- Department of Pharmaceutical and Biomedical Sciences, The Raabe College of Pharmacy, Ohio Northern University, Ada, OH, United States
Addiction to psychostimulants like cocaine, methamphetamine, and nicotine poses a continuing medical and social challenge both in the United States and all over the world. Despite a desire to quit drug use, return to drug use after a period of abstinence is a common problem among individuals dependent on psychostimulants. Recovery for psychostimulant drug-dependent individuals is particularly challenging because psychostimulant drugs induce significant changes in brain regions associated with cognitive functions leading to cognitive deficits. These cognitive deficits include impairments in learning/memory, poor decision making, and impaired control of behavioral output. Importantly, these drug-induced cognitive deficits often impact adherence to addiction treatment programs and predispose abstinent addicts to drug use relapse. Additionally, these cognitive deficits impact effective social and professional rehabilitation of abstinent addicts. The goal of this paper is to review neural substrates based on animal studies that could be pharmacologically targeted to reverse psychostimulant-induced cognitive deficits such as impulsivity and impairment in learning and memory. Further, the review will discuss neural substrates that could be used to facilitate extinction learning and thus reduce emotional and behavioral responses to drug-associated cues. Moreover, the review will discuss some non-pharmacological approaches that could be used either alone or in combination with pharmacological compounds to treat the above-mentioned cognitive deficits. Psychostimulant addiction treatment, which includes treatment for cognitive deficits, will help promote abstinence and allow for better rehabilitation and integration of abstinent individuals into society.
Introduction
Addiction to psychostimulant drugs such as cocaine, methamphetamine, and nicotine adds a significant burden on healthcare budgets in the form of premature morbidity and mortality. Alarmingly, the use and abuse of illicit psychostimulant drugs like cocaine and methamphetamine is showing a trend of steady increase than in the last decade (1). In addition to illicit stimulant use, use and abuse of licit weak stimulant like nicotine continues to increase especially in the form of e-cigarettes and vaping (2). In addition, abuse of prescription stimulants like amphetamine, which are used to treat patients with attention deficit hyperactivity (ADHD), also adds to the problem of psychostimulant addiction. While not all people who experiment with psychostimulants will get addicted, an increasing trend of initiation does not augur well for psychostimulant addiction rates. Importantly, factors that promote transition from use/abuse to addiction are not fully understood (3, 4).
Considerable progress has been made over the last few decades in understanding the brain circuitry and pathological changes that facilitate and promote abuse of drugs (5). Despite this progress, significant challenges remain in the treatment of psychostimulant drug addiction (6). For example, currently, among the different psychostimulants described above, the Food and Drug Administration (FDA) has approved treatments for only nicotine (7, 8). Current treatment protocol for psychostimulant addiction depends largely on managing withdrawal symptoms of dependent individuals, providing behavioral/psychotherapy and utilizing self-help support groups (6). The inadequacy of current psychostimulant drug addiction is supported by high rates of relapse among abstinent addicts.
The goal of behavioral/psychotherapy is to help prevent relapse among abstinent addicts by helping them develop coping strategies to deal with cravings and emotional disturbances occurring as a result of withdrawal from psychostimulant drugs (9). This requires engagement of various cognitive domains such as attention, learning, and memory. Ironically, research over the last two decades and more has demonstrated that abuse of psychostimulants results in several cognitive deficits such as impulsivity (i.e., inability to inhibit disadvantageous rapid behavioral responses), risky and/or poor decision making, impaired cognitive flexibility (i.e., impaired ability to alter behavioral responses based on changing environmental contingencies), deficits in learning and memory, and/or hyperattentiveness to drug-associated cues compared with non-drug associated cues (10–13). Interestingly, individuals with pre-existing deficits in cognition and/or suffering from psychiatric disease states that are associated with impaired cognitive function (e.g., schizophrenia and depression) are more vulnerable to abusing illicit and licit stimulants (14, 15). Importantly, recovering addicts with significant cognitive deficits are more vulnerable to relapse (12, 16). Thus, cognitive deficits in recovering drug addicts irrespective of whether they were pre-existing or drug induced need to be adequately treated to promote abstinence among drug addicts (Figure 1).
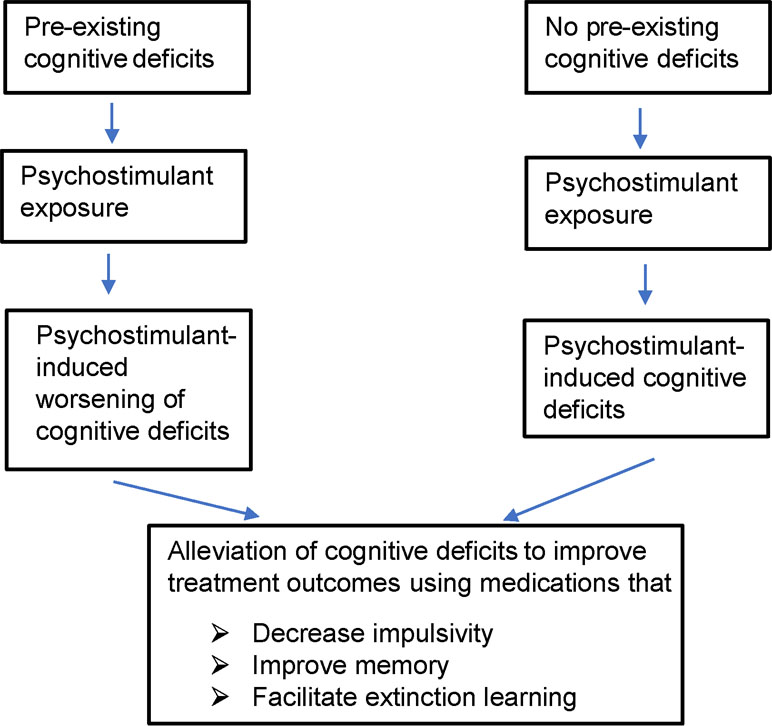
Figure 1 Figure shows overall hypothesis of the review and possible treatment strategies to improve outcomes of psychostimulant addiction treatment. Psychostimulant-induced cognitive deficits include impulsivity, learning/memory impairments, attentional impairment, and impairment in decision making. In this review, we mainly restrict ourselves to targets that could potentially alleviate impulsivity and/or learning/memory impairments and facilitate extinction learning. Patients with pre-existing cognitive deficits prior to drug abuse may need more aggressive treatment to break the vicious cycle of drug addiction.
Among the different psychostimulant-induced cognitive deficits, this review will focus on psychostimulant-induced cognitive deficits such as impulsivity and impairments in learning and memory. The review will primarily identify neural substrates that could be pharmacologically targeted to alleviate psychostimulant-induced cognitive deficits. Finally, the review will discuss evidence from animal studies that support use of non-pharmacological approaches to alleviate the above-mentioned cognitive deficits.
Drug-Induced Cognitive Deficits
Impulsivity
Impulsivity in the human literature is often conceptualized as a personality trait (17). However, in the cognitive neuroscience field and for the purpose of this article, we will refer to impulsivity as behavior resulting from impaired inhibition in specific brain regions that play a role in regulating behavioral output (18). Based on the specific cognitive domains that are disrupted, impulsivity can be divided broadly into behavioral and decisional impulsivity (19). Behavioral impulsivity as the name suggests usually involves a quick behavioral response without consideration to consequences of the behavioral response (19). In contrast, decisional impulsivity involves actions and decisions taken by the individual that are less advantageous to the individual.
Psychostimulant addicts show high levels of both behavioral and decisional impulsivity than do healthy controls (20–27). It is hypothesized that this impulsive behavior is responsible for high rates of relapse among these addicts during abstinence. Consistent with these data, a recent human study reported that smokers and polysubstance abusers who abused nicotine, cocaine, and alcohol were more impulsive than controls (28).
Impulsivity observed in drug-dependent individuals can exist prior to drug abuse and worsens with repeated drug use (Figure 1). In support of this hypothesis, several studies have shown that individuals who are impulsive have greater sensitivity to drugs of abuse, are more likely to experiment with drugs of abuse, and are more vulnerable to develop drug dependence (29–36). This hypothesis is also supported by animal studies. For example, animals showing poor inhibitory control prior to exposure to drugs of abuse (i.e., showed more impulsive behavior) acquired cocaine self-administration behavior much more rapidly than did animals that showed good inhibitory control (37). Additionally, animals that showed more risk-taking behavior as assessed using the rodent model of Iowa gambling task (rIGT) self-administered greater amount of cocaine than did animals that did not display high risk behavior in the same task (38). However, it is not known if repeated use of drugs of abuse induces impulsivity in humans. In animals, repeated administration of cocaine, methamphetamine, and nicotine increased impulsive behavior in animals (39–43). This increase in impulsivity was observed both when animals were challenged with the drug of abuse and during withdrawal from the drug (i.e., when animals were not under the influence of the drug). Thus, these studies support the hypothesis that exposure to drugs of abuse may de novo induce impulsivity.
Keeping with focus of this review, we will only discuss assessment of behavioral and decisional impulsivity in animals. Behavioral impulsivity in animals can be assessed by measuring either premature responding or the ability of an animal to stop already initiated action. In animals, premature responding is measured using the five-choice serial reaction time task (5-CSRTT), while ability of an animal to stop already initiated action is measured using the stop-signal reaction time (SSRT) (44, 45) (Box 1). Several brain regions such as the nucleus accumbens (NAcc), dorsal striatum, infralimbic prefrontal cortex (infralimbic PFC), insula, and hippocampus have been shown to mediate behavioral impulsivity (19, 46–49). In contrast, decisional impulsivity in animals is usually assessed by measuring either temporal discounting or probability discounting. Temporal discounting is assessed using the delay discounting task (DDT), which involves assessing the ability of animal to wait for a larger reward compared with opting for an immediate smaller reward (50) (Box 1). Several studies have identified the role of the basolateral amygdala, orbitofrontal cortex (OFC), and hippocampus in mediating the DDT (51–53). In contrast, probability discounting is assessed using a task known as rIGT or probability discounting task and involves choosing a smaller sure reward (i.e., 100% chance to obtain the reward) compared with a larger reward, which is not always assured (i.e., approximately 50% chance or risky choice) (54, 55) (Box 1). Research has shown that the OFC, amygdala, habenula, and prelimbic PFC play a role in mediating probability discounting (56–58). Despite identifying the role of specific brain regions in specific types of impulsive behavior, more work is required to identify specific signaling mechanisms between the different brain regions.
Box 1. Tasks used to measure psychostimulant-induced impulsivity.
Learning and Memory Deficits
Both learning and working memory deficits have been reported in abstinent psychostimulant addicts (21, 59–63). These learning/memory deficits are hypothesized to result in poor treatment outcomes among abstinent addicts. It is also hypothesized that working memory deficits prior to drug exposure increase vulnerability to drug addiction. Consistent with this hypothesis, individuals suffering from psychiatric disorders with significant learning and memory deficits such as depression and schizophrenia have high rates of stimulant addiction (14, 15, 64, 65). Also, a recent study reported that adolescents with weak working memory were more vulnerable to get addicted to drugs of abuse (66). In fact, acute administration of drugs like nicotine and cocaine enhances hippocampal function (67–69). Thus, individuals may compensate for memory deficits by abusing psychostimulants. Together, these findings suggest that use of psychostimulants induces memory deficits and that memory deficits present prior to drug use promote experimentation with stimulants leading to drug addiction.
Several models such as the Morris water maze, novel object recognition, and delayed match-to-sample task are used to assess learning/memory deficits in animals (Box 2) (70, 71). Similar to humans, chronic exposure and/or withdrawal from psychostimulants induced working memory deficits in animals. For example, animals with chronic extended-access cocaine self-administration experience showed working memory and learning deficits (72, 73). Further, animals undergoing withdrawal after chronic extended access to cocaine showed decreased functional activity of brain circuits mediating learning and memory such as the PFC, hippocampus, and striatum as measured by determining glucose utilization by these brain regions (74). Further memory deficits have been reported after withdrawal from nicotine, methamphetamine, and 3,4-methylenedioxymethamphetamine (MDMA) (75–79). Moreover, consistent with human studies, animals with memory deficits show significantly greater drug-seeking behavior than do controls. For example, neonatal ventral hippocampal lesions in rats, which lead to working memory deficits, resulted in increased reinstatement of nicotine seeking (80).
Box 2. Tasks used to measure psychostimulant-induced memory impairment and described in this review.
In abstinent addicts, exposure to stress, drug of abuse itself, and/or drug-associated environmental cues induces cravings, which promotes drug seeking often resulting in relapse (81–84). In humans, several behavioral and cognitive therapies, such as behavioral therapy, cue exposure therapy, motivational enhancement therapy, and contingency management, are used to help abstinent addicts overcome craving (6, 85). The main goal of all these therapies is to decrease emotional and physiological responses to drug-associated cues among abstinent addicts. In animals, extinction learning is used to suppress learned responses to drug-associated cues (86–88). Extinction learning is a form of learning that involves exposure to drug-associated cues/contexts in the absence of the drug, which ultimately leads to decreased responses to drug-associated cues/contexts. In fact, reinstatement of drug seeking in response to drug-associated cues/environments after extinction training is a putative model of relapse in humans (89, 90). Several brain regions such as the infralimbic PFC, basolateral amygdala and NAcc shell, hypothalamus, and thalamus play a role in extinction learning (88). In fact, extinction learning resulted in decrease in activity of neurons in the prelimbic PFC and increase in activity of neurons in the infralimbic PFC (91–93). It has been hypothesized that facilitation of extinction learning could help in attenuating responses to drug-associated cues and prevent relapse (94, 95). Interestingly, there is significant overlap in pathways that mediate extinction of fear-associated memories and extinction of drug-associated memories (93). In fact, currently, behavioral therapies are being used to concurrently treat both substance abuse and post-traumatic stress disorder (96). Thus, in this review where direct evidence is lacking, we suggest neural substrates that play a role in extinction of fear-associated memories as possible targets for promoting extinction of drug-associated memories. It goes without saying that any such proposed targets will need to be assessed in models assessing extinction of drug-associated memories (Box 3). In summary, treatment of psychostimulant-dependent subjects must include procognitive agents that could alleviate working memory deficits and enhance learning/memory. Importantly, facilitation of extinction learning will help improve efficacy of cognitive behavioral therapies in humans especially cue exposure therapy.
Box 3. Tasks used to assess facilitation of extinction learning.
Pharmacological Targets to Treat Psychostimulant-Induced Cognitive Impairments
Dopamine Receptors and Uptake Transporters
Changes in dopamine neurotransmission and dopamine receptors after exposure to psychostimulants like nicotine, cocaine, and methamphetamine have been previously described (97–100). Dopamine neurotransmission is primarily mediated via D1-like (D1 and D5) and D2-like (D2, D3, and D4) dopamine receptors. Most of the action of synaptic dopamine is terminated via uptake of dopamine by the dopamine uptake transporter (DAT). The dopamine uptake transporter is one of the primary targets for medications that are used to treat ADHD (101, 102). Thus, dopamine neurotransmission plays a role in both impulsivity and psychostimulant addiction. In this section, the role of D1- and D2-like dopamine receptors as possible targets for treatment of psychostimulant-induced cognitive deficits is discussed.
D1-Like Dopamine Receptors
Several studies have evaluated the role of D1-like dopamine receptors in impulsivity [see Jupp and Dalley (103) for review]. Blockade of D1 receptors alone after systemic administration of a D1 receptor antagonist had no influence on decisional impulsivity (104). However, blockade of D1 receptors after systemic administration of a D1 receptor antagonist in mice lacking DAT attenuated behavioral impulsivity as assessed using the 5-CSRTT (105). Interestingly, D1 receptors in specific brain regions such as the NAcc and PFC play a differential role in impulsivity. For example, blockade of D1 receptors in the NAcc core and shell decreased behavioral impulsivity (106). Consistent with these data, blockade of D1-like receptors in the NAcc shell attenuated reinstatement of cocaine seeking in rats (107). In contrast, blockade of D1-like receptors in the medial PFC (mPFC) induced decisional impulsivity (108). Together, these data suggest that D1-like receptors in specific brain regions and circuits may play a differential role in impulsivity. A recent study reported that mice lacking D1 receptors compared with control did not show premature responding after morphine exposure (109). However, the effects of D1 receptor activation and blockade in psychostimulant-induced impulsivity have not been investigated.
D1-mediated dopamine neurotransmission in the PFC has been shown to play a role in extinction of drug-associated memories. For example, genetically induced overexpression of D1 dopamine receptors on glutamate neurons in the PFC facilitated extinction of cocaine-induced CPP in juvenile male rats compared with controls (110) (Table 1 ; Figure 2). Activation of dopamine D1-like receptors results in increase in activity of the cAMP/protein kinase A/cyclic AMP-dependent response binding element (CREB) pathway. Rolipram, a phosphodiesterase 4 (PDE-4) inhibitor, increases cAMP levels and PKA activation that resulted in facilitation of fear extinction (116). Moreover, rolipram via an increase in CREB levels alleviated working memory deficits associated with alcohol withdrawal (117). Withdrawal from psychostimulants is also associated with decreased activity in PKA/CREB pathway especially in brain regions mediating learning/memory such the hippocampus and PFC (118, 119). Therefore, it is possible that rolipram may help facilitate extinction learning and/or working memory deficits associated with psychostimulant withdrawal. In summary, targeting D1 receptors in specific brain regions and circuits may have utility in the treatment of psychostimulant-induced cognitive deficits especially learning and memory deficits.
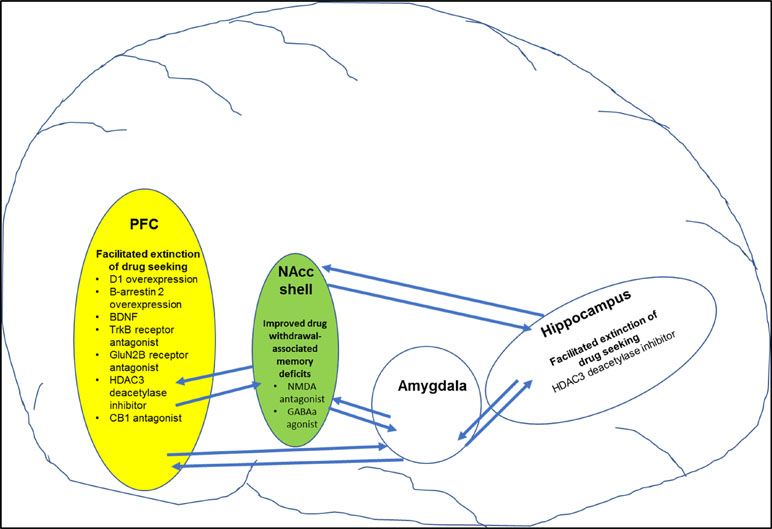
Figure 2 Figure shows specific targets in brain regions that play a role in improving drug-induced cognitive deficits (also see Table 3 for more details). For example, pharmacological manipulation of targets in the prefrontal cortex (PFC) and hippocampus facilitated extinction of drug-seeking behavior. In addition, pharmacological manipulation of targets in the nucleus accumbens shell (NAcc shell) improved drug-withdrawal associated memory.
D2-Like Dopamine Receptors
Acute cocaine dose dependently decreased decisional impulsivity in rats as assessed using the DDT (120). The same study showed that systemic administration of D2 receptor antagonist, eticlopride, reversed acute cocaine-induced inhibition of decisional impulsivity, suggesting that the effects of cocaine on decisional impulsivity are mediated by D2 receptor activation. Further, the study showed that D2 receptors in the amygdala possibly mediate the inhibitory effect of acute cocaine on decisional impulsivity. Chronic cocaine exposure decreased striatal D2 receptor mRNA in both high and low impulsive rats and selectively decreased immediate early gene zif268 mRNA in the OFC and infralimbic cortices of high impulsive animals (121). Thus, impulsive behavior observed after chronic cocaine exposure was possibly due to decreased D2-mediated dopamine signaling in the above-described brain regions.
D2 dopamine receptors located in the NAcc, ventral tegmental area (VTA), and PFC also play a role in impulsive behavior. Specifically, D2/3 receptor availability was significantly decreased in the NAcc of high impulsive rats compared with low impulsive rats (122, 123). Further chronic methylphenidate treatment decreased impulsivity in high impulsive rats by increasing expression of D2 receptor availability in the dorsal striatum and NAcc (123). Similarly, decreased D2-mediated dopamine transmission in the PFC and VTA induced decisional impulsivity (124, 125). Interestingly, systemic administration of D2 agonist ropinirole induced decisional impulsivity as assessed using the rIGT (126). However, ex vivo analyses of brain slices revealed that chronic ropinirole treatment led to upregulation of the β-arrestin-AKT-GSK3β intracellular cascade, which usually suggests D2-mediated signaling under hyperdopaminergic conditions.
Interestingly, activation of D3 receptors induced decisional impulsivity as assessed using the rIGT (109, 127–129). In contrast, blockade of D3 receptors decreased decisional impulsivity. In addition, blockade of D3 receptors attenuated cocaine and methamphetamine seeking (130, 131). Together, the data suggest that blockade of D3 receptors may help to attenuate decisional impulsivity and drug seeking. Further studies are required to assess the effects of D3 antagonists on psychostimulant-induced impulsivity.
In summary, the above-described evidence suggests that D2-mediated dopamine neurotransmission in specific brain regions such as striatum and mPFC receptors may help to alleviate decisional impulsivity associated with psychostimulant addiction (Box 4). In contrast to D2 receptors, blockade of D3 dopamine receptors may help alleviate psychostimulant-induced decisional impulsivity. Overall, D2-like dopamine receptors are useful targets in the treatment of psychostimulant addiction.
Box 4. Potential targets/approaches for alleviation of psychostimulant-induced impulsivity, memory impairment, and/or facilitation extinction of drug-associated memories. BDNF, brain-derived neurotrophic factor; CRF, corticotrophin-related factor; Trk B, tropomyosin-related kinase B; nACh, nicotinic acetylcholine; IGF, insulin growth factor; PAM, positive allosteric modulator; PPARγ, peroxisome proliferator agonist receptor gamma.
Adrenergic Receptors and Noradrenergic Reuptake Transporters
The role of noradrenaline in impulsivity is evident by use of medications that increase noradrenergic transmission in the treatment of ADHD (101, 102). Noradrenergic transmission is mediated by α (α1 and α2) and β (β1 and β2) adrenergic receptors, and the action of synaptic noradrenaline is terminated by the noradrenaline uptake transporter (NET). Several drugs approved by the FDA for ADHD treatment include α2 adrenergic receptor agonists (e.g., guanfacine and clonidine), NET and DAT inhibitors (e.g., amphetamine and methylphenidate), and selective NET inhibitor (e.g., atomoxetine). Importantly, exposure to psychostimulants like cocaine, nicotine, and methamphetamine alters noradrenergic neurotransmission in the brain (132–135). In this section the role of α2, β2, and NET in psychostimulant-induced cognitive deficits is discussed.
α2 Adrenergic Receptors and NET
Like in humans, drugs that increase noradrenergic transmission decreased impulsivity in animal models (136–138). Guanfacine, a selective α2A adrenergic receptor agonist, attenuated cocaine-induced behavioral impulsivity and memory impairment in monkeys (139) (Tables 2 and 3). More recently, it was reported that guanfacine improved inhibitory control in abstinent cocaine-dependent subjects (153). Also, α1 and α2 adrenergic receptor agonists decreased reinstatement of cocaine seeking (154). In contrast, α2 adrenergic receptor antagonist yohimbine is commonly used to pharmacologically induce reinstatement of psychostimulant drug seeking (155).
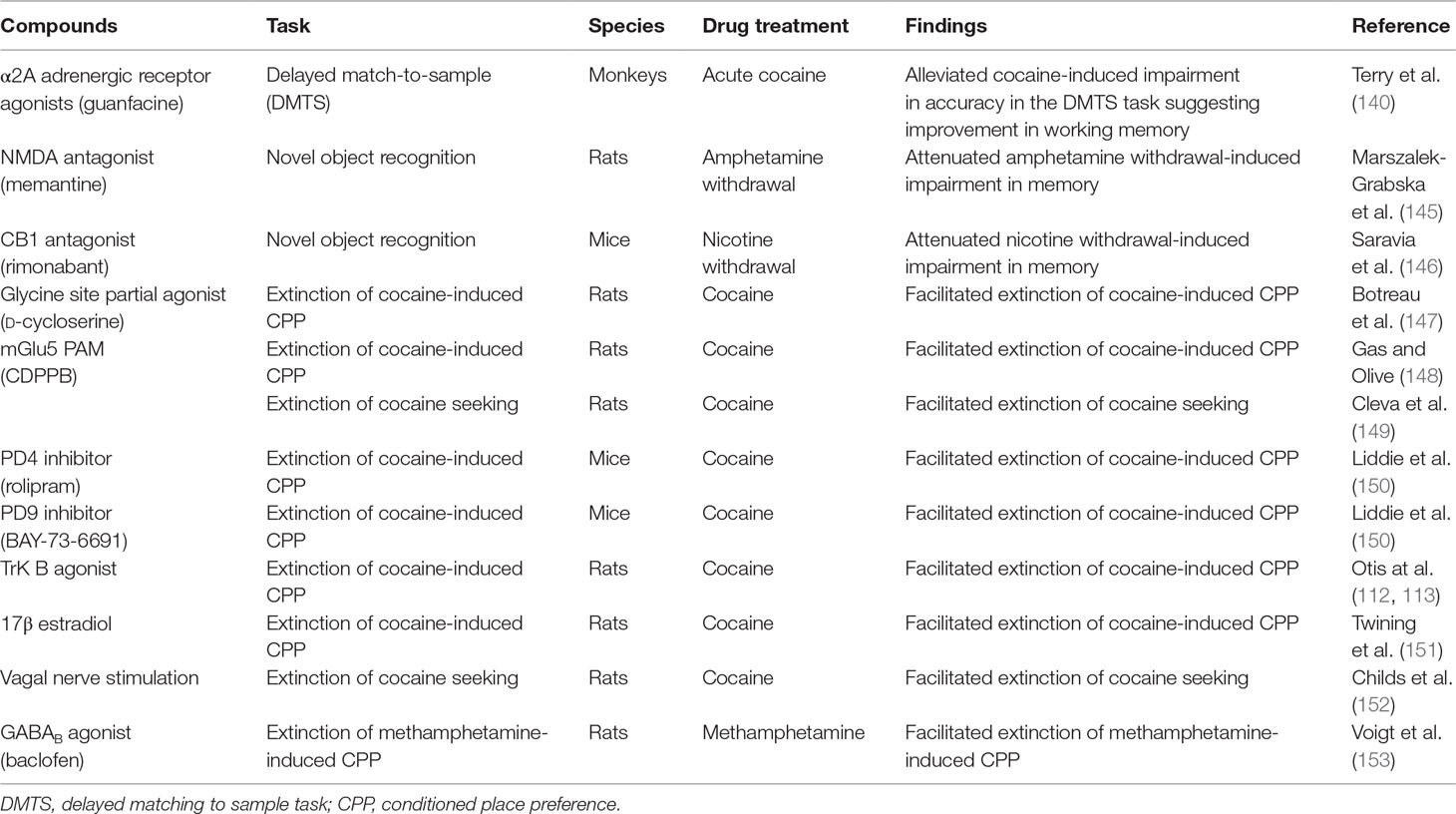
Table 3 Pharmacological alleviation of psychostimulant-induced memory impairment and/or facilitation of extinction learning.
In addition, direct injection of atomoxetine in the NAcc shell, but not NAcc core or the PFC, reduced behavioral impulsivity as assessed using the 5-CSRTT (156). However, decisional impulsivity as measured using DDT was not altered by atomoxetine injections into either the mPFC or OFC (125). Importantly, relevant to this review, atomoxetine reduced decisional impulsivity for cocaine rewards using the DDT in male rats [(144) (Table 2), but see Ref. (157)]. In contrast, atomoxetine alone did not attenuate decisional impulsivity associated with cocaine rewards in female rats. However, decisional impulsivity for cocaine rewards in females was attenuated after treatment with either progesterone alone or progesterone in combination with atomoxetine. Together, the data suggest atomoxetine may not be as effective in female compared with male cocaine abusers, suggesting a role for gender in psychostimulant-induced impulsivity treatment (discussed later). Systemic administration of atomoxetine also attenuated reinstatement of cocaine seeking (157–159). Overall, the data support the role of α2 adrenergic receptor agonists and/or selective NET inhibitors in the treatment of psychostimulant-induced impulsivity (Box 4).
β Adrenergic Receptors
The role of noradrenergic neurotransmission via β adrenergic receptors has been explored in both consolidation of drug-associated memory and extinction learning. Specifically, administration of β receptor antagonist propranolol immediately after nicotine administration attenuated reinstatement of nicotine seeking in animals (160). Consistent with these findings, administration of propranolol attenuated craving among abstinent smokers for a novel conditioned stimulus associated with nicotine. Inhibition of hippocampal β receptors attenuated expression of cocaine-associated memory as assessed using the cocaine-induced CPP model (113). Importantly, propranolol facilitated extinction of fear in rabbits (162). However, a recent study has reported that direct injections of propranolol in the infralimbic PFC attenuated extinction learning of cocaine-induced CPP via inhibition of ERK-signaling pathway (111) (Table 1; Figure 2). In fact, the study also reported that overexpression of β-arrestin 2 in the infralimbic PFC promoted extinction of cocaine-induced CPP. Further, knockout of β-arrestin 2 in the infralimbic PFC impaired extinction of cocaine-induced CPP. Taken together, the data suggest a role for β-adrenergic receptors in facilitating extinction of drug-associated memories. Further, development of β-adrenergic ligands that selectively promote signaling via β-arrestin 2 pathway will help in better understanding the role of β-adrenergic receptors in extinction learning. In summary, α2 and β adrenergic receptors and NET are very viable targets for treatment of cognitive impairments associated with psychostimulant addiction. Future work must focus on determining specific circuits that are targeted by α2 and β adrenergic receptor agonists and/or selective NET inhibitors to decrease impulsivity and facilitate working memory and/or extinction of drug-associated cues.
Serotoninergic Receptors
Alteration in serotoninergic neurotransmission after chronic exposure to cocaine and other psychostimulants has been previously described (163). Serotoninergic tone in the brain plays an important role in inhibitory control (164). Several lines of evidence suggest that a decrease in brain serotonin (5-HT) increases impulsivity, while elevation of brain 5-HT levels decreased impulsivity (165–167). Interestingly, increased 5-HT release in the PFC was found to be associated with higher levels of behavioral impulsivity as assessed using the 5-CSRTT (168, 169). Overall, a majority of the data suggest that elevation of serotoninergic transmission improves impulsive behavior.
In addition to 5-HT, several studies support a role of both 5-HT2A and 5-HT2C receptors in impulsive behavior. For example, 5-HT2A receptor expression in the mPFC was higher in high compared with low impulsive animals (170). Further, activation of 5-HT2A receptors induced behavioral impulsivity, while blockade of 5-HT2A receptors inhibited behavioral impulsivity (170, 171). Moreover, 5-HT2A receptor activation in the OFC induced decisional impulsivity (172). Future work needs to assess the effects of 5-HT2A receptor antagonists in psychostimulant-induced impulsivity. Similar to 5-HT2A, 5-HT2C receptor expression was significantly greater in the OFC in high compared with low impulsive animals (122). In contrast, no difference in 5-HT2C receptor expression was reported in the striatum between high and low impulsive animals. Blockade of 5-HT2C receptors selectively improved decisional impulsivity in the rIGT (173). Chronic cocaine self-administration decreased 5-HT2C receptor expression in the NAcc shell in the high impulsive animals but decreased 5-HT2C receptor expression in the infralimbic PFC in the low impulsive animals (122). Together, the data suggest that cocaine differentially influences 5-HT2C receptor expression in different brain regions depending on the impulsivity in the animals prior to cocaine exposure. Based on the above data, it is hypothesized that 5-HT2C receptor antagonists will attenuate psychostimulant-induced impulsivity.
5-HT3 antagonists, granisetron and ondansetron, decreased decisional impulsivity in the DDT (173) (Box 4). This decrease in decisional impulsivity was not observed after administration of the 5-HT reuptake blocker (paroxetine) or the 5-HT1A receptor agonist (8-OH-DPAT). Interestingly, infusion of 5-HT1A receptor agonist 8-OH-DPAT into the OFC decreased decisional impulsivity (124). Together, 5-HT3 receptor antagonists and 5-HT1A receptor agonists could be potentially useful in treating psychostimulant-induced impulsivity. In summary, establishing serotoninergic tone in psychostimulant-dependent subjects may help ameliorate cognitive deficits induced by abuse of psychostimulants. Further, 5-HT1A, 5-HT2A, 5-HT2C, and 5-HT3 receptors are possible targets that need to be further explored for the treatment of psychostimulant-induced impulsivity.
GABA Receptors
Exposure to psychostimulants like cocaine, nicotine, and methamphetamine alters GABAergic neurotransmission (174–176). GABA also plays a role in both impulsivity and learning/memory (177–179). Activation of GABAA receptors in the mPFC induced behavioral impulsivity, while blockade of GABAA receptors in the same region reduced behavioral impulsivity (180–182). Moreover, activation of GABAA receptors in the lateral habenula increased cue-induced cocaine seeking, suggesting lack of inhibitory control in response to drug-associated cues (183). Importantly, activation of GABAA receptors in the NAcc shell improved methamphetamine-induced working memory deficit as measured using the Morris water maze (115). Together, the data suggest that GABAA-mediated neurotransmission in different brain regions plays a differential role in psychostimulant-induced impulsivity and memory deficits (Box 4).
In addition to GABAA receptors, GABAB receptors play a role in drug seeking. For example, GABAB agonists and positive allosteric modulators (PAMs) attenuated reinstatement of nicotine and cocaine seeking (174, 184, 185). Importantly, activation of GABAB receptors facilitated extinction of methamphetamine-induced CPP (152) (Table 3). In summary, both GABAA and GABAB receptors mediate psychostimulant-induced cognitive deficits. However, further work is required to fully exploit the potential of GABAA and GABAB receptors in the treatment of psychostimulant-induced cognitive deficits.
Glutamate Neurotransmission
Dysregulation in glutamate transmission has been reported after exposure to psychostimulants (174, 186–188). Further, research has shown that both ionotropic and metabotropic glutamate (mGlu) receptors play a role in impulsivity, memory deficits, and extinction of drug-associated memories (189, 190) (Box 4).
NMDA Receptors
Systemically administered GluN2B antagonists Ro 63-1908 and traxoprodil increased premature responses in the 5-CSRTT, suggesting behavioral impulsivity (191). Similarly, systemic administration of NMDA antagonists induced decisional impulsivity as assessed using the DDT [(192, 193); but also see Higgins et al. (191)]. Together, the data suggest that blockade of NMDA-mediated glutamate transmission after systemic administration of NMDA antagonists induced behavioral and decisional impulsivity. However, blockade of NMDA receptors in specific brain regions had a differential effect on behavioral and decisional impulsivity. For example, blockade of NMDA receptors in the infralimbic PFC induced behavioral impulsivity (181). In contrast, blockade of GluN2B-containing NMDA receptors in the NAcc core induced decisional impulsivity in rats (194). Together, these data suggest that NMDA-mediated glutamate transmission in the infralimbic PFC and NAcc core plays a role in behavioral and decisional impulsivity, respectively. The effects of NMDA-antagonists and specifically GluN2B antagonists in psychostimulant-induced impulsivity still need to be assessed.
The role of NMDA-mediated glutamate transmission in learning and memory including extinction learning is well documented (189, 195, 196). Relevant to this review, systemic administration of NMDA antagonist memantine improved amphetamine withdrawal-induced memory deficit as assessed using the novel object recognition test (143) (Table 3). Similarly, blockade of NMDA receptors in the NAcc using NMDA antagonist AP-5 improved methamphetamine-induced working memory deficit as measured using the Morris water maze (115) (Table 1; Figure 2). Interestingly, increasing activity of NMDA-mediated glutamate transmission via manipulation of the glycine site facilitated extinction of fear- and cocaine-associated memories (145, 197, 198) (Table 3). In addition, increased NMDA-mediated transmission especially via GluN2B-containing NMDA receptors facilitated extinction of fear memories (199). Consistent with these findings, increasing glutamate transmission via GluN2B-containing NMDA receptors in the infralimbic cortex facilitated extinction of cocaine-associated memory (112) (Table 1; Figure 2). More recently, it was reported that aquaporin-4 (AQP-4) deletion increased GluN2B-mediated glutamate transmission in the CA3–CA1 hippocampal pathway (200). AQP-4 is the predominant water channel primarily expressed in astrocytes and plays a role in regulating synaptic plasticity. Importantly, deficiency of AQP-4 facilitated fear memory extinction (200). Future work must investigate if AQP-4 may be a potential target for facilitating extinction of psychostimulant drug-associated memories. In summary, NMDA receptors could serve as targets for alleviation of psychostimulant-induced impulsivity and memory deficits. Furthermore, NMDA receptors could be targeted to facilitate extinction of drug-associated memories.
AMPA Receptors
The AMPA receptors are also involved in extinction learning. For example, AMPA receptor activation facilitated extinction of fear-associated memories (199, 201). Activation of AMPA receptors in the infralimbic PFC facilitated extinction of heroin-associated memories (202). However, the effects of AMPA receptor activation on extinction of psychostimulant-associated memories have not been evaluated. Surface expression of AMPA receptors can be regulated by a process called ubiquitination. Ubiquitination of AMPA receptors results in internalization of AMPA receptors, which indirectly decreases AMPA-mediated glutamate transmission. More recent work has shown that ubiquitination of AMPA receptors is partially regulated by epidermal growth factor receptor substrate 15 (Eps15). Decreased expression of Eps15 resulted in decreased internalization of GluA1-containing AMPA receptors possibly by decreased ubiquitination of GluA1 subunits of the AMPA receptors (204). However, further work is required to determine if knockdown of Eps15 facilitates extinction learning via decreased internalization of AMPA receptors. In summary, Eps15 via AMPA-mediated glutamate transmission could be a potential target to facilitate extinction learning of psychostimulant-associated memories.
Metabotropic Glutamate (mGlu) Receptors
Several experimental studies support the role of mGlu receptors in cognitive deficits. For example, blockade of mGlu1 receptors resulted in decisional impulsivity (195). These data suggest that PAMs of the mGlu1 receptors may help to reduce decisional impulsivity, although this hypothesis needs to be experimentally tested in psychostimulant-induced impulsivity. In addition to mGlu1 receptors, mGlu2/3 receptors also mediate behavioral and decisional impulsivity. Pretreatment with the mGlu2/3 agonist LY379268 attenuated 5-HT2A agonist-induced behavioral impulsivity (205). Furthermore, direct injections of mGlu2/3 agonist in the OFC and mPFC attenuated intra-OFC and intra-PFC 5-HT2A agonist-induced decisional and behavioral impulsivity, respectively (172). Moreover, systemic administration of mGlu4 PAM, 4-((F)-styryl)-pyrimidin-2-ylamine (Cpd11), induced behavioral impulsivity but decreased decisional impulsivity (206). In contrast to mGlu4 receptors, blockade of mGlu5 receptors, using a mGlu5 negative allosteric modulator (NAM), attenuated behavioral impulsivity (207). In addition, activation of mGlu5 receptors, using a mGlu5 receptor PAM, attenuated NMDA antagonist MK-801-induced behavioral impulsivity. Interestingly, no effects of mGlu5 receptor modulation on decisional impulsivity were observed (207). Importantly, systemic administration of mGlu5 PAM, CDPPB, facilitated extinction of fear- and cocaine-associated memories (148, 149, 208) (Table 3). Consistent with these findings, decreased glutamate transmission via mGlu1 and mGlu5 receptors in the mPFC facilitated resistance to extinction of cocaine-associated memories in animals with chronic cocaine self-administration experience (209). Taken together, the data suggest that mGlu5 receptors have a role in behavioral impulsivity and can also be targeted to facilitate extinction of psychostimulant-associated memories. Based on the above-described data, mGlu1, mGlu2/3, mGlu4, and mGlu5 receptors can serve as potential targets in psychostimulant-induced impulsivity.
Drugs That Restore Glutamatergic Tone
As described above, dysregulation in glutamate transmission has been reported after exposure to psychostimulants. Thus, agents that restore glutamatergic tone may help to treat psychostimulant addiction. Administration of riluzole, a pharmacological compound that reestablishes glutamatergic tone, decreased activity of the prelimbic PFC and increased activity of the infralimbic PFC (210). Also, direct injections of riluzole in the amygdala facilitated extinction of fear-associated memories, possibly due to the increase infralimbic PFC activity (211). Importantly, riluzole attenuated reinstatement of cocaine seeking (210). Like riluzole, N-acetylcysteine, a cystine–glutamate antiporter that helps restore glutamatergic tone, attenuated reinstatement of cocaine and nicotine seeking (212, 213). N-Acetylcysteine also reduced reinstatement of nicotine seeking observed in animals with neonatal ventral hippocampal lesions (80, 214). As described above, animals with neonatal ventral hippocampal lesions show memory deficits and higher nicotine seeking than do controls. It is hypothesized that memory deficits associated with ventral hippocampal lesions are partially responsible for this increased nicotine seeking. Together, the data suggest that N-acetylcysteine helps animals overcome memory deficits and thus possibly helps reduce drug seeking. Overall, the above data with riluzole and N-acetylcysteine suggest that correcting the dysregulation in glutamate transmission can improve memory deficits and/or facilitate extinction learning. Future work needs to determine if these drugs can facilitate extinction of drug-associated memories. In summary, both ionotropic and metabotropic glutamate receptors are viable targets for treatment of psychostimulant-induced cognitive deficits. However, more work is required to understand glutamate dysregulation in specific brain circuits after psychostimulant exposure to fully exploit the various glutamate targets for treatment of psychostimulant-induced cognitive deficits.
Nicotinic Acetylcholine Receptors (nAChRs)
The role of nAChRs in impulsive behavior has been discussed previously (215). In humans, polymorphism in the α4 subunits of the nAChRs (CHRNA4) was associated with pathological gambling in Korean gamblers (216). Also, systemic administration of varenicline, a partial agonist of α4β2 nAChRs, induced behavioral impulsivity in animals (217). Importantly, blockade of α4β2 nAChRs in the infralimbic PFC attenuated varenicline-induced behavioral impulsivity, suggesting that the effects of varenicline are mediated by α4β2 nAChRs in the infralimbic PFC (218). Also, intra-cerebroventricular injection of α4β2 nAChR antagonist decreased behavioral impulsivity in animals (219). Together, the data highlight that the role of α4β2 nAChRs in behavioral impulsivity and α4β2 nAChR antagonists may help to alleviate behavioral impulsivity. More recently, compounds that decrease signaling via α4β2 nAChRs attenuated cocaine and methamphetamine seeking (220). In addition, systemic administration of partial agonists of the α7-containing nAChRs decreased behavioral impulsivity and improved attention as assessed using the five choice-continuous performance task (5-CCPT) (221). The decrease was specifically observed in female rats that had been classified as animals with low attention at baseline. α7 nAChR agonists have also been shown to improve memory (222, 223). Together, the data suggest a possible role for α4- and α7-containing nAChRs in cognitive deficits such as impulsivity and impairment of memory. However, the role of the different nAChR subunits in psychostimulant-induced cognitive deficits is yet to be evaluated.
Opioid Receptors
Several lines of evidence support the role of endogenous opioids in impulsive behavior. For example, human imaging studies suggest upregulation of µ opioid receptor (MORs) in the mPFC and OFC in individuals with traits suggestive of impulsivity (223). Further, pathological gamblers, who are known to be impulsive and impaired in making rational decisions, show decreased endogenous opioid release in the brain than do healthy volunteers (224). Consistent with these findings, administration of MOR antagonist decreased pathological gambling (225, 226). MORs and opioid peptides are extensively found in PFC and regulate PFC neuronal activity (227). Activation of MORs in the PFC induced behavioral impulsivity (228). In addition, mice lacking MORs showed markedly decreased behavioral impulsivity (229). In contrast, the same study showed that mice lacking delta opioid receptors (DORs) showed increased behavioral impulsivity. More recently, it was reported that α2 agonist yohimbine-induced increase in behavioral impulsivity was attenuated by blockade of kappa opioid receptors (KORs) (230). Interestingly, KOR activation on its own decreased behavioral impulsivity possibly due to impairment of motor activity. Together, the data from these pharmacological and genetic studies suggest a differential role for MORs, DORs, and KORs in behavioral impulsivity. Further, the data suggest that MOR and KOR antagonists may help to reduce impulsivity.
Chronic cocaine administration is associated with upregulation of MORs and KORs in the PFC (231). Furthermore, upregulation of MORs in the anterior cingulate cortex predicts both severity of craving and relapse in cocaine users (232, 233). Similarly, dysregulation of endogenous opioid neurotransmission occurs after exposure to nicotine (234). However, much work needs to be done in determining the role of MORs, MOR antagonists, and other opioid receptors in psychostimulant-induced cognitive deficits especially impulsivity (Box 4). Moreover, most of the research on the role of opioid receptors in impulsivity has focused on the PFC. However, further work must be carried out in other brain regions to determine the role of endogenous opioids in psychostimulant-induced impulsivity.
Cannabinoid Receptors
The endogenous cannabinoid system is altered after exposure to psychostimulant drugs. For example, exposure to cocaine administration during adolescence increased expression of CB1 receptors and decreased expression of CB2 receptors in the PFC and hippocampus (235). In contrast in adult rats, chronic cocaine self-administration resulted in decreased CB1 and CB2 receptor expression in the PFC, dorsal striatum, and amygdala (236). Further, blockade of CB1 receptors attenuated both cocaine intake and reinstatement of cocaine seeking (237). Importantly, blockade of CB1 receptors prevented cocaine-induced impairment in decisional impulsivity as assessed using the DDT (39) (Table 2). In addition, acute administration of CB1 antagonists prior to DDT in cocaine-treated rats reversed cocaine-induced decisional impulsivity. Together, these data support a role for endogenous cannabinoids in both preventing and reversing cocaine-induced impulsivity. Consistent with the findings of this study, activation of CB1 receptors using cannabidiol (CBD) did not improve impulsivity during tobacco abstinence in human smokers (238).
In addition, CB1 receptors located in the amygdala and hippocampus play a role in learning and memory. For example, blockade of CB1 receptors attenuated nicotine withdrawal-induced memory deficits (144) (Table 3). Interestingly, the same study also showed that selective deletion of CB1 receptors in the GABA neurons also mitigated nicotine withdrawal-induced memory deficits. Overall, these data suggest that CB1 receptor antagonists may have therapeutic utility in promoting smoking cessation by decreasing memory deficits associated with nicotine withdrawal. Importantly, systemic or intra-mPFC administration of CB1 receptor antagonist rimonabant enhanced extinction of cocaine-associated memories (114) (Table 1; Figure 2). Together, these data suggest that CB1 receptor antagonists could be potentially used to treat psychostimulant-induced impulsivity and memory impairment (Box 4). However, further research is required to fully exploit the potential of the endocannabinoid system as a potential treatment for psychostimulant-induced cognitive deficits.
Phosphodiesterase Inhibitors
The role of cAMP/protein kinase A/cyclic-AMP response element binding (CREB) protein pathway plays an important role in both memory and reinforcing effects of psychostimulant drugs (239–241). The enzyme phosphodiesterase (PDE) plays a role in breakdown of cAMP and thus indirectly decreases CREB formation. Phosphodiesterase inhibitors, which increase CREB formation, facilitate learning and memory (242). For example, subchronic administration of rolipram, a PDE4 inhibitor, using osmotic pumps facilitated learning of conditioned fear (243). Importantly, more recently it has been reported that rolipram facilitated extinction of fear-associated memory in mice (116). Interestingly, PDE4 inhibitors did not facilitate extinction of cocaine-induced CPP (148) (Table 3). However, the same study showed that PDE9 inhibitor BAY-73-6691 facilitated extinction of cocaine-induced CPP (Table 3). This effect of PDE9 inhibitor was possibly mediated by an increase in cGMP levels in the hippocampus and amygdala. Further work is required to assess the effects of PDE9 inhibitors and other PDE inhibitors in facilitation of extinction of drug-associated memories. In summary, the various isoforms of the PDE enzyme continue to be viable targets for treatment of psychostimulant addiction.
Orexin
Orexin neurons (also referred to as hypocretin) are found in the hypothalamus and release the neuropeptides orexin A and orexin B (also referred to as hypocretins 1 and 2) throughout the CNS (244). With its widespread targets, the orexin system is involved in a number of functions including stress, reward, wakefulness, and food seeking (245). The hypocretin/orexin system plays an important role in the reinforcing effects of cocaine. For example, suvorexant, a dual orexin receptor antagonist, attenuated both the rewarding and motivational effects of cocaine (141). Also, knockdown of hypocretin/orexin neurons in the dorsal hypothalamus attenuated cocaine self-administration (246). Further knockdown of orexin 1 receptor in the VTA both altered dopamine signaling in the NAcc and attenuated cocaine-induced increase in NAcc DA (248). The hypocretin/orexin system also plays a role in opioid- and alcohol-dependent behaviors (249, 250).
Increased activation of medial hypothalamic orexin neurons, but not lateral hypothalamic neurons, was reported during a Go/No-Go task involving food reward, suggesting a role for medial hypothalamic orexin neurons in behavioral impulsivity (251). More recently, systemic or intra-VTA administration of suvorexant, a dual orexin receptor antagonist, attenuated cocaine-induced behavioral impulsivity (140) (Table 2). Interestingly, neither suvorexant nor orexin 1 (SB334867) nor orexin 2 (TCS-OX2-29) receptor-selective compounds altered decisional impulsivity. Taken together, the data suggest that orexin receptor antagonists may be useful in reducing psychostimulant-induced behavioral impulsivity.
The hypocretin/orexin receptors are also found in brain regions that play a role in memory especially the hippocampus. Administration of orexin peptides increased firing of hippocampal neurons and facilitated learning and memory (252–256). The orexin-induced facilitation of learning is mediated by increasing neurogenesis in the hippocampus (257). The hypocretin/orexin system also plays a role in extinction learning. For example, blockade of orexin 1 receptor facilitated extinction of fear-associated memories possibly by increasing amygdalar input to the infralimbic PFC during extinction learning (258). However, the role of the orexin system in facilitation of extinction of drug-associated memories has not been explored. In summary, blocking orexin-mediated signaling decreased behavioral impulsivity and facilitated extinction learning (Box 4). The hypocretin/orexin system is a very promising target, but further work is required to fully exploit the orexin system for the treatment of psychostimulant-induced cognitive deficits.
Brain-Derived Neurotrophic Factor (BDNF)
BDNF, a neurotrophin, is extensively distributed in the brain (259). BDNF plays a role in psychostimulant-induced behavioral effects. Inhibition of BDNF signaling and/or decreased expression of BDNF attenuated the rewarding effects of cocaine and cocaine-seeking behaviors (260–262). Methamphetamine withdrawal was associated with elevated BDNF levels in the dorsal striatum (263). In addition, genetically induced depletion of BDNF expression resulted in social cognitive deficits after chronic methamphetamine treatment compared with controls (264). Impaired BDNF signaling in the frontal and striatal regions during nicotine withdrawal was also associated with cognitive deficits (265). Together, the data suggest that decreased BDNF signaling possibly mediates psychostimulant-induced cognitive deficits.
Importantly, increase in BDNF signaling plays a role in consolidation of both recognition and spatial memory (266). Intracerebroventicular injection of antibodies to BDNF attenuated spatial learning in rats (267). Increase in BDNF signaling was also associated with extinction of fear-associated memories (268, 269). Interestingly, infusing BDNF into the ventral hippocampus increased the firing rate of neurons in the infralimbic PFC, which plays an important role in extinction learning (270). Importantly, increased BDNF signaling via stimulating tropomyosin-related kinase B (Trk B) receptors in the infralimbic PFC facilitated extinction of cocaine-induced CPP (112) (Table 1; Figure 2). Also, the study showed that systemic administration of Trk B receptor agonist facilitated extinction of cocaine-associated memories (Table 3). Overall, receptors mediating BDNF signaling are promising targets for facilitation of extinction of drug-associated memories and could be used for advancing treatment of psychostimulant addiction (Box 4). However, further work is required to understand BDNF signaling in specific circuits to maximally exploit its receptors as a therapeutic target.
Corticotrophin Releasing Factor (CRF) Receptors
The role of CRF receptors in the behavioral and rewarding effects of psychostimulants has been previously reviewed (271–273). Blockade of CRF1 and CRF2 receptors in the VTA attenuated the reinforcing effects of cocaine (274). Interestingly, the rewarding effects of cocaine were enhanced in mice lacking CRF1 receptors compared with wild-type controls (275). Importantly, chronic cocaine administration induced memory deficits in wild-type mice but not in CRF1 deficient mice (276). In addition, cocaine withdrawal-induced memory deficits were observed in CRF2-deficient mice compared with wild-type controls (277). These data suggest that CRF receptors mediate cocaine withdrawal-induced impairment of memory and other cocaine-dependent effects.
CRF receptors are extensively distributed in brain regions that play a role in learning and memory (278). Blockade of CRF receptors, using CRF antagonist d-Phe-CRF, improved cognitive performance (279). Further, the study also showed that blockade of CRF1 receptors using CRF1 selective antagonist NBI 35965 improved memory in PFC-dependent tasks. Taken together, the data suggest that CRF receptors can be targeted to alleviate psychostimulant-induced memory deficits.
Importantly, CRF receptors in the VTA play a role in reinstatement of cocaine seeking (280). In addition, the same study showed that after cocaine self-administration and extinction training, stimulation of CRF2 receptors in brain slices resulted in increased glutamate release and decreased GABA release as compared in cocaine-naïve animals. These data suggest that extinction training and cocaine exposure altered CRF2-mediated transmission. Importantly, infusions of the CRF receptor antagonist α-helical CRF(9-41) into the basolateral amygdala enhanced extinction of fear-associated memories (281). However, further work needs to be carried out to determine if CRF receptors play a role in extinction of psychostimulant drug-associated memories. In summary, CRF receptors could serve as a potential target to alleviate psychostimulant-induced memory deficits and/or promote extinction of drug-associated memories.
Non-Pharmacological Approaches for Treatment of Psychostimulant-Induced Cognitive Deficits
Brain Stimulation
Brain stimulation can be achieved using a variety of different approaches such as transcranial magnetic stimulation, deep brain stimulation (DBS) using intracranial electrodes, transcranial direct current stimulation, and vagus nerve stimulation (282). Evidence from both human and animal studies supports use of brain stimulation to ameliorate cognitive deficits and improve learning and memory. For example, in humans, increase in verbal working memory accuracy was observed following transcranial magnetic stimulation (283). Similarly, DBS of the ventromedial PFC resulted in improvement in novel object recognition memory compared with that in controls in animals (284). Intracranial DBS also improved spatial memory in rats as assessed using the Morris water maze task (285). Also, DBS facilitated extinction of fear-associated memories (286, 287).
More importantly, DBS using intracranial electrodes attenuated reinstatement of cocaine seeking (288). Also, low-frequency DBS, but not high-frequency DBS, of the ventral striatum strengthened extinction of morphine-associated memories in rats (289). In addition, low-frequency stimulation of the ventral striatum was accompanied by an increase in immediate early gene c-fos synthesis in brain regions associated with extinction such as the infralimbic PFC and amygdala, suggesting increased activity of these regions. Importantly, vagal nerve stimulation during extinction training improved rates of extinction and reduced reinstatement of cocaine seeking in rats (151) (Table 3). Interestingly, DBS of subthalamic nucleus and vagal nerve stimulation also helped in decreasing decisional impulsivity in “risk preferring” rats compared with controls (290, 291).
Together, these data suggest that brain stimulation can help in both decreasing impulsivity and facilitating extinction of psychostimulant-associated memories. Thus, brain stimulation has the potential to alleviate multiple cognitive deficits. Future work must focus on identifying precise neural substrates and brain stimulation parameters to fully exploit the benefits of brain stimulation in psychostimulant addiction treatment. Furthermore, identification of pharmacological compounds that will help in improving efficacy of brain stimulation in addiction treatment will also be very useful.
Exercise
Exercise in animals influences psychostimulant-dependent behavioral effects. For example, exercise attenuated reinstatement of cocaine seeking after a period of abstinence (292, 293). In addition, reinstatement of cocaine seeking in high impulsive rats was attenuated when animals were treated with a combination of atomoxetine and exercise during withdrawal from cocaine compared with either treatment alone (294). Importantly, post-extinction exercise training was more effective than extinction alone in attenuating reinstatement of cocaine seeking (295).
Exercise in the form of wheel running and swimming has been shown to improve learning and memory (296). Consistent with these findings, exercise using a treadmill attenuated morphine withdrawal-induced memory deficit in rats (297). Also, exercise facilitated extinction of fear-associated memories (298, 299). However, it is not known if exercise facilitates extinction of psychostimulant-associated drug memories. Further, effects of exercise on amelioration of psychostimulant withdrawal-associated memory deficits have not been explored. Several questions such as intensity and duration of exercise, neural changes as a consequence of exercise, and optimal combination of exercise with pharmacological medications need to be determined to use exercise most efficaciously as a tool for psychostimulant addiction treatment.
Promoting Neurogenesis
Psychostimulant exposure impairs neurogenesis in the hippocampus in adult animals. For example, chronic exposure to nicotine, methamphetamine, and cocaine altered/blunted neurogenesis in the hippocampus (300–303). In addition, cocaine withdrawal-induced memory deficits were associated with blunted neurogenesis in the hippocampus (304). Hippocampal neurogenesis has been shown to play a role in consolidation of memory (305). Also, disruption of adult hippocampal neurogenesis impaired short- and long-term memory formation (306).
Relevant to this review, enhancing neurogenesis facilitated extinction of fear-associated memories (307, 308). Furthermore, pharmacological facilitation of neurogenesis facilitated extinction of morphine-associated memory (309). Importantly, increasing hippocampal neurogenesis in adult animals using chronic intracerebroventricular infusions of lysophosphatidic acid (LPA; an endogenous lysophospholipid with pro-neurogenesis effects) facilitated extinction of cocaine-associated memories (310). In contrast, suppression of neurogenesis using cranial irradiation resulted in resistance to extinction of cocaine seeking (311). Together, the above data suggest that pharmacological manipulation of adult hippocampal neurogenesis could facilitate extinction of drug-associated memories (Box 4). In summary, promoting neurogenesis can serve as an important strategy to treat psychostimulant addiction. However, future research must focus on understanding cellular mechanisms that underlie psychostimulant-induced impairment of hippocampal neurogenesis and identify pathways that can promote neurogenesis. Together, both of the above-described approaches will help to effectively treat psychostimulant-induced cognitive deficits.
Future Directions
Ghrelin
Ghrelin is an orexigenic peptide hormone acting on receptors in both the brain and periphery (312). Modulation of ghrelin altered effects of psychostimulants. For example, administration of ghrelin enhanced the rewarding effects of cocaine (313). Consistent with these findings, blockade of ghrelin-mediated transmission attenuated behavioral effects of cocaine, amphetamine, and nicotine (314, 315). In cocaine-experienced animals, during early withdrawal, ghrelin levels were elevated possibly in anticipation of cocaine (316). Similarly, in abstinent smokers, elevated ghrelin levels were associated with increased craving and relapse (317).
More importantly, ghrelin is neuroprotective, promotes hippocampal neurogenesis, and enhances learning and memory (318–320). Elevation of ghrelin levels as a consequence of food deprivation facilitated extinction of fear-associated memories, possibly by inhibition of long-term depression in the lateral amygdala (321). Consistent with these findings, a human clinical study reported facilitated extinction of fear-associated memories in subjects that had increased ghrelin levels as a result of overnight fasting (322). Based on these data, it is hypothesized here that increasing ghrelin-mediated signaling during extinction training may facilitate extinction of drug-associated memories. However, experimental data supporting this hypothesis are currently lacking. Besides, the precise mechanism of how ghrelin facilitates learning still needs to be explored. Nevertheless, there exists strong rationale for assessing the effects of ghrelin in extinction of drug-associated memories. Finally, based on the above data, it appears that elevated ghrelin levels are associated with craving in abstinent drug-dependent individuals, facilitation of extinction learning, and neuroprotection/neurogenesis. It is possible that ghrelin in different brain regions may have a differential role. Future work may need to understand the role of ghrelin in specific brain circuitries to fully exploit the therapeutic potential of ghrelin.
Oxytocin
Oxytocin is synthesized by hypothalamic nuclei such as the supraoptic, parvocellular, and accessory nuclei. Oxytocin-containing neurons from these nuclei primarily project to posterior pituitary, but they also innervate brain regions mediating reward and emotion such as the PFC and amygdala (323). Systemic administration of oxytocin attenuated reinstatement of cocaine and methamphetamine seeking (324, 325). Consistent with this study, direct injection of oxytocin in the NAcc attenuated methamphetamine-induced CPP (326). Together, these data suggest that activation of oxytocin receptors attenuated drug-associated memories. Additionally, cocaine withdrawal was associated with increased oxytocin receptor binding in the piriform cortex, lateral septum, and amygdala (327).
Oxytocin receptors are extensively found in the PFC (328). Interestingly, activation of oxytocin receptors in the infralimbic PFC facilitated extinction of fear-associated memories (329, 330). Further social cues, such as presence of an animal, during extinction learning increased PFC oxytocin transmission (330). Overall, the data suggest that oxytocin receptor activation in the PFC facilitated extinction learning. However, the effects of increased oxytocin transmission on extinction of drug-associated memories have not been investigated. Together, these findings suggest that changes in oxytocin transmission may mediate some of the emotional and cognitive deficits associated with cocaine use. Based on the above-described findings, oxytocin receptors may serve as useful targets for the treatment of psychostimulant addiction, especially in promoting extinction of drug-associated memories (Box 4).
Vasopressin
Vasopressin and its receptors play a role in psychostimulant-dependent behavioral effects. For example, elevated levels of vasopressin mRNA in the amygdala were observed in animals during withdrawal from cocaine (331). Additionally, blockade of vasopressin 1a receptors in the NAcc during conditioning attenuated expression of cocaine-induced CPP. Blockade of vasopressin 1b receptor also attenuated reinstatement of methamphetamine-induced CPP (332). Finally, blockade of vasopressin 1a receptors reversed oxytocin-induced attenuation of reinstatement of methamphetamine seeking (325). Together, the above evidence suggests a role for vasopressin in cocaine- and methamphetamine-dependent behavioral effects.
Vasopressin neurons and receptors are extensively found in brain regions involved in learning and memory such as the hippocampus, PFC, and amygdala (333–335). Knockout of vasopressin 1b receptor impaired hippocampal-dependent memory tasks (336). Vasopressin also plays an important role in social memory (337). Furthermore, blockade of vasopressin 1b receptor attenuated stress-induced impairment of memory (338). Elevated levels of vasopressin mRNA in the amygdala were also reported in animals showing high predisposition to stress-induced reinstatement of heroin seeking (339). A recent study has suggested that vasopressin may be involved in risky behaviors in humans, which suggest that it may have a role in impulsivity (340). In summary, the above data suggest that vasopressin-mediated neurotransmission is involved in memory and drug-dependent effects. Although still early, vasopressin receptors may serve as targets for treatment of psychostimulant-induced cognitive deficits.
Protein Kinase Cε
PKCɛ is extensively found in the brain and is a downstream mediator of G-protein receptor signaling (341). Recent studies suggest that PKCɛ possibly mediates the reinforcing effects of psychostimulants like nicotine and cocaine. For example, mice lacking PKCɛ showed reduced mRNA levels of α6 and β3 nAChR subunits in brain regions associated with drug reward such as the VTA and striatum (342). Consistent with these findings, knockout of PKCɛ reduced nicotine-induced CPP and attenuated nicotine self-administration compared with wild-type controls. Relevant to this review, the infralimbic PFC showed elevated levels of PKCɛ after with withdrawal from extended cocaine self-administration experience (343). More importantly, inhibition of PKCɛ in the infralimbic PFC attenuated reinstatement of cocaine seeking. However, the effects of PKCɛ expression in the infralimbic PFC on extinction learning have not been assessed.
Activation of PKCɛ facilitates learning and memory (344, 345). In fact, inhibition of PKCɛ using peptides that directly bind to PKCɛ attenuated recognition memory as assessed using novel object recognition task (345). It is postulated that the memory-enhancing effects of PKCɛ activation are mediated via increased activity of ERK1/2 in the hippocampus. Together, the above data suggest that activation of PKCɛ could be useful in facilitating extinction of drug-associated memories. Based on the role of PKCɛ in memory and cocaine-dependent behaviors, it is hypothesized that PKCɛ may be an attractive target for treating psychostimulant addiction by promoting extinction learning.
Peroxisome Proliferator-Activated Receptor γ (PPARγ) Receptors and Insulin
Insulin and PPARγ agonists influence the behavioral and psychological effects of drugs of abuse. For example, a recent double-blind randomized study reported that patients receiving PPARγ agonist pioglitazone compared with placebo reduced cocaine craving and improved brain white matter integrity in cocaine-dependent patients (346). In animals with cocaine self-administration experience, insulin levels were reduced by approximately 40–70% during cocaine self-administration (316). In addition, intra-VTA injections of insulin attenuated cocaine-induced increase in NAcc dopamine and decreased cocaine-induced increase in locomotor activity (347).
Additionally, insulin and PPARγ agonists play a role in alleviating memory deficits. For example, systemic administration of insulin and insulin-growth factor 2 (IGF-2) facilitated learning and memory (348, 349). Additionally, intranasal insulin administration improved memory in patients with either mild cognitive impairment or early Alzheimer’s disease (350). Further, PPARγ agonists improved memory in some humans with early Alzheimer’s disease (351). These memory-enhancing effects of PPARγ agonists are possibly mediated by actions of PPARγ agonists on hippocampal dentate neurons (352, 353). In summary, both insulin and PPARγ play a role in cognition and memory and could influence the behavioral effects of psychostimulants.
Importantly, PPARγ agonist pioglitazone attenuated alcohol-induced spatial memory deficit as assessed using the Morris water maze (354). Additionally, pioglitazone attenuated drug-induced heroin seeking (355). Finally, increased IGF-2-mediated transmission in the hippocampus facilitated extinction of fear-associated memories (356). It is hypothesized that this IGF-2-mediated facilitation of extinction occurs via stimulation of neurogenesis (357). However, it is not known if insulin and PPARγ agonists could facilitate extinction of drug-associated memories? Could insulin and PPARγ agonists be used to ameliorate psychostimulant withdrawal-induced memory impairment? Future work will need to address these and other questions.
Enzymes Involved in Epigenetic Changes
Epigenetic changes occur as a consequence of behavioral activity, learning, and/or drug exposure (358). In fact, enzymes involved in epigenetic DNA changes are involved in psychostimulant and non-psychostimulant drug-associated memories. For example, DNA methylation via chronic l-methionine (MET) attenuated reinstatement of cocaine seeking (359). Also, knockdown of histone methyltransferase PR containing domain 2 (PRDM2) in the dorsomedial PFC using viral vectors enhanced stress-induced reinstatement of alcohol seeking (360). Genetically induced loss of histone acetyltransferase CREB-binding protein (CBP) in the NAcc attenuated cocaine-induced CPP.
Activity-dependent epigenetic changes play an important role in learning and memory consolidation (361). Importantly, the enzymes that mediate these epigenetic changes could be targeted to facilitate learning and memory. For example, blocking of histone deacetylase (HDAC3) enzyme activity in the dorsal hippocampus enhanced long-term memory for object location (114). Additionally, manipulation of enzymes involved in epigenetic changes facilitated extinction learning. For example, inhibition of histone acetyltransferase (HAT) p300 enzyme, which is highly expressed in pyramidal neurons of the infralimbic PFC, facilitated extinction of fear-associated memories (362). Importantly, blocking of HDAC3 deacetylase activity in the dorsal hippocampus, but not the infralimbic PFC, facilitated extinction of cocaine-associated memories (114) (Table 1; Figure 2). Overall, these data suggest that enzymes involved in epigenetic changes could play a role in facilitation of extinction of psychostimulant-associated memories. More generally, they could also play a role in the treatment of cognitive deficits associated with psychostimulants such as impulsivity and memory impairments. However, much work remains to not only identify specific enzymes but also to identify specific brain regions where these enzymes are actively involved in psychostimulant-induced cognitive deficits.
MicroRNAs (miRs)
The role of non-coding microRNAs (miRs) has been implicated in psychostimulant-dependent behaviors. For example, methyl CpG binding protein 2 (MeCP2) and miR-212 in the dorsal striatum play a role in regulating escalation of cocaine intake in rats with extended access to cocaine (363). Further, upregulation of miR-212 and miR-132 in the dorsal striatum persisted for approximately 10 days after withdrawal of cocaine (364). Similarly, miR-496-3p, miR-194-5p, miR-200b-3p, and miR-181a-5p were upregulated significantly following methamphetamine exposure (365). Together, the data suggest that exposure to psychostimulants alters expression of microRNAs.
The role of non-coding miRs has been implicated in cognitive processes such as impulsivity, learning, and memory. For example, several miRs in the amygdala such as miR-190b, miR-28a, miR-340, miR-219a, and miR-491 have been reported to correlate with inhibitory control (366). Thus, theoretically decreased expression of these miRs could result in impulsive behaviors, although direct experimental evidence for this hypothesis is currently lacking. Similarly, miR-641, which binds to SNAP-25 gene, has been implicated in impulsive behaviors (367). In addition, miR-183-96-182 has been associated with comorbid ADHD and drug addiction (368). Together, these data suggest that miRs play a role in regulating impulsive behavioral traits.
miRs also play a role in memory (369). For example, inhibition of miR-9-3p resulted in deficits in hippocampal-dependent tasks (370). Overexpression of miR-144-3p in the basolateral amygdala facilitated extinction of fear-associated memories in C57BL/6 mice (371). In addition, the same study showed that overexpression of miR-144-3p in the basolateral amygdala rescued extinction of fear memories in S1 mice, which show resistance to extinction of fear memories. Similarly, extinction training after fear conditioning trials resulted in increase in expression of miR-128b in the infralimbic PFC, and overexpression of miR-128b in the infralimbic PFC facilitated extinction of fear-associated memories (372). Importantly, significant increases in the expression of miR-101b, miR-137, miR-212, and miR-132 in NAcc shell and miR-137 in the dorsal striatum were observed after extinction training and reinstatement of cocaine seeking in rats (373). Future studies must focus on brain regions associated with extinction learning such as the basolateral amygdala and infralimbic PFC to identify miRs that are involved in extinction of drug-associated memories. Although currently data are lacking, based on the above data, non-coding miRs could be targeted to facilitate extinction of drug-associated memories and to reduce psychostimulant-associated impulsivity.
Gender and Sex Gonadal Hormones
Both gender and sex gonadal hormones influence cognition. For example, behavioral impulsivity was greater in males compared with females (374). In contrast, females compared with males showed more decisional impulsivity, preferring small immediate rewards compared with larger delayed rewards. Treatment with progesterone attenuated decisional impulsivity for food reward in both males and females (375). Interestingly, progesterone alone attenuated both behavioral and decisional impulsivity for cocaine rewards in female but not male rats (141, 142) (Table 2). These data suggest that sex gonadal hormones influence impulsive behaviors. Also, amphetamine worsened impulsive behavior in females compared with males (376). Together, the above data suggest that gender and sex gonadal hormones influence psychostimulant-induced impulsive behaviors.
With respect to extinction of fear-associated memories, differential electrophysiological responses in the infralimbic and prelimbic PFC have been reported between males and females. For example, female rats compared with male rats showed persistent activity in the prelimbic PFC during extinction training, and there was lack of activity in the infralimbic PFC during extinction recall (377). Additionally, the role of estrogen and progesterone in extinction of fear-associated memories has been evaluated. In ovariectomized female rats, estrogen alone or in combination with progesterone facilitated extinction of fear-associated memories (378). Several other studies support the role of estrogen in extinction of fear-associated memories (379, 380). Together, the data suggest that gender and sex gonadal hormones may influence extinction learning.
Gender and sex gonadal hormones also influence psychostimulant drug-associated memories. Extinction of cocaine-induced CPP took longer in male compared with female adolescent rats (110). More recent work has shown that after similar extinction training, context-induced reinstatement of methamphetamine seeking was more pronounced in male compared with female rats (381). Further, the study showed that this difference in methamphetamine seeking between male and female rats was possibly mediated by differential plasticity in the dentate gyrus in the hippocampus. Together, the data suggest differential gender-dependent responses to extinction of psychostimulant drug-associated memories. Treatment with 17β estradiol compared with controls facilitated extinction of cocaine-induced CPP in female rats (150) (Table 3). Allopregnanolone, a steroid synthesized from progesterone, attenuated reinstatement of drug-induced cocaine seeking in female but not male rats (382). Allopregnanolone also attenuated reinstatement of cocaine seeking in low impulsive female rats but not in high impulsive female rats, classified as such on baseline performance prior to cocaine exposure (383). However, further studies are required to fully exploit the role of estrogen and progesterone in facilitation of extinction of psychostimulant drug-associated memories. In summary, the above data suggest that gender and sex-gonadal hormones could play an important role in cognitive deficits associated with psychostimulant drugs. However, further work is required to develop more efficacious gender-based treatments for cognitive deficits in human drug-dependent subjects.
Conclusion
Addiction to psychostimulant drugs continues to be a challenge, and current treatment options available for psychostimulant addiction are not adequate. Targeting cognitive deficits in patients dependent on psychostimulants provides an excellent opportunity to improve retention and clinical outcomes of addiction treatment programs. Cognitive deficits should especially be targeted in psychostimulant-dependent patients with a history of prenatal drug exposure and patients with comorbid psychiatric disorders known to be associated with cognitive deficits. In this review, several neural substrates mediating psychostimulant-induced cognitive deficits and identified using preclinical animal models have been discussed. It remains to be seen if these could be translated into viable pharmacological targets for medications to be used in humans to improve clinical outcomes of patients dependent on drugs of abuse. However, the main question is which of the described targets would be most ideal to carry forward into the clinic. Among the various targets described, it will be important to focus on targets that could help alleviate multiple psychostimulant-induced cognitive deficits such as impulsivity and memory impairment (e.g., orexin and cannabinoid receptors). Further, drugs that facilitate/strengthen extinction of drug-associated memories should be an essential strategy of addiction treatment programs.
Future studies must focus on identifying specific circuits mediating psychostimulant-induced cognitive deficits. Better understanding of the role of non-coding miRs, neurogenesis, and enzymes involved in epigenetic changes will greatly help in developing highly selective treatments. Finally, combining non-pharmacological strategies such as brain stimulation and exercise with pharmacological compounds will enhance alleviation of psychostimulant-induced cognitive deficits. In this review, the focus has been on targeting specific psychostimulant-induced cognitive deficits such as impulsivity and impairment of learning/memory. However, psychostimulant-induced cognitive deficits include other deficits such as impairment in attention, lack of cognitive flexibility, and impaired decision making, which have not been discussed in this review but need to be therapeutically addressed. In conclusion, a multipronged strategy targeting behavioral, emotional, and cognitive deficits in recovering abstinent addicts will greatly improve outcomes of psychostimulant addiction treatment.
Author Contributions
This paper was conceived, researched, and written by MD’S.
Funding
This work was supported by Bower, Bennet, and Bennet Endowed Chair Research Award awarded to MD’S by The Raabe College of Pharmacy, Ohio Northern University (ONU), Ada, Ohio.
Conflict of Interest Statement
The author declares that the research was conducted in the absence of any commercial or financial relationships that could be construed as a potential conflict of interest.
Abbreviations
ACPC, 1-aminocyclopropanecarboxylic acid; AMPA, amino-3-hydroxy-5-methyl-4-isoxazolepropionate/kainate; AP-5, (2R)-amino-5-phosphonovaleric acid; AQP-4, aquaporin-4; CDPPB, 3-cyano-N-(1,3-diphenyl-1H-pyrazol-5-yl)benzamide; CPP, conditioned place preference; 5-CSRTT, 5-choice serial reaction time task; DBS, deep brain stimulation; DDT, delay discounting task; 5HT, serotonin; GABA, γ-aminobutyric acid; GLT, glutamate transporter; MDMA, 3,4-methylenedioxymethamphetamine; MK-801, (5R,10S)-(−)-5-methyl-10,11-dihydro-5H-dibenzo[a,d]cylcohepten-5,10-imine; mPFC, medial prefrontal cortex; mGlu, metabotropic glutamate; MPEP, 2-methyl-6-(phenylethynyl)pyridine; MTEP, 3-(2-methyl-1,3-thiazol-4-yl)ethynyl)pyridine; mRNA, microRNA; MOR, mu opioid receptor; NAcc, nucleus accumbens; NMDA, N-methyl-d-aspartate; OFC, orbitofrontal cortex; PAMs, positive allosteric modulators; Trk B, tropomyosin-related kinase B; VTA, ventral tegmental area; xCT, cystine–glutamate exchanger.
References
1. S.A.M.H.S.A. Key substance use and mental health indicators in the United States: results from the 2017 National Survey on Drug Use and Health (ed). Hhs. Rockville, MD: Center for Behavioral Health Statistics and Quality, Substance Abuse and Mental Health Services Administration (2018).
2. Johnson M, Pennington N. Adolescent use of electronic cigarettes: an emergent health concern for pediatric nurses. J Pediatr Nurs (2015) 30:611–5. doi: 10.1016/j.pedn.2014.11.006
3. Koob GF, Ahmed SH, Boutrel B, Chen SA, Kenny PJ, Markou A, et al. Neurobiological mechanisms in the transition from drug use to drug dependence. Neurosci Biobehav Rev (2004) 27:739–49. doi: 10.1016/j.neubiorev.2003.11.007
4. Everitt BJ, Robbins TW. Drug addiction: updating actions to habits to compulsions ten years on. Annu Rev Psychol (2016) 67:23–50. doi: 10.1146/annurev-psych-122414-033457
5. Koob GF, Volkow ND. Neurocircuitry of addiction. Neuropsychopharmacology (2010) 35:217–38. doi: 10.1038/npp.2009.110
6. Phillips KA, Epstein DH, Preston KL. Psychostimulant addiction treatment. Neuropharmacology (2014) 87:150–60. doi: 10.1016/j.neuropharm.2014.04.002
7. D’souza MS, Markou A. Neuronal mechanisms underlying development of nicotine dependence: implications for novel smoking-cessation treatments. Addict Sci Clin Pract (2011) 6:4–16.
8. Prochaska JJ, Benowitz NL. The past, present, and future of nicotine addiction therapy. Annu Rev Med (2016) 67:467–86. doi: 10.1146/annurev-med-111314-033712
9. An H, He RH, Zheng YR, Tao R. Cognitive-behavioral therapy. Adv Exp Med Biol (2017) 1010:321–9. doi: 10.1007/978-981-10-5562-1_16
10. Stevens L, Verdejo-Garcia A, Goudriaan AE, Roeyers H, Dom G, Vanderplasschen W. Impulsivity as a vulnerability factor for poor addiction treatment outcomes: a review of neurocognitive findings among individuals with substance use disorders. J Subst Abuse Treat (2014) 47:58–72. doi: 10.1016/j.jsat.2014.01.008
11. Stevens L, Verdejo-Garcia A, Roeyers H, Goudriaan AE, Vanderplasschen W. Delay discounting, treatment motivation and treatment retention among substance-dependent individuals attending an in inpatient detoxification program. J Subst Abuse Treat (2015) 49:58–64. doi: 10.1016/j.jsat.2014.08.007
12. Dominguez-Salas S, Diaz-Batanero C, Lozano-Rojas OM, Verdejo-Garcia A. Impact of general cognition and executive function deficits on addiction treatment outcomes: systematic review and discussion of neurocognitive pathways. Neurosci Biobehav Rev (2016) 71:772–801. doi: 10.1016/j.neubiorev.2016.09.030
13. Mizoguchi H, Yamada K. Methamphetamine use causes cognitive impairment and altered decision-making. Neurochem Int (2019) 124:106–13. doi: 10.1016/j.neuint.2018.12.019
14. Khantzian EJ. The self-medication hypothesis of addictive disorders: focus on heroin and cocaine dependence. Am J Psychiatry (1985) 142:1259–64. doi: 10.1176/ajp.142.11.1259
15. D’souza MS, Markou A. Neural substrates of psychostimulant withdrawal-induced anhedonia. Curr Top Behav Neurosci (2010) 3:119–78. doi: 10.1007/7854_2009_20
16. Bell RP, Garavan H, Foxe JJ. Neural correlates of craving and impulsivity in abstinent former cocaine users: towards biomarkers of relapse risk. Neuropharmacology (2014) 85:461–70. doi: 10.1016/j.neuropharm.2014.05.011
17. Eysenck SB, Eysenck HJ. The place of impulsiveness in a dimensional system of personality description. Br J Soc Clin Psychol (1977) 16:57–68. doi: 10.1111/j.2044-8260.1977.tb01003.x
18. Aron AR. The neural basis of inhibition in cognitive control. Neuroscientist (2007) 13:214–28. doi: 10.1177/1073858407299288
19. Dalley JW, Robbins TW. Fractionating impulsivity: neuropsychiatric implications. Nat Rev Neurosci (2017) 18:158–71. doi: 10.1038/nrn.2017.8
20. Harris M, Penfold RB, Hawkins A, Maccombs J, Wallace B, Reynolds B. Dimensions of impulsive behavior and treatment outcomes for adolescent smokers. Exp Clin Psychopharmacol (2014) 22:57–64. doi: 10.1037/a0034403
21. Potvin S, Stavro K, Rizkallah E, Pelletier J. Cocaine and cognition: a systematic quantitative review. J Addict Med (2014) 8:368–76. doi: 10.1097/ADM.0000000000000066
22. Smith JL, Mattick RP, Jamadar SD, Iredale JM. Deficits in behavioural inhibition in substance abuse and addiction: a meta-analysis. Drug Alcohol Depend (2014) 145:1–33. doi: 10.1016/j.drugalcdep.2014.08.009
23. Jones HW, Dean AC, Price KA, London ED. Increased self-reported impulsivity in methamphetamine users maintaining drug abstinence. Am J Drug Alcohol Abuse (2016) 42:500–6. doi: 10.1080/00952990.2016.1192639
24. Hess ARB, Menezes CB, De Almeida RMM. Inhibitory control and impulsivity levels in women crack users. Subst Use Misuse (2018) 53:972–9. doi: 10.1080/10826084.2017.1387568
25. Kale D, Stautz K, Cooper A. Impulsivity related personality traits and cigarette smoking in adults: a meta-analysis using the UPPS-P model of impulsivity and reward sensitivity. Drug Alcohol Depend (2018) 185:149–67. doi: 10.1016/j.drugalcdep.2018.01.003
26. Mashhoon Y, Betts J, Farmer SL, Lukas SE. Early onset tobacco cigarette smokers exhibit deficits in response inhibition and sustained attention. Drug Alcohol Depend (2018) 184:48–56. doi: 10.1016/j.drugalcdep.2017.11.020
27. Moallem NR, Courtney KE, Ray LA. The relationship between impulsivity and methamphetamine use severity in a community sample. Drug Alcohol Depend (2018) 187:1–7. doi: 10.1016/j.drugalcdep.2018.01.034
28. Schulte MHJ, Kaag AM, Wiers RW, Schmaal L, Van Den Brink W, Reneman L, et al. Prefrontal Glx and GABA concentrations and impulsivity in cigarette smokers and smoking polysubstance users. Drug Alcohol Depend (2017) 179:117–23. doi: 10.1016/j.drugalcdep.2017.06.025
29. Martin CA, Kelly TH, Rayens MK, Brogli BR, Brenzel A, Smith WJ, et al. Sensation seeking, puberty, and nicotine, alcohol, and marijuana use in adolescence. J Am Acad Child Adolesc Psychiatry (2002) 41:1495–502. doi: 10.1097/00004583-200212000-00022
30. Moeller FG, Barratt ES, Fischer CJ, Dougherty DM, Reilly EL, Mathias CW, et al. P300 event-related potential amplitude and impulsivity in cocaine-dependent subjects. Neuropsychobiology (2004) 50:167–73. doi: 10.1159/000079110
31. Verdejo-Garcia AJ, Perales JC, Perez-Garcia M. Cognitive impulsivity in cocaine and heroin polysubstance abusers. Addict Behav (2007) 32:950–66. doi: 10.1016/j.addbeh.2006.06.032
32. Verdejo-Garcia A, Lawrence AJ, Clark L. Impulsivity as a vulnerability marker for substance-use disorders: review of findings from high-risk research, problem gamblers and genetic association studies. Neurosci Biobehav Rev (2008) 32:777–810. doi: 10.1016/j.neubiorev.2007.11.003
33. Albein-Urios N, Martinez-Gonzalez JM, Lozano O, Clark L, Verdejo-Garcia A. Comparison of impulsivity and working memory in cocaine addiction and pathological gambling: implications for cocaine-induced neurotoxicity. Drug Alcohol Depend (2012) 126:1–6. doi: 10.1016/j.drugalcdep.2012.03.008
34. Moreno-Lopez L, Catena A, Fernandez-Serrano MJ, Delgado-Rico E, Stamatakis EA, Perez-Garcia M, et al. Trait impulsivity and prefrontal gray matter reductions in cocaine dependent individuals. Drug Alcohol Depend (2012) 125:208–14. doi: 10.1016/j.drugalcdep.2012.02.012
35. Kirkpatrick MG, Johanson CE, De Wit H. Personality and the acute subjective effects of d-amphetamine in humans. J Psychopharmacol (2013) 27:256–64. doi: 10.1177/0269881112472564
36. Van Wel JH, Spronk DB, Kuypers KP, Theunissen EL, Toennes SW, Verkes RJ, et al. Psychedelic symptoms of cannabis and cocaine use as a function of trait impulsivity. J Psychopharmacol (2015) 29:324–34. doi: 10.1177/0269881114563633
37. Cervantes MC, Laughlin RE, Jentsch JD. Cocaine self-administration behavior in inbred mouse lines segregating different capacities for inhibitory control. Psychopharmacology (Berl) (2013) 229:515–25. doi: 10.1007/s00213-013-3135-4
38. Ferland JN, Winstanley CA. Risk-preferring rats make worse decisions and show increased incubation of craving after cocaine self-administration. Addict Biol (2017) 22:991–1001. doi: 10.1111/adb.12388
39. Hernandez G, Oleson EB, Gentry RN, Abbas Z, Bernstein DL, Arvanitogiannis A, et al. Endocannabinoids promote cocaine-induced impulsivity and its rapid dopaminergic correlates. Biol Psychiatry (2014) 75:487–98. doi: 10.1016/j.biopsych.2013.09.005
40. Kayir H, Semenova S, Markou A. Baseline impulsive choice predicts the effects of nicotine and nicotine withdrawal on impulsivity in rats. Prog Neuropsychopharmacol Biol Psychiatry (2014) 48:6–13. doi: 10.1016/j.pnpbp.2013.09.007
41. Kolokotroni KZ, Rodgers RJ, Harrison AA. Trait differences in response to chronic nicotine and nicotine withdrawal in rats. Psychopharmacology (Berl) (2014) 231:567–80. doi: 10.1007/s00213-013-3270-y
42. Furlong TM, Leavitt LS, Keefe KA, Son JH. Methamphetamine-, d-amphetamine-, and p-chloroamphetamine-induced neurotoxicity differentially effect impulsive responding on the stop-signal task in rats. Neurotox Res (2016) 29:569–82. doi: 10.1007/s12640-016-9605-9
43. Broos N, Van Mourik Y, Schetters D, De Vries TJ, Pattij T. Dissociable effects of cocaine and yohimbine on impulsive action and relapse to cocaine seeking. Psychopharmacology (Berl) (2017) 234:3343–51. doi: 10.1007/s00213-017-4711-9
44. Robbins TW. The 5-choice serial reaction time task: behavioural pharmacology and functional neurochemistry. Psychopharmacology (Berl). (2002) 163:362–80. doi: 10.1007/s00213-002-1154-7
45. Logan GD, Van Zandt T, Verbruggen F, Wagenmakers EJ. On the ability to inhibit thought and action: general and special theories of an act of control. Psychol Rev (2014) 121:66–95. doi: 10.1037/a0035230
46. Belin-Rauscent A, Daniel ML, Puaud M, Jupp B, Sawiak S, Howett D, et al. Impulsivity is predicted by the thinness of the insular cortex in rats. Mol Psychiatry (2016) 21:445. doi: 10.1038/mp.2016.32
47. Tsutsui-Kimura I, Ohmura Y, Izumi T, Matsushima T, Amita H, Yamaguchi T, et al. Neuronal codes for the inhibitory control of impulsive actions in the rat infralimbic cortex. Behav Brain Res (2016) 296:361–72. doi: 10.1016/j.bbr.2015.08.025
48. Rotge JY, Cocker PJ, Daniel ML, Belin-Rauscent A, Everitt BJ, Belin D. Bidirectional regulation over the development and expression of loss of control over cocaine intake by the anterior insula. Psychopharmacology (Berl) (2017) 234:1623–31. doi: 10.1007/s00213-017-4593-x
49. Naaijen J, Lythgoe DJ, Zwiers MP, Hartman CA, Hoekstra PJ, Buitelaar JK, et al. Anterior cingulate cortex glutamate and its association with striatal functioning during cognitive control. Eur Neuropsychopharmacol (2018) 28:381–91. doi: 10.1016/j.euroneuro.2018.01.002
50. Ho MY, Mobini S, Chiang TJ, Bradshaw CM, Szabadi E. Theory and method in the quantitative analysis of “impulsive choice” behaviour: implications for psychopharmacology. Psychopharmacology (Berl) (1999) 146:362–72. doi: 10.1007/PL00005482
51. Cardinal RN, Winstanley CA, Robbins TW, Everitt BJ. Limbic corticostriatal systems and delayed reinforcement. Ann N Y Acad Sci (2004) 1021:33–50. doi: 10.1196/annals.1308.004
52. Winstanley CA, Theobald DE, Cardinal RN, Robbins TW. Contrasting roles of basolateral amygdala and orbitofrontal cortex in impulsive choice. J Neurosci (2004) 24:4718–22. doi: 10.1523/JNEUROSCI.5606-03.2004
53. Cheung TH, Cardinal RN. Hippocampal lesions facilitate instrumental learning with delayed reinforcement but induce impulsive choice in rats. BMC Neurosci (2005) 6:36. doi: 10.1186/1471-2202-6-36
54. Mobini S, Chiang TJ, Ho MY, Bradshaw CM, Szabadi E. Effects of central 5-hydroxytryptamine depletion on sensitivity to delayed and probabilistic reinforcement. Psychopharmacology (Berl) (2000) 152:390–7. doi: 10.1007/s002130000542
55. Zeeb FD, Robbins TW, Winstanley CA. Serotonergic and dopaminergic modulation of gambling behavior as assessed using a novel rat gambling task. Neuropsychopharmacology (2009) 34:2329–43. doi: 10.1038/npp.2009.62
56. St Onge JR, Floresco SB. Prefrontal cortical contribution to risk-based decision making. Cereb Cortex (2010) 20:1816–28. doi: 10.1093/cercor/bhp250
57. Stopper CM, Floresco SB. What’s better for me? Fundamental role for lateral habenula in promoting subjective decision biases. Nat Neurosci (2014) 17:33–5. doi: 10.1038/nn.3587
58. Stopper CM, Green EB, Floresco SB. Selective involvement by the medial orbitofrontal cortex in biasing risky, but not impulsive, choice. Cereb Cortex (2014) 24:154–62. doi: 10.1093/cercor/bhs297
59. Berry J, Van Gorp WG, Herzberg DS, Hinkin C, Boone K, Steinman L, et al. Neuropsychological deficits in abstinent cocaine abusers: preliminary findings after two weeks of abstinence. Drug Alcohol Depend (1993) 32:231–7. doi: 10.1016/0376-8716(93)90087-7
60. Pompili M, Lester D, Girardi P, Tatarelli R. High suicide risk after the development of cognitive and working memory deficits caused by cannabis, cocaine and ecstasy use. Subst Abus (2007) 28:25–30. doi: 10.1300/J465v28n01_04
61. Fernandez-Serrano MJ, Perez-Garcia M, Perales JC, Verdejo-Garcia A. Prevalence of executive dysfunction in cocaine, heroin and alcohol users enrolled in therapeutic communities. Eur J Pharmacol (2010) 626:104–2. doi: 10.1016/j.ejphar.2009.10.019
62. Almeida PP, De Araujo Filho GM, Malta SM, Laranjeira RR, Marques A, Bressan RA, et al. Attention and memory deficits in crack-cocaine users persist over four weeks of abstinence. J Subst Abuse Treat (2017) 81:73–8. doi: 10.1016/j.jsat.2017.08.002
63. Wunderli MD, Vonmoos M, Furst M, Schadelin K, Kraemer T, Baumgartner MR, et al. Discrete memory impairments in largely pure chronic users of MDMA. Eur Neuropsychopharmacol (2017) 27:987–99. doi: 10.1016/j.euroneuro.2017.08.425
64. Kilbey MM, Breslau N, Andreski P. Cocaine use and dependence in young adults: associated psychiatric disorders and personality traits. Drug Alcohol Depend (1992) 29:283–90. doi: 10.1016/0376-8716(92)90103-J
65. Falck RS, Wang J, Carlson RG, Eddy M, Siegal HA. The prevalence and correlates of depressive symptomatology among a community sample of crack-cocaine smokers. J Psychoactive Drugs (2002) 34:281–8. doi: 10.1080/02791072.2002.10399964
66. Khurana A, Romer D, Betancourt LM, Hurt H. Working memory ability and early drug use progression as predictors of adolescent substance use disorders. Addiction (2017) 112:1220–8. doi: 10.1111/add.13792
67. Thompson AM, Swant J, Wagner JJ. Cocaine-induced modulation of long-term potentiation in the CA1 region of rat hippocampus. Neuropharmacology (2005) 49:185–94. doi: 10.1016/j.neuropharm.2005.03.005
68. Stramiello M, Wagner JJ. Cocaine enhancement of long-term potentiation in the CA1 region of rat hippocampus: lamina-specific mechanisms of action. Synapse (2010) 64:644–8. doi: 10.1002/syn.20764
69. Kutlu MG, Gould TJ. Nicotinic modulation of hippocampal cell signaling and associated effects on learning and memory. Physiol Behav (2016) 155:162–71. doi: 10.1016/j.physbeh.2015.12.008
70. Chudasama Y. Animal models of prefrontal-executive function. Behav Neurosci (2011) 125:327–43. doi: 10.1037/a0023766
71. Floresco SB, Jentsch JD. Pharmacological enhancement of memory and executive functioning in laboratory animals. Neuropsychopharmacology (2011) 36:227–50. doi: 10.1038/npp.2010.158
72. Briand LA, Gross JP, Robinson TE. Impaired object recognition following prolonged withdrawal from extended-access cocaine self-administration. Neuroscience (2008) 155:1–6. doi: 10.1016/j.neuroscience.2008.06.004
73. George O, Mandyam CD, Wee S, Koob GF. Extended access to cocaine self-administration produces long-lasting prefrontal cortex-dependent working memory impairments. Neuropsychopharmacology (2008) 33:2474–82. doi: 10.1038/sj.npp.1301626
74. Calipari ES, Beveridge TJ, Jones SR, Porrino LJ. Withdrawal from extended-access cocaine self-administration results in dysregulated functional activity and altered locomotor activity in rats. Eur J Neurosci (2013) 38:3749–57. doi: 10.1111/ejn.12381
75. Ashare RL, Falcone M, Lerman C. Cognitive function during nicotine withdrawal: implications for nicotine dependence treatment. Neuropharmacology (2014) 76 Pt B:581–91. doi: 10.1016/j.neuropharm.2013.04.034
76. Krall DM, Lim SL, Cooper AM, Burleson PW, Rhoades DJ, Jacquemin SJ, et al. Withdrawal effect of chronic amphetamine exposure during adolescence on complex maze performance. Addict Biol (2014) 19:634–42. doi: 10.1111/adb.12029
77. Janetsian SS, Linsenbardt DN, Lapish CC. Memory impairment and alterations in prefrontal cortex gamma band activity following methamphetamine sensitization. Psychopharmacology (Berl) (2015) 232:2083–95. doi: 10.1007/s00213-014-3840-7
78. Garcia-Pardo MP, La Rubia Orti JE, Aguilar Calpe MA. Differential effects of MDMA and cocaine on inhibitory avoidance and object recognition tests in rodents. Neurobiol Learn Mem (2017) 146:1–11. doi: 10.1016/j.nlm.2017.10.013
79. Gobin C, Schwendt M. The effects of extended-access cocaine self-administration on working memory performance, reversal learning and incubation of cocaine-seeking in adult male rats. J Addict Prev (2017) 5. doi: 10.13188/2330-2178.1000035
80. Rao KN, Sentir AM, Engleman EA, Bell RL, Hulvershorn LA, Breier A, et al. Toward early estimation and treatment of addiction vulnerability: radial arm maze and N-acetyl cysteine before cocaine sensitization or nicotine self-administration in neonatal ventral hippocampal lesion rats. Psychopharmacology (Berl) (2016) 233:3933–45. doi: 10.1007/s00213-016-4421-8
81. Wallace BC. Psychological and environmental determinants of relapse in crack cocaine smokers. J Subst Abuse Treat (1989) 6:95–106. doi: 10.1016/0740-5472(89)90036-6
82. O’Brien CP, Childress AR, Ehrman R, Robbins SJ. Conditioning factors in drug abuse: can they explain compulsion? J Psychopharmacol (1998) 12:15–22. doi: 10.1177/026988119801200103
83. Childress AR, Mozley PD, Mcelgin W, Fitzgerald J, Reivich M, O’Brien CP. Limbic activation during cue-induced cocaine craving. Am J Psychiatry (1999) 156:11–8. doi: 10.1176/ajp.156.1.11
84. Sinha R, Fuse T, Aubin LR, O’Malley SS. Psychological stress, drug-related cues and cocaine craving. Psychopharmacology (Berl) (2000) 152:140–8. doi: 10.1007/s002130000499
85. Perry CJ, Lawrence AJ. Addiction, cognitive decline and therapy: seeking ways to escape a vicious cycle. Genes Brain Behav (2017) 16:205–8. doi: 10.1111/gbb.12325
86. Quirk GJ, Mueller D. Neural mechanisms of extinction learning and retrieval. Neuropsychopharmacology (2008) 33:56–72. doi: 10.1038/sj.npp.1301555
87. Myers KM, Carlezon WA Jr. Extinction of drug- and withdrawal-paired cues in animal models: relevance to the treatment of addiction. Neurosci Biobehav Rev (2010) 35:285–302. doi: 10.1016/j.neubiorev.2010.01.011
88. Mcnally GP. Extinction of drug seeking: neural circuits and approaches to augmentation. Neuropharmacology (2014) 76 Pt B:528–32. doi: 10.1016/j.neuropharm.2013.06.007
89. Shaham Y, Shalev U, Lu L, De Wit H, Stewart J. The reinstatement model of drug relapse: history, methodology and major findings. Psychopharmacology (Berl) (2003) 168:3–20. doi: 10.1007/s00213-002-1224-x
90. Crombag HS, Bossert JM, Koya E, Shaham Y. Review. Context-induced relapse to drug seeking: a review. Philos Trans R Soc Lond B Biol Sci (2008) 363:3233–43. doi: 10.1098/rstb.2008.0090
91. Morgan MA, Ledoux JE. Differential contribution of dorsal and ventral medial prefrontal cortex to the acquisition and extinction of conditioned fear in rats. Behav Neurosci (1995) 109:681–8. doi: 10.1037//0735-7044.109.4.681
92. Peters J, Lalumiere RT, Kalivas PW. Infralimbic prefrontal cortex is responsible for inhibiting cocaine seeking in extinguished rats. J Neurosci (2008) 28:6046–53. doi: 10.1523/JNEUROSCI.1045-08.2008
93. Peters J, Kalivas PW, Quirk GJ. Extinction circuits for fear and addiction overlap in prefrontal cortex. Learn Mem (2009) 16:279–88. doi: 10.1101/lm.1041309
94. Nic Dhonnchadha BA, Kantak KM. Cognitive enhancers for facilitating drug cue extinction: insights from animal models. Pharmacol Biochem Behav (2011) 99:229–44. doi: 10.1016/j.pbb.2011.01.018
95. Torregrossa MM, Taylor JR. Learning to forget: manipulating extinction and reconsolidation processes to treat addiction. Psychopharmacology (Berl) (2013) 226:659–72. doi: 10.1007/s00213-012-2750-9
96. Back SE, Killeen T, Badour CL, Flanagan JC, Allan NP, Ana ES, et al. Concurrent treatment of substance use disorders and PTSD using prolonged exposure: a randomized clinical trial in military veterans. Addict Behav (2019) 90:369–77. doi: 10.1016/j.addbeh.2018.11.032
97. Dani JA, De Biasi M. Cellular mechanisms of nicotine addiction. Pharmacol Biochem Behav (2001) 70:439–46. doi: 10.1016/S0091-3057(01)00652-9
98. Guilarte TR, Nihei MK, Mcglothan JL, Howard AS. Methamphetamine-induced deficits of brain monoaminergic neuronal markers: distal axotomy or neuronal plasticity. Neuroscience (2003) 122:499–513. doi: 10.1016/S0306-4522(03)00476-7
99. Edwards S, Whisler KN, Fuller DC, Orsulak PJ, Self DW. Addiction-related alterations in D1 and D2 dopamine receptor behavioral responses following chronic cocaine self-administration. Neuropsychopharmacology (2007b) 32:354–66. doi: 10.1038/sj.npp.1301062
100. Thomas MJ, Kalivas PW, Shaham Y. Neuroplasticity in the mesolimbic dopamine system and cocaine addiction. Br J Pharmacol (2008) 154:327–42. doi: 10.1038/bjp.2008.77
101. Sharma A, Couture J. A review of the pathophysiology, etiology, and treatment of attention-deficit hyperactivity disorder (ADHD). Ann Pharmacother (2014) 48:209–25. doi: 10.1177/1060028013510699
102. Caye A, Swanson JM, Coghill D, Rohde LA. Treatment strategies for ADHD: an evidence-based guide to select optimal treatment. Mol Psychiatry (2019) 24:390–408. doi: 10.1038/s41380-018-0116-3
103. Jupp B, Dalley JW. Convergent pharmacological mechanisms in impulsivity and addiction: insights from rodent models. Br J Pharmacol (2014) 171:4729–66. doi: 10.1111/bph.12787
104. Wade TR, De Wit H, Richards JB. Effects of dopaminergic drugs on delayed reward as a measure of impulsive behavior in rats. Psychopharmacology (Berl) (2000) 150:90–101. doi: 10.1007/s002130000402
105. Milienne-Petiot M, Groenink L, Minassian A, Young JW. Blockade of dopamine D1-family receptors attenuates the mania-like hyperactive, risk-preferring, and high motivation behavioral profile of mice with low dopamine transporter levels. J Psychopharmacol (2017) 31:1334–46. doi: 10.1177/0269881117731162
106. Pattij T, Janssen MC, Vanderschuren LJ, Schoffelmeer AN, Van Gaalen MM. Involvement of dopamine D1 and D2 receptors in the nucleus accumbens core and shell in inhibitory response control. Psychopharmacology (Berl) (2007) 191:587–98. doi: 10.1007/s00213-006-0533-x
107. Anderson SM, Bari AA, Pierce RC. Administration of the D1-like dopamine receptor antagonist SCH-23390 into the medial nucleus accumbens shell attenuates cocaine priming-induced reinstatement of drug-seeking behavior in rats. Psychopharmacology (Berl) (2003) 168:132–8. doi: 10.1007/s00213-002-1298-5
108. Loos M, Pattij T, Janssen MC, Counotte DS, Schoffelmeer AN, Smit AB, et al. Dopamine receptor D1/D5 gene expression in the medial prefrontal cortex predicts impulsive choice in rats. Cereb Cortex (2010) 20:1064–70. doi: 10.1093/cercor/bhp167
109. Wang Y, Yin F, Guo H, Zhang J, Yan P, Lai J. The role of dopamine D1 and D3 receptors in N-methyl-d-aspartate (NMDA)/glycineB site-regulated complex cognitive behaviors following repeated morphine administration. Int J Neuropsychopharmacol (2017) 20:562–74. doi: 10.1093/ijnp/pyx010
110. Brenhouse HC, Thompson BS, Sonntag KC, Andersen SL. Extinction and reinstatement to cocaine-associated cues in male and female juvenile rats and the role of D1 dopamine receptor. Neuropharmacology (2015) 95:22–8. doi: 10.1016/j.neuropharm.2015.02.017
111. Huang B, Li Y, Cheng D, He G, Liu X, Ma L. Beta-arrestin-biased beta-adrenergic signaling promotes extinction learning of cocaine reward memory. Sci Signal (2018) 11 (512): eaam 5402. doi: 10.1126/scisignal.aam5402
112. Otis JM, Fitzgerald MK, Mueller D. Infralimbic BDNF/TrkB enhancement of GluN2B currents facilitates extinction of a cocaine-conditioned place preference. J Neurosci (2014a) 34:6057–64. doi: 10.1523/JNEUROSCI.4980-13.2014
113. Alaghband Y, Kwapis JL, Lopez AJ, White AO, Aimiuwu OV, Al-Kachak A, et al. Distinct roles for the deacetylase domain of HDAC3 in the hippocampus and medial prefrontal cortex in the formation and extinction of memory. Neurobiol Learn Mem (2017) 145:94–104. doi: 10.1016/j.nlm.2017.09.001
114. Hu SS, Liu YW, Yu L. Medial prefrontal cannabinoid CB1 receptors modulate consolidation and extinction of cocaine-associated memory in mice. Psychopharmacology (Berl) (2015) 232:1803–15. doi: 10.1007/s00213-014-3812-y
115. Heysieattalab S, Naghdi N, Zarrindast MR, Haghparast A, Mehr SE, Khoshbouei H. The effects of GABAA and NMDA receptors in the shell-accumbens on spatial memory of METH-treated rats. Pharmacol Biochem Behav (2016) 142:23–35. doi: 10.1016/j.pbb.2015.12.008
116. Kinoshita KI, Muroi Y, Unno T, Ishii T. Rolipram improves facilitation of contextual fear extinction in the 1-methyl-4-phenyl-1,2,3,6-tetrahydropyridine-induced mouse model of Parkinson’s disease. J Pharmacol Sci (2017) 134:55–8. doi: 10.1016/j.jphs.2017.04.002
117. Dominguez G, Dagnas M, Decorte L, Vandesquille M, Belzung C, Beracochea D, et al. Rescuing prefrontal cAMP-CREB pathway reverses working memory deficits during withdrawal from prolonged alcohol exposure. Brain Struct Funct (2016) 221:865–77. doi: 10.1007/s00429-014-0941-3
118. Edwards S, Graham DL, Bachtell RK, Self DW. Region-specific tolerance to cocaine-regulated cAMP-dependent protein phosphorylation following chronic self-administration. Eur J Neurosci (2007a) 25:2201–13. doi: 10.1111/j.1460-9568.2007.05473.x
119. Ru Q, Xiong Q, Zhou M, Chen L, Tian X, Xiao H, et al. Withdrawal from chronic treatment with methamphetamine induces anxiety and depression-like behavior in mice. Psychiatry Res (2018) 271:476–83. doi: 10.1016/j.psychres.2018.11.072
120. Li Y, Zuo Y, Yu P, Ping X, Cui C. Role of basolateral amygdala dopamine D2 receptors in impulsive choice in acute cocaine-treated rats. Behav Brain Res (2015b) 287:187–95. doi: 10.1016/j.bbr.2015.03.039
121. Besson M, Pelloux Y, Dilleen R, Theobald DE, Lyon A, Belin-Rauscent A, et al. Cocaine modulation of frontostriatal expression of Zif268, D2, and 5-HT2c receptors in high and low impulsive rats. Neuropsychopharmacology (2013) 38:1963–73. doi: 10.1038/npp.2013.95
122. Caprioli D, Hong YT, Sawiak SJ, Ferrari V, Williamson DJ, Jupp B, et al. Baseline-dependent effects of cocaine pre-exposure on impulsivity and D2/3 receptor availability in the rat striatum: possible relevance to the attention-deficit hyperactivity syndrome. Neuropsychopharmacology (2013) 38:1460–71. doi: 10.1038/npp.2013.44
123. Caprioli D, Jupp B, Hong YT, Sawiak SJ, Ferrari V, Wharton L, et al. Dissociable rate-dependent effects of oral methylphenidate on impulsivity and D2/3 receptor availability in the striatum. J Neurosci (2015) 35:3747–55. doi: 10.1523/JNEUROSCI.3890-14.2015
124. Yates JR, Perry JL, Meyer AC, Gipson CD, Charnigo R, Bardo MT. Role of medial prefrontal and orbitofrontal monoamine transporters and receptors in performance in an adjusting delay discounting procedure. Brain Res (2014) 1574:26–36. doi: 10.1016/j.brainres.2014.06.004
125. Bernosky-Smith KA, Qiu YY, Feja M, Lee YB, Loughlin B, Li JX, et al. Ventral tegmental area D2 receptor knockdown enhances choice impulsivity in a delay-discounting task in rats. Behav Brain Res (2018) 341:129–34. doi: 10.1016/j.bbr.2017.12.029
126. Cocker PJ, Tremblay M, Kaur S, Winstanley CA. Chronic administration of the dopamine D2/3 agonist ropinirole invigorates performance of a rodent slot machine task, potentially indicative of less distractible or compulsive-like gambling behaviour. Psychopharmacology (Berl) (2017) 234:137–53. doi: 10.1007/s00213-016-4447-y
127. Seeman P. Parkinson’s disease treatment may cause impulse-control disorder via dopamine D3 receptors. Synapse (2015) 69:183–9. doi: 10.1002/syn.21805
128. Barrus MM, Winstanley CA. Dopamine D3 receptors modulate the ability of win-paired cues to increase risky choice in a rat gambling task. J Neurosci (2016) 36:785–94. doi: 10.1523/JNEUROSCI.2225-15.2016
129. Di Ciano P, Cormick PM, Stefan C, Wong E, Kim A, Remington G, et al. The effects of buspirone on occupancy of dopamine receptors and the rat gambling task. Psychopharmacology (Berl) (2017) 234:3309–20. doi: 10.1007/s00213-017-4715-5
130. Chen Y, Song R, Yang RF, Wu N, Li J. A novel dopamine D3 receptor antagonist YQA14 inhibits methamphetamine self-administration and relapse to drug-seeking behaviour in rats. Eur J Pharmacol (2014) 743:126–32. doi: 10.1016/j.ejphar.2014.09.026
131. Song R, Bi GH, Zhang HY, Yang RF, Gardner EL, Li J, et al. Blockade of D3 receptors by YQA14 inhibits cocaine’s rewarding effects and relapse to drug-seeking behavior in rats. Neuropharmacology (2014) 77:398–405. doi: 10.1016/j.neuropharm.2013.10.010
132. Picciotto MR, Corrigall WA. Neuronal systems underlying behaviors related to nicotine addiction: neural circuits and molecular genetics. J Neurosci (2002) 22:3338–41. doi: 10.1523/JNEUROSCI.22-09-03338.2002
133. Howell LL, Kimmel HL. Monoamine transporters and psychostimulant addiction. Biochem Pharmacol (2008) 75:196–217. doi: 10.1016/j.bcp.2007.08.003
134. Smith RJ, Aston-Jones G. Noradrenergic transmission in the extended amygdala: role in increased drug-seeking and relapse during protracted drug abstinence. Brain Struct Funct (2008) 213:43–61. doi: 10.1007/s00429-008-0191-3
135. Sofuoglu M, Sewell RA. Norepinephrine and stimulant addiction. Addict Biol (2009) 14:119–29. doi: 10.1111/j.1369-1600.2008.00138.x
136. Fernando AB, Economidou D, Theobald DE, Zou MF, Newman AH, Spoelder M, et al. Modulation of high impulsivity and attentional performance in rats by selective direct and indirect dopaminergic and noradrenergic receptor agonists. Psychopharmacology (Berl) (2012) 219:341–52. doi: 10.1007/s00213-011-2408-z
137. Freund N, Jordan CJ, Lukkes JL, Norman KJ, Andersen SL. Juvenile exposure to methylphenidate and guanfacine in rats: effects on early delay discounting and later cocaine-taking behavior. Psychopharmacology (Berl) (2019) 236:685–698. doi: 10.1007/s00213-018-5096-0
138. Nishitomi K, Yano K, Kobayashi M, Jino K, Kano T, Horiguchi N, et al. Systemic administration of guanfacine improves food-motivated impulsive choice behavior primarily via direct stimulation of postsynaptic alpha2A-adrenergic receptors in rats. Behav Brain Res (2018) 345:21–9. doi: 10.1016/j.bbr.2018.02.022
139. Terry AV Jr., Callahan PM, Schade R, Kille NJ, Plagenhoef M. Alpha 2A adrenergic receptor agonist, guanfacine, attenuates cocaine-related impairments of inhibitory response control and working memory in animal models. Pharmacol Biochem Behav (2014) 126:63–72. doi: 10.1016/j.pbb.2014.09.010
140. Gentile TA, Simmons SJ, Watson MN, Connelly KL, Brailoiu E, Zhang Y, et al. Effects of suvorexant, a dual orexin/hypocretin receptor antagonist, on impulsive behavior associated with cocaine. Neuropsychopharmacology (2018b) 43:1001–9. doi: 10.1038/npp.2017.158
141. Swalve N, Smethells JR, Younk R, Mitchell J, Dougen B, Carroll ME. Sex-specific attenuation of impulsive action by progesterone in a Go/No-Go task for cocaine in rats. Psychopharmacology (Berl) (2018) 235:135–43. doi: 10.1007/s00213-017-4750-2
142. Smethells JR, Swalve NL, Eberly LE, Carroll ME. Sex differences in the reduction of impulsive choice (delay discounting) for cocaine in rats with atomoxetine and progesterone. Psychopharmacology (Berl) (2016) 233:2999–3008. doi: 10.1007/s00213-016-4345-3
143. Marszalek-Grabska M, Gibula-Bruzda E, Jenda M, Gawel K, Kotlinska JH. Memantine improves memory impairment and depressive-like behavior induced by amphetamine withdrawal in rats. Brain Res (2016) 1642:389–96. doi: 10.1016/j.brainres.2016.04.026
144. Saravia R, Flores A, Plaza-Zabala A, Busquets-Garcia A, Pastor A, De La Torre R, et al. CB1 cannabinoid receptors mediate cognitive deficits and structural plasticity changes during nicotine withdrawal. Biol Psychiatry (2017) 81:625–34. doi: 10.1016/j.biopsych.2016.07.007
145. Botreau F, Paolone G, Stewart J. d-Cycloserine facilitates extinction of a cocaine-induced conditioned place preference. Behav Brain Res (2006) 172:173–8. doi: 10.1016/j.bbr.2006.05.012
146. Gass JT, Olive MF. Positive allosteric modulation of mGluR5 receptors facilitates extinction of a cocaine contextual memory. Biol Psychiatry (2009) 65:717–20. doi: 10.1016/j.biopsych.2008.11.001
147. Cleva RM, Hicks MP, Gass JT, Wischerath KC, Plasters ET, Widholm JJ, et al. mGluR5 positive allosteric modulation enhances extinction learning following cocaine self-administration. Behav Neurosci (2011) 125:10–9. doi: 10.1037/a0022339
148. Liddie S, Anderson KL, Paz A, Itzhak Y. The effect of phosphodiesterase inhibitors on the extinction of cocaine-induced conditioned place preference in mice. J Psychopharmacol (2012) 26:1375–82. doi: 10.1177/0269881112447991
149. Otis JM, Fitzgerald MK, Mueller D. Inhibition of hippocampal beta-adrenergic receptors impairs retrieval but not reconsolidation of cocaine-associated memory and prevents subsequent reinstatement. Neuropsychopharmacology (2014b) 39:303–10. doi: 10.1038/npp.2013.187
150. Twining RC, Tuscher JJ, Doncheck EM, Frick KM, Mueller D. 17Beta-estradiol is necessary for extinction of cocaine seeking in female rats. Learn Mem (2013) 20:300–6. doi: 10.1101/lm.030304.113
151. Childs JE, Deleon J, Nickel E, Kroener S. Vagus nerve stimulation reduces cocaine seeking and alters plasticity in the extinction network. Learn Mem (2017) 24:35–42. doi: 10.1101/lm.043539.116
152. Voigt RM, Herrold AA, Napier TC. Baclofen facilitates the extinction of methamphetamine-induced conditioned place preference in rats. Behav Neurosci (2011) 125:261–7. doi: 10.1037/a0022893
153. Fox H, Sofuoglu M, Sinha R. Guanfacine enhances inhibitory control and attentional shifting in early abstinent cocaine-dependent individuals. J Psychopharmacol (2015) 29:312–23. doi: 10.1177/0269881114562464
154. Smith RJ, Aston-Jones G. Alpha(2) adrenergic and imidazoline receptor agonists prevent cue-induced cocaine seeking. Biol Psychiatry (2011) 70:712–9. doi: 10.1016/j.biopsych.2011.06.010
155. Feltenstein MW, See RE. Potentiation of cue-induced reinstatement of cocaine-seeking in rats by the anxiogenic drug yohimbine. Behav Brain Res (2006) 174:1–8. doi: 10.1016/j.bbr.2006.06.039
156. Economidou D, Theobald DE, Robbins TW, Everitt BJ, Dalley JW. Norepinephrine and dopamine modulate impulsivity on the five-choice serial reaction time task through opponent actions in the shell and core sub-regions of the nucleus accumbens. Neuropsychopharmacology (2012) 37:2057–66. doi: 10.1038/npp.2012.53
157. Broos N, Loonstra R, Van Mourik Y, Schetters D, Schoffelmeer AN, Pattij T, et al. Subchronic administration of atomoxetine causes an enduring reduction in context-induced relapse to cocaine seeking without affecting impulsive decision making. Addict Biol (2015) 20:714–23. doi: 10.1111/adb.12168
158. Economidou D, Pelloux Y, Robbins TW, Dalley JW, Everitt BJ. High impulsivity predicts relapse to cocaine-seeking after punishment-induced abstinence. Biol Psychiatry (2009) 65:851–6. doi: 10.1016/j.biopsych.2008.12.008
159. Economidou D, Cippitelli A, Stopponi S, Braconi S, Clementi S, Ubaldi M, et al. Activation of brain NOP receptors attenuates acute and protracted alcohol withdrawal symptoms in the rat. Alcohol Clin Exp Res (2011) 35:747–55. doi: 10.1111/j.1530-0277.2010.01392.x
160. Xue YX, Deng JH, Chen YY, Zhang LB, Wu P, Huang GD, et al. Effect of selective inhibition of reactivated nicotine-associated memories with propranolol on nicotine craving. JAMA Psychiatry (2017) 74:224–32. doi: 10.1001/jamapsychiatry.2016.3907
161. Burhans LB, Smith-Bell CA, Schreurs BG. Propranolol produces short-term facilitation of extinction in a rabbit model of post-traumatic stress disorder. Neuropharmacology (2018) 135:386–98. doi: 10.1016/j.neuropharm.2018.03.029
162. Muller CP, Homberg JR. The role of serotonin in drug use and addiction. Behav Brain Res (2015) 277:146–92. doi: 10.1016/j.bbr.2014.04.007
163. Pattij T, Schoffelmeer AN. Serotonin and inhibitory response control: focusing on the role of 5-HT(1A) receptors. Eur J Pharmacol (2015) 753:140–5. doi: 10.1016/j.ejphar.2014.05.064
164. Marek GJ, Li AA, Seiden LS. Evidence for involvement of 5-hydroxytryptamine1 receptors in antidepressant-like drug effects on differential-reinforcement-of-low-rate 72-second behavior. J Pharmacol Exp Ther (1989) 250:60–71.
165. Homberg JR, Pattij T, Janssen MC, Ronken E, De Boer SF, Schoffelmeer AN, et al. Serotonin transporter deficiency in rats improves inhibitory control but not behavioural flexibility. Eur J Neurosci (2007) 26:2066–73. doi: 10.1111/j.1460-9568.2007.05839.x
166. Baarendse PJ, Vanderschuren LJ. Dissociable effects of monoamine reuptake inhibitors on distinct forms of impulsive behavior in rats. Psychopharmacology (Berl) (2012) 219:313–26. doi: 10.1007/s00213-011-2576-x
167. Puumala T, Sirvio J. Changes in activities of dopamine and serotonin systems in the frontal cortex underlie poor choice accuracy and impulsivity of rats in an attention task. Neuroscience (1998) 83:489–99. doi: 10.1016/S0306-4522(97)00392-8
168. Dalley JW, Theobald DE, Eagle DM, Passetti F, Robbins TW. Deficits in impulse control associated with tonically-elevated serotonergic function in rat prefrontal cortex. Neuropsychopharmacology (2002) 26:716–28. doi: 10.1016/S0893-133X(01)00412-2
169. Fink LH, Anastasio NC, Fox RG, Rice KC, Moeller FG, Cunningham KA. Individual differences in impulsive action reflect variation in the cortical serotonin 5-HT2A receptor system. Neuropsychopharmacology (2015) 40:1957–68. doi: 10.1038/npp.2015.46
170. Sholler DJ, Stutz SJ, Fox RG, Boone EL, Wang Q, Rice KC, et al. The 5-HT2A receptor (5-HT2AR) regulates impulsive action and cocaine cue reactivity in male Sprague–Dawley rats. J Pharmacol Exp Ther (2019) 368:41–9. doi: 10.1124/jpet.118.251199
171. Wischhof L, Hollensteiner KJ, Koch M. Impulsive behaviour in rats induced by intracortical DOI infusions is antagonized by co-administration of an mGlu2/3 receptor agonist. Behav Pharmacol (2011) 22:805–13. doi: 10.1097/FBP.0b013e32834d6279
172. Adams WK, Barkus C, Ferland JN, Sharp T, Winstanley CA. Pharmacological evidence that 5-HT2C receptor blockade selectively improves decision making when rewards are paired with audiovisual cues in a rat gambling task. Psychopharmacology (Berl) (2017a) 234:3091–104. doi: 10.1007/s00213-017-4696-4
173. Mori M, Tsutsui-Kimura I, Mimura M, Tanaka KF. 5-HT3 antagonists decrease discounting rate without affecting sensitivity to reward magnitude in the delay discounting task in mice. Psychopharmacology (Berl) (2018) 235:2619–29. doi: 10.1007/s00213-018-4954-0
174. Li X, Semenova S, D’Souza MS, Stoker AK, Markou A. Involvement of glutamatergic and GABAergic systems in nicotine dependence: implications for novel pharmacotherapies for smoking cessation. Neuropharmacology (2014) 76 Pt B:554–65. doi: 10.1016/j.neuropharm.2013.05.042
175. Mccreary AC, Muller CP, Filip M. Psychostimulants: basic and clinical pharmacology. Int Rev Neurobiol (2015) 120:41–83. doi: 10.1016/bs.irn.2015.02.008
176. Berro LF, Andersen ML, Tufik S, Howell LL. GABAA receptor positive allosteric modulators modify the abuse-related behavioral and neurochemical effects of methamphetamine in rhesus monkeys. Neuropharmacology (2017) 123:299–309. doi: 10.1016/j.neuropharm.2017.05.010
177. Hayes DJ, Jupp B, Sawiak SJ, Merlo E, Caprioli D, Dalley JW. Brain gamma-aminobutyric acid: a neglected role in impulsivity. Eur J Neurosci (2014) 39:1921–32. doi: 10.1111/ejn.12485
178. Heaney CF, Kinney JW. Role of GABA(B) receptors in learning and memory and neurological disorders. Neurosci Biobehav Rev (2016) 63:1–28. doi: 10.1016/j.neubiorev.2016.01.007
179. Mick I, Ramos AC, Myers J, Stokes PR, Chandrasekera S, Erritzoe D, et al. Evidence for GABA-A receptor dysregulation in gambling disorder: correlation with impulsivity. Addict Biol (2017) 22:1601–09. doi: 10.1111/adb.12457
180. Paine TA, Slipp LE, Carlezon WA Jr.. Schizophrenia-like attentional deficits following blockade of prefrontal cortex GABAA receptors. Neuropsychopharmacology (2011) 36:1703–13. doi: 10.1038/npp.2011.51
181. Murphy ER, Fernando AB, Urcelay GP, Robinson ES, Mar AC, Theobald DE, et al. Impulsive behaviour induced by both NMDA receptor antagonism and GABAA receptor activation in rat ventromedial prefrontal cortex. Psychopharmacology (Berl) (2012) 219:401–10. doi: 10.1007/s00213-011-2572-1
182. Feja M, Koch M. Ventral medial prefrontal cortex inactivation impairs impulse control but does not affect delay-discounting in rats. Behav Brain Res (2014) 264:230–9. doi: 10.1016/j.bbr.2014.02.013
183. Zapata A, Hwang EK, Lupica CR. Lateral habenula involvement in impulsive cocaine seeking. Neuropsychopharmacology (2017) 42:1103–12. doi: 10.1038/npp.2016.286
184. Froger-Colleaux C, Castagne V. Effects of baclofen and raclopride on reinstatement of cocaine self-administration in the rat. Eur J Pharmacol (2016) 777:147–55. doi: 10.1016/j.ejphar.2016.03.008
185. Li X, Sturchler E, Kaczanowska K, Cameron M, Finn MG, Griffin P, et al. KK-92A, a novel GABAB receptor positive allosteric modulator, attenuates nicotine self-administration and cue-induced nicotine seeking in rats. Psychopharmacology (Berl) (2017b) 234:1633–44. doi: 10.1007/s00213-017-4594-9
186. Kalivas PW. The glutamate homeostasis hypothesis of addiction. Nat Rev Neurosci (2009) 10:561–72. doi: 10.1038/nrn2515
187. D’souza MS. Glutamatergic transmission in drug reward: implications for drug addiction. Front Neurosci (2015) 9:404. doi: 10.3389/fnins.2015.00404
188. Bobadilla AC, Heinsbroek JA, Gipson CD, Griffin WC, Fowler CD, Kenny PJ, et al. Corticostriatal plasticity, neuronal ensembles, and regulation of drug-seeking behavior. Prog Brain Res (2017) 235:93–112. doi: 10.1016/bs.pbr.2017.07.013
189. Myers KM, Carlezon WA Jr., Davis M. Glutamate receptors in extinction and extinction-based therapies for psychiatric illness. Neuropsychopharmacology (2011) 36:274–93. doi: 10.1038/npp.2010.88
190. Yates JR, Batten SR, Bardo MT, Beckmann JS. Role of ionotropic glutamate receptors in delay and probability discounting in the rat. Psychopharmacology (Berl) (2015) 232:1187–96. doi: 10.1007/s00213-014-3747-3
191. Higgins GA, Silenieks LB, Macmillan C, Sevo J, Zeeb FD, Thevarkunnel S. Enhanced attention and impulsive action following NMDA receptor GluN2B-selective antagonist pretreatment. Behav Brain Res (2016) 311:1–14. doi: 10.1016/j.bbr.2016.05.025
192. Floresco SB, Tse MT, Ghods-Sharifi S. Dopaminergic and glutamatergic regulation of effort- and delay-based decision making. Neuropsychopharmacology (2008) 33:1966–79. doi: 10.1038/sj.npp.1301565
193. Cottone P, Iemolo A, Narayan AR, Kwak J, Momaney D, Sabino V. The uncompetitive NMDA receptor antagonists ketamine and memantine preferentially increase the choice for a small, immediate reward in low-impulsive rats. Psychopharmacology (Berl) (2013) 226:127–38. doi: 10.1007/s00213-012-2898-3
194. Yates JR, Bardo MT. Effects of intra-accumbal administration of dopamine and ionotropic glutamate receptor drugs on delay discounting performance in rats. Behav Neurosci (2017) 131:392–405. doi: 10.1037/bne0000214
195. Mcentee WJ, Crook TH. Glutamate: its role in learning, memory, and the aging brain. Psychopharmacology (Berl) (1993) 111:391–401. doi: 10.1007/BF02253527
196. Riedel G, Platt B, Micheau J. Glutamate receptor function in learning and memory. Behav Brain Res (2003) 140:1–47. doi: 10.1016/S0166-4328(02)00272-3
197. Paolone G, Botreau F, Stewart J. The facilitative effects of D-cycloserine on extinction of a cocaine-induced conditioned place preference can be long lasting and resistant to reinstatement. Psychopharmacology (Berl) (2009) 202:403–9. doi: 10.1007/s00213-008-1280-y
198. Wu IT, Tang TH, Ko MC, Chiu CY, Lu KT. Amygdaloid zif268 participated in the d-cycloserine facilitation effect on the extinction of conditioned fear. Psychopharmacology (Berl) (2015) 232:3809–19. doi: 10.1007/s00213-015-4042-7
199. Trent S, Barnes P, Hall J, Thomas KL. AMPA receptors control fear extinction through an Arc-dependent mechanism. Learn Mem (2017) 24:375–80. doi: 10.1101/lm.045013.117
200. Wu X, Zhang JT, Li D, Zhou J, Yang J, Zheng HL, et al. Aquaporin-4 deficiency facilitates fear memory extinction in the hippocampus through excessive activation of extrasynaptic GluN2B-containing NMDA receptors. Neuropharmacology (2017) 112:124–34. doi: 10.1016/j.neuropharm.2016.06.031
201. Yamada D, Wada K, Sekiguchi M. Facilitating actions of an AMPA receptor potentiator upon extinction of contextually conditioned fear response in stressed mice. Neurosci Lett (2011) 488:242–6. doi: 10.1016/j.neulet.2010.11.038
202. Chen W, Wang Y, Sun A, Zhou L, Xu W, Zhu H, et al. Activation of AMPA receptor in the infralimbic cortex facilitates extinction and attenuates the heroin-seeking behavior in rats. Neurosci Lett (2016) 612:126–31. doi: 10.1016/j.neulet.2015.11.024
203. Lin A, Man HY. Endocytic adaptor epidermal growth factor receptor substrate 15 (Eps15) is involved in the trafficking of ubiquitinated alpha-amino-3-hydroxy-5-methyl-4-isoxazolepropionic acid receptors. J Biol Chem (2014) 289:24652–64. doi: 10.1074/jbc.M114.582114
204. Wischhof L, Koch M. Pre-treatment with the mGlu2/3 receptor agonist LY379268 attenuates DOI-induced impulsive responding and regional c-Fos protein expression. Psychopharmacology (Berl) (2012) 219:387–400. doi: 10.1007/s00213-011-2441-y
205. Isherwood SN, Robbins TW, Nicholson JR, Dalley JW, Pekcec A. Selective and interactive effects of D2 receptor antagonism and positive allosteric mGluR4 modulation on waiting impulsivity. Neuropharmacology (2017) 123:249–60. doi: 10.1016/j.neuropharm.2017.05.006
206. Isherwood SN, Pekcec A, Nicholson JR, Robbins TW, Dalley JW. Dissociable effects of mGluR5 allosteric modulation on distinct forms of impulsivity in rats: interaction with NMDA receptor antagonism. Psychopharmacology (Berl) (2015) 232:3327–44. doi: 10.1007/s00213-015-3984-0
207. Sethna F, Wang H. Pharmacological enhancement of mGluR5 facilitates contextual fear memory extinction. Learn Mem (2014) 21:647–50. doi: 10.1101/lm.035857.114
208. Ben-Shahar O, Sacramento AD, Miller BW, Webb SM, Wroten MG, Silva HE, et al. Deficits in ventromedial prefrontal cortex group 1 metabotropic glutamate receptor function mediate resistance to extinction during protracted withdrawal from an extensive history of cocaine self-administration. J Neurosci (2013) 33:495–506a. doi: 10.1523/JNEUROSCI.3710-12.2013
209. Sepulveda-Orengo MT, Healey KL, Kim R, Auriemma AC, Rojas J, Woronoff N, et al. Riluzole impairs cocaine reinstatement and restores adaptations in intrinsic excitability and GLT-1 expression. Neuropsychopharmacology (2018) 43:1212–23. doi: 10.1038/npp.2017.244
210. Sugiyama A, Yamada M, Saitoh A, Oka JI, Yamada M. Administration of riluzole to the basolateral amygdala facilitates fear extinction in rats. Behav Brain Res (2018) 336:8–14. doi: 10.1016/j.bbr.2017.08.031
211. Kupchik YM, Moussawi K, Tang XC, Wang X, Kalivas BC, Kolokithas R, et al. The effect of N-acetylcysteine in the nucleus accumbens on neurotransmission and relapse to cocaine. Biol Psychiatry (2012) 71:978–86. doi: 10.1016/j.biopsych.2011.10.024
212. Ramirez-Nino AM, D’Souza MS, Markou A. N-Acetylcysteine decreased nicotine self-administration and cue-induced reinstatement of nicotine seeking in rats: comparison with the effects of N-acetylcysteine on food responding and food seeking. Psychopharmacology (Berl) (2013) 225:473–82. doi: 10.1007/s00213-012-2837-3
213. Chambers RA, Moore J, Mcevoy JP, Levin ED. Cognitive effects of neonatal hippocampal lesions in a rat model of schizophrenia. Neuropsychopharmacology (1996) 15:587–94. doi: 10.1016/S0893-133X(96)00132-7
214. Ohmura Y, Tsutsui-Kimura I, Yoshioka M. Impulsive behavior and nicotinic acetylcholine receptors. J Pharmacol Sci (2012) 118:413–22. doi: 10.1254/jphs.11R06CR
215. Jeong JE, Rhee JK, Kim TM, Kwak SM, Bang SH, Cho H, et al. The association between the nicotinic acetylcholine receptor alpha4 subunit gene (CHRNA4) rs1044396 and Internet gaming disorder in Korean male adults. PLoS One (2017) 12:e0188358. doi: 10.1371/journal.pone.0188358
216. Fletcher PJ, Li Z, Silenieks LB, Macmillan C, Delannoy I, Higgins GA. Preclinical evidence for combining the 5-HT2C receptor agonist lorcaserin and varenicline as a treatment for nicotine dependence. Addict Biol (2019) 24:376–87. doi: 10.1111/adb.12602
217. Ohmura Y, Sasamori H, Tsutsui-Kimura I, Izumi T, Yoshida T, Yoshioka M. Varenicline provokes impulsive action by stimulating alpha4beta2 nicotinic acetylcholine receptors in the infralimbic cortex in a nicotine exposure status-dependent manner. Pharmacol Biochem Behav (2017) 154:1–10. doi: 10.1016/j.pbb.2017.01.002
218. Tsutsui-Kimura I, Ohmura Y, Izumi T, Yamaguchi T, Yoshida T, Yoshioka M. Endogenous acetylcholine modulates impulsive action via alpha4beta2 nicotinic acetylcholine receptors in rats. Eur J Pharmacol (2010) 641:148–53. doi: 10.1016/j.ejphar.2010.05.028
219. Levin ED, Rezvani AH, Wells C, Slade S, Yenugonda VM, Liu Y, et al. Alpha4beta2 nicotinic receptor desensitizing compounds can decrease self-administration of cocaine and methamphetamine in rats. Eur J Pharmacol (2018) 845:1–7. doi: 10.1016/j.ejphar.2018.12.010
220. Hayward A, Adamson L, Neill JC. Partial agonism at the alpha7 nicotinic acetylcholine receptor improves attention, impulsive action and vigilance in low attentive rats. Eur Neuropsychopharmacol (2017) 27:325–35. doi: 10.1016/j.euroneuro.2017.01.013
221. Levin ED, Bradley A, Addy N, Sigurani N. Hippocampal alpha 7 and alpha 4 beta 2 nicotinic receptors and working memory. Neuroscience (2002) 109:757–65. doi: 10.1016/S0306-4522(01)00538-3
222. Nikiforuk A, Kos T, Potasiewicz A, Popik P. Positive allosteric modulation of alpha 7 nicotinic acetylcholine receptors enhances recognition memory and cognitive flexibility in rats. Eur Neuropsychopharmacol (2015) 25:1300–13. doi: 10.1016/j.euroneuro.2015.04.018
223. Love TM, Stohler CS, Zubieta JK. Positron emission tomography measures of endogenous opioid neurotransmission and impulsiveness traits in humans. Arch Gen Psychiatry (2009) 66:1124–34. doi: 10.1001/archgenpsychiatry.2009.134
224. Mick I, Myers J, Ramos AC, Stokes PR, Erritzoe D, Colasanti A, et al. Blunted endogenous opioid release following an oral amphetamine challenge in pathological gamblers. Neuropsychopharmacology (2016) 41:1742–50. doi: 10.1038/npp.2015.340
225. Kim SW, Grant JE, Adson DE, Shin YC. Double-blind naltrexone and placebo comparison study in the treatment of pathological gambling. Biol Psychiatry (2001) 49:914–21. doi: 10.1016/S0006-3223(01)01079-4
226. Yoon G, Kim SW. Monthly injectable naltrexone for pathological gambling. Am J Psychiatry (2013) 170:682–3. doi: 10.1176/appi.ajp.2013.12111469
227. Baldo BA. Prefrontal cortical opioids and dysregulated motivation: a network hypothesis. Trends Neurosci (2016) 39:366–77. doi: 10.1016/j.tins.2016.03.004
228. Selleck RA, Lake C, Estrada V, Riederer J, Andrzejewski M, Sadeghian K, et al. Endogenous opioid signaling in the medial prefrontal cortex is required for the expression of hunger-induced impulsive action. Neuropsychopharmacology (2015) 40:2464–74. doi: 10.1038/npp.2015.97
229. Olmstead MC, Ouagazzal AM, Kieffer BL. Mu and delta opioid receptors oppositely regulate motor impulsivity in the signaled nose poke task. PLoS One (2009) 4:e4410. doi: 10.1371/journal.pone.0004410
230. Funk D, Tamadon S, Coen K, Fletcher PJ, Le AD. Kappa opioid receptors mediate yohimbine-induced increases in impulsivity in the 5-choice serial reaction time task. Behav Brain Res (2019) 359:258–65. doi: 10.1016/j.bbr.2018.11.006
231. Unterwald EM, Rubenfeld JM, Kreek MJ. Repeated cocaine administration upregulates kappa and mu, but not delta, opioid receptors. Neuroreport (1994) 5:1613–16. doi: 10.1097/00001756-199408150-00018
232. Ghitza UE, Fabbricatore AT, Prokopenko V, Pawlak AP, West MO. Persistent cue-evoked activity of accumbens neurons after prolonged abstinence from self-administered cocaine. J Neurosci. (2003) 23:7239–45. doi: 10.1523/JNEUROSCI.23-19-07239.2003
233. Gorelick DA, Kim YK, Bencherif B, Boyd SJ, Nelson R, Copersino M, et al. Imaging brain mu-opioid receptors in abstinent cocaine users: time course and relation to cocaine craving. Biol Psychiatry (2005) 57:1573–82. doi: 10.1016/j.biopsych.2005.02.026
234. Norman H, D’Souza MS. Endogenous opioid system: a promising target for future smoking cessation medications. Psychopharmacology (Berl) (2017) 234:1371–94. doi: 10.1007/s00213-017-4582-0
235. Garcia-Cabrerizo R, Garcia-Fuster MJ. Opposite regulation of cannabinoid CB1 and CB2 receptors in the prefrontal cortex of rats treated with cocaine during adolescence. Neurosci Lett (2016) 615:60–5. doi: 10.1016/j.neulet.2016.01.018
236. Bystrowska B, Frankowska M, Smaga I, Pomierny-Chamiolo L, Filip M. Effects of cocaine self-administration and its extinction on the rat brain cannabinoid CB1 and CB2 receptors. Neurotox Res (2018) 34:547–58. doi: 10.1007/s12640-018-9910-6
237. Mcreynolds JR, Doncheck EM, Vranjkovic O, Ganzman GS, Baker DA, Hillard CJ, et al. CB1 receptor antagonism blocks stress-potentiated reinstatement of cocaine seeking in rats. Psychopharmacology (Berl) (2016) 233:99–109. doi: 10.1007/s00213-015-4092-x
238. Hindocha C, Freeman TP, Grabski M, Stroud JB, Crudgington H, Davies AC, et al. Cannabidiol reverses attentional bias to cigarette cues in a human experimental model of tobacco withdrawal. Addiction (2018). doi: 10.1111/add.14243
239. Silva AJ, Kogan JH, Frankland PW, Kida S. CREB and memory. Annu Rev Neurosci (1998) 21:127–48. doi: 10.1146/annurev.neuro.21.1.127
240. Nestler EJ. Molecular neurobiology of addiction. Am J Addict (2001) 10:201–17. doi: 10.1080/105504901750532094
241. Kandel ER. The molecular biology of memory: cAMP, PKA, CRE, CREB-1, CREB-2, and CPEB. Mol Brain (2012) 5:14. doi: 10.1186/1756-6606-5-14
242. Gurney ME, D’Amato EC, Burgin AB. Phosphodiesterase-4 (PDE4) molecular pharmacology and Alzheimer’s disease. Neurotherapeutics (2015) 12:49–56. doi: 10.1007/s13311-014-0309-7
243. Monti B, Berteotti C, Contestabile A. Subchronic rolipram delivery activates hippocampal CREB and arc, enhances retention and slows down extinction of conditioned fear. Neuropsychopharmacology (2006) 31:278–86. doi: 10.1038/sj.npp.1300813
244. Yeoh JW, Campbell EJ, James MH, Graham BA, Dayas CV. Orexin antagonists for neuropsychiatric disease: progress and potential pitfalls. Front Neurosci (2014) 8:36. doi: 10.3389/fnins.2014.00036
245. James MH, Campbell EJ, Dayas CV. Role of the orexin/hypocretin system in stress-related psychiatric disorders. Curr Top Behav Neurosci (2017) 33:197–219. doi: 10.1007/7854_2016_56
246. Gentile TA, Simmons SJ, Barker DJ, Shaw JK, Espana RA, Muschamp JW. Suvorexant, an orexin/hypocretin receptor antagonist, attenuates motivational and hedonic properties of cocaine. Addict Biol (2018a) 23:247–55. doi: 10.1111/adb.12507
247. Schmeichel BE, Matzeu A, Koebel P, Vendruscolo LF, Sidhu H, Shahryari R, et al. Knockdown of hypocretin attenuates extended access of cocaine self-administration in rats. Neuropsychopharmacology (2018) 43:2373–82. doi: 10.1038/s41386-018-0054-4
248. Bernstein DL, Badve PS, Barson JR, Bass CE, Espana RA. Hypocretin receptor 1 knockdown in the ventral tegmental area attenuates mesolimbic dopamine signaling and reduces motivation for cocaine. Addict Biol (2017) 23:1032–45. doi: 10.1111/adb.12553
249. Alijanpour S, Tirgar F, Zarrindast MR. Role of dorsal hippocampal orexin-1 receptors in memory restoration induced by morphine sensitization phenomenon. Neuroscience (2016) 312:215–26. doi: 10.1016/j.neuroscience.2015.11.023
250. Moorman DE, James MH, Kilroy EA, Aston-Jones G. Orexin/hypocretin-1 receptor antagonism reduces ethanol self-administration and reinstatement selectively in highly-motivated rats. Brain Res (2017) 1654:34–42. doi: 10.1016/j.brainres.2016.10.018
251. Freeman LR, Aston-Jones G. Activation of medial hypothalamic orexin neurons during a Go/No-Go task. Brain Res (2018). doi: 10.1016/j.brainres.2018.08.031
252. Deadwyler SA, Porrino L, Siegel JM, Hampson RE. Systemic and nasal delivery of orexin-A (hypocretin-1) reduces the effects of sleep deprivation on cognitive performance in nonhuman primates. J Neurosci (2007) 27:14239–47. doi: 10.1523/JNEUROSCI.3878-07.2007
253. Fronczek R, Van Geest S, Frolich M, Overeem S, Roelandse FW, Lammers GJ, et al. Hypocretin (orexin) loss in Alzheimer’s disease. Neurobiol Aging (2012) 33:1642–50. doi: 10.1016/j.neurobiolaging.2011.03.014
254. Aitta-Aho T, Pappa E, Burdakov D, Apergis-Schoute J. Cellular activation of hypothalamic hypocretin/orexin neurons facilitates short-term spatial memory in mice. Neurobiol Learn Mem (2016) 136:183–8. doi: 10.1016/j.nlm.2016.10.005
255. Chen XY, Chen L, Du YF. Orexin-A increases the firing activity of hippocampal CA1 neurons through orexin-1 receptors. J Neurosci Res (2017) 95:1415–26. doi: 10.1002/jnr.23975
256. Mavanji V, Butterick TA, Duffy CM, Nixon JP, Billington CJ, Kotz CM. Orexin/hypocretin treatment restores hippocampal-dependent memory in orexin-deficient mice. Neurobiol Learn Mem (2017) 146:21–30. doi: 10.1016/j.nlm.2017.10.014
257. Ito N, Yabe T, Gamo Y, Nagai T, Oikawa T, Yamada H, et al. I.c.v. administration of orexin-A induces an antidepressive-like effect through hippocampal cell proliferation. Neuroscience (2008) 157:720–32. doi: 10.1016/j.neuroscience.2008.09.042
258. Flores A, Herry C, Maldonado R, Berrendero F. Facilitation of contextual fear extinction by orexin-1 receptor antagonism is associated with the activation of specific amygdala cell subpopulations. Int J Neuropsychopharmacol (2017) 20:654–9. doi: 10.1093/ijnp/pyx029
259. Hofer M, Pagliusi SR, Hohn A, Leibrock J, Barde YA. Regional distribution of brain-derived neurotrophic factor mRNA in the adult mouse brain. EMBO J (1990) 9:2459–64. doi: 10.1002/j.1460-2075.1990.tb07423.x
260. Hall FS, Drgonova J, Goeb M, Uhl GR. Reduced behavioral effects of cocaine in heterozygous brain-derived neurotrophic factor (BDNF) knockout mice. Neuropsychopharmacology (2003) 28:1485–90. doi: 10.1038/sj.npp.1300192
261. St Laurent R, Helm SR, Glenn MJ. Reduced cocaine-seeking behavior in heterozygous BDNF knockout rats. Neurosci Lett (2013) 544:94–9. doi: 10.1016/j.neulet.2013.03.050
262. Verheij MM, Vendruscolo LF, Caffino L, Giannotti G, Cazorla M, Fumagalli F, et al. Systemic delivery of a brain-penetrant TrkB antagonist reduces cocaine self-administration and normalizes TrkB signaling in the nucleus accumbens and prefrontal cortex. J Neurosci (2016) 36:8149–59. doi: 10.1523/JNEUROSCI.2711-14.2016
263. Li X, Rubio FJ, Zeric T, Bossert JM, Kambhampati S, Cates HM, et al. Incubation of methamphetamine craving is associated with selective increases in expression of Bdnf and trkb, glutamate receptors, and epigenetic enzymes in cue-activated fos-expressing dorsal striatal neurons. J Neurosci (2015a) 35:8232–44. doi: 10.1523/JNEUROSCI.1022-15.2015
264. Manning EE, Van Den Buuse M. Altered social cognition in male BDNF heterozygous mice and following chronic methamphetamine exposure. Behav Brain Res (2016) 305:181–5. doi: 10.1016/j.bbr.2016.03.014
265. Parikh V, Cole RD, Patel PJ, Poole RL, Gould TJ. Cognitive control deficits during mecamylamine-precipitated withdrawal in mice: possible links to frontostriatal BDNF imbalance. Neurobiol Learn Mem (2016) 128:110–6. doi: 10.1016/j.nlm.2016.01.003
266. Ozawa T, Yamada K, Ichitani Y. Hippocampal BDNF treatment facilitates consolidation of spatial memory in spontaneous place recognition in rats. Behav Brain Res (2014) 263:210–6. doi: 10.1016/j.bbr.2014.01.034
267. Mu JS, Li WP, Yao ZB, Zhou XF. Deprivation of endogenous brain-derived neurotrophic factor results in impairment of spatial learning and memory in adult rats. Brain Res (1999) 835:259–65. doi: 10.1016/S0006-8993(99)01592-9
268. Heldt SA, Stanek L, Chhatwal JP, Ressler KJ. Hippocampus-specific deletion of BDNF in adult mice impairs spatial memory and extinction of aversive memories. Mol Psychiatry (2007) 12:656–70. doi: 10.1038/sj.mp.4001957
269. Peters J, Dieppa-Perea LM, Melendez LM, Quirk GJ. Induction of fear extinction with hippocampal–infralimbic BDNF. Science (2010) 328:1288–90. doi: 10.1126/science.1186909
270. Rosas-Vidal LE, Do-Monte FH, Sotres-Bayon F, Quirk GJ. Hippocampal–prefrontal BDNF and memory for fear extinction. Neuropsychopharmacology (2014) 39:2161–9. doi: 10.1038/npp.2014.64
271. Roberto M, Cruz MT, Gilpin NW, Sabino V, Schweitzer P, Bajo M, et al. Corticotropin releasing factor-induced amygdala gamma-aminobutyric acid release plays a key role in alcohol dependence. Biol Psychiatry (2010) 67(9):831–9. doi: 10.1016/j.biopsych.2009.11.007
272. Zorrilla EP, Logrip ML, Koob GF. Corticotropin releasing factor: a key role in the neurobiology of addiction. Front Neuroendocrinol (2014) 35:234–44. doi: 10.1016/j.yfrne.2014.01.001
273. Bruijnzeel AW. Neuropeptide systems and new treatments for nicotine addiction. Psychopharmacology (Berl) (2017) 234:1419–37. doi: 10.1007/s00213-016-4513-5
274. Boyson CO, Holly EN, Shimamoto A, Albrechet-Souza L, Weiner LA, Debold JF, et al. Social stress and CRF-dopamine interactions in the VTA: role in long-term escalation of cocaine self-administration. J Neurosci (2014) 34:6659–67. doi: 10.1523/JNEUROSCI.3942-13.2014
275. Contarino A, Kitchener P, Vallee M, Papaleo F, Piazza PV. CRF1 receptor-deficiency increases cocaine reward. Neuropharmacology (2017) 117:41–8. doi: 10.1016/j.neuropharm.2017.01.024
276. Morisot N, Millan MJ, Contarino A. CRF1 receptor-deficiency induces anxiety-like vulnerability to cocaine. Psychopharmacology (Berl) (2014b) 231:3965–72. doi: 10.1007/s00213-014-3534-1
277. Morisot N, Le Moine C, Millan MJ, Contarino A. CRF(2) receptor-deficiency reduces recognition memory deficits and vulnerability to stress induced by cocaine withdrawal. Int J Neuropsychopharmacol (2014a) 17:1969–79. doi: 10.1017/S1461145714000625
278. Swanson LW, Sawchenko PE, Rivier J, Vale WW. Organization of ovine corticotropin-releasing factor immunoreactive cells and fibers in the rat brain: an immunohistochemical study. Neuroendocrinology (1983) 36:165–86. doi: 10.1159/000123454
279. Hupalo S, Berridge CW. Working memory impairing actions of corticotropin-releasing factor (CRF) neurotransmission in the prefrontal cortex. Neuropsychopharmacology (2016) 41:2733–40. doi: 10.1038/npp.2016.85
280. Williams CL, Buchta WC, Riegel AC. CRF-R2 and the heterosynaptic regulation of VTA glutamate during reinstatement of cocaine seeking. J Neurosci (2014) 34:10402–14. doi: 10.1523/JNEUROSCI.0911-13.2014
281. Abiri D, Douglas CE, Calakos KC, Barbayannis G, Roberts A, Bauer EP. Fear extinction learning can be impaired or enhanced by modulation of the CRF system in the basolateral nucleus of the amygdala. Behav Brain Res (2014) 271:234–9. doi: 10.1016/j.bbr.2014.06.021
282. Salling MC, Martinez D. Brain stimulation in addiction. Neuropsychopharmacology (2016) 41:2798–809. doi: 10.1038/npp.2016.80
283. Shields J, Mock J, Devier D, Foundas A. Unilateral repetitive transcranial magnetic stimulation differentially affects younger and older adults completing a verbal working memory task. J Neurol Sci (2018) 384:15–20. doi: 10.1016/j.jns.2017.10.021
284. Liu A, Jain N, Vyas A, Lim LW. Ventromedial prefrontal cortex stimulation enhances memory and hippocampal neurogenesis in the middle-aged rats. Elife (2015) 4. doi: 10.7554/eLife.04803
285. Garcia-Brito S, Morgado-Bernal I, Biosca-Simon N, Segura-Torres P. Intracranial self-stimulation also facilitates learning in a visual discrimination task in the Morris water maze in rats. Behav Brain Res (2017) 317:360–6. doi: 10.1016/j.bbr.2016.09.069
286. Rodriguez-Romaguera J, Do Monte FH, Quirk GJ. Deep brain stimulation of the ventral striatum enhances extinction of conditioned fear. Proc Natl Acad Sci U S A (2012) 109:8764–9. doi: 10.1073/pnas.1200782109
287. Rodriguez-Romaguera J, Do-Monte FH, Tanimura Y, Quirk GJ, Haber SN. Enhancement of fear extinction with deep brain stimulation: evidence for medial orbitofrontal involvement. Neuropsychopharmacology (2015) 40:1726–33. doi: 10.1038/npp.2015.20
288. Guercio LA, Schmidt HD, Pierce RC. Deep brain stimulation of the nucleus accumbens shell attenuates cue-induced reinstatement of both cocaine and sucrose seeking in rats. Behav Brain Res (2015) 281:125–30. doi: 10.1016/j.bbr.2014.12.025
289. Martinez-Rivera FJ, Rodriguez-Romaguera J, Lloret-Torres ME, Do Monte FH, Quirk GJ, Barreto-Estrada JL. Bidirectional modulation of extinction of drug seeking by deep brain stimulation of the ventral striatum. Biol Psychiatry (2016) 80:682–90. doi: 10.1016/j.biopsych.2016.05.015
290. Cao B, Wang J, Shahed M, Jelfs B, Chan RH, Li Y. Vagus nerve stimulation alters phase synchrony of the anterior cingulate cortex and facilitates decision making in rats. Sci Rep (2016) 6:35135. doi: 10.1038/srep35135
291. Adams WK, Vonder Haar C, Tremblay M, Cocker PJ, Silveira MM, Kaur S, et al. Deep-brain stimulation of the subthalamic nucleus selectively decreases risky choice in risk-preferring rats. eNeuro (2017b) 4(4):ENEURO.0094-17. doi: 10.1523/ENEURO.0094-17.2017
292. Zlebnik NE, Carroll ME. Prevention of the incubation of cocaine seeking by aerobic exercise in female rats. Psychopharmacology (Berl) (2015b) 232:3507–13. doi: 10.1007/s00213-015-3999-6
293. Beiter RM, Peterson AB, Abel J, Lynch WJ. Exercise during early, but not late abstinence, attenuates subsequent relapse vulnerability in a rat model. Transl Psychiatry (2016) 6:e792. doi: 10.1038/tp.2016.58
294. Zlebnik NE, Carroll ME. Effects of the combination of wheel running and atomoxetine on cue- and cocaine-primed reinstatement in rats selected for high or low impulsivity. Psychopharmacology (Berl) (2015a) 232:1049–59. doi: 10.1007/s00213-014-3744-6
295. Ogbonmwan YE, Schroeder JP, Holmes PV, Weinshenker D. The effects of post-extinction exercise on cocaine-primed and stress-induced reinstatement of cocaine seeking in rats. Psychopharmacology (Berl) (2015) 232:1395–403. doi: 10.1007/s00213-014-3778-9
296. Nasehi M, Nasehi M, Rahmani-Nia F, Mirzaei B, Torabi-Nami M, Zarrindast MR. Swimming improves the emotional memory deficit by scopolamine via mu opioid receptors. Physiol Behav (2014) 128:237–46. doi: 10.1016/j.physbeh.2014.02.011
297. Mokhtari-Zaer A, Ghodrati-Jaldbakhan S, Vafaei AA, Miladi-Gorji H, Akhavan MM, Bandegi AR, et al. Effects of voluntary and treadmill exercise on spontaneous withdrawal signs, cognitive deficits and alterations in apoptosis-associated proteins in morphine-dependent rats. Behav Brain Res (2014) 271:160–70. doi: 10.1016/j.bbr.2014.05.061
298. Bouchet CA, Lloyd BA, Loetz EC, Farmer CE, Ostrovskyy M, Haddad N, et al. Acute exercise enhances the consolidation of fear extinction memory and reduces conditioned fear relapse in a sex-dependent manner. Learn Mem (2017) 24:358–68. doi: 10.1101/lm.045195.117
299. Faria RS, Bereta ALB, Reis GHT, Santos LBB, Pereira MSG, Cortez PJO, et al. Effects of swimming exercise on the extinction of fear memory in rats. J Neurophysiol (2018) 120:2649–53. doi: 10.1152/jn.00586.2018
300. Mandyam CD, Koob GF. The addicted brain craves new neurons: putative role for adult-born progenitors in promoting recovery. Trends Neurosci (2012) 35:250–60. doi: 10.1016/j.tins.2011.12.005
301. Cohen A, Soleiman MT, Talia R, Koob GF, George O, Mandyam CD. Extended access nicotine self-administration with periodic deprivation increases immature neurons in the hippocampus. Psychopharmacology (Berl) (2015) 232:453–63. doi: 10.1007/s00213-014-3685-0
302. Garcia-Fuster MJ, Parsegian A, Watson SJ, Akil H, Flagel SB. Adolescent cocaine exposure enhances goal-tracking behavior and impairs hippocampal cell genesis selectively in adult bred low-responder rats. Psychopharmacology (Berl) (2017) 234:1293–305. doi: 10.1007/s00213-017-4566-0
303. Takashima Y, Mandyam CD. The role of hippocampal adult neurogenesis in methamphetamine addiction. Brain Plast (2018) 3:157–68. doi: 10.3233/BPL-170058
304. Ladron De Guevara-Miranda D, Millon C, Rosell-Valle C, Perez-Fernandez M, Missiroli M, Serrano A, et al. Long-lasting memory deficits in mice withdrawn from cocaine are concomitant with neuroadaptations in hippocampal basal activity, GABAergic interneurons and adult neurogenesis. Dis Model Mech (2017) 10:323–36. doi: 10.1242/dmm.026682
305. Koyanagi I, Akers KG, Vergara P, Srinivasan S, Sakurai T, Sakaguchi M. Memory consolidation during sleep and adult hippocampal neurogenesis. Neural Regen Res (2019) 14:20–3. doi: 10.4103/1673-5374.243695
306. Pristera A, Saraulli D, Farioli-Vecchioli S, Strimpakos G, Costanzi M, Di Certo MG, et al. Impact of N-tau on adult hippocampal neurogenesis, anxiety, and memory. Neurobiol Aging (2013) 34:2551–63. doi: 10.1016/j.neurobiolaging.2013.05.010
307. Ishikawa R, Fukushima H, Frankland PW, Kida S. Hippocampal neurogenesis enhancers promote forgetting of remote fear memory after hippocampal reactivation by retrieval. Elife (2016) 5:e17464. doi: 10.7554/eLife.17464
308. Li J, Han Z, Cao B, Cai CY, Lin YH, Li F, et al. Disrupting nNOS-PSD-95 coupling in the hippocampal dentate gyrus promotes extinction memory retrieval. Biochem Biophys Res Commun (2017a) 493:862–8. doi: 10.1016/j.bbrc.2017.09.003
309. Zhang Y, Kibaly C, Xu C, Loh HH, Law PY. Temporal effect of manipulating neuroD1 expression with the synthetic small molecule KHS101 on morphine contextual memory. Neuropharmacology (2017) 126:58–69. doi: 10.1016/j.neuropharm.2017.08.030
310. Ladron De Guevara-Miranda D, Moreno-Fernandez RD, Gil-Rodriguez S, Rosell-Valle C, Estivill-Torrus G, Serrano A, et al. Lysophosphatidic acid-induced increase in adult hippocampal neurogenesis facilitates the forgetting of cocaine-contextual memory. Addict Biol (2019) 24:458–70. doi: 10.1111/adb.12612
311. Noonan MA, Bulin SE, Fuller DC, Eisch AJ. Reduction of adult hippocampal neurogenesis confers vulnerability in an animal model of cocaine addiction. J Neurosci (2010) 30:304–15. doi: 10.1523/JNEUROSCI.4256-09.2010
312. Panagopoulos VN, Ralevski E. The role of ghrelin in addiction: a review. Psychopharmacology (Berl) (2014) 231:2725–40. doi: 10.1007/s00213-014-3640-0
313. Davis KW, Wellman PJ, Clifford PS. Augmented cocaine conditioned place preference in rats pretreated with systemic ghrelin. Regul Pept (2007) 140:148–52. doi: 10.1016/j.regpep.2006.12.003
314. Jerlhag E, Egecioglu E, Dickson SL, Engel JA. Ghrelin receptor antagonism attenuates cocaine- and amphetamine-induced locomotor stimulation, accumbal dopamine release, and conditioned place preference. Psychopharmacology (Berl) (2010) 211:415–22. doi: 10.1007/s00213-010-1907-7
315. Jerlhag E, Engel JA. Ghrelin receptor antagonism attenuates nicotine-induced locomotor stimulation, accumbal dopamine release and conditioned place preference in mice. Drug Alcohol Depend (2011) 117:126–31. doi: 10.1016/j.drugalcdep.2011.01.010
316. You ZB, Wang B, Gardner EL, Wise RA. Cocaine and cocaine expectancy increase growth hormone, ghrelin, GLP-1, IGF-1, adiponectin, and corticosterone while decreasing leptin, insulin, GIP, and prolactin. Pharmacol Biochem Behav (2019) 176:53–6. doi: 10.1016/j.pbb.2018.11.001
317. Al’absi M, Lemieux A, Nakajima M. Peptide YY and ghrelin predict craving and risk for relapse in abstinent smokers. Psychoneuroendocrinology (2014) 49:253–9. doi: 10.1016/j.psyneuen.2014.07.018
318. Toth K, Laszlo K, Lukacs E, Lenard L. Intraamygdaloid microinjection of acylated-ghrelin influences passive avoidance learning. Behav Brain Res (2009) 202:308–11. doi: 10.1016/j.bbr.2009.03.031
319. Chen L, Xing T, Wang M, Miao Y, Tang M, Chen J, et al. Local infusion of ghrelin enhanced hippocampal synaptic plasticity and spatial memory through activation of phosphoinositide 3-kinase in the dentate gyrus of adult rats. Eur J Neurosci (2011) 33:266–75. doi: 10.1111/j.1460-9568.2010.07491.x
320. Beck B, Pourie G. Ghrelin, neuropeptide Y, and other feeding-regulatory peptides active in the hippocampus: role in learning and memory. Nutr Rev (2013) 71:541–61. doi: 10.1111/nure.12045
321. Huang CC, Chou D, Yeh CM, Hsu KS. Acute food deprivation enhances fear extinction but inhibits long-term depression in the lateral amygdala via ghrelin signaling. Neuropharmacology (2016) 101:36–45. doi: 10.1016/j.neuropharm.2015.09.018
322. Shi L, Deng J, Chen S, Que J, Sun Y, Wang Z, et al. Fasting enhances extinction retention and prevents the return of fear in humans. Transl Psychiatry (2018) 8:214. doi: 10.1038/s41398-018-0260-1
323. Bowen MT, Neumann ID. Rebalancing the addicted brain: oxytocin interference with the neural substrates of addiction. Trends Neurosci (2017) 40:691–708. doi: 10.1016/j.tins.2017.10.003
324. Zhou L, Sun WL, Young AB, Lee K, Mcginty JF, See RE. Oxytocin reduces cocaine seeking and reverses chronic cocaine-induced changes in glutamate receptor function. Int J Neuropsychopharmacol (2014) 18 (1):pyu009. doi: 10.1093/ijnp/pyu009
325. Everett NA, Mcgregor IS, Baracz SJ, Cornish JL. The role of the vasopressin V1A receptor in oxytocin modulation of methamphetamine primed reinstatement. Neuropharmacology (2018) 133:1–11. doi: 10.1016/j.neuropharm.2017.12.036
326. Baracz SJ, Rourke PI, Pardey MC, Hunt GE, Mcgregor IS, Cornish JL. Oxytocin directly administered into the nucleus accumbens core or subthalamic nucleus attenuates methamphetamine-induced conditioned place preference. Behav Brain Res (2012) 228:185–93. doi: 10.1016/j.bbr.2011.11.038
327. Georgiou P, Zanos P, Hourani S, Kitchen I, Bailey A. Cocaine abstinence induces emotional impairment and brain region-specific upregulation of the oxytocin receptor binding. Eur J Neurosci (2016) 44:2446–54. doi: 10.1111/ejn.13348
328. Skuse DH, Gallagher L. Dopaminergic–neuropeptide interactions in the social brain. Trends Cogn Sci (2009) 13:27–35. doi: 10.1016/j.tics.2008.09.007
329. Lahoud N, Maroun M. Oxytocinergic manipulations in corticolimbic circuit differentially affect fear acquisition and extinction. Psychoneuroendocrinology (2013) 38:2184–95. doi: 10.1016/j.psyneuen.2013.04.006
330. Brill-Maoz N, Maroun M. Extinction of fear is facilitated by social presence: synergism with prefrontal oxytocin. Psychoneuroendocrinology (2016) 66:75–81. doi: 10.1016/j.psyneuen.2016.01.003
331. Zhou Y, Bendor JT, Yuferov V, Schlussman SD, Ho A, Kreek MJ. Amygdalar vasopressin mRNA increases in acute cocaine withdrawal: evidence for opioid receptor modulation. Neuroscience (2005) 134:1391–7. doi: 10.1016/j.neuroscience.2005.05.032
332. Subiah CO, Mabandla MV, Phulukdaree A, Chuturgoon AA, Daniels WM. The effects of vasopressin and oxytocin on methamphetamine-induced place preference behaviour in rats. Metab Brain Dis (2012) 27:341–50. doi: 10.1007/s11011-012-9297-7
333. Paban V, Alescio-Lautier B, Devigne C, Soumireu-Mourat B. Fos protein expression induced by intracerebroventricular injection of vasopressin in unconditioned and conditioned mice. Brain Res (1999) 825:115–31. doi: 10.1016/S0006-8993(99)01232-9
334. Brunnlieb C, Munte TF, Tempelmann C, Heldmann M. Vasopressin modulates neural responses related to emotional stimuli in the right amygdala. Brain Res (2013) 1499:29–42. doi: 10.1016/j.brainres.2013.01.009
335. Caldwell HK, Aulino EA, Rodriguez KM, Witchey SK, Yaw AM. Social context, stress, neuropsychiatric disorders, and the vasopressin 1b receptor. Front Neurosci (2017) 11:567. doi: 10.3389/fnins.2017.00567
336. Devito LM, Konigsberg R, Lykken C, Sauvage M, Young WS 3rd, Eichenbaum H. Vasopressin 1b receptor knock-out impairs memory for temporal order. J Neurosci (2009) 29:2676–83. doi: 10.1523/JNEUROSCI.5488-08.2009
337. Stevenson EL, Caldwell HK. The vasopressin 1b receptor and the neural regulation of social behavior. Horm Behav (2012) 61:277–82. doi: 10.1016/j.yhbeh.2011.11.009
338. Barsegyan A, Atsak P, Hornberger WB, Jacobson PB, Van Gaalen MM, Roozendaal B. The vasopressin 1b receptor antagonist a-988315 blocks stress effects on the retrieval of object-recognition memory. Neuropsychopharmacology (2015) 40:1979–89. doi: 10.1038/npp.2015.48
339. Zhou Y, Leri F, Cummins E, Kreek MJ. Individual differences in gene expression of vasopressin, D2 receptor, POMC and orexin: vulnerability to relapse to heroin-seeking in rats. Physiol Behav (2015) 139:127–35. doi: 10.1016/j.physbeh.2014.11.002
340. Brunnlieb C, Nave G, Camerer CF, Schosser S, Vogt B, Munte TF, et al. Vasopressin increases human risky cooperative behavior. Proc Natl Acad Sci U S A (2016) 113:2051–6. doi: 10.1073/pnas.1518825113
341. Chen Y, Tian Q. The role of protein kinase C epsilon in neural signal transduction and neurogenic diseases. Front Med (2011) 5:70–6. doi: 10.1007/s11684-011-0119-9
342. Lee AM, Messing RO. Protein kinase C epsilon modulates nicotine consumption and dopamine reward signals in the nucleus accumbens. Proc Natl Acad Sci U S A (2011) 108:16080–5. doi: 10.1073/pnas.1106277108
343. Miller BW, Wroten MG, Sacramento AD, Silva HE, Shin CB, Vieira PA, et al. Cocaine craving during protracted withdrawal requires PKCepsilon priming within vmPFC. Addict Biol (2017) 22:629–39. doi: 10.1111/adb.12354
344. Hongpaisan J, Xu C, Sen A, Nelson TJ, Alkon DL. PKC activation during training restores mushroom spine synapses and memory in the aged rat. Neurobiol Dis (2013) 55:44–62. doi: 10.1016/j.nbd.2013.03.012
345. Zisopoulou S, Asimaki O, Leondaritis G, Vasilaki A, Sakellaridis N, Pitsikas N, et al. PKC-epsilon activation is required for recognition memory in the rat. Behav Brain Res (2013) 253:280–9. doi: 10.1016/j.bbr.2013.07.036
346. Schmitz JM, Green CE, Hasan KM, Vincent J, Suchting R, Weaver MF, et al. PPAR-gamma agonist pioglitazone modifies craving intensity and brain white matter integrity in patients with primary cocaine use disorder: a double-blind randomized controlled pilot trial. Addiction (2017) 112:1861–8. doi: 10.1111/add.13868
347. Naef L, Seabrook L, Hsiao J, Li C, Borgland SL. Insulin in the ventral tegmental area reduces cocaine-evoked dopamine in the nucleus accumbens in vivo. Eur J Neurosci. (2018). doi: 10.1111/ejn.14291
348. Stern SA, Chen DY, Alberini CM. The effect of insulin and insulin-like growth factors on hippocampus- and amygdala-dependent long-term memory formation. Learn Mem (2014a) 21:556–63. doi: 10.1101/lm.029348.112
349. Stern SA, Kohtz AS, Pollonini G, Alberini CM. Enhancement of memories by systemic administration of insulin-like growth factor II. Neuropsychopharmacology (2014b) 39:2179–90. doi: 10.1038/npp.2014.69
350. Avgerinos KI, Kalaitzidis G, Malli A, Kalaitzoglou D, Myserlis PG, Lioutas VA. Intranasal insulin in Alzheimer’s dementia or mild cognitive impairment: a systematic review. J Neurol (2018) 265:1497–510. doi: 10.1007/s00415-018-8768-0
351. Read S, Wu P, Biscow M. Sustained 4-year cognitive and functional response in early Alzheimer’s disease with pioglitazone. J Am Geriatr Soc (2014) 62:584–6. doi: 10.1111/jgs.12722
352. Nenov MN, Laezza F, Haidacher SJ, Zhao Y, Sadygov RG, Starkey JM, et al. Cognitive enhancing treatment with a PPARgamma agonist normalizes dentate granule cell presynaptic function in Tg2576 APP mice. J Neurosci (2014) 34:1028–36. doi: 10.1523/JNEUROSCI.3413-13.2014
353. Nenov MN, Tempia F, Denner L, Dineley KT, Laezza F. Impaired firing properties of dentate granule neurons in an Alzheimer’s disease animal model are rescued by PPARgamma agonism. J Neurophysiol (2015) 113:1712–26. doi: 10.1152/jn.00419.2014
354. Cippitelli A, Domi E, Ubaldi M, Douglas JC, Li HW, Demopulos G, et al. Protection against alcohol-induced neuronal and cognitive damage by the PPARgamma receptor agonist pioglitazone. Brain Behav Immun (2017) 64:320–9. doi: 10.1016/j.bbi.2017.02.001
355. De Guglielmo G, Kallupi M, Scuppa G, Demopulos G, Gaitanaris G, Ciccocioppo R. Pioglitazone attenuates the opioid withdrawal and vulnerability to relapse to heroin seeking in rodents. Psychopharmacology (Berl) (2017) 234:223–34. doi: 10.1007/s00213-016-4452-1
356. Agis-Balboa RC, Arcos-Diaz D, Wittnam J, Govindarajan N, Blom K, Burkhardt S, et al. A hippocampal insulin-growth factor 2 pathway regulates the extinction of fear memories. EMBO J (2011) 30:4071–83. doi: 10.1038/emboj.2011.293
357. Agis-Balboa RC, Fischer A. Generating new neurons to circumvent your fears: the role of IGF signaling. Cell Mol Life Sci (2014) 71:21–42. doi: 10.1007/s00018-013-1316-2
358. Sen N. Epigenetic regulation of memory by acetylation and methylation of chromatin: implications in neurological disorders, aging, and addiction. Neuromolecular Med (2015) 17:97–110. doi: 10.1007/s12017-014-8306-x
359. Wright KN, Hollis F, Duclot F, Dossat AM, Strong CE, Francis TC, et al. Methyl supplementation attenuates cocaine-seeking behaviors and cocaine-induced c-Fos activation in a DNA methylation-dependent manner. J Neurosci (2015) 35:8948–58. doi: 10.1523/JNEUROSCI.5227-14.2015
360. Barbier E, Johnstone AL, Khomtchouk BB, Tapocik JD, Pitcairn C, Rehman F, et al. Dependence-induced increase of alcohol self-administration and compulsive drinking mediated by the histone methyltransferase PRDM2. Mol Psychiatry (2017) 22:1746–58. doi: 10.1038/mp.2016.131
361. Woldemichael BT, Bohacek J, Gapp K, Mansuy IM. Epigenetics of memory and plasticity. Prog Mol Biol Transl Sci (2014) 122:305–40. doi: 10.1016/B978-0-12-420170-5.00011-8
362. Marek R, Coelho CM, Sullivan RK, Baker-Andresen D, Li X, Ratnu V, et al. Paradoxical enhancement of fear extinction memory and synaptic plasticity by inhibition of the histone acetyltransferase p300. J Neurosci (2011) 31:7486–91. doi: 10.1523/JNEUROSCI.0133-11.2011
363. Im HI, Hollander JA, Bali P, Kenny PJ. MeCP2 controls BDNF expression and cocaine intake through homeostatic interactions with microRNA-212. Nat Neurosci (2010) 13:1120–7. doi: 10.1038/nn.2615
364. Sadakierska-Chudy A, Frankowska M, Miszkiel J, Wydra K, Jastrzebska J, Filip M. Prolonged induction of miR-212/132 and REST expression in rat striatum following cocaine self-administration. Mol Neurobiol (2017) 54:2241–54. doi: 10.1007/s12035-016-9817-2
365. Sim MS, Soga T, Pandy V, Wu YS, Parhar IS, Mohamed Z. MicroRNA expression signature of methamphetamine use and addiction in the rat nucleus accumbens. Metab Brain Dis (2017) 32:1767–83. doi: 10.1007/s11011-017-0061-x
366. Pietrzykowski AZ, Spijker S. Impulsivity and comorbid traits: a multi-step approach for finding putative responsible microRNAs in the amygdala. Front Neurosci (2014) 8:389. doi: 10.3389/fnins.2014.00389
367. Nemeth N, Kovacs-Nagy R, Szekely A, Sasvari-Szekely M, Ronai Z. Association of impulsivity and polymorphic microRNA-641 target sites in the SNAP-25 gene. PLoS One (2013) 8:e84207. doi: 10.1371/journal.pone.0084207
368. Sanchez-Mora C, Ramos-Quiroga JA, Garcia-Martinez I, Fernandez-Castillo N, Bosch R, Richarte V, et al. Evaluation of single nucleotide polymorphisms in the miR-183-96-182 cluster in adulthood attention-deficit and hyperactivity disorder (ADHD) and substance use disorders (SUDs). Eur Neuropsychopharmacol (2013) 23:1463–73. doi: 10.1016/j.euroneuro.2013.07.002
370. Sim SE, Lim CS, Kim JI, Seo D, Chun H, Yu NK, et al. The brain-enriched microRNA miR-9-3p regulates synaptic plasticity and memory. J Neurosci (2016) 36:8641–52. doi: 10.1523/JNEUROSCI.0630-16.2016
371. Murphy CP, Li X, Maurer V, Oberhauser M, Gstir R, Wearick-Silva LE, et al. MicroRNA-mediated rescue of fear extinction memory by miR-144-3p in extinction-impaired mice. Biol Psychiatry (2017) 81:979–9. doi: 10.1016/j.biopsych.2016.12.021
372. Lin Q, Wei W, Coelho CM, Li X, Baker-Andresen D, Dudley K, et al. The brain-specific microRNA miR-128b regulates the formation of fear-extinction memory. Nat Neurosci (2011) 14:1115–7. doi: 10.1038/nn.2891
373. Quinn RK, James MH, Hawkins GE, Brown AL, Heathcote A, Smith DW, et al. Temporally specific miRNA expression patterns in the dorsal and ventral striatum of addiction-prone rats. Addict Biol (2018) 23:631–42. doi: 10.1111/adb.12520
374. Weafer J, De Wit H. Sex differences in impulsive action and impulsive choice. Addict Behav (2014) 39:1573–9. doi: 10.1016/j.addbeh.2013.10.033
375. Swalve N, Smethells JR, Carroll ME. Progesterone attenuates impulsive action in a Go/No-Go task for sucrose pellets in female and male rats. Horm Behav (2016) 85:43–7. doi: 10.1016/j.yhbeh.2016.08.001
376. Hammerslag LR, Waldman AJ, Gulley JM. Effects of amphetamine exposure in adolescence or young adulthood on inhibitory control in adult male and female rats. Behav Brain Res (2014) 263:22–33. doi: 10.1016/j.bbr.2014.01.015
377. Fenton GE, Halliday DM, Mason R, Bredy TW, Stevenson CW. Sex differences in learned fear expression and extinction involve altered gamma oscillations in medial prefrontal cortex. Neurobiol Learn Mem (2016) 135:66–72. doi: 10.1016/j.nlm.2016.06.019
378. Graham BM, Daher M. Estradiol and progesterone have opposing roles in the regulation of fear extinction in female rats. Neuropsychopharmacology (2016) 41:774–80. doi: 10.1038/npp.2015.202
379. Milad MR, Igoe SA, Lebron-Milad K, Novales JE. Estrous cycle phase and gonadal hormones influence conditioned fear extinction. Neuroscience (2009) 164:887–95. doi: 10.1016/j.neuroscience.2009.09.011
380. Zeidan MA, Igoe SA, Linnman C, Vitalo A, Levine JB, Klibanski A, et al. Estradiol modulates medial prefrontal cortex and amygdala activity during fear extinction in women and female rats. Biol Psychiatry (2011) 70:920–7. doi: 10.1016/j.biopsych.2011.05.016
381. Takashima Y, Tseng J, Fannon MJ, Purohit DC, Quach LW, Terranova MJ, et al. Sex differences in context-driven reinstatement of methamphetamine seeking is associated with distinct neuroadaptations in the dentate gyrus. Brain Sci (2018) 8. doi: 10.3390/brainsci8120208
382. Anker JJ, Carroll ME. Sex differences in the effects of allopregnanolone on yohimbine-induced reinstatement of cocaine seeking in rats. Drug Alcohol Depend (2010) 107:264–7. doi: 10.1016/j.drugalcdep.2009.11.002
Keywords: cocaine, nicotine, methamphetamine, memory, extinction, nucleus accumbens, prefrontal cortex
Citation: D’Souza MS (2019) Brain and Cognition for Addiction Medicine: From Prevention to Recovery Neural Substrates for Treatment of Psychostimulant-Induced Cognitive Deficits. Front. Psychiatry 10:509. doi: 10.3389/fpsyt.2019.00509
Received: 14 January 2019; Accepted: 28 June 2019;
Published: 24 July 2019.
Edited by:
Antonio Verdejo-García, Monash University, AustraliaReviewed by:
Chiara Montemitro, G. d’Annunzio University of Chieti and Pescara, ItalyJanice Bartholomew, Teesside University, United Kingdom
Jane Elizabeth Joseph, Medical University of South Carolina, United States
Copyright © 2019 D’Souza. This is an open-access article distributed under the terms of the Creative Commons Attribution License (CC BY). The use, distribution or reproduction in other forums is permitted, provided the original author(s) and the copyright owner(s) are credited and that the original publication in this journal is cited, in accordance with accepted academic practice. No use, distribution or reproduction is permitted which does not comply with these terms.
*Correspondence: Manoranjan S. D’Souza, bS1kc291emFAb251LmVkdQ==