillus at genus level (37). It has been established that early life events are potential risk factors for schizophrenia (93, 94). C-section and preterm birth have been linked as a risk factor for schizophrenia (93, 94). Bifidobacterium spp., which is in line with the microbial profile of C-section and preterm birth, were decreased in first-episode psychosis patients (55) (Figure 6 and Table 2). Chronic schizophrenia patients had increased levels of Proteobacteria at phylum level, which is related to preterm birth (57) (Figure 6 and Table 2). In first-episode psychosis, UHR and chronic patients with schizophrenia Lactobacillus, which is again related to preterm birth, was increased (4, 54, 57) (Figure 6 and Table 2).
These early life events seem to alter the gut microbiome and may influence the development of psychosis later in life, perhaps through brain development influenced by the microbiome. Future research should specifically investigate if the changes in the microbiome due to the aforementioned factors contribute to the development of psychosis.
Stress
It has long been established that stress and the activity of the hypothalamus-pituitary-adrenal (HPA) axis can alter the composition of the gut microbiome (39). Maternal separation, a model of stress, results in prolonged HPA activity, which resulted in rhesus monkeys (95), rats (96) in altered microbiome composition (95). Chronic restraint stress in adult mice resulted in differential gut microbiota composition compared to nonstressed mice (80). Stress decreased Bacteroides spp. and increased Clostridium spp., which was accompanied by an activation of the immune system and a “leaky” gut (97), allowing for the translocation of luminal content such as lipopolysaccharides.
Stress is involved in the development of psychotic disorders such as schizophrenia (79, 98). Life events perceived as stressful can increase the occurrence of psychotic episodes (99). Individuals with schizophrenia experience stress more intensely; therefore even minor everyday stressors might exacerbate positive symptoms (100, 101). Management of day-to-day stress can be used in the management of psychosis (102); however, this intervention, in combination with antipsychotics, is only partially protective (103). This hypersensitivity to stress might be attributed to inappropriate autonomic nervous system and HPA axis function (79). Psychosis itself is a stressful event for the body, activating the stress response (98). Hypercortisolemia has been shown in patients with schizophrenia (104), which has been linked to the negative symptoms of schizophrenia (79). However, increased cortisol levels are not consistently found in individuals with schizophrenia (105). A meta-analysis found dysregulation of cortisol in psychotic patients (106). Allostatic load is the adaptation in response to stimuli such as stress (98). An increased allostatic load was seen in first-episode psychosis and schizophrenia patients compared to controls (98). This study found a positive correlation between positive symptoms with allostatic load in schizophrenia patients (98).
The genus Clostridium was increased in animals treated with phencyclidine (52) and chronic schizophrenia patients (57); however, Clostridium also decreased in chronic schizophrenia patients (56) (Figure 6 and Table 2), consistent with a stress response. However, the stress level of schizophrenia patients was not described, and therefore it is unknown if the microbiota was altered due to increased stress or visa versa (57). A decrease in Bacteroides spp. is associated with stress (97), while in chronic schizophrenic patients, this genus was decreased (57) (Figure 6 and Table 2).
In conclusion, based on the limited data available, it is difficult to establish if stress altered the microbiome. Future studies should assess stress levels and allostatic load to understand the impact of stress in psychosis on the gut microbiome.
Infectious Agents
Infectious agents, such as Toxoplasma gondii, have been suggested to contribute to the development of schizophrenia (107, 108). It has been recently demonstrated in a preclinical study that chronic Toxoplasma gondii infection results in an enrichment of Bacteroidetes in CD1 mice compared to noninfected controls (109). Interestingly, Bacteroidetes were also increased in a pharmacological model of schizophrenia (52) and in individuals with chronic schizophrenia (57). However, on the basis of the available evidence it is not possible to conclude about causality of the link between Toxoplasma gondii infection, alteration in the gut microbiome and the development of schizophrenia.
Lifestyle Factors
Diet
Diet is shaping the composition of the gut microbiome (5). The gut microbiome, in turn, is important in metabolizing the ingredients of food (18) and host fat storage, through the absorption of monosaccharides by the gut microbiota from the lumen of the gut, promoting hepatic lipogenesis by fasting-induced adipocyte factor suppression (110). Multiple studies have demonstrated that altering diet rapidly changes the gut microbiome (5, 111) and microbial beta-diversity (112). Early life nutrition, through changing the gut microbiome, is important in the infant’s development (113). Different diets in adulthood have been shown to modulate the gut microbiome, such as a high-protein, reduced carbohydrate diet (114), ketogenic diet (115), high fat, high sugar diet (western diet) (116, 117), and mediterranean diet (118). This indicates the strong impact of diet on the gut microbiome.
Nutrition is an important factor in schizophrenia due to poor dietary choices, causing obesity and secondary diseases (119). Obesity is twice as likely in schizophrenia/psychosis compared to the general population, affecting more than 50% of schizophrenic individuals (120). Drug naïve patients with schizophrenia show higher rates of obesity and type II diabetes compared to healthy individuals (120). Patients with schizophrenia consumed more fat (121, 122), saturated fat (122, 123), proteins (122), carbohydrates (122) and less fiber (121), than healthy controls. However, it was found that similar choices were made, but the overall food consumption was increased compared to healthy controls (122). Overall, people with psychosis tend to prefer unhealthy, fast food-type foods (120). This results in a dietary pattern high in saturated fats and sugars (“Western” diet). However, the gut microbiota of schizophrenia patients did not reflect that of individuals on a Western diet, characterized by a decrease in Bifidobacteria, Bacteroides, and Prevotella and an increase in Firmicutes (5). Within this review, first-episode psychosis had an increased abundance in Bifidobacteria (54) (Figure 6 and Table 2). Different food types were not significantly associated with clustering of the microbiota (54). Increased Prevotella were found in UHR individuals (4); however, no conclusion can be drawn on the impact of nutrition as no metabolic or nutritional assessments were reported. In chronic schizophrenia patients, Firmicutes decreased, and the abundance of Bacteroides and Prevotella increased (57). It has to be noted that individuals in that study were Chinese patients, who did not show metabolic symptoms commonly seen in schizophrenia. This lack of weight gain has previously been reported in other Chinese schizophrenia patients (124). One study predicted an altered glucose metabolism (4), which is particularly interesting in the context of energy metabolism abnormalities that have been recently identified in schizophrenia [27-30]. A review investigated the link between the gut microbiome and glucose metabolism and found that the gut microbiome has substantial influence on glucose homeostasis through short chain fatty acids, bile acid metabolism, hormone secretion and synthesis of amino acids (125). However, future studies should address this point in individuals with psychosis to better understand the relationship between the gut, brain and energy metabolism.
In summary, individuals with psychosis show unhealthy dietary choices, which may influence the gut microbiome. However, the microbiome profiles described in studies on patients with psychosis do not support this notion. Nevertheless, at this point we can neither prove nor disprove the influence of diet to influence the gut microbiome in psychosis due to conflicting evidence and lack of reporting of dietary habits in these studies. Future studies should incorporate dietary patterns to be able to make a more definitive conclusion on the effects of dietary factors on the gut microbiome in individuals with psychosis.
Exercise
Exercise can impact microbial abundance in animals (126) and the human gut (127–131). The gut microbiota is different between sedentary individuals and people performing physical exercise (129, 132). Exercise reduced Bacteroidetes (129, 130) and Proteobacteria (132). Activity increased the undefined genus in the S24-7 family (129), Verrucomicrobia (132), Bifidobacteriaceae (132), the Streptococcaceae family (129) and Firmicutes (130), compared to sedentary controls. In sedentary woman bacteria belonging to the families, Barnesiellaceae and Odoribacteraceae were more abundant compared to active women (130).
Individuals with schizophrenia and other psychotic disorders are significantly less physically active than healthy individuals, and are also less active then patients with other psychotic disorder (133, 134). Patients with chronic schizophrenia show an increased abundance in Bacteroidetes and Proteobacteria and a decreased abundance in Streptococcaceae and Firmicutes (57) (Figure 6 and Table 2), which could possibly be mitigated by exercise as active individuals have opposing abundances (129, 130, 132). Further supporting this argument, Firmicutes were increased in phencyclidine treated animals (52), which could have been related to hyperactivity induced by the phencyclidine administration. The increase in the phylum Bacteroidetes is associated with a sedentary lifestyle (130). Bacteroidetes were increased in chronic schizophrenia patients (57) (Figure 6 and Table 2); however, this increase was as well seen in the phencyclidine, hyperactive animals (52) (Figure 6 and Table 2). Therefore, it is unclear at this stage if physical activity altered the gut microbiome. The activities of patients with schizophrenia are not explicit in the publications reviewed here. Nevertheless, physical activity has been considered to influence the gut microbiome composition in the context of schizophrenia Schwarz, Maukonen (54). Pateints with first-episode psychosis, stratified for amount of exercise, demonstrated an increased abundance of Lactobacillaceae and decreased abundance of Veillonellaceae at family level in physically active, first-episode psychosis individuals compared to physically active, healthy controls (54) (Figure 6 and Table 2). Future studies need to assess physical activity levels as a potential cofounder to influence the gut microbiome.
Smoking
Environmental contaminants, such as smoking, influence the gut microbiota (135). Furthermore, smoking can lead to DNA damage and epithelial cell methylation (136), resulting in altered gut function and possibly altered microbiota composition. Smoking increased within-participant diversity, Dialister invisus, and Megaspaera micronuciformis were more abundant in the upper gastrointestinal tract in current smokers compared to the ones who never smoked (135). In rats, cigarette smoke decreased Bifidobacteria and SCFA, such as propionic and butyric acid (137), and increased Lachnospiraceae spp. (138). Passive smoking increases Clostridium spp. and reduces Firmicutes and Enterobacteriaceae in animals (139). In humans smoking increased Clostridium (140), Bacteroidetes, and Proteobacteria (141) and decreased Firmicutes and Actinobacteria (141).
Smoking is more prevalent in individuals with schizophrenia than in healthy individuals (142).
As reviewed above, in first-episode psychosis, Bifidobacteria were increased (54) (Figure 6 and Table 2), whereas smoking decreased this bacteria. However, this study did not specify smoking status (54). In human studies, Lachnospiraceae (57) and Firmicutes (57) were decreased (Figure 6 and Table 2). Decreased Firmicutes are in line with smoking; however, in patients with chronic schizophrenia, tobacco usage was not different compared to healthy control (57). At genus level contradictory results were found for Clostridium, however as mentioned before an increase in Clostridium, which is associated with smoking, was seen both in an animal model of schizophrenia (52) and in patients with chronic schizophrenia (57), where no difference in tobacco usage was seen between patients and controls. On the contrary, patients with schizophrenia, who were significantly likelier to smoke, had decreased abundance of Clostridium (56) (Figure 6 and Table 2). Enterobacteriaceae were increased in chronic schizophrenia patients (41) (Figure 6 and Table 2), which would be in line with possible tobacco usage; however, smoking status was not reported within that study.
In conclusion, according to the studies reviewed here individuals with schizophrenia either did not smoke more than the general population, or tobacco usage was not reported, except Nguyen, Kosciolek (56) where contradictory results were found with regards to smoking and the gut microbiome. Considering animal models of psychosis that do show altered gut microbiome despite the lack of smoking exposure, one can argue that the change in the gut microbiome seen in individuals with psychosis is likely to be independent of smoking status. However, most studies did not report smoking status, which predicts a firm conclusion regarding the link between smoking status and altered gut microbiome at this stage. Future studies should report smoking status and investigate if tobacco usage might be a cofounding factor influencing the gut microbiome.
Overall, although it has been widely acknowledged that life style-factors are essential in shaping the gut microbiome, the studies covered in this systematic review do not support the notion that the difference in the gut microbiome between controls and individuals with psychosis is causally related to lifestyle factors. Potentially, lifestyle factors, such as diet, exercise, and smoking may improve the gut microbiota of individuals with psychosis. Therefore, the completeness of reporting to provide a detailed account of the lifestyle factors is of great importance for future studies.
Genetics
Host genetic variation can influence the diversity of the gut microbiome (143). However, the relationship between host genetics and gut microbiota is largely unknown (143). Although, lifestyle factors such as diet and exercise will contribute to similar gut microbiota composition of close relatives, suggesting that genetics might be an important factor (143). For example, monozygotic twins share a more similar gut microbiota profile than dizygotic twins (143).
Genetic factors are important in the etiology of psychotic disorders such as schizophrenia (144). At this stage, no studies have investigated the effect of host genetic variation in individuals with schizophrenia on the gut microbiota. Therefore, we can only speculate that variations of bacterial abundances found in this population may be due to genetic variation involved in the pathogenesis of psychosis. Future studies should incorporate genetic analysis to understand the importance of host genetic variation on the gut microbiome.
Antipsychotics
Antipsychotics are the primary medications used for the management of schizophrenia (145). However, the knowledge of the effects of antipsychotics on the gut microbiome is currently in its infancy. Antipsychotic use can cause severe metabolic side effects such as weight gain, increased visceral fat, and glucose dysregulation (146), of which the mechanism of action is not fully understood (146). It is believed that the convergence of central and peripheral mechanisms are involved in metabolic side effects (146). It has been demonstrated that the composition of the gut microbiome is linked to obesity (147). Olanzapine treatment increases body weight (146, 148) and leads to a shift of the gut microbiome, which involves the increase of the phylum Firmicutes (146, 148) and decreases in the phyla Bacteriodetes (146, 148) in rodents. In female rats, olanzapine reduced the abundance of Actinobacteria and Proteobacteria compared to controls (148). Another study found an increase in Erysipelotrichia and Gammaproteobacteria and a reduced abundance of Bacteroidia at class level (149). Olanzapine inhibited the growth of Escherichia coli NC101 (149). One study found no change in microbial composition after olanzapine treatment, which may have been due to the short duration of treatment (150). However, this study demonstrated that acetate concentration changed, suggesting that olanzapine did affect on this by-product of the microbiome function (150). Risperidone increased Firmicutes, where Lactobacillus spp. were reduced, and decreased Bacteroidetes, where Bacterioides spp. were increased and Alistipes spp. decreased and Proteobacteria in female C57BL/6J mice compared to controls (151). The most abundant genera were Allabaculum spp. in risperidone treated animals compared to controls (151). Risperidone treatment resulted in weight gain (151). Transplant of fecal matter of female C57BL/6J mice on risperidone treatment to naïve mice resulted in a decreased resting metabolic rate, which may have contributed to the increase in body weight (151). Donor fecal matter was analyzed for risperidone concentration, which was 10-fold less than to establish a dose-response curve (151). Therefore, investigators concluded that the microbiota of risperidone treated animals was the obesogenic factor rather than the remaining risperidone within donor fecal samples (151). In medically healthy males, risperidone treatment led to weight gain with an altered gut microbiome compared to psychiatrically ill, but untreated patients. These findings with risperidone are similar to the gut microbiota changes after olanzapine administration. Antipsychotic treatment increased the abundance of Lachnospiraceae and decreased the abundance of Akkermansia after adjustment for body mass index in patients with bipolar disorder (61). However, Akkermansia was less abundant in nonobese, antipsychotic-treated patients (61). One publication covered in this systematic review assessed the changes in first-episode schizophrenia after 24-weeks of risperidone treatment (55). Chronic risperidone treatment altered metabolic parameters such as an increase in weight, body mass index, fasting serum glucose levels, triglycerides, and low-density lipoproteins (Table 2). Risperidone treatment increased Bifidobacterium spp. and Escherichia coli and decrease the abundance of Clostridium coccoides and Lactobacillus spp. (55) (Figure 6 and Table 2).
Oxytocin
As the ever evolving literature recognizes the need for new drug treatments to complement the presently used antipsychotic medication, the neuropeptide oxytocin has been suggested as a potential novel treatment approach (152). Evidence from preclinical and clinical studies suggest therapeutic effects on all symptom domains of schizophrenia, with particular improvement in the negative symptoms (152). A potential link with the microbiome is suggested by the finding that the bacterium Lactobacillus reuteri upregulates oxytocin (153). One study we presented in this systematic review in first episode psychosis patients showed an increased abundance of Lactobacillus reuteri (54). However, the exact details of the interaction between oxytocin and the microbiome are currently unknown.
In our systematic review, six articles assessed different stages of the development of schizophrenia, such as high-risk, UHR, first-episode psychosis, first-episode schizophrenia, chronic schizophrenia. High-risk, UHR, and first-episode schizophrenia, first-episode psychosis patients were at study onset drug naïve (4, 54, 55). For chronic patients, antipsychotic treatment had to be more than six months of use. Therefore, changes might be cofounded by antipsychotic treatment (40, 56, 57). More studies are needed to identify the influence of antipsychotic medication on the gut microbiome.
Functional Implication of the Change in Microbiota on the Psychopathology of Psychosis
Symptoms of Psychosis and the Gut Microbiome
Of the publications reviewed here, some have linked the gut microbiome to symptoms seen in schizophrenia. In the pharmacological model of schizophrenia, hyperactivity was associated with an increase in Lachnospiraceae and Clostridiaceae and at genus level an increase of Roseburia, Clostridium, and Odoribacter (52) (Figure 6 and Table 2). In socially isolated animals, activity was positively correlated with the abundance of Bacillales. On the other hand, Clostridales was negatively correlated with locomotor activity (46). Socially isolated animals had increased locomotor activity in conjunction with reduced Clostridales (46). Furthermore, Clostridiales was negatively correlated with cognitive performance (46). Additionally, taxa belonging to the order Clostridales, at family level Ruminococcaceae and genus level Papillibacter were positively correlated to anxiety-like behaviors (46). Bacillales were negatively correlated to anxiety-like behaviors. Impaired contextual fear task, which investigates the associative learning process, was associated with an increase in Veillonella and Defluvitaleaceae UCG-011 (46).
In people with schizophrenia, negative symptoms were related to decreased Ruminococcaceae, and self-reported mental well-being was positively correlated with the phylum Verrucomicrobia (56). Correlation analysis revealed that age of disease onset positively correlated with Cyanobacteria at phylum level, indicating that the earlier the disease onset, the higher the abundance of Cyanobacteria. Depressive-like symptoms were associated with an increased abundance of Bacteroides. Veillonellaceae OTU191 were negatively correlated with the Positive and Negative Syndrome Scale (40). On the other hand, Bacteroidaceae OTU172, Streptococcaceae OTU834, and two Lachnospiraceae OTUs (477 and 629) were positively correlated with PANSS (40). Bacterial numbers of Lactobacillus group, Lachnospiraceae, Ruminococcaceae, and Bacteroides spp. were correlated with symptom severity, particularly for negative symptoms and poorer functioning (54). Positive symptoms were correlated with bacteria of the Lactobacillus group (54).
Systemic biochemical changes and their relationship with the gut microbiome have been investigated. It was identified that Blautia, Coprococcus, and Roseburia were negatively associated with vitamin B6, taurine, and hypotaurine metabolic pathway and positively associated with methane metabolic pathways (57). Increased inflammatory cytokines have been seen in patients with schizophrenia (73). Hippocampal IL-10 and IL-6 correlated with Peptostreptococcaceae positively (46), while Bacillales correlated negatively with hippocampal IL-6 (46, 54). Interestingly, if the microbial composition of first-episode psychosis clustered with controls, these patients were more likely to show remission (70% of patients) (54). However, patients with abnormal microbial composition at baseline showed low remission rates (28% of patients) (54). Analysis revealed that this remission was not due to symptom severity at baseline (54). Clustering was not influenced by physical activity, body mass index, type of psychosis, duration of antipsychotic treatment, and the food consumed one week before fecal sample collection (54).
In summary, the abundance of specific bacteria correlated with behaviors and biochemical changes. This raises the possibility of targeted treatment approaches, such as pre/probiotics, to alleviate individual symptoms, however at this point in time causation has not been established. Further studies are required to link individual behaviors and biochemical changes in psychosis to the gut microbiome.
Does the Microbiome Have a Pathogenic Role in Psychosis?
To address this question, we investigated retrospective studies to see if antibiotic use increased the prevalence of psychosis. A nationwide, register-based cohort study in Denmark outlined that antibiotics increased the risk of mental health disorders, which was independent of age, compared to antivirals and antimycotics (154). The risk for mental disorders increased in a dose-dependent manner, were a risk for mental disorders were more likely in individuals with more treated infections (154). The study showed that anti-infective agents were associated with an increased risk for schizophrenia spectrum disorders (154). Another study investigating the same Danish cohort identified that maternal infection during pregnancy treated with anti-infective agents increased the risk of mental disorders in the offspring, compared to offspring without maternal infection with anti-infective agents during pregnancy (155). The risk of mental health was increased if maternal infection treated with anti-infective medications occurred during the second and third trimester (155). It can be argued that maternal infection during pregnancy is known to be a risk factor to develop schizophrenia (156). However, Lydholm, Kohler-Forsberg (155) demonstrated that mental health disorder risk increased in response to maternal prescriptions during and after pregnancy. These recent studies suggest that antibiotic treatment during pregnancy and later in life may result in the later onset of schizophrenia. However, these studies are merely observational and would need further research to create causality.
Interestingly, animals, which received gut microbiome from patients with schizophrenia via fecal microbial transplant, showed a schizophrenia-like behavioral phenotype (40). Investigators further verified that gut composition was altered through the fecal microbial transplant and found that the gut microbiome significantly differed compared to that of control mice and was similar to that of patients with schizophrenia (40). Furthermore, the gut microbiota was altered in UHR individuals (4). Therefore, the changes in gut microbiota composition may contribute to the development of the disease pathophysiology. If confirmed, this raises the possibility to utilize the gut microbiome as an early biomarker for schizophrenia spectrum disorders.
Further research should be directed toward the understanding of bacteriophages, viruses that infect bacteria leading to the death of the bacteria or integration of the phage into the gut microbiome (78). Interestingly, a significantly increased abundance of Lactobacillus phage phiadh has been identified at species level in first-episode psychosis (54) and in patients with chronic schizophrenia (57), as well as in the oropharyngeal microbiome samples of patients with schizophrenia (78) (Figure 6 and Table 2). However, the importance of bacteriophages is currently unknown and will require further investigation.
If alterations in the gut microbiome do indeed play a pathogenetic role in psychosis, agents, which modify the gut microbiome, can be considered as a potential modifiers of the disease process in schizophrenia. No research to date has investigated the effects of pre-or probiotics, antibiotics, fecal microbial transplant, or dietary intervention on the gut microbiome, specifically in people with schizophrenia. Bacteroides fragilis, a probiotic, in MIA model of schizophrenia in mice resulted in the restoration of gut permeability, gene expression, and IL-6 in the colon and normalized at family level Lachnospiraceae and unclassified Bacteroidales (53). Behaviorally, Bacteroides fragilis improved anxiety-like, sensorimotor, repetitive and communicative behavior; however, Bacteroides fragilis treatment did not affect sociability and social preference in MIA mice (53). Similar behavioral effects were found with Bacteroides thetaiotaomicron, whereas Enterococcus faecalis did not improve anxiety-like and repetitive behavior (53). Therefore, probiotic treatment might be a novel treatment for schizophrenia by improving gut functioning. However, a current review comes to the conclusion that the most recent evidence does not yet support the use of probiotics for the treatment of psychiatric disorders and more research is needed (157). Fecal microbial transplant, first performed approximately 1700 years ago (158), has not yet been described in either animal models or individuals with schizophrenia, but has been trialed for multiple sclerosis, patients showing normalization of neurological symptoms (159). These promising results may open up a new area in which the therapeutic effects of the microbiome can be taken advantage of. However, clinical trails are needed as currently only speculations can be made.
The antibiotic minocycline, which exerts neuroprotective and anti-inflammatory actions through supressing microglia activation and the modulation of excitatory neurotransmission, have attracted attention as potential treatments for schizophrenia, as shown by a number of small pilot studies with encouraging results (160). Although, a recent, large, randomized, placebo controlled clinical trial has provided unequivocal results showing no measurable therapeutic benefit on negative and other symptoms in patients with schizophrenia spectrum disorders (161), it has to be emphasized that active neuroinflammation involving microglial activation and neuropathology was unlikely to be present during the first years of schizophrenia, potentially explaining the lack of effect. The involvement of the gut microbiome in the mediation of the effects of minocycline has not been investigated.
Lastly, it is feasible to assume that diet could shift and normalize the gut microbiome in individuals with psychosis. The high fat, low carbohydrate ketogenic diet modifies the gut microbiome in an animal model of autism (115). Ketogenic diet increased Enterobacteriaceae and decreased Firmicutes, Lactobacillus spp., and Roseburia (115), which were altered in schizophrenia (Figure 6 and Table 2). We have recently reported that ketogenic diet improves schizophrenia-like behaviors in an animal model of schizophrenia (162, 163), which can be due to the diet-induced alterations of the gut microbiome.
Conclusion
Overall, the studies covered by this systematic review demonstrate that the gut microbiome in patients with schizophrenia spectrum disorders and animal models of schizophrenia is different from the microbiome of healthy controls. Once these initial findings are replicated and further extended in different patient populations the changes in the microbiome might be used as an independent biomarker of psychosis for high-risk and UHR individuals. While lifestyle factors do shape the gut microbiome, it is currently uncertain how they contribute to psychosis. Lifestyle changes such as diet, exercise, and cessation of smoking may influence the gut microbiome positively in individuals with schizophrenia. Stress and other early life events are possible further environmental factors contributing to this change. Multiple pathways by which alterations in the gut microbiome may have occurred, such as inflammation, the vagus nerve communication, stress response, and metabolites produced by the microbiota have been reviewed elsewhere (1, 5, 22). Clearly, more research is needed to clarify the role of specific host extrinsic and intrinsic factors and to identify specific mechanistic links, if any, between the gut microbiota and psychosis. Animal and human studies showed both similarities and differences in gut microbiota composition, which reflect the well-known difficulties to translate between preclinical and clinical research in the area of psychosis. Greater understanding and reporting of methodology of the gut microbiome will improve translatability from murine to human studies.
We conclude that the gut microbiome changes may precede the appearance of the diagnostic clinical symptoms of schizophrenia spectrum disorders and may contribute to the disease pathophysiology and the development of the behavioral symptoms. Further rigorous, well reported preclinical and longitudinal, mechanistically oriented clinical studies are needed to provide more evidence to support these potential links. Normalizing the altered gut microbiome with diet, pre- or probiotics, fecal microbiome transfer, or pharmacological interventions, may lead to improved symptom control and mitigation of the metabolic side effects of antipsychotic medication. However, randomized controlled clinical trials are urgently required to substantiate the potential use of targeting the microbiome as a novel therapeutic intervention in psychotic disorders.
Data Availability Statement
The datasets used and/or analyzed during the current study are available from the corresponding author on reasonable request.
Author Contributions
A-KK performed the data search, report selection, data extraction, and quality assessment and wrote the manuscript. RP was the independent assessor for the quality assessment and revised the final draft of the manuscript. ZS edited and revised all drafts of the manuscript.
Funding
A James Cook University (JCU) Postgraduate Research Scholarship and a Higher Degree Research Enhancement Scheme from JCU supported A-KK and RP.
Conflict of Interest
The authors declare that the research was conducted in the absence of any commercial or financial relationships that could be construed as a potential conflict of interest.
Abbreviations
C-section, Cesarean delivery; CoR, completeness of reporting; GABA, gamma-aminobutyric acid; HPA, hypothalamic pituitary adrenal; MIA, maternal immune activation; NMDA, N-methyl-D-aspartate; OTU, operational taxonomic units; PCoA, principal coordinate analysis; PICRUSt, Phylogenetic Investigation of Communities by Reconstruction of Unobserved States; PRISM-P, Preferred Items for Reporting Systematic Reviews and Meta-analysis Protocols; RoB, risk of bias; SCFA, short-chain fatty acid; TNF, tumor necrosis factor.
References
1. Cryan JF, Dinan TG. Mind-altering microorganisms: the impact of the gut microbiota on brain and behaviour. Nat Rev Neurosci (2012) 13(10):701–12. doi: 10.1038/nrn3346
2. Schmidt TSB, Raes J, Bork P. The human gut microbiome: from association to modulation. Cell (2018) 172(6):1198–215. doi: 10.1016/j.cell.2018.02.044
3. Clemente JC, Ursell LK, Parfrey LW, Knight R. The impact of the gut microbiota on human health: an integrative view. Cell (2012) 148(6):1258–70. doi: 10.1016/j.cell.2012.01.035
4. He Y, Kosciolek T, Tang J, Zhou Y, Li Z, Ma X, et al. Gut microbiome and magnetic resonance spectroscopy study of subjects at ultra-high risk for psychosis may support the membrane hypothesis. Eur Psychiatry (2018) 53:37–45. doi: 10.1016/j.eurpsy.2018.05.011
5. Sandhu KV, Sherwin E, Schellekens H, Stanton C, Dinan TG, Cryan JF. Feeding the microbiota-gut-brain axis: diet, microbiome, and neuropsychiatry. Transl Res (2017) 179:223–44. doi: 10.1016/j.trsl.2016.10.002
6. Turnbaugh PJ, Quince C, Faith JJ, McHardy AC, Yatsunenko T, Niazi F, et al. Organismal, genetic, and transcriptional variation in the deeply sequenced gut microbiomes of identical twins. Proc Natl Acad Sci U S A (2010) 107(16):7503–8. doi: 10.1073/pnas.1002355107
7. Hall AB, Tolonen AC, Xavier RJ. Human genetic variation and the gut microbiome in disease. Nat Rev Genet (2017) 18(11):690–9. doi: 10.1038/nrg.2017.63
8. Arnold IC, Dehzad N, Reuter S, Martin H, Becher B, Taube C, et al. Helicobacter pylori infection prevents allergic asthma in mouse models through the induction of regulatory T cells. J Clin Invest (2011) 121(8):3088–93. doi: 10.1172/JCI45041
9. Round JL, Mazmanian SK. The gut microbiota shapes intestinal immune responses during health and disease. Nat Rev Immunol (2009) 9(5):313–23. doi: 10.1038/nri2515
10. Round JL, Lee SM, Li J, Tran G, Jabri B, Chatila TA, et al. The Toll-like receptor 2 pathway establishes colonization by a commensal of the human microbiota. Science (2011) 332(6032):974–7. doi: 10.1126/science.1206095
11. Elinav E, Strowig T, Kau AL, Henao-Mejia J, Thaiss CA, Booth CJ, et al. NLRP6 inflammasome regulates colonic microbial ecology and risk for colitis. Cell (2011) 145(5):745–57. doi: 10.1016/j.cell.2011.04.022
12. Lathrop SK, Bloom SM, Rao SM, Nutsch K, Lio CW, Santacruz N, et al. Peripheral education of the immune system by colonic commensal microbiota. Nature (2011) 478(7368):250–4. doi: 10.1038/nature10434
13. Spor A, Koren O, Ley R. Unravelling the effects of the environment and host genotype on the gut microbiome. Nat Rev Microbiol (2011) 9(4):279–90. doi: 10.1038/nrmicro2540
14. Perry S, de la Luz Sanchez M, Yang S, Haggerty TD, Hurst P, Perez-Perez G, et al. Gastroenteritis and transmission of Helicobacter pylori infection in households. Emerg Infect Dis (2006) 12(11):1701–8. doi: 10.3201/eid1211.060086
15. Dicksved J, Halfvarson J, Rosenquist M, Jarnerot G, Tysk C, Apajalahti J, et al. Molecular analysis of the gut microbiota of identical twins with Crohn’s disease. ISME J (2008) 2(7):716–27. doi: 10.1038/ismej.2008.37
16. Sekirov I, Russell SL, Antunes LC, Finlay BB. Gut microbiota in health and disease. Physiol Rev (2010) 90(3):859–904. doi: 10.1152/physrev.00045.2009
17. Pflughoeft KJ, Versalovic J. Human microbiome in health and disease. Annu Rev Pathol (2012) 7:99–122. doi: 10.1146/annurev-pathol-011811-132421
18. Ley RE, Backhed F, Turnbaugh P, Lozupone CA, Knight RD, Gordon JI. Obesity alters gut microbial ecology. Proc Natl Acad Sci U S A (2005) 102(31):11070–5. doi: 10.1073/pnas.0504978102
19. Armougom F, Henry M, Vialettes B, Raccah D, Raoult D. Monitoring bacterial community of human gut microbiota reveals an increase in Lactobacillus in obese patients and Methanogens in anorexic patients. PloS One (2009) 4(9):e7125. doi: 10.1371/journal.pone.0007125
20. Brown LM. Helicobacter pylori: epidemiology and routes of transmission. Epidemiol Rev (2000) 22(2):283–97. doi: 10.1093/oxfordjournals.epirev.a018040
21. Ridaura VK, Faith JJ, Rey FE, Cheng J, Duncan AE, Kau AL, et al. Gut microbiota from twins discordant for obesity modulate metabolism in mice. Science (2013) 341(6150):1241214. doi: 10.1126/science.1241214
22. Cryan JF, O’Mahony SM. The microbiome-gut-brain axis: from bowel to behavior. Neurogastroenterol Motil Off J Eur Gastrointestinal Motil Soc (2011) 23(3):187–92. doi: 10.1111/j.1365-2982.2010.01664.x
23. Rhee SH, Pothoulakis C, Mayer EA. Principles and clinical implications of the brain-gut-enteric microbiota axis. Nat Rev Gastroenterol Hepatol (2009) 6(5):306–14. doi: 10.1038/nrgastro.2009.35
24. Tandon R, Keshavan MS, Nasrallah HA. Schizophrenia, “Just the Facts”: What we know in 2008 Part 1: Overview. Schizophr Res (2008) 100:4–19. doi: 10.1016/j.schres.2008.01.022
26. McCutcheon RA, Reis Marques T, Howes OD. Schizophrenia-An Overview. JAMA Psychiatry (2020) 77(2):201–10. doi: 10.1001/jamapsychiatry.2019.3360
27. van Os J, Kapur S. Schizophrenia. Lancet (2009) 374(9690):635–45. doi: 10.1016/s0140-6736(09)60995-8
28. Javitt DC, Zukin SR. Recent advances in the phencyclidine model of schizophrenia. Am J Psychiatry (1991) 148(10):1301–8. doi: 10.1176/ajp.148.10.1301
29. Fujimoto T, Takeuch K, Matsumoto T, Kamimura K, Hamada R, Nakamura K, et al. Abnormal glucose metabolism in the anterior cingulate cortex in patients with schizophrenia. Psychiatry Res (2007) 154(1):49–58. doi: 10.1016/j.pscychresns.2006.04.002
30. Dwyer DS, Bradley RJ, Kablinger AS, Freeman AM. 3rd. Glucose metabolism in relation to schizophrenia and antipsychotic drug treatment. Ann Clin Psychiatry (2001) 13(2):103–13. doi: 10.3109/10401230109148955
31. Du F, Cooper AJ, Thida T, Sehovic S, Lukas SE, Cohen BM, et al. In vivo evidence for cerebral bioenergetic abnormalities in schizophrenia measured using 31P magnetization transfer spectroscopy. JAMA Psychiatry (2014) 71(1):19–27. doi: 10.1001/jamapsychiatry.2013.2287
32. Nascimento JM, Martins-de-Souza D. The proteome of schizophrenia. NPJ Schizophr (2015) 1:14003. doi: 10.1038/npjschz.2014.3
33. Davis J, Eyre H, Jacka FN, Dodd S, Dean O, McEwen S, et al. A review of vulnerability and risks for schizophrenia: Beyond the two hit hypothesis. Neurosci Biobehav Rev (2016) 65:185–94. doi: 10.1016/j.neubiorev.2016.03.017
34. Craddock N, O’Donovan MC, Owen MJ. The genetics of schizophrenia and bipolar disorder: dissecting psychosis. J Med Genet (2005) 42(3):193–204. doi: 10.1136/jmg.2005.030718
35. Kavanagh DH, Tansey KE, O’Donovan MC, Owen MJ. Schizophrenia genetics: emerging themes for a complex disorder. Mol Psychiatry (2015) 20(1):72–6. doi: 10.1038/mp.2014.148
36. Misiak B, Stramecki F, Gaweda L, Prochwicz K, Sasiadek MM, Moustafa AA, et al. Interactions Between Variation in Candidate Genes and Environmental Factors in the Etiology of Schizophrenia and Bipolar Disorder: a Systematic Review. Mol Neurobiol (2018) 55(6):5075–100. doi: 10.1007/s12035-017-0708-y
37. Tamburini S, Shen N, Wu HC, Clemente JC. The microbiome in early life: implications for health outcomes. Nat Med (2016) 22(7):713–22. doi: 10.1038/nm.4142
38. Dinan TG, Borre YE, Cryan JF. Genomics of schizophrenia: time to consider the gut microbiome? Mol Psychiatry (2014) 19(12):1252–7. doi: 10.1038/mp.2014.93
39. Tannock GW, Savage DC. Influences of dietary and environmental stress on microbial populations in the murine gastrointestinal tract. Infect Immun (1974) 9(3):591–8. doi: 10.1128/IAI.9.3.591-598.1974
40. Zheng P, Zeng B, Liu M, Chen J, Pan J, Han Y, et al. The gut microbiome from patients with schizophrenia modulates the glutamate-glutamine-GABA cycle and schizophrenia-relevant behaviors in mice. Sci Adv (2019) 5(2):eaau8317. doi: 10.1126/sciadv.aau8317
41. Rodrigues-Amorim D, Rivera-Baltanas T, Regueiro B, Spuch C, de Las Heras ME, Vazquez-Noguerol Mendez R, et al. The role of the gut microbiota in schizophrenia: Current and future perspectives. World J Biol Psychiatry Off J World Fed Soc Biol Psychiatry (2018) 19(8):571–85. doi: 10.1080/15622975.2018.1433878
42. Nguyen TT, Hathaway H, Kosciolek T, Knight R, Jeste DV. Gut microbiome in serious mental illnesses: A systematic review and critical evaluation. Schizophr Res (2019) S0920-9964(19):30382–2. doi: 10.1016/j.schres.2019.08.026
43. Severance EG, Yolken RH, Eaton WW. Autoimmune diseases, gastrointestinal disorders and the microbiome in schizophrenia: more than a gut feeling. Schizophr Res (2016) 176(1):23–35. doi: 10.1016/j.schres.2014.06.027
44. Shamseer L, Moher D, Clarke M, Ghersi D, Liberati A, Petticrew M, et al. Preferred reporting items for systematic review and meta-analysis protocols (PRISMA-P) 2015: elaboration and explanation. BMJ (2015) 350:g7647. doi: 10.1136/bmj.g7647
45. Hooijmans CR, Rovers MM, de Vries RBM, Leenaars M, Ritskes-Hoitinga M, Langendam MW. SYRCLE’s risk of bias tool for animal studies. BMC Med Res Methodol (2014) 14(1):43. doi: 10.1186/1471-2288-14-43
46. Dunphy-Doherty F, O’Mahony SM, Peterson VL, O’Sullivan O, Crispie F, Cotter PD, et al. Post-weaning social isolation of rats leads to long-term disruption of the gut microbiota-immune-brain axis. Brain Behav Immun (2018) 68:261–73. doi: 10.1016/j.bbi.2017.10.024
47. Moher DLA, Tetzlaff J, Altman DG. The PRISMA Group Preferred Reporting Items for Systematic Reviews and Meta-Analyses: The PRISMA Statement. PLoS Med (2009) 6(7):e1000097. doi: 10.1371/journal.pmed1000097
48. Li Q, Cheung C, Wei R, Hui ES, Feldon J, Meyer U, et al. Prenatal immune challenge is an environmental risk factor for brain and behavior change relevant to schizophrenia: evidence from MRI in a mouse model. PloS One (2009) 4(7):e6354. doi: 10.1371/journal.pone.0006354
49. Coyle JT. NMDA receptor and schizophrenia: a brief history. Schizophr Bull (2012) 38(5):920–6. doi: 10.1093/schbul/sbs076
50. McCutcheon RA, Krystal JH, Howes OD. Dopamine and glutamate in schizophrenia: biology, symptoms and treatment. World Psychiatry Off J World Psychiatr Assoc (WPA) (2020) 19(1):15–33. doi: 10.1002/wps.20693
51. Robbins TW. Neurobehavioural sequelae of social deprivation in rodents revisited: Modelling social adversity for developmental neuropsychiatric disorders. J Psychopharmacol (2016) 30(11):1082–9. doi: 10.1177/0269881116664450
52. Pyndt Jørgensen B, Krych L, Pedersen TB, Plath N, Redrobe JP, Hansen AK, et al. Investigating the long-term effect of subchronic phencyclidine-treatment on novel object recognition and the association between the gut microbiota and behavior in the animal model of schizophrenia. Physiol Behav (2015) 141:32–9. doi: 10.1016/j.physbeh.2014.12.042
53. Hsiao EY, McBride SW, Hsien S, Sharon G, Hyde ER, McCue T, et al. Microbiota modulate behavioral and physiological abnormalities associated with neurodevelopmental disorders. Cell (2013) 155(7):1451–63. doi: 10.1016/j.cell.2013.11.024
54. Schwarz E, Maukonen J, Hyytiäinen T, Kieseppä T, Orešič M, Sabunciyan S, et al. Analysis of microbiota in first episode psychosis identifies preliminary associations with symptom severity and treatment response. Schizophr Res (2018) 192:398–403. doi: 10.1016/j.schres.2017.04.017
55. Yuan X, Zhang P, Wang Y, Liu Y, Li X, Kumar BU, et al. Changes in metabolism and microbiota after 24-week risperidone treatment in drug naive, normal weight patients with first episode schizophrenia. Schizophr Res (2018) 201:299–306. doi: 10.1016/j.schres.2018.05.017
56. Nguyen TT, Kosciolek T, Maldonado Y, Daly RE, Martin AS, McDonald D, et al. Differences in gut microbiome composition between persons with chronic schizophrenia and healthy comparison subjects. Schizophr Res (2018) 204:23–9. doi: 10.1016/j.schres.2018.09.014
57. Shen Y, Xu JT, Li ZY, Huang YC, Yuan Y, Wang JX, et al. Analysis of gut microbiota diversity and auxiliary diagnosis as a biomarker in patients with schizophrenia: a cross-sectional study. Schizophr Res (2018) 197:470–7. doi: 10.1016/j.schres.2018.01.002
58. Yung AR, McGorry PD, McFarlane CA, Jackson HJ, Patton GC, Rakkar A. Monitoring and care of young people at incipient risk of psychosis. Schizophr Bull (1996) 22(2):283–303. doi: 10.1093/schbul/22.2.283
59. Yung AR. Treatment of people at ultra-high risk for psychosis. World Psychiatry Off J World Psychiatr Assoc (WPA) (2017) 16(2):207–8. doi: 10.1002/wps.20424
60. Nguyen NP, Warnow T, Pop M, White B. A perspective on 16S rRNA operational taxonomic unit clustering using sequence similarity. NPJ Biofilms Microbiomes (2016) 2:16004. doi: 10.1038/npjbiofilms.2016.4
61. Flowers SA, Evans SJ, Ward KM, McInnis MG, Ellingrod VL. Interaction Between Atypical Antipsychotics and the Gut Microbiome in a Bipolar Disease Cohort. Pharmacotherapy (2017) 37(3):261–7. doi: 10.1002/phar.1890
62. Graessler J, Qin Y, Zhong H, Zhang J, Licinio J, Wong ML, et al. Metagenomic sequencing of the human gut microbiome before and after bariatric surgery in obese patients with type 2 diabetes: correlation with inflammatory and metabolic parameters. Pharmacogenomics J (2013) 13(6):514–22. doi: 10.1038/tpj.2012.43
63. Burcelin R, Garidou L, Pomie C. Immuno-microbiota cross and talk: the new paradigm of metabolic diseases. Semin Immunol (2012) 24(1):67–74. doi: 10.1016/j.smim.2011.11.011
64. Carvalho FA, Koren O, Goodrich JK, Johansson ME, Nalbantoglu I, Aitken JD, et al. Transient inability to manage proteobacteria promotes chronic gut inflammation in TLR5-deficient mice. Cell Host Microbe (2012) 12(2):139–52. doi: 10.1016/j.chom.2012.07.004
65. Martinez-Medina M, Denizot J, Dreux N, Robin F, Billard E, Bonnet R, et al. Western diet induces dysbiosis with increased E coli in CEABAC10 mice, alters host barrier function favouring AIEC colonisation. Gut (2014) 63(1):116–24. doi: 10.1136/gutjnl-2012-304119
66. Castro-Nallar E, Bendall ML, Pérez-Losada M, Sabuncyan S, Severance EG, Dickerson FB, et al. Composition, taxonomy and functional diversity of the oropharynx microbiome in individuals with schizophrenia and controls. PeerJ (2015) 3(8):e1140. doi: 10.7717/peerj.1140
67. Jakobsson HE, Abrahamsson TR, Jenmalm MC, Harris K, Quince C, Jernberg C, et al. Decreased gut microbiota diversity, delayed Bacteroidetes colonisation and reduced Th1 responses in infants delivered by caesarean section. Gut (2014) 63(4):559–66. doi: 10.1136/gutjnl-2012-303249
68. Liou AP, Paziuk M, Luevano JM Jr., Machineni S, Turnbaugh PJ, Kaplan LM. Conserved shifts in the gut microbiota due to gastric bypass reduce host weight and adiposity. Sci Transl Med (2013) 5(178):178ra41. doi: 10.1126/scitranslmed.3005687
69. Fei N, Zhao L. An opportunistic pathogen isolated from the gut of an obese human causes obesity in germfree mice. ISME J (2013) 7(4):880–4. doi: 10.1038/ismej.2012.153
70. Morgan XC, Tickle TL, Sokol H, Gevers D, Devaney KL, Ward DV, et al. Dysfunction of the intestinal microbiome in inflammatory bowel disease and treatment. Genome Biol (2012) 13(9):R79. doi: 10.1186/gb-2012-13-9-r79
71. Annamalai A, Kosir U, Tek C. Prevalence of obesity and diabetes in patients with schizophrenia. World J Diabetes (2017) 8(8):390–6. doi: 10.4239/wjd.v8.i8.390
72. Severance EG, Prandovszky E, Castiglione J, Yolken RH. Gastroenterology issues in schizophrenia: why the gut matters. Curr Psychiatry Rep (2015) 17(5):27. doi: 10.1007/s11920-015-0574-0
73. Muller N, Weidinger E, Leitner B, Schwarz MJ. The role of inflammation in schizophrenia. Front Neurosci (2015) 9:372. doi: 10.3389/fnins.2015.00372
74. Hand TW, Vujkovic-Cvijin I, Ridaura VK, Belkaid Y. Linking the Microbiota, Chronic Disease, and the Immune System. Trends Endocrinol Metab (2016) 27(12):831–43. doi: 10.1016/j.tem.2016.08.003
75. Bahr SM, Tyler BC, Wooldridge N, Butcher BD, Burns TL, Teesch LM, et al. Use of the second-generation antipsychotic, risperidone, and secondary weight gain are associated with an altered gut microbiota in children. Transl Psychiatry (2015) 5:e652. doi: 10.1038/tp.2015.135
76. Nicholson JK, Holmes E, Kinross J, Burcelin R, Gibson G, Jia W, et al. Host-gut microbiota metabolic interactions. Science (2012) 336(6086):1262–7. doi: 10.1126/science.1223813
77. Lafaye A, Junot C, Ramounet-Le Gall B, Fritsch P, Ezan E, Tabet JC. Profiling of sulfoconjugates in urine by using precursor ion and neutral loss scans in tandem mass spectrometry. Application to the investigation of heavy metal toxicity in rats. J Mass Spectrom (2004) 39(6):655–64. doi: 10.1002/jms.635
78. Yolken RH, Severance EG, Sabunciyan S, Gressitt KL, Chen O, Stallings C, et al. Metagenomic Sequencing Indicates That the Oropharyngeal Phageome of Individuals With Schizophrenia Differs From That of Controls. Schizophr Bull (2015) 41(5):1153–61. doi: 10.1093/schbul/sbu197
79. Gispen-de Wied CC. Stress in schizophrenia: an integrative view. Eur J Pharmacology (2000) 405(1-3):375–84. doi: 10.1016/s0014-2999(00)00567-7
80. Bailey MT, Dowd SE, Galley JD, Hufnagle AR, Allen RG, Lyte M. Exposure to a social stressor alters the structure of the intestinal microbiota: implications for stressor-induced immunomodulation. Brain Behav Immun (2011) 25(3):397–407. doi: 10.1016/j.bbi.2010.10.023
81. Barka EA, Vatsa P, Sanchez L, Gaveau-Vaillant N, Jacquard C, Meier-Kolthoff JP, et al. Taxonomy, Physiology, and Natural Products of Actinobacteria. Microbiol Mol Biol Rev (2016) 80(1):1–43. doi: 10.1128/MMBR.00019-15
82. Chen J, Wright K, Davis JM, Jeraldo P, Marietta EV, Murray J, et al. An expansion of rare lineage intestinal microbes characterizes rheumatoid arthritis. Genome Med (2016) 8(1):43. doi: 10.1186/s13073-016-0299-7
83. Wood NC, Hamilton I, Axon ATR, Khan SA, Quirke P, Mindham RHS, et al. Abnormal Intestinal Permeability. Br J Psychiatry J Ment Sci (2018) 150(06):853–6. doi: 10.1192/bjp.150.6.853
84. Sahbaz C, Zibandeyeh N, Kurtulmuş A, Avaroglu G, Kırpınar İ, Sahin F, et al. F239. Role of Lymphocyte Subsets and T-Cell Profiles in the Immune Dysfunction of Schizophrenia. Biol Psychiatry (2018) 83(9):S331–S2. doi: 10.1016/j.biopsych.2018.02.853
85. Ding M, Song X, Zhao J, Gao J, Li X, Yang G, et al. Activation of Th17 cells in drug naive, first episode schizophrenia. Prog Neuropsychopharmacol Biol Psychiatry (2014) 51:78–82. doi: 10.1016/j.pnpbp.2014.01.001
86. Cabal A, Wassenaar TM, Ussery DW. Gender Differences in the Gut Microbiome and How These Affect Cardiovascular Diseases. Gender Differences in the Pathogenesis and Management of Heart Disease. (2018). p.89–100.
87. Yatsunenko T, Rey FE, Manary MJ, Trehan I, Dominguez-Bello MG, Contreras M, et al. Human gut microbiome viewed across age and geography. Nature (2012) 486(7402):222–7. doi: 10.1038/nature11053
88. Salazar N, Valdes-Varela L, Gonzalez S, Gueimonde M, de Los Reyes-Gavilan CG. Nutrition and the gut microbiome in the elderly. Gut Microbes (2017) 8(2):82–97. doi: 10.1080/19490976.2016.1256525
89. Franklin CL, Ericsson AC. Microbiota and reproducibility of rodent models. Lab Anim (NY) (2017) 46(4):114–22. doi: 10.1038/laban.1222
90. Penders J, Thijs C, Vink C, Stelma FF, Snijders B, Kummeling I, et al. Factors influencing the composition of the intestinal microbiota in early infancy. Pediatrics (2006) 118(2):511–21. doi: 10.1542/peds.2005-2824
91. Backhed F, Roswall J, Peng Y, Feng Q, Jia H, Kovatcheva-Datchary P, et al. Dynamics and Stabilization of the Human Gut Microbiome during the First Year of Life. Cell Host Microbe (2015) 17(5):690–703. doi: 10.1016/j.chom.2015.04.004
92. Dominguez-Bello MG, De Jesus-Laboy KM, Shen N, Cox LM, Amir A, Gonzalez A, et al. Partial restoration of the microbiota of cesarean-born infants via vaginal microbial transfer. Nat Med (2016) 22(3):250–3. doi: 10.1038/nm.4039
93. McGrath JJ, Féron FP, Burne THJ, Mackay-Sim A, Eyles DW. The neurodevelopmental hypothesis of schizophrenia: a review of recent developments. Ann medicine (2009) 35(2):86–93. doi: 10.1080/07853890310010005
94. Boksa P, El-Khodor BF. Birth insult interacts with stress at adulthood to alter dopaminergic function in animal models: possible implications for schizophrenia and other disorders. Neurosci Biobehav Rev (2003) 27(1-2):91–101. doi: 10.1016/s0149-7634(03)00012-5
95. Bailey MT, Coe CL. Maternal separation disrupts the integrity of the intestinal microflora in infant rhesus monkeys. Dev Psychobiol (1999) 35(2):146–55. doi: 10.1002/(sici)1098-2302(199909)35:2<146::aid-dev7>3.0.co;2-g
96. O’Mahony SM, Marchesi JR, Scully P, Codling C, Ceolho AM, Quigley EM, et al. Early life stress alters behavior, immunity, and microbiota in rats: implications for irritable bowel syndrome and psychiatric illnesses. Biol Psychiatry (2009) 65(3):263–7. doi: 10.1016/j.biopsych.2008.06.026
97. Santos J, Yang PC, Soderholm JD, Benjamin M, Perdue MH. Role of mast cells in chronic stress induced colonic epithelial barrier dysfunction in the rat. Gut (2001) 48(5):630–6. doi: 10.1136/gut.48.5.630
98. Berger M, Juster RP, Westphal S, Amminger GP, Bogerts B, Schiltz K, et al. Allostatic load is associated with psychotic symptoms and decreases with antipsychotic treatment in patients with schizophrenia and first-episode psychosis. Psychoneuroendocrinology (2018) 90:35–42. doi: 10.1016/j.psyneuen.2018.02.001
99. Birley JLT, Brown GW. Crises and Life Changes preceding the Onset or Relapse of Acute Schizophrenia: Clinical Aspects. Br J Psychiatry (1970) 116(532):327–33. doi: 10.1192/bjp.116.532.327
100. Norman RM, Malla AK. A prospective study of daily stressors and symptomatology in schizophrenic patients. Soc Psychiatry Psychiatr Epidemiol (1994) 29(6):244–9. doi: 10.1007/BF00802047
101. Reininghaus U, Kempton MJ, Valmaggia L, Craig TK, Garety P, Onyejiaka A, et al. Stress Sensitivity, Aberrant Salience, and Threat Anticipation in Early Psychosis: An Experience Sampling Study. Schizophr Bull (2016) 42(3):712–22. doi: 10.1093/schbul/sbv190
102. Liberman RP, Mueser KT, Wallace CJ. Social skills training for schizophrenic individuals at risk for relapse. Am J Psychiatry (1986) 143(4):523–6. doi: 10.1176/ajp.143.4.523
103. Barrelet L, Ferrero F, Szigethy L, Giddey C, Pellizzer G. Expressed Emotion and First-Admission Schizophrenia. Br J Psychiatry J Ment Sci (2018) 156(03):357–62. doi: 10.1192/bjp.156.3.357
104. Breier A, Buchanan RW. The effects of metabolic stress on plasma progesterone in healthy volunteers and schizophrenic patients. Life Sci (1992) 51(19):1527–34. doi: 10.1016/0024-3205(92)90563-5
105. Jansen LM, Gispen-de Wied CC, Kahn RS. Selective impairments in the stress response in schizophrenic patients. Psychopharmacol (Berl) (2000) 149(3):319–25. doi: 10.1007/s002130000381
106. Berger M, Kraeuter AK, Romanik D, Malouf P, Amminger GP, Sarnyai Z. Cortisol awakening response in patients with psychosis: Systematic review and meta-analysis. Neurosci Biobehav Rev (2016) 68:157–66. doi: 10.1016/j.neubiorev.2016.05.027
107. Torrey EF, Yolken RH. Toxoplasma gondii and schizophrenia. Emerg Infect Dis (2003) 9(11):1375–80. doi: 10.3201/eid0911.030143
108. Severance EG, Xiao J, Jones-Brando L, Sabunciyan S, Li Y, Pletnikov M, et al. Toxoplasma gondii-A Gastrointestinal Pathogen Associated with Human Brain Diseases. Int Rev neurobiology (2016) 131:143–63. doi: 10.1016/bs.irn.2016.08.008
109. Prandovszky E, Li Y, Sabunciyan S, Steinfeldt CB, Avalos LN, Gressitt KL, et al. Toxoplasma gondii-Induced Long-Term Changes in the Upper Intestinal Microflora during the Chronic Stage of Infection. Scientifica (Cairo) (2018) 2018:2308619. doi: 10.1155/2018/2308619
110. Backhed F, Ding H, Wang T, Hooper LV, Koh GY, Nagy A, et al. The gut microbiota as an environmental factor that regulates fat storage. Proc Natl Acad Sci U S A (2004) 101(44):15718–23. doi: 10.1073/pnas.0407076101
111. Kovatcheva-Datchary P, Arora T. Nutrition, the gut microbiome and the metabolic syndrome. Best Pract Res Clin Gastroenterol (2013) 27(1):59–72. doi: 10.1016/j.bpg.2013.03.017
112. Li H, Li T, Beasley DE, Hedenec P, Xiao Z, Zhang S, et al. Diet Diversity Is Associated with Beta but not Alpha Diversity of Pika Gut Microbiota. Front microbiology (2016) 7:1169. doi: 10.3389/fmicb.2016.01169
113. Blanton LV, Charbonneau MR, Salih T, Barratt MJ, Venkatesh S, Ilkaveya O, et al. Gut bacteria that prevent growth impairments transmitted by microbiota from malnourished children. Science (2016) 351(6275):aad3311. doi: 10.1126/science.aad3311
114. Russell WR, Gratz SW, Duncan SH, Holtrop G, Ince J, Scobbie L, et al. High-protein, reduced-carbohydrate weight-loss diets promote metabolite profiles likely to be detrimental to colonic health. Am J Clin Nutr (2011) 93(5):1062–72. doi: 10.3945/ajcn.110.002188
115. Newell C, Bomhof MR, Reimer RA, Hittel DS, Rho JM, Shearer J. Ketogenic diet modifies the gut microbiota in a murine model of autism spectrum disorder. Mol Autism (2016) 7(1):37. doi: 10.1186/s13229-016-0099-3
116. Hildebrandt MA, Hoffmann C, Sherrill-Mix SA, Keilbaugh SA, Hamady M, Chen YY, et al. High-fat diet determines the composition of the murine gut microbiome independently of obesity. Gastroenterology (2009) 137(5):1716–24 e1-2. doi: 10.1053/j.gastro.2009.08.042
117. Murphy EA, Velazquez KT, Herbert KM. Influence of high-fat diet on gut microbiota: a driving force for chronic disease risk. Curr Opin Clin Nutr Metab Care (2015) 18(5):515–20. doi: 10.1097/MCO.0000000000000209
118. De Filippis F, Pellegrini N, Vannini L, Jeffery IB, La Storia A, Laghi L, et al. High-level adherence to a Mediterranean diet beneficially impacts the gut microbiota and associated metabolome. Gut (2016) 65(11):1812–21. doi: 10.1136/gutjnl-2015-309957
119. Strassnig M, Brar JS, Ganguli R. Dietary Intake of Patients with Schizophrenia. Psychiatry (Edgmont) (2005) 2(2):31–5.
120. Elman I, Borsook D, Lukas SE. Food intake and reward mechanisms in patients with schizophrenia: implications for metabolic disturbances and treatment with second-generation antipsychotic agents. Neuropsychopharmacology (2006) 31(10):2091–120. doi: 10.1038/sj.npp.1301051
121. Brown S, Birtwistle J, Roe L, Thompson C. The unhealthy lifestyle of people with schizophrenia. psychol Med (1999) 29(3):697–701. doi: 10.1017/S0033291798008186
122. Strassnig M, Brar JS, Qanguli R. Nutritional Assessment of Patients With Schizophrenia: A Preliminary Study. Schizophr Bull (2003) 29(2):393–7. doi: 10.1093/oxfordjournals.schbul.a007013
123. McCreadie R, Macdonald E, Blacklock C, Tilak-Singh D, Wiles D, Halliday J, et al. Dietary intake of schizophrenic patients in Nithsdale, Scotland: case-control study. BMJ (1998) 317(7161):784–5. doi: 10.1136/bmj.317.7161.784
124. Zhang ZJ, Yao ZJ, Liu W, Fang Q, Reynolds GP. Effects of antipsychotics on fat deposition and changes in leptin and insulin levels. Magnetic resonance imaging study of previously untreated people with schizophrenia. Br J Psychiatry J Ment Sci (2004) 184:58–62. doi: 10.1192/bjp.184.1.58
125. Utzschneider KM, Kratz M, Damman CJ, Hullar M. Mechanisms Linking the Gut Microbiome and Glucose Metabolism. J Clin Endocrinol Metab (2016) 101(4):1445–54. doi: 10.1210/jc.2015-4251
126. Lambert JE, Myslicki JP, Bomhof MR, Belke DD, Shearer J, Reimer RA. Exercise training modifies gut microbiota in normal and diabetic mice. Appl Physiol Nutr Metab (2015) 40(7):749–52. doi: 10.1139/apnm-2014-0452
127. Zhao X, Zhang Z, Hu B, Huang W, Yuan C, Zou L. Response of Gut Microbiota to Metabolite Changes Induced by Endurance Exercise. Front microbiology (2018) 9:765:765. doi: 10.3389/fmicb.2018.00765
128. Allen JM, Mailing LJ, Niemiro GM, Moore R, Cook MD, White BA, et al. Exercise Alters Gut Microbiota Composition and Function in Lean and Obese Humans. Med Sci Sports Exerc (2018) 50(4):747–57. doi: 10.1249/MSS.0000000000001495
129. Welly RJ, Liu TW, Zidon TM, Rowles JL,3, Park YM, Smith TN, et al. Comparison of Diet versus Exercise on Metabolic Function and Gut Microbiota in Obese Rats. Med Sci Sports Exerc (2016) 48(9):1688–98. doi: 10.1249/MSS.0000000000000964
130. Bressa C, Bailen-Andrino M, Perez-Santiago J, Gonzalez-Soltero R, Perez M, Montalvo-Lominchar MG, et al. Differences in gut microbiota profile between women with active lifestyle and sedentary women. PloS One (2017) 12(2):e0171352. doi: 10.1371/journal.pone.0171352
131. Clarke SF, Murphy EF, O’Sullivan O, Lucey AJ, Humphreys M, Hogan A, et al. Exercise and associated dietary extremes impact on gut microbial diversity. Gut (2014) 63(12):1913–20. doi: 10.1136/gutjnl-2013-306541
132. Munukka E, Ahtiainen JP, Puigbo P, Jalkanen S, Pahkala K, Keskitalo A, et al. Six-Week Endurance Exercise Alters Gut Metagenome That Is not Reflected in Systemic Metabolism in Over-weight Women. Front microbiology (2018) 9:2323:2323. doi: 10.3389/fmicb.2018.02323
133. Chamove AS. Positive short-term effects of activity on behavior in chronic schizophrenic pa- tients. Br J Clin Psychol (1986) 25:125–33. doi: 10.1111/j.2044-8260.1986.tb00681.x
134. Vancampfort D, Stubbs B, Sienaert P, Wyckaert S, De Hert M, Soundy A, et al. A comparison of physical fitness in patients with bipolar disorder, schizophrenia and healthy controls. Disabil Rehabil (2016) 38(20):2047–51. doi: 10.3109/09638288.2015.1114037
135. Vogtmann E, Flores R, Yu G, Freedman ND, Shi J, Gail MH, et al. Association between tobacco use and the upper gastrointestinal microbiome among Chinese men. Cancer Causes Control (2015) 26(4):581–8. doi: 10.1007/s10552-015-0535-2
136. Capurso G, Lahner E. The interaction between smoking, alcohol and the gut microbiome. Best Pract Res Clin Gastroenterol (2017) 31(5):579–88. doi: 10.1016/j.bpg.2017.10.006
137. Tomoda K, Kubo K, Asahara T, Andoh A, Nomoto K, Nishii Y, et al. Cigarette smoke decreases organic acids levels and population of bifidobacterium in the caecum of rats. J Toxicol Sci (2011) 36(3):261–6. doi: 10.2131/jts.36.261
138. Allais L, Kerckhof FM, Verschuere S, Bracke KR, De Smet R, Laukens D, et al. Chronic cigarette smoke exposure induces microbial and inflammatory shifts and mucin changes in the murine gut. Environ Microbiol (2016) 18(5):1352–63. doi: 10.1111/1462-2920.12934
139. Wang H, Zhao JX, Hu N, Ren J, Du M, Zhu MJ. Side-stream smoking reduces intestinal inflammation and increases expression of tight junction proteins. World J Gastroenterol (2012) 18(18):2180–7. doi: 10.3748/wjg.v18.i18.2180
140. Rogers MA, Greene MT, Saint S, Chenoweth CE, Malani PN, Trivedi I, et al. Higher rates of Clostridium difficile infection among smokers. PloS One (2012) 7(7):e42091. doi: 10.1371/journal.pone.0042091
141. Biedermann L, Zeitz J, Mwinyi J, Sutter-Minder E, Rehman A, Ott SJ, et al. Smoking cessation induces profound changes in the composition of the intestinal microbiota in humans. PloS One (2013) 8(3):e59260. doi: 10.1371/journal.pone.0059260
142. Hartz SM, Pato CN, Medeiros H, Cavazos-Rehg P, Sobell JL, Knowles JA, et al. Comorbidity of severe psychotic disorders with measures of substance use. JAMA Psychiatry (2014) 71(3):248–54. doi: 10.1001/jamapsychiatry.2013.3726
143. Goodrich JK, Waters JL, Poole AC, Sutter JL, Koren O, Blekhman R, et al. Human genetics shape the gut microbiome. Cell (2014) 159(4):789–99. doi: 10.1016/j.cell.2014.09.053
144. Matheson SL, Shepherd AM, Pinchbeck RM, Laurens KR, Carr VJ. Childhood adversity in schizophrenia: a systematic meta-analysis. psychol Med (2013) 43(2):225–38. doi: 10.1017/S0033291712000785
145. Miyamoto S, Duncan GE, Marx CE, Lieberman JA. Treatments for schizophrenia: a critical review of pharmacology and mechanisms of action of antipsychotic drugs. Mol Psychiatry (2004) 10:79–104. doi: 10.1038/sj.mp.4001556
146. Davey KJ, Cotter PD, O’Sullivan O, Crispie F, Dinan TG, Cryan JF, et al. Antipsychotics and the gut microbiome: olanzapine-induced metabolic dysfunction is attenuated by antibiotic administration in the rat. Transl Psychiatry (2013) 3:e309. doi: 10.1038/tp.2013.83
147. Nadal I, Santacruz A, Marcos A, Warnberg J, Garagorri JM, Moreno LA, et al. Shifts in clostridia, bacteroides and immunoglobulin-coating fecal bacteria associated with weight loss in obese adolescents. Int J Obes (Lond) (2009) 33(7):758–67. doi: 10.1038/ijo.2008.260
148. Davey KJ, O’Mahony SM, Schellekens H, O’Sullivan O, Bienenstock J, Cotter PD, et al. Gender-dependent consequences of chronic olanzapine in the rat: effects on body weight, inflammatory, metabolic and microbiota parameters. Psychopharmacol (Berl) (2012) 221(1):155–69. doi: 10.1007/s00213-011-2555-2
149. Morgan AP, Crowley JJ, Nonneman RJ, Quackenbush CR, Miller CN, Ryan AK, et al. The Antipsychotic Olanzapine Interacts with the Gut Microbiome to Cause Weight Gain in Mouse. PloS One (2014) 9(12):e115225. doi: 10.1371/journal.pone.0115225
150. Kao AC, Spitzer S, Anthony DC, Lennox B, Burnet PWJ. Prebiotic attenuation of olanzapine-induced weight gain in rats: analysis of central and peripheral biomarkers and gut microbiota. Transl Psychiatry (2018) 8(1):66. doi: 10.1038/s41398-018-0116-8
151. Bahr SM, Weidemann BJ, Castro AN, Walsh JW, deLeon O, Burnett CML, et al. Risperidone-induced weight gain is mediated through shifts in the gut microbiome and suppression of energy expenditure. EBioMedicine (2015) 2(11):1725–34. doi: 10.1016/j.ebiom.2015.10.018
152. Shilling PD, Feifel D. Potential of Oxytocin in the Treatment of Schizophrenia. CNS Drugs (2016) 30(3):193–208. doi: 10.1007/s40263-016-0315-x
153. Erdman SE, Poutahidis T. Microbes and Oxytocin: Benefits for Host Physiology and Behavior. Int Rev neurobiology (2016) 131:91–126. doi: 10.1016/bs.irn.2016.07.004
154. Kohler-Forsberg O, Petersen L, Gasse C, Mortensen PB, Dalsgaard S, Yolken RH, et al. A Nationwide Study in Denmark of the Association Between Treated Infections and the Subsequent Risk of Treated Mental Disorders in Children and Adolescents. JAMA Psychiatry (2018) 76(3):271–9. doi: 10.1001/jamapsychiatry.2018.3428
155. Lydholm CN, Kohler-Forsberg O, Nordentoft M, Yolken RH, Mortensen PB, Petersen L, et al. Parental Infections Before, During, and After Pregnancy as Risk Factors for Mental Disorders in Childhood and Adolescence: A Nationwide Danish Study. Biol Psychiatry (2019) 85(4):317–25. doi: 10.1016/j.biopsych.2018.09.013
156. Mednick SA, Machon RA, Huttunen MO, Bonett D. Adult schizophrenia following prenatal exposure to an influenza epidemic. Arch Gen Psychiatry (1988) 45(2):189–92. doi: 10.1001/archpsyc.1988.01800260109013
157. Ng QX, Soh AYS, Venkatanarayanan N, Ho CYX, Lim DY. Yeo WS. A Systematic Review of the Effect of Probiotic Supplementation on Schizophrenia Symptoms. Neuropsychobiology (2019) 78(1):1–6. doi: 10.1159/000498862
158. Zhang F, Luo W, Shi Y, Fan Z, Ji G. Should we standardize the 1,700-year-old fecal microbiota transplantation? Am J gastroenterology (2012) 107(11):1755. doi: 10.1038/ajg.2012.251. author reply p -6.
159. Borody T, Leis S, Campbell J, Torres M, Nowak A. Fecal microbiota transplantation (FMT) in multiple sclerosis (MS). Am J gastroenterology (2011) 106:S352. doi: 10.14309/00000434-201110002-00942
160. Oya K, Kishi T, Iwata N. Efficacy and tolerability of minocycline augmentation therapy in schizophrenia: a systematic review and meta-analysis of randomized controlled trials. Hum Psychopharmacol (2014) 29(5):483–91. doi: 10.1002/hup.2426
161. Deakin B, Suckling J, Barnes TRE, Byrne K, Chaudhry IB, Dazzan P, et al. The benefit of minocycline on negative symptoms of schizophrenia in patients with recent-onset psychosis (BeneMin): a randomised, double-blind, placebo-controlled trial. Lancet Psychiatry (2018) 5(11):885–94. doi: 10.1016/s2215-0366(18)30345-6
162. Kraeuter AK, Loxton H, Lima BC, Rudd D, Sarnyai Z. Ketogenic diet reverses behavioral abnormalities in an acute NMDA receptor hypofunction model of schizophrenia. Schizophr Res (2015) 169(1-3):491–3. doi: 10.1016/j.schres.2015.10.041
Keywords: gut microbiota, schizophrenia, early life events, inflammation, microbiota metabolites, stress
Citation: Kraeuter A-K, Phillips R and Sarnyai Z (2020) The Gut Microbiome in Psychosis From Mice to Men: A Systematic Review of Preclinical and Clinical Studies. Front. Psychiatry 11:799. doi: 10.3389/fpsyt.2020.00799
Received: 10 December 2019; Accepted: 24 July 2020;
Published: 11 August 2020.
Edited by:
Guillaume Gourcerol, Université de Rouen, FranceReviewed by:
Sergueï O. Fetissov, Université de Rouen, FranceKiran Veer Sandhu, University College Cork, Ireland
Copyright © 2020 Kraeuter, Phillips and Sarnyai. This is an open-access article distributed under the terms of the Creative Commons Attribution License (CC BY). The use, distribution or reproduction in other forums is permitted, provided the original author(s) and the copyright owner(s) are credited and that the original publication in this journal is cited, in accordance with accepted academic practice. No use, distribution or reproduction is permitted which does not comply with these terms.
*Correspondence: Zoltán Sarnyai, em9sdGFuLnNhcm55YWlAamN1LmVkdS5hdQ==