Commentary: Sleep Disturbance in Bipolar Disorder: Neuroglia and Circadian Rhythms
- 1Psychiatric Unit, Department of Health Sciences, University Magna Graecia, Catanzaro, Italy
- 2Department of Medical and Surgical Sciences, University Magna Graecia of Catanzaro, Catanzaro, Italy
- 3Faculty of Biology, Medicine and Health, The University of Manchester, Manchester, United Kingdom
- 4Achucarro Center for Neuroscience, IKERBASQUE, Bilbao, Spain
The worldwide prevalence of sleep disorders is approximately 50%, with an even higher occurrence in a psychiatric population. Bipolar disorder (BD) is a severe mental illness characterized by shifts in mood and activity. The BD syndrome also involves heterogeneous symptomatology, including cognitive dysfunctions and impairments of the autonomic nervous system. Sleep abnormalities are frequently associated with BD and are often a good predictor of a mood swing. Preservation of stable sleep–wake cycles is therefore a key to the maintenance of stability in BD, indicating the crucial role of circadian rhythms in this syndrome. The symptom most widespread in BD is insomnia, followed by excessive daytime sleepiness, nightmares, difficulty falling asleep or maintaining sleep, poor sleep quality, sleep talking, sleep walking, and obstructive sleep apnea. Alterations in the structure or duration of sleep are reported in all phases of BD. Understanding the role of neuroglia in BD and in various aspects of sleep is in nascent state. Contributions of the different types of glial cells to BD and sleep abnormalities are discussed in this paper.
Introduction
Bipolar disorder (BD) is a recurrent disorder that affects in excess of 1% of the world population and usually has its onset in young age. The resulting cognitive deficits, the high risk of suicide, and the occurrence of severe psychiatric and medical comorbidities all make BD one of the major causes of mortality and disability worldwide (1). The concept of BD was introduced at the end of the 19th century by Emil Kraepelin (2) who referred to this disorder as “manic depressive insanity.” About 70 years later, the term “bipolar” was proposed to indicate the condition in which both depression and mania, the opposite poles of mood, alternate in the course of the illness (3). In modern psychiatry, BD is conceptualized as a cyclical mood disorder involving episodes of mania, hypomania, and alternating or intertwining episodes of depression. The last edition of the Diagnostic and Statistical Manual of Mental Disorders (DSM-5) categorizes clinical features of BD according to severity (4). Classical BD type I is identified by the occurrence of major depression and full-blown manic episodes, whereas in BD type II, depression is more prominent, with interspersed episodes of less severe manic symptoms, classified as hypomanic episodes. However, despite this general description, the clinical presentation of BD is polymorphic with regard to symptomatology, progression, efficacy of therapies, and functional outcome. Consequently, the DSM-5 introduces additional specifics for diagnosis, such as BD “with mixed features,” or “with rapid cycling,” or “with melancholic features,” or “with mood congruent or incongruent psychotic features,” to mention only a few. Far from being a discrete diagnostic entity, there is increasing recognition of a spectrum of BDs that ranges from marked and severe mood disturbance into milder mood variations (5). In this context, “cyclothymia” is the term assigned to recurrent hypomanic episodes and subclinical episodes of depression. It represents a subsyndromal condition, although mood disturbance is a continuing problem and interferes with everyday functioning (5). Moreover, unlike previous versions that included BD along with all other mood disorders, DSM-5 now assigns a separate chapter to BD and places it between depressive disorders and the spectrum of schizophrenia and other psychotic disorders. The rationale for this new diagnostic taxonomy stems from the assumption that BD could be considered as a bridge that, in terms of genetics, familiarity, and clinical picture, holds together the other two pathologies, sharing some clinical aspects of both.
Despite numerous studies performed in recent decades, little is known about the etiopathogenetic mechanisms responsible for the BD. The most recent research is focusing on the possible biologic mechanisms underlying the disorder, including genetic components, neurochemical abnormalities, and morphostructural brain differences, along with psychosocial factors, such as life experience and social environment context (6). Hitherto, there is no sufficient explanation to account for the pathobiology of such a multiform condition while the disease heterogeneity prompts us to contemplate multifactorial genesis. Indeed, no single paradigm can explain the occurrence and the variability in course and severity of manic-depressive disorder. Because the key phenotype of BD is a biphasic dysregulation in mood, behavior and sleep remain of great interest and could help expand the understanding of pathogenic mechanisms.
Sleep has a critical significance in the regulation of mood, and sleep disturbances can be seen in BD primarily or because of BD itself (7). These alterations have been linked to a lower quality of life, suicide attempts, poorer clinical and cognitive functioning, and higher relapse rates of mood episodes (8).
Sleep Disorders and Bipolar Disorder: Epidemiology
The “sleep disorders” are defined as every significant alteration of quality of sleep, timing, and quantity, with different adverse impacts on function and quality of life (9). Sleep disturbances are very common in the general population (10). The prevalence of symptoms of sleep disorders range between 41% and 52% worldwide, with the most widespread symptoms being insomnia, followed by excessive daytime sleepiness, nightmares, difficulty falling asleep or maintaining sleep, poor sleep quality, sleep talking, sleep walking, and obstructive sleep apnea (11).
Sleep disorders also have a high prevalence in the psychiatric population. Furthermore, sleep disturbances exert a negative impact on the course and treatment of every psychiatric illness, and aberrant sleep represents a core symptom of BD. For example, 23% to 78% of patients with BD have reported symptoms of hypersomnia (10). The circadian rhythm hypothesis of BD postulates that variability of the circadian rhythms represents a critical step in BD evolution, whereas disturbances in circadian rhythms are considered a core element for the onset and progress of BD (12, 13). It is universally acknowledged that the increased risk of suicidal ideation and manic switch is linked to insomnia (14, 15).
Sleep disturbances are frequent in BD patients in different phases of illness, including the euthymic state (16) and remission (17). These sleep aberrations are represented not only by insomnia but also by sleep–wake rhythm disorders, especially delayed sleep–wake phase disorders (18–20) albeit the disturbance pattern can change with the specific mood phase. During the manic state, most patients (66–99%) experience a reduced need for sleep (21–23) and longer sleep onset latency (7), and vice versa sleep deprivation is well known as a trigger factor for manic episodes (24). Likewise, in the depressive state, insomnia (40–100%) and hypersomnia (23–78%) are commonly observed (25–27). A prevalence of 32.4% of circadian rhythm sleep–wake disorders (CRSWD) was found in a sample of 127 patients affected by BD type I or II, whereas younger onset age of BD and family history of suicide were associated with CRSWD in BD patients (28). Meta-analyses of trials conducted on remitted BD patients demonstrated prolonged total sleep time, increased awakenings after sleep onset, greater variability of sleep–wake variables, and reduced sleep efficiency (16, 29).
Overall, all kinds of sleep disorders and parasomnias are very common especially in youth patients with BD (30). Thus, compared to the general population, youth with BD exhibit lower sleep efficiency, longer slow wave sleep, and reduced REM sleep, features that could affect the genesis and prognosis of the disorder (7, 31). Sleep disturbances may also be used as predictors of the onset of BD in a subset of high-risk young subjects (32).
Circadian Rhythms and Bipolar Disorder
Several types of rhythms rule the human body. Based on the approximate duration, these rhythms can be classified as circadian (about of 24 h), infradian (of longer duration), and ultradian (of shorter length). Temporal organization of physiological, cellular, organ, biochemical, and behavioral processes is controlled by circadian clocks (33).
Endogenously generated circadian rhythms are tuned by and adapted to the environment so that the body is able to synchronize the internal time with the geophysical time. The clock system captures exogenous time signals, called “zeitgebers,” which include the day/night (or light/dark) cycle, temperature, and food intake (33). Environmental information is processed by a central clock, which is located in the anterior region of the hypothalamus, in the suprachiasmatic nuclei (SCN) (34). The central clock receives light and dark information from the visual input through the retino-hypothalamic tract; increased levels of light elevate alertness whereas decreased levels of light reduce sleep latency (35, 36). The processed information is transmitted to the peripheral clocks and to other clocks in the brain (located in other hypothalamic nuclei, thalamus, amygdala) to synchronize all individual endogenous rhythms (33, 37). The stable relationship between internal rhythms and the external environment is ensured by exposure to a normal light–dark schedule (Figure 1) (36). Lack of coordination between the endogenous circadian system and the sleep/wake cycle is a critical factor in the clinical status of illness associated to the disruption of the circadian timing of sleep and the alteration levels of alertness, vigilance, and performance (36, 38). In pathological conditions, the SCN and peripheral clocks lose their normal phase relationship, and thus, a state of internal desynchronization develops that, if sustained, may predispose individuals to a disease (36). The SCN received multiple feedbacks from the periphery that include information regarding metabolic status and the levels of activity (39).
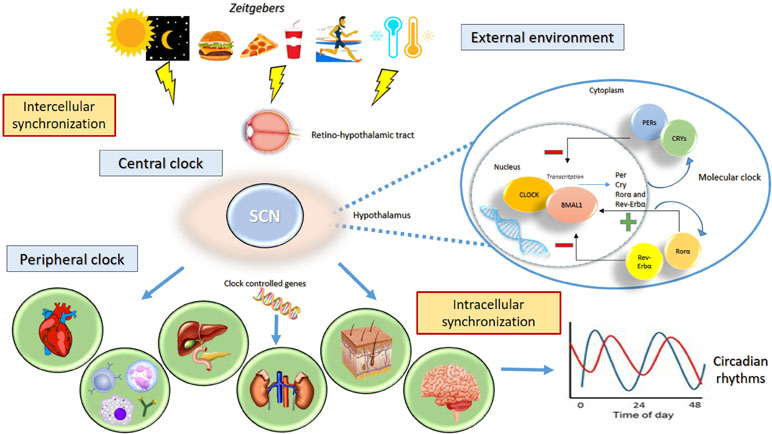
Figure 1 Circadian rhythms. The clock system captures exogenous “zeitgebers” (light/dark cycle, temperature, exercise, food intake) and triggers the central clock in the suprachiasmatic nucleus (SCN) of the hypothalamus through the retino-hypothalamic tract. The activity the group of clock genes governs the generation of circadian rhythms. The genes CLOCK and ARNTL encode the transcription factors CLOCK and ARNTL, which together activate the transcription of Per, Cry, RORα, and REV-ERBα genes. The proteins PER1, PER2, PER3, CRY 1, and CRY 2 combine to inhibit their own transcription, whereas RORα and REV-ERBα act on ARNTL to activate and inhibit transcription, respectively. The processed information is transmitted to the peripheral clocks and to other clocks in the brain to stabilize 24-h periodicity. The stable relationship between internal rhythms and the external environment is needed to ensure the synchronization of individual endogenous rhythms.
Various pathological conditions are associated with sleep and circadian disturbances, including allergies, hypothyroidism and hyperthyroidism, coronary artery disease, congestive heart failure, diabetes, arthritis, asthma, gastroesophageal reflux disease, and chronic pain (40). Disorderly circadian system contributes to the etiology and progression of major psychiatric disorders (38, 41, 42). About three-quarters of individuals with delayed sleep phase syndrome have a past or current history of depression, whereas depression severity correlates with circadian misalignment (43). Patients with different psychiatric conditions, such as anxiety disorders and schizophrenia, often show circadian deregulation contributing to major functional impairments (44).
Sleep disturbances are common in BD with a great variability in sleep duration (45). The decreased need for sleep predicts the onset of a manic or hypomanic episode the following day (46), whereas sleep extension occurs frequently in the depressive episode (13, 16, 47, 48). The disruption in sleep–awake cycle tends to precipitate or exacerbate mood episodes (49), and they are risk factors for the recurrence of a mood episode (Figure 2) (50). Sleep deprivation was also found to induce manic episodes in animal BD models (51, 52). Loss of sleep confers a poor prognosis, increasing the risk of suicide in patients with a suicide attempt history (53). Even in euthymia, sleep alterations occur in BD patients (16). Given all this evidence and based on the rhythmic nature of BD, it has been suggested that the endogenous circadian system may play a role in BD etiology, clinical manifestations, and outcome (42, 54).
The activity of a group of clock genes governs the generation of circadian rhythms. There are molecular positive and negative transcriptional/translational feedback loops that drive the expression of different genes to stabilize 24-h periodicity (55). Several of these clock genes have been linked directly to the abnormal sleep/circadian phenotypes (36). Mutations of any of these circadian genes can potentially have an impact on the circadian clock and thus subtly or dramatically alter sleep, mood, or behavior in ways that contribute to physical and mental illness, and indeed many circadian genes have been associated with BD (56). The strongest evidence for genetic abnormalities is associated with polymorphisms of clock genes and an increased susceptibility to BD (57). In humans, genetic association studies of patients with BD have suggested that polymorphisms in the period gene (PER) is linked to specific phenotypes, such as a good lithium responder (36).
Particularly, a variant of PER3 gene has been linked to different chronotypes. The PER3 gene contains a variable number tandem repeat polymorphism, in which a 54-nucleotide coding-region segment is repeated four (PER4/4) or five (PER 5/5) times. The long allele variant of PER5/5 has been linked to extreme morning chronotypes, whereas the shorter allele PER4/4 is associated with extreme eveningness and delayed sleep phase syndrome (58). Incidentally, the early onset in BD is associated with the longer allele (PER5/5), whereas the later onset is associated with the shorter allele (PER4/4) (36) (Table 1).
One of the consequences of sleep/circadian disruption is an abnormality in the stress axis, with particular emphasis on atypical neurotransmitter release. The hypercortisolemia can arise from a breakdown in glucocorticoid receptor-mediated negative feedback mechanisms in the hypothalamic–pituitary–adrenal (HPA) axis (36). Circadian disturbances, such as a phase advance of the diurnal rhythm of plasma melatonin (72) and plasma cortisol (73), have been observed in BD, although these were not universally confirmed (74). In relation to oxidative stress, circadian rhythm disturbance was associated with increased lipid peroxidation in BD (75). Studying alteration of the wake–sleep rhythm may provide yet unknown insights into the pathophysiology of BD.
Neuroglia in Bipolar Disorder and Sleep Disorders
Neuroglia: An Overview
Neuroglia represent the homeostatic and defensive arm of the nervous system; neuroglial cells of the central nervous system (CNS) are classified into astrocytes, microglia, and oligodendrocytes and their precursors, also known as NG2 glia (76). The functions of neuroglia are diverse; these nonexcitable cells are indispensable companions of neurons, supporting them in physiology and protecting them against pathological lesions. Astrocytes are the main homeostatic cells of the CNS, which control the homeostasis of the nerve tissue at all level of organization from molecular to organ (77, 78). Astroglial perisynaptic processes cover synaptic contacts and form synaptic cradle, which through various mechanisms control synaptogenesis, synaptic maturation, synaptic maintenance, and synaptic extinction (79). Microglial cells invade the neural tube early in development and are fundamental for early shaping of neuronal connections by synaptic stripping (80). Finally, oligodendrocytes support and protect axons and provide for gray and white matter myelination, which supports brain connectome (81). The fundamental role of neuroglia in neuropathology has been considered by many prominent neuroanatomists (including Santiago Ramon y Cajal, Alois Alzheimer, Nicolas Achucarro, and Franz Nissl, to name a few) a century ago. The recent decade has witnessed the revival of interest to pathological potential of neuroglia, challenging universally accepted neurono-centric neuropathological doctrine (82–86).
Pathological Classifications of Neuroglia
Conceptually, neuroglial cells contribute to all neurological diseases either as primary elements driving pathology or by responding to lesions through an evolutionary conserved defensive program of reactive gliosis. Neuroglial changes in pathological conditions are context- and disease-specific, are complex, and evolve through the stages of neuropathology. Astrogliopathology in particular is subclassified (86) into i) reactive astrogliosis, which represents a graded response to various types of lesions.Reactive astrogliosis is fundamentally neuroprotective and produces a wide spectrum of reactive phenotypes that are disease- and disease stage-specific (84, 86–90); ii) pathological remodeling of astrocytes—when astrocytes acquire new properties driving neuropathology, Alexander disease (91) being a signal example; and iii) astroglial atrophy and loss of function. Similarly, microglial cells in pathology assume a multitude of phenotypes with various degrees of activation with both neuroprotective and neurotoxic functions. In chronic pathologies, microglial cells often undergo degeneration that limits their defensive capabilities (92, 93) or pathological remodeling (94). Pathological classification of oligodendrocytes is yet to be produced.
Neuroglial Abnormalities in Psychiatric Disorders
Neuroglial abnormalities are widely manifested in all major psychiatric diseases; and they are particularly prominent in bipolar disease and in major depression (95–98). In contrast to many other neuropathologies, there are no signs of astroglial reactivity in BD (as well as in other major psychiatric diseases); instead, astrocytes demonstrate prominent atrophy and asthenia, which most likely is associated with loss of homeostatic and supportive functions that in turn underlie failures in information processing and neurotransmission. Already in early stereological studies using Nissl staining (that revealed a total glial population), a prominent decrease in the overall number of neuroglial cells has been described in human postmortem samples from both major depressive disorder and BD (99). Subsequent morphometric studies have confirmed a significant reduction in glial numbers (up to 20–40%) in relevant brain regions (including the prefrontal cortex, orbitofrontal cortex, subgenual cortex, anterior cingulate cortex, and amygdala) in BD and major depression (95, 100–104). The expression of glial fibrillary acidic protein (GFAP), the marker of astroglial reactivity, which reveals the cytoskeleton of astrocytes, is generally suppressed in brain samples from young or adult subjects with depression and BD (105–107). In older subjects, GFAP expression was sometimes increased, which reflects general age-dependent changes or neuroinflammatory changes (105). Very significant (up to 95%) decrease in GFAP expression and GFAP-positive astroglial profiles have been recently detected in the white matter of the ventral prefrontal cortex of subjects with major depression (108). Impairment of astroglial networks and aberrant signaling in astroglial syncytia were evidenced by a significant decrease in the expression of major astroglial connexins XC30 and Cx 43 in the prefrontal cortex of depression-associated suicide victims (109). Major depression (but not BD) was found to be associated with a significant decrease in the density of astrocytes expressing glutamine syntethase and with downregulation of astroglial expression of glutamate transporter GLT-1, suggesting thus aberrant operation of glutamine–glutamate shuttle (110, 111). Likewise, the population of S100B-positive astrocytes was decreased in hippocampi of patients with BD and major depression (112).
Similar reduction in glial numbers and GFAP expression and astroglial morphological profiles have been detected in animal models of depressive behavior. These models are often based on exposure of animals to various types of chronic stress that instigate depressive-like behavior manifested by anhedonia or aberrant social communications. The density of GFAP-positive astrocytes and morphological astroglial profiles were reduced after the stress of separating juveniles from their family (113), chronic social defeat (114), or chronic mild stress (115), which induces prominent morphological atrophy of astroglial cells (116). Astroglial atrophy in chronic stress animal models may be associated with aberrant glycogen processing and decreased glycogen content (117). Significant astroglial atrophy was also observed in the repeated corticosterone injection-induced mouse depression model (118). Likewise, the density of astrocytes was significantly reduced in the prefrontal cortex, anterior cingulate cortex, amygdala, and hippocampus of Wistar–Kyoto strain of rats susceptible to depressive-like behavior (119). Chronic stress-induced astroglial asthenia and loss of function, as well as depressive behavior, were reversed by treating animals with riluzole, the drug that limits glutamate excitotoxicity (115). Selective ablation of astrocytes after injection of L-α aminoadipic acid into either rodent prefrontal cortex or prelimbic cortex triggered depressive-like behavior (120, 121); injection of neuronal toxin ibotenate had no such an effect (120). Emergence of depressive phenotype is associated with astroglia-specific decrease of expression of several genes associated with signaling systems, including serotonin 5-HT2B receptors, cytosolic phospholipase 2α, ionotropic kainate receptor GluK2, and adenosine deaminase acting on RNA 2 (ADAR2); treatment with fluoxetine restored altered expression (122, 123). The chronic stress-induced depressive phenotypes were also linked to a downregulation of astroglial expression of multiple endocrine neoplasia type 1 gene encoding protein menin; the efficiency in menin was associated with increased activation of NF-κB activation and elevated production of IL-1β (124). Depression after traumatic brain injury was associated with a decrease in astroglial expression of glutamate transporters (125), this being another example of astroglial asthenia with loss of function. All in all, these data underlie the hypothesis of the role of astroglial asthenia in the pathophysiology of mood disorders, including BD (97, 126, 127).
Astrocytes are recognized as therapeutic targets for the treatment of psychiatric disorders and, in particular, depression and BD (128–130). Treatment of animals subjected to psychosocial stress prevented the loss of astrocytes (114), whereas riluzole (the drug that limits glutamate excitotoxicity) similarly prevented loss of astrocytes in animals subjected to mild chronic stress (115). Even electroconvulsive therapy (ECT) has been shown to increase the expression of GFAP in the piriform cortex, amygdala, and hippocampus (131). Recent findings identified astrocytes as primary targets for transcranial direct current stimulation used for the management of depression (132). Moreover, it has been documented that two classical mood stabilizers used as first-line therapy for BD, lithium (Li+) and valproic acid (VPA), have a neuroprotective role reducing neuroinflammation through modulating the activation of astrocytes (133). Chronic treatments of astrocytes in vitro with Li+, VPA, and another classic antidepressant, carbamazepine (CBZ), suppress glutamate release, thus contributing to alleviation of excitotoxicity (134). Long-lasting exposure of astrocytes to antidepressant fluoxetine, a selective serotonin reuptake inhibitor, increased cytosolic pH from 7.18 to 7.58 by stimulating sodium-proton transporter 1, thus affecting brain pH homeostasis (135). Fluoxetine, as well as Li+, VPA, and CBZ, also affects astroglial glycogen content in a concentration-dependent manner, increasing glycogen at low concentrations and decreasing at high concentrations—this action being mediated by caveoline-1 (Cav-1) - phosphatase and tensin homologue (PTEN) - phosphoinositide 3-kinase (PI3K) - glycogen synthase kinase 3 (GSK-3β) cascade (Figure 3) (136, 137). These multiple actions of fluoxetine on astrocytes are mediated through direct activation of serotonin 5-HT2B receptors and transactivation of epidermal growth factor receptor (EGFR) (138, 139). Chronic treatment with antidepressants, as well as stimulation of adrenoceptors, was also reported to stimulate astroglial secretion of brain-derived neurotrophic factor (BDNF), which may boost synaptic transmission and provide neuroprotection (140, 141).
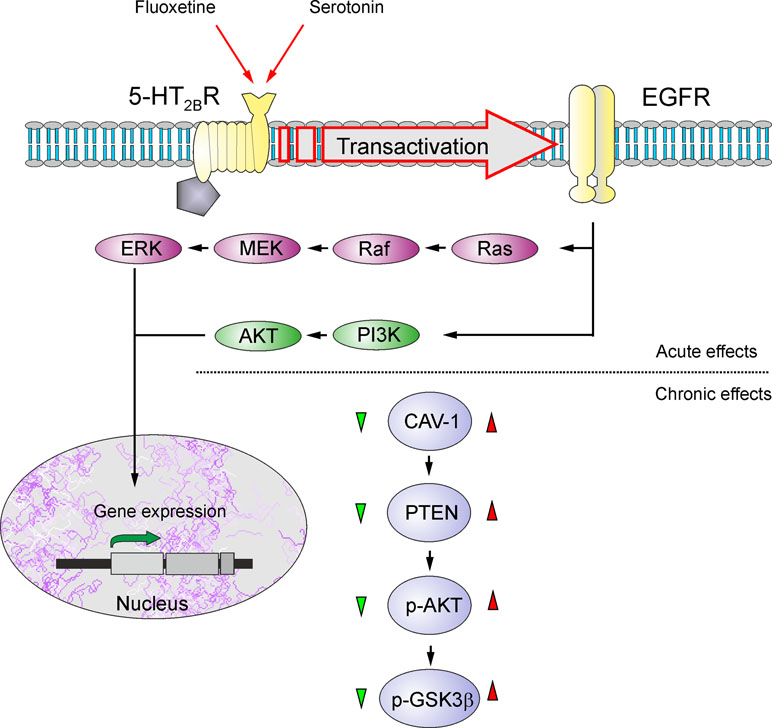
Figure 3 Schematic illustration of biphasic concentration-dependent regulation of Cav-1 gene expression and GSK-3β activity by fluoxetine in astrocytes. Acute treatment with fluoxetine stimulates 5-HT2B receptors, induces transactivation of EGF receptors and activates its MAPK/ERK and PI3K/AKT signal pathways that, in turn, regulate gene expression in astrocytes. Chronic treatment with fluoxetine at low concentrations (green triangle) decreases Cav-1 gene expression, which decreases membrane content of PTEN, induces dephosphorylation and inhibition of PI3K and elevates GSK-3β phosphorylation thus suppressing its activity. At higher concentrations (red triangle) fluoxetine increases Cav-1 gene expression that acts on PTEN/PI3K/AKT/GSK-3β in an inverse fashion. Reproduced from Ref. (139).
Analysis of lipopolysaccharide (LPS)-induced inflammation in rat primary mixed (80% astrocytes and 15% microglia) glial cultures found that Li+ decreases the secretion of TNF-α, IL1-β, prostaglandin E2, and nitric oxide (142). Pretreatment of LPS-stimulated microglial cells with Li+ significantly inhibited LPS-induced microglial activation and proinflammatory cytokine production (143). Similarly, VPA modulates microglial response to inflammatory insults mediated by LPS and may affect the synaptic excitatory inhibitory balance through its effect on astrocytes in rats (144, 145).
Astrocytes and Sleep Regulation
The role of astroglia in the regulation of sleep has been suggested more than a century ago by Santiago Ramon y Cajal, who suggested that astroglial processes, by entering the synaptic cleft, may slow down communication in neuronal networks, thus instigating sleep (146); a very similar mechanism was also considered by Carl-Ludwig Schleich (147) as a basis for general anesthesia.
Astrocytes of the suprachiasmatic nucleus do contain clock genes and do produce circadian rhythms of GFAP expression; astrocytes, in addition, may contribute to timekeeping through regulating glutamate levels (148). Nonetheless, it seems that the major role of astrocytes is the regulation of sleep homeostasis. The latter refers to a regulation mechanism that increases urge to sleep proportionally to the time spent awake (149). Sleep homeostasis is regulated by accumulation of adenosine in the brain during wakefulness (150), and the data accumulated demonstrated that the main source for adenosine in the physiological conditions is associated with astrocytes (151). Another important role of astroglia in sleep is associated with cleansing the brain parenchyma (152). It is, therefore, plausible to speculate that astroglial asthenia observed in mood disorders and in BD impairs astroglial sleep-regulating capabilities.
Sleep, Astroglia, and Bipolar Disorder
As has been mentioned above, sleep plays a key role in the clinical manifestations of BD. Alterations in the structure or duration of sleep are reported in all phases of the disorder—in the manic, depressive, and euthymic phases (65). During manic or hypomanic episodes, there is a reduced need for sleep, whereas during depressive episodes, there may be difficulty in achieving adequate quality or amount of sleep or, alternatively, patients experience hypersomnia (12, 153). Sleep abnormalities are strongly associated with immune dysfunction. Aberrant sleep is associated with increased levels of proinflammatory cytokines with a bidirectional causal association identified (154, 155). As such, interest has grown in immune dysfunction as a potential link that underwent two-way interaction between sleep dysfunction and BD (156, 157). Both postmortem and in vivo studies showed that microglial activation is involved in the neurobiology of BD (158, 159). These findings agree with the presence of peripheral inflammatory markers and the blood–brain barrier disruption revealed by meta-analyses. If as it seems it is true that modifications of inflammatory markers and microglial function may play an important role in progression of BD, several drugs used in the treatment of this disorder could have effects on glial cells, and future studies may use these cells as targets for the development of new treatments in this way (160, 161).
Conclusion
Sleep disturbances are common in patients with BD; these sleep alterations are present even during euthymia, as insomnia, increased sleep latency, and variability in sleep hours. Recent research has sought to identify the biological markers that underlie sleep disorders in patients with BD. The focus of the latest studies has highlighted the role for neuroglial cells. Astrocytes, the primary homeostatic cells of the CNS, undergo atrophy, asthenia, and loss in BD-specific brain regions, and deficiency in glial support and neuroprotection may have a key role to the pathophysiology of BD (84, 160), even though the precise mechanisms need to be further explored and clarified. Several drugs used for the treatment of BD have specific effects on glial cells indicating neuroglia as a target for the development of new treatments. Further research should concentrate on investigations of glial cells in vivo and in “humanized” preparations to increase our understanding of the role of glia in sleep regulation in people with BD. Additional systematic studies are also needed to highlight the importance of sleep disorders in patients with BD to offer a tailor-made treatment for these patients.
Author Contributions
LS, AV conceived the manuscript. LS, AV, RF, EC wrote the manuscript. LS, AV, CS-G, PDF edited the text and supervised the paper. The manuscript was critically revised and finally approved by AV, LS, CS-G and PDF. LS and AV coordinated the work.
Conflict of Interest Statement
The authors declare that the research was conducted in the absence of any commercial or financial relationships that could be construed as a potential conflict of interest.
Acknowledgments
The authors thank the Department of Health Sciences, University Magna Graecia, Catanzaro, Italy, for support for their work.
References
1. Grande I, Berk M, Birmaher B, Vieta E. Bipolar disorder. Lancet (2016) 387:1561–72. doi: 10.1016/S0140-6736(15)00241-X
2. Kraepelin E. Manic-depressive insanity and paranoia. Edinbourg: livingstone (1921). doi: 10.1097/00005053-192104000-00057
3. Baldessarini RJ, Perez J, Trede K, Maggini C. History of bipolar manic-depressive disorder. In: Yildiz A, Ruiz P, Nemeroff CB, editors. The Bipolar Book: History, Neurobiology, and Treatment. New York: Oxford University Press (2015). doi: 10.1093/med/9780199300532.003.0001
4. Association AP. Diagnostic and statistical manual of mental disorders. 5th edn (DSM-5). Washington: American Psychiatric Publishing (2013).
5. Salagre E, Dodd S, Aedo A, Rosa A, Amoretti S, Pinzon J, et al. Toward precision psychiatry in bipolar disorder: staging 2.0 . Front Psychiatry (2018) 9:641. doi: 10.3389/fpsyt.2018.00641
6. Andreazza AC, Young LT. The neurobiology of bipolar disorder: identifying targets for specific agents and synergies for combination treatment. Int J Neuropsychopharmacol (2014) 17:1039–52. doi: 10.1017/S1461145713000096
7. Harvey AG, Talbot LS, Gershon A. Sleep disturbance in bipolar disorder across the lifespan. Clin Psychol (New York) (2009) 16:256–77. doi: 10.1111/j.1468-2850.2009.01164.x
8. Gershon A, Do D, Satyanarayana S, Shah S, Yuen LD, Hooshmand F, et al. Abnormal sleep duration associated with hastened depressive recurrence in bipolar disorder. J Affect Disord (2017) 218:374–9. doi: 10.1016/j.jad.2017.05.015
9. Szentkiralyi A, Madarasz CZ, Novak M. Sleep disorders: impact on daytime functioning and quality of life. Expert Rev Pharmacoecon Outcomes Res (2009) 9:49–64. doi: 10.1586/14737167.9.1.49
10. Hombali A, Seow E, Yuan Q, Chang SHS, Satghare P, Kumar S, et al. Prevalence and correlates of sleep disorder symptoms in psychiatric disorders. Psychiatry Res (2018) S0165-1781(18)30268-3. doi: 10.1016/j.psychres.2018.07.009
11. Stubbs B, Vancampfort D, Veronese N, Solmi M, Gaughran F, Manu P, et al. The prevalence and predictors of obstructive sleep apnea in major depressive disorder, bipolar disorder and schizophrenia: a systematic review and meta-analysis. J Affect Disord (2016) 197:259–67. doi: 10.1016/j.jad.2016.02.060
12. Harvey AG. Sleep and circadian rhythms in bipolar disorder: seeking synchrony, harmony, and regulation. Am J Psychiatry (2008) 165:820–9. doi: 10.1176/appi.ajp.2008.08010098
13. Murray G, Harvey A. Circadian rhythms and sleep in bipolar disorder. Bipolar Disord (2010) 12:459–72. doi: 10.1111/j.1399-5618.2010.00843.x
14. Sjostrom N, Waern M, Hetta J. Nightmares and sleep disturbances in relation to suicidality in suicide attempters. Sleep (2007) 30:91–5. doi: 10.1093/sleep/30.1.91
15. Wehr TA. Sleep-loss as a possible mediator of diverse causes of mania. Br J Psychiatry (1991) 159:576–8. doi: 10.1192/bjp.159.4.576
16. Ng TH, Chung KF, Ho FY, Yeung WF, Yung KP, Lam TH. Sleep-wake disturbance in interepisode bipolar disorder and high-risk individuals: a systematic review and meta-analysis. Sleep Med Rev (2015) 20:46–58. doi: 10.1016/j.smrv.2014.06.006
17. Kanady JC, Soehnera AM, Harvey AG. A Retrospective Examination of Sleep Disturbance across the Course of Bipolar Disorder. J Sleep Disord Ther (2015) 4(2):1000193. doi: 10.4172/2167-0277.1000193
18. Bradley AJ, Webb-Mitchell R, Hazu A, Slater N, Middleton B, Gallagher P, et al. Sleep and circadian rhythm disturbance in bipolar disorder. Psychol Med (2017) 47:1678–89. doi: 10.1017/S0033291717000186
19. Rocha PM, Neves FS, Correa H. Significant sleep disturbances in euthymic bipolar patients. Compr Psychiatry (2013) 54:1003–8. doi: 10.1016/j.comppsych.2013.04.006
20. Steinan MK, Morken G, Lagerberg TV, Melle I, Andreassen OA, Vaaler AE, et al. Delayed sleep phase: an important circadian subtype of sleep disturbance in bipolar disorders. J Affect Disord (2016a) 191:156–63. doi: 10.1016/j.jad.2015.11.025
21. Cassidy F, Murry E, Forest K, Carroll BJ. Signs and symptoms of mania in pure and mixed episodes. J Affect Disord (1998) 50:187–201. doi: 10.1016/S0165-0327(98)00016-0
22. Loudon JB, Blackburn IM, Ashworth CM. A study of the symptomatology and course of manic illness using a new scale. Psychol Med (1977) 7:723–9. doi: 10.1017/S0033291700006395
23. Serretti A, Olgiati P. Profiles of “manic” symptoms in bipolar I, bipolar II and major depressive disorders. J Affect Disord (2005) 84:159–66. doi: 10.1016/j.jad.2003.09.011
24. Colombo C, Benedetti F, Barbini B, Campori E, Smeraldi E. Rate of switch from depression into mania after therapeutic sleep deprivation in bipolar depression. Psychiatry Res (1999) 86:267–70. doi: 10.1016/S0165-1781(99)00036-0
25. Casper RC, Redmond DE Jr., Katz MM, Schaffer CB, Davis JM, Koslow SH. Somatic symptoms in primary affective disorder. Presence and relationship to the classification of depression. Arch Gen Psychiatry (1985) 42:1098–104. doi: 10.1001/archpsyc.1985.01790340082012
26. Detre T, Himmelhoch J, Swartzburg M, Anderson CM, Byck R, Kupfer DJ. Hypersomnia and manic-depressive disease. Am J Psychiatry (1972) 128:1303–5. doi: 10.1176/ajp.128.10.1303
27. Steinan MK, Scott J, Lagerberg TV, Melle I, Andreassen OA, Vaaler AE, et al. Sleep problems in bipolar disorders: more than just insomnia. Acta Psychiatr Scand (2016b) 133:368–77. doi: 10.1111/acps.12523
28. Takaesu Y, Inoue Y, Murakoshi A, Komada Y, Otsuka A, Futenma K, et al. Prevalence of circadian rhythm sleep-wake disorders and associated factors in euthymic patients with bipolar disorder. PLoS One (2016) 11:e0159578. doi: 10.1371/journal.pone.0159578
29. Geoffroy PA, Scott J, Boudebesse C, Lajnef M, Henry C, Leboyer M, et al. Sleep in patients with remitted bipolar disorders: a meta-analysis of actigraphy studies. Acta Psychiatr Scand (2015) 131:89–99. doi: 10.1111/acps.12367
30. Jones SH, Tai S, Evershed K, Knowles R, Bentall R. Early detection of bipolar disorder: a pilot familial high-risk study of parents with bipolar disorder and their adolescent children. Bipolar Disord (2006) 8:362–72. doi: 10.1111/j.1399-5618.2006.00329.x
31. Faedda GL, Teicher MH. Objective measures of activity and attention in the differential diagnosis of psychiatric disorders of childhood. Essent Psychopharmacol (2005) 6:239–49.
32. Duffy A, Alda M, Crawford L, Milin R, Grof P. The early manifestations of bipolar disorder: a longitudinal prospective study of the offspring of bipolar parents. Bipolar Disord (2007) 9:828–38. doi: 10.1111/j.1399-5618.2007.00421.x
33. Dibner C, Schibler U, Albrecht U. The mammalian circadian timing system: organization and coordination of central and peripheral clocks. Annu Rev Physiol (2010) 72:517–49. doi: 10.1146/annurev-physiol-021909-135821
34. Foster RG, Hankins MW, Peirson SN. Light, photoreceptors, and circadian clocks. Methods Mol Biol (2007) 362:3–28. doi: 10.1007/978-1-59745-257-1_1
35. Lockley SW, Evans EE, Scheer FA, Brainard GC, Czeisler CA, Aeschbach D. Short-wavelength sensitivity for the direct effects of light on alertness, vigilance, and the waking electroencephalogram in humans. Sleep (2006) 29:161–8.
36. Wulff K, Gatti S, Wettstein JG, Foster RG. Sleep and circadian rhythm disruption in psychiatric and neurodegenerative disease. Nat Rev Neurosci (2010) 11:589–99. doi: 10.1038/nrn2868
37. Schibler U. The 2008 Pittendrigh/Aschoff lecture: peripheral phase coordination in the mammalian circadian timing system. J Biol Rhythms (2009) 24:3–15. doi: 10.1177/0748730408329383
38. Lamont EW, Coutu DL, Cermakian N, Boivin DB. Circadian rhythms and clock genes in psychotic disorders. Isr J Psychiatry Relat Sci (2010) 47:27–35.
39. Malek ZS, Sage D, Pevet P, Raison S. Daily rhythm of tryptophan hydroxylase-2 messenger ribonucleic acid within raphe neurons is induced by corticoid daily surge and modulated by enhanced locomotor activity. Endocrinology (2007) 148:5165–72. doi: 10.1210/en.2007-0526
40. Khurshid KA. Comorbid insomnia and psychiatric disorders: an update. Innov Clin Neurosci (2018) 15:28–32.
41. Boivin DB. Influence of sleep-wake and circadian rhythm disturbances in psychiatric disorders. J Psychiatry Neurosci (2000) 25:446–58.
42. Lamont EW, James FO, Boivin DB, Cermakian N. From circadian clock gene expression to pathologies. Sleep Med (2007) 8:547–56. doi: 10.1016/j.sleep.2006.11.002
43. Hasler BP, Allen JJ, Sbarra DA, Bootzin RR, Bernert RA. Morningness-eveningness and depression: preliminary evidence for the role of the behavioral activation system and positive affect. Psychiatry Res (2010) 176:166–73. doi: 10.1016/j.psychres.2009.06.006
44. Melo MCA, Abreu RLC, Linhares Neto VB, de Bruin PFC, de Bruin VMS. Chronotype and circadian rhythm in bipolar disorder: a systematic review. Sleep Med Rev (2017) 34:46–58. doi: 10.1016/j.smrv.2016.06.007
45. Franzen PL, Buysse DJ. Sleep disturbances and depression: risk relationships for subsequent depression and therapeutic implications. Dialogues Clin Neurosci (2008) 10:473–81.
46. Leibenluft E, Albert PS, Rosenthal NE, Wehr TA. Relationship between sleep and mood in patients with rapid-cycling bipolar disorder. Psychiatry Res (1996) 63:161–8. doi: 10.1016/0165-1781(96)02854-5
47. Ritter PS, Hofler M, Wittchen HU, Lieb R, Bauer M, Pfennig A, et al. Disturbed sleep as risk factor for the subsequent onset of bipolar disorder–data from a 10-year prospective-longitudinal study among adolescents and young adults. J Psychiatr Res (2015) 68:76–82. doi: 10.1016/j.jpsychires.2015.06.005
48. Wehr TA. Sleep loss: a preventable cause of mania and other excited states. J Clin Psychiatry (1989) 50:8–16; discussion 45-7.
49. Hirata FC, Lima MC, de Bruin VM, Nobrega PR, Wenceslau GP, de Bruin PF. Depression in medical school: the influence of morningness-eveningness. Chronobiol Int (2007) 24:939–46. doi: 10.1080/07420520701657730
50. Sylvia LG, Dupuy JM, Ostacher MJ, Cowperthwait CM, Hay AC, Sachs GS, et al. Sleep disturbance in euthymic bipolar patients. J Psychopharmacol (2012) 26:1108–12. doi: 10.1177/0269881111421973
51. Arent CO, Valvassori SS, Steckert AV, Resende WR, Dal-Pont GC, Lopes-Borges J, et al. The effects of n-acetylcysteine and/or deferoxamine on manic-like behavior and brain oxidative damage in mice submitted to the paradoxal sleep deprivation model of mania. J Psychiatr Res (2015) 65:71–9. doi: 10.1016/j.jpsychires.2015.04.011
52. Logan RW, McClung CA. Animal models of bipolar mania: the past, present and future. Neuroscience (2016) 321:163–88. doi: 10.1016/j.neuroscience.2015.08.041
53. Stange JP, Kleiman EM, Sylvia LG, Magalhaes PV, Berk M, Nierenberg AA, et al. Specific mood symptoms confer risk for subsequent suicidal ideation in bipolar disorder with and without suicide attempt history: Multi-Wave Data from Step-Bd. Depress Anxiety (2016) 33:464–72. doi: 10.1002/da.22464
54. Wirz-Justice A. Biological rhythm disturbances in mood disorders. Int Clin Psychopharmacol (2006) 21(Suppl 1):S11–5. doi: 10.1097/01.yic.0000195660.37267.cf
55. Ko CH, Takahashi JS. Molecular components of the mammalian circadian clock. Hum Mol Genet (2006) 15(Spec No 2):R271–7. doi: 10.1093/hmg/ddl207
56. Gonzalez R, Gonzalez S, Villa E, Ramirez M, Zavala J, Armas R, et al. Identification of circadian gene variants in bipolar disorder in Latino populations. J Affect Disord (2015) 186:367–75. doi: 10.1016/j.jad.2015.07.014
57. Wulff K, Joyce E, Middleton B, Dijk DJ, Foster RG. The suitability of actigraphy, diary data, and urinary melatonin profiles for quantitative assessment of sleep disturbances in schizophrenia: a case report. Chronobiol Int (2006) 23:485–95. doi: 10.1080/07420520500545987
58. Dijk DJ, Archer SN. PERIOD3, circadian phenotypes, and sleep homeostasis. Sleep Med Rev (2010) 14:151–60. doi: 10.1016/j.smrv.2009.07.002
59. Song HM, Cho CH, Lee HJ, Moon JH, Kang SG, Yoon HK, et al. Association of CLOCK, ARNTL, PER2, and GNB3 polymorphisms with diurnal preference in a Korean population. Chronobiol Int (2016) 33:1455-1463. doi: 10.1080/07420528.2016.1231199
60. Nievergelt CM, Kripke DF, Barett TB, Burg E, Remick RA, Sadonick AD, et al. Suggestive evidence for association of the circadian genes PERIOD3 and ARNTL with bipolar disorder. Am J Med Genet B Neuropsychiatr Genet (2006) 141B:234–41. doi: 10.1002/ajmg.b.30252
61. Dmitrak-Weglarz MP, Pawlak JM, Maciukiewicz M, Moczko J, Wilkosc M, Leszczynska Rodziewicz A, et al. Clock gene variants differentiate mood disorders. Mol Biol Rep (2015) 42:277–88. doi: 10.1007/s11033-014-3770-9
62. Soria V, Martínez-Amorós È, Escaramís G, Valero J, Pérez-Egea R, García C. Differential association of circadian genes with mood disorders: cRY1 and NPAS2 are associated with unipolar major depression and CLOCK and VIP with bipolar disorder. Neuropsychopharmacology (2010) 35:1279–89. doi: 10.1038/npp.2009.230
63. Maciukiewicz M, Dmitrzak-Weglarz M, Pawlak J, Leszczynska-Rodziewics A, Zaremba D, Skibinska M, et al. Analysis of genetic association and epistasis interactions between circadian clock genes and symptom dimensions of bipolar affective disorder. Chronobiol Int (2014) 31:770–8. doi: 10.3109/07420528.2014.899244
64. Benedetti F, Riccaboni R, Dallaspezia S, Locatelli C, Smeraldi E, Colombo C. Effects of CLOCK gene variants and early stress on hopelessness and suicide in bipolar depression. Chronobiol Int (2015) 32:1156–116. doi: 10.3109/07420528.2015.1060603
65. Bollettini I, Melloni EM, Aggio V, Poletti S, Lorenzi C, Pirovano A, et al. Clock genes associate with white matter integrity in depressed bipolar patients. Chronobiol Int (2017) 34:212–24. doi: 10.1080/07420528.2016.1260026
66. Geoffroy PA, Micoulaud Franchi JA, Lopez R, Poirot I, Brion A, Royant-Parola S, et al. How to characterize and treat sleep complaints in bipolar disorders? Encephale (2016) 43:363–73. doi: 10.1016/j.encep.2016.06.007
67. Rajendran B, Janakarajan VN. Circadian clock gene aryl hydrocarbon receptor nuclear translocator-like polymorphisms are associated with seasonal affective disorder: an Indian family study. Indian J Psychiatry (2016) 58:57–60. doi: 10.4103/0019-5545.174374
68. Pawlak J, Dmitrzak-Weglarz M, Maciukiewicz M, Wilkosc M, Leszczynska-Rodziewics A, Zaremba D, et al. Suicidal behavior in the context of disrupted rhythmicity in bipolar disorder–data from an association study of suicide attempts with clock genes. Psychiatry Res (2015) 226:517–20. doi: 10.1016/j.psychres.2015.01.010
69. Kuratomi G, Iwamoto K, Bundo M, Kusumi I, Kato N, Iwata N, et al. Aberrant DNA methylation associated with bipolar disorder identified from discordant monozygotic twins. Mol Psychiatry (2008) 13:429–41. doi: 10.1038/sj.mp.4002001
70. McCarthy MJ, Nievergelt CM, Shekhtman T, Kripke DF, Welsh DK, Kelsoe JR. Functional genetic variation in the Rev-Erbα pathway and lithium response in the treatment of bipolar disorder. Genes Brain Behav (2011) 10:852–61. doi: 10.1111/j.1601-183X.2011.00725.x
71. Oliveira T, Marinho V, Carvalho V, Magalhães F, Rocha K, Ayres C, et al. Genetic polymorphisms associated with circadian rhythm dysregulation provide new perspectives on bipolar disorder. Bipolar Disord (2018) 20(6):515–22. doi: 10.1111/bdi.12624
72. Beck-Friis J, Ljunggren JG, Thoren M, von Rosen D, Kjellman BF, Wetterberg L. Melatonin, cortisol and ACTH in patients with major depressive disorder and healthy humans with special reference to the outcome of the dexamethasone suppression test. Psychoneuroendocrinology (1985) 10:173–86. doi: 10.1016/0306-4530(85)90055-1
73. Linkowski P, Kerkhofs M, Van Onderbergen A, Hubain P, Copinschi G, L’Hermite-Baleriaux M, et al. The 24-hour profiles of cortisol, prolactin, and growth hormone secretion in mania. Arch Gen Psychiatry (1994) 51:616–24. doi: 10.1001/archpsyc.1994.03950080028004
74. Cervantes P, Gelber S, Kin FN, Nair VN, Schwartz G. Circadian secretion of cortisol in bipolar disorder. J Psychiatry Neurosci (2001) 26:411–6.
75. Shen GH, Alloy LB, Abramson LY, Sylvia LG. Social rhythm regularity and the onset of affective episodes in bipolar spectrum individuals. Bipolar Disord (2008) 10:520–9. doi: 10.1111/j.1399-5618.2008.00583.x
76. Verkhratsky A, Butt AM. Glial Physiology and Pathophysiology. Chichester: Wiley-Blackwell (2013). 560. doi: 10.1002/9781118402061
77. Verkhratsky A, Nedergaard M. The homeostatic astroglia emerges from evolutionary specialization of neural cells. Philos Trans R Soc Lond B Biol Sci (2016) 371(1700):20150428. doi: 10.1098/rstb.2015.0428
78. Verkhratsky A, Nedergaard M. Physiology of Astroglia. Physiol Rev (2018) 98:239–389. doi: 10.1152/physrev.00042.2016
79. Verkhratsky A, Nedergaard M. Astroglial cradle in the life of the synapse. Philos Trans R Soc Lond B Biol Sci (2014) 369:20130595. doi: 10.1098/rstb.2013.0595
80. Kettenmann H, Kirchhoff F, Verkhratsky A. Microglia: new roles for the synaptic stripper. Neuron (2013) 77:10–18. doi: 10.1016/j.neuron.2012.12.023
81. Michalski JP, Kothary R. Oligodendrocytes in a nutshell. Front Cell Neurosci (2015) 9:340. doi: 10.3389/fncel.2015.00340
82. Heneka MT, Carson MJ, El Khoury J, Landreth GE, Brosseron F, Feinstein DL, et al. Neuroinflammation in Alzheimer’s disease. Lancet Neurol (2015) 14:388–405. doi: 10.1016/S1474-4422(15)70016-5
83. Parpura V, Heneka MT, Montana V, Oliet SH, Schousboe A, Haydon PG, et al. Glial cells in (patho)physiology. J Neurochem (2012) 121:4–27. doi: 10.1111/j.1471-4159.2012.07664.x
84. Pekny M, Pekna M, Messing A, Steinhauser C, Lee JM, Parpura V, et al. Astrocytes: a central element in neurological diseases. Acta Neuropathol (2016) 131:323–45. doi: 10.1007/s00401-015-1513-1
85. Rodriguez JJ, Butt AM, Gardenal E, Parpura V, Verkhratsky A. Complex and differential glial responses in Alzheimer’s disease and ageing. Curr Alzheimer Res (2016) 13:343–58. doi: 10.2174/1567205013666160229112911
86. Verkhratsky A, Zorec R, Parpura V. Stratification of astrocytes in healthy and diseased brain. Brain Pathol (2017) 27:629–44. doi: 10.1111/bpa.12537
87. Anderson MA, Ao Y, Sofroniew MV. Heterogeneity of reactive astrocytes. Neurosci Lett (2014) 565:23–9. doi: 10.1016/j.neulet.2013.12.030
88. Anderson MA, Burda JE, Ren Y, Ao Y, O’Shea TM, Kawaguchi R, et al. Astrocyte scar formation aids central nervous system axon regeneration. Nature (2016) 532:195–200. doi: 10.1038/nature17623
89. Burda JE, Sofroniew MV. Reactive gliosis and the multicellular response to CNS damage and disease. Neuron (2014) 81:229–48. doi: 10.1016/j.neuron.2013.12.034
90. Sofroniew MV. Astrogliosis. Cold Spring Harb Perspect Biol (2014) 7:a020420. doi: 10.1101/cshperspect.a020420
91. Messing A, Brenner M, Feany MB, Nedergaard M, Goldman JE. Alexander disease. J Neurosci (2012) 32:5017–23. doi: 10.1523/JNEUROSCI.5384-11.2012
92. Streit WJ, Braak H, Xue QS, Bechmann I. Dystrophic (senescent) rather than activated microglial cells are associated with tau pathology and likely precede neurodegeneration in Alzheimer’s disease. Acta Neuropathol (2009) 118:475–85. doi: 10.1007/s00401-009-0556-6
93. Streit WJ, Xue QS, Tischer J, Bechmann I. Microglial pathology. Acta Neuropathol Commun (2014) 2:142. doi: 10.1186/PREACCEPT-1035265697142235
94. Bisht K, Sharma KP, Lecours C, Sanchez MG, El Hajj H, Milior G, et al. Dark microglia: a new phenotype predominantly associated with pathological states. Glia (2016) 64:826–39. doi: 10.1002/glia.22966
95. Rajkowska G, Stockmeier CA. Astrocyte pathology in major depressive disorder: insights from human postmortem brain tissue. Curr Drug Targets (2013) 14:1225–36. doi: 10.2174/13894501113149990156
96. Verkhratsky A, Parpura V. Astrogliopathology in neurological, neurodevelopmental and psychiatric disorders. Neurobiol Dis (2016) 85:254–61. doi: 10.1016/j.nbd.2015.03.025
97. Verkhratsky A, Rodriguez JJ, Steardo L. Astrogliopathology: a central element of neuropsychiatric diseases? Neuroscientist (2014) 20:576–88. doi: 10.1177/1073858413510208
98. Verkhratsky A, Steardo L, Parpura V, Montana V. Translational potential of astroc in brain disorders. Prog Neurobiol (2016) 144:188–205. doi: 10.1016/j.pneurobio.2015.09.003
99. Ongur D, Drevets WC, Price JL. Glial reduction in the subgenual prefrontal cortex in mood disorders. Proc Natl Acad Sci U S A (1998) 95:13290–5. doi: 10.1073/pnas.95.22.13290
100. Bowley MP, Drevets WC, Ongur D, Price JL. Low glial numbers in the amygdala in major depressive disorder. Biol Psychiatry (2002) 52:404–12. doi: 10.1016/S0006-3223(02)01404-X
101. Cotter D, Mackay D, Chana G, Beasley C, Landau S, Everall IP. Reduced neuronal size and glial cell density in area 9 of the dorsolateral prefrontal cortex in subjects with major depressive disorder. Cereb Cortex (2002) 12:386–94. doi: 10.1093/cercor/12.4.386
102. Cotter D, Mackay D, Landau S, Kerwin R, Everall I. Reduced glial cell density and neuronal size in the anterior cingulate cortex in major depressive disorder. Arch Gen Psychiatry (2001) 58:545–53. doi: 10.1001/archpsyc.58.6.545
103. Gittins RA, Harrison PJ. A morphometric study of glia and neurons in the anterior cingulate cortex in mood disorder. J Affect Disord (2011) 133:328–32. doi: 10.1016/j.jad.2011.03.042
104. Rajkowska G, Miguel-Hidalgo JJ, Wei J, Dilley G, Pittman SD, Meltzer HY, et al. Morphometric evidence for neuronal and glial prefrontal cell pathology in major depression. Biol Psychiatry (1999) 45:1085–98. doi: 10.1016/S0006-3223(99)00041-4
105. Miguel-Hidalgo JJ, Baucom C, Dilley G, Overholser JC, Meltzer HY, Stockmeier CA, et al. Glial fibrillary acidic protein immunoreactivity in the prefrontal cortex distinguishes younger from older adults in major depressive disorder. Biol Psychiatry (2000) 48:861–73. doi: 10.1016/S0006-3223(00)00999-9
106. Miguel-Hidalgo JJ, Waltzer R, Whittom AA, Austin MC, Rajkowska G, Stockmeier CA. Glial and glutamatergic markers in depression, alcoholism, and their comorbidity. J Affect Disord (2010) 127:230–40. doi: 10.1016/j.jad.2010.06.003
107. Muller MB, Lucassen PJ, Yassouridis A, Hoogendijk WJ, Holsboer F, Swaab DF. Neither major depression nor glucocorticoid treatment affects the cellular integrity of the human hippocampus. Eur J Neurosci (2001) 14:1603–12. doi: 10.1046/j.0953-816x.2001.01784.x
108. Rajkowska G, Legutko B, Moulana M, Syed M, Romero DG, Stockmeier CA, et al. Astrocyte pathology in the ventral prefrontal white matter in depression. J Psychiatr Res (2018) 102:150–8. doi: 10.1016/j.jpsychires.2018.04.005
109. Nagy C, Torres-Platas SG, Mechawar N, Turecki G. Repression of Astrocytic Connexins in cortical and subcortical brain regions and prefrontal enrichment of H3K9me3 in Depression and Suicide. Int J Neuropsychopharmacol (2017) 20:50–7. doi: 10.1093/ijnp/pyw071
110. Bernstein HG, Meyer-Lotz G, Dobrowolny H, Bannier J, Steiner J, Walter M, et al. Reduced density of glutamine synthetase immunoreactive astrocytes in different cortical areas in major depression but not in bipolar I disorder. Front Cell Neurosci (2015) 9:273. doi: 10.3389/fncel.2015.00273
111. Rappeneau V, Blaker A, Petro JR, Yamamoto BK, Shimamoto A. Disruption of the glutamate-glutamine cycle involving astrocytes in an animal model of Depression for Males and Females. Front Behav Neurosci (2016) 10:231. doi: 10.3389/fnbeh.2016.00231
112. Gos T, Schroeter ML, Lessel W, Bernstein HG, Dobrowolny H, Schiltz K, et al. S100B-immunopositive astrocytes and oligodendrocytes in the hippocampus are differentially afflicted in unipolar and bipolar depression: a postmortem study. J Psychiatr Res (2013) 47:1694–9. doi: 10.1016/j.jpsychires.2013.07.005
113. Braun K, Antemano R, Helmeke C, Buchner M, Poeggel G. Juvenile separation stress induces rapid region- and layer-specific changes in S100ss- and glial fibrillary acidic protein-immunoreactivity in astrocytes of the rodent medial prefrontal cortex. Neuroscience (2009) 160:629–38. doi: 10.1016/j.neuroscience.2009.02.074
114. Czeh B, Simon M, Schmelting B, Hiemke C, Fuchs E. Astroglial plasticity in the hippocampus is affected by chronic psychosocial stress and concomitant fluoxetine treatment. Neuropsychopharmacology (2006) 31:1616–26. doi: 10.1038/sj.npp.1300982
115. Banasr M, Chowdhury GM, Terwilliger R, Newton SS, Duman RS, Behar KL, et al. Glial pathology in an animal model of depression: reversal of stress-induced cellular, metabolic and behavioral deficits by the glutamate-modulating drug riluzole. Mol Psychiatry (2010) 15:501–11. doi: 10.1038/mp.2008.106
116. Tynan RJ, Beynon SB, Hinwood M, Johnson SJ, Nilsson M, Woods JJ, et al. Chronic stress-induced disruption of the astrocyte network is driven by structural atrophy and not loss of astrocytes. Acta Neuropathol (2013) 126:75–91. doi: 10.1007/s00401-013-1102-0
117. Zhao Y, Zhang Q, Shao X, Ouyang L, Wang X, Zhu K, et al. Decreased glycogen content might contribute to chronic stress-induced atrophy of hippocampal astrocyte volume and depression-like behavior in rats. Sci Rep (2017) 7:43192. doi: 10.1038/srep43192
118. Zhao Y, Lin Z, Chen L, Ouyang L, Gu L, Chen F, et al. Hippocampal astrocyte atrophy in a mouse depression model induced by corticosterone is reversed by fluoxetine instead of benzodiazepine diazepam. Prog Neuropsychopharmacol Biol Psychiatry (2018) 83:99–109. doi: 10.1016/j.pnpbp.2018.01.011
119. Gosselin RD, Gibney S, O’Malley D, Dinan TG, Cryan JF. Region specific decrease in glial fibrillary acidic protein immunoreactivity in the brain of a rat model of depression. Neuroscience (2009) 159:915–25. doi: 10.1016/j.neuroscience.2008.10.018
120. Banasr M, Duman RS. Glial loss in the prefrontal cortex is sufficient to induce depressive-like behaviors. Biol Psychiatry (2008) 64:863–70. doi: 10.1016/j.biopsych.2008.06.008
121. Lee Y, Son H, Kim G, Kim S, Lee DH, Roh GS, et al. Glutamine deficiency in the prefrontal cortex increases depressive-like behaviours in male mice. J Psychiatry Neurosci (2013) 38:183–91. doi: 10.1503/jpn.120024
122. Dong L, Li B, Verkhratsky A, Peng L. Cell type-specific in vivo expression of genes encoding signalling molecules in the brain in response to chronic mild stress and chronic treatment with fluoxetine. Psychopharmacology (Berl) (2015) 232:2827–35. doi: 10.1007/s00213-015-3921-2
123. Zhang X, Song D, Gu L, Ren Y, Verkhratsky A, Peng L. Decrease of gene expression of astrocytic 5-HT2B receptors parallels development of depressive phenotype in a mouse model of Parkinson’s disease. Front Cell Neurosci (2015) 9:388. doi: 10.3389/fncel.2015.00388
124. Leng L, Zhuang K, Liu Z, Huang C, Gao Y, Chen G, et al. Menin deficiency leads to depressive-like behaviors in mice by modulating astrocyte-mediated neuroinflammation. Neuron (2018) 100:551–63 e557. doi: 10.1016/j.neuron.2018.08.031
125. Piao CS, Holloway AL, Hong-Routson S, Wainwright MS. Depression following traumatic brain injury in mice is associated with down-regulation of hippocampal astrocyte glutamate transporters by thrombin. J Cereb Blood Flow Metab (2019) 39:58–73. doi: 10.1177/0271678X17742792
126. Czeh B, Nagy SA. Clinical findings documenting cellular and molecular abnormalities of glia in depressive disorders. Front Mol Neurosci (2018) 11:56. doi: 10.3389/fnmol.2018.00056
127. Rajkowska G, Miguel-Hidalgo JJ. Gliogenesis and glial pathology in depression. CNS Neurol Disord Drug Targets (2007) 6:219–33. doi: 10.2174/187152707780619326
128. Czeh B, Di Benedetto B. Antidepressants act directly on astrocytes: evidences and functional consequences. Eur Neuropsychopharmacol (2013) 23:171–85. doi: 10.1016/j.euroneuro.2012.04.017
129. Peng L, Li B, Verkhratsky A. Targeting astrocytes in bipolar disorder. Expert Rev Neurother (2016) 16:649–57. doi: 10.1586/14737175.2016.1171144
130. Peng L, Verkhratsky A, Gu L, Li B. Targeting astrocytes in major depression. Expert Rev Neurother (2015) 15:1299–06. doi: 10.1586/14737175.2015.1095094
131. Kragh J, Bolwig TG, Woldbye DP, Jorgensen OS. Electroconvulsive shock and lidocaine-induced seizures in the rat activate astrocytes as measured by glial fibrillary acidic protein. Biol Psychiatry (1993) 33:794–800. doi: 10.1016/0006-3223(93)90020-E
132. Monai H, Hirase H. Astrocytes as a target of transcranial direct current stimulation (tDCS) to treat depression. Neurosci Res (2018) 126:15–21. doi: 10.1016/j.neures.2017.08.012
133. Li N, Zhang X, Dong H, Zhang S, Sun J, Qian Y. Lithium ameliorates LPS-induced astrocytes activation partly via inhibition of toll-like receptor 4 expression. Cell Physiol Biochem (2016) 38:714–25. doi: 10.1159/000443028
134. Liu Z, Song D, Yan E, Verkhratsky A, Peng L. Chronic treatment with anti-bipolar drugs suppresses glutamate release from astroglial cultures. Amino Acids (2015) 47:1045–51. doi: 10.1007/s00726-015-1936-y
135. Ren J, Song D, Bai Q, Verkhratsky A, Peng L. Fluoxetine induces alkalinization of astroglial cytosol through stimulation of sodium-hydrogen exchanger 1: dissection of intracellular signaling pathways. Front Cell Neurosci (2015) 9:61. doi: 10.3389/fncel.2015.00061
136. Bai Q, Song D, Gu L, Verkhratsky A, Peng L. Bi-phasic regulation of glycogen content in astrocytes via Cav-1/PTEN/PI3K/AKT/GSK-3beta pathway by fluoxetine. Psychopharmacology (Berl) (2017) 234:1069–77. doi: 10.1007/s00213-017-4547-3
137. Jia S, Li B, Huang J, Verkhratsky A, Peng L. Regulation of glycogen content in astrocytes via Cav-1/PTEN/AKT/GSK-3beta pathway by three anti-bipolar Drugs. Neurochem Res (2018) 43:1692–701. doi: 10.1007/s11064-018-2585-9
138. Li B, Jia S, Yue T, Yang L, Huang C, Verkhratsky A, et al. Biphasic regulation of Caveolin-1 gene expression by fluoxetine in astrocytes: opposite effects of PI3K/AKT and MAPK/ERK Signaling Pathways on c-fos. Front Cell Neurosci (2017) 11:335. doi: 10.3389/fncel.2017.00335
139. Peng L, Song D, Li B, Verkhratsky A. Astroglial 5-HT2B receptor in mood disorders. Expert Rev Neurother (2018), 30:1–8. doi: 10.1080/14737175.2018.1458612
140. Koppel I, Jaanson K, Klasche A, Tuvikene J, Tiirik T, Parn A, et al. Dopamine cross-reacts with adrenoreceptors in cortical astrocytes to induce BDNF expression, CREB signaling and morphological transformation. Glia (2018) 66:206–16. doi: 10.1002/glia.23238
141. Marathe SV, D’Almeida P L, Virmani G, Bathini P, Alberi L. Effects of Monoamines and antidepressants on astrocyte physiology: implications for Monoamine hypothesis of depression. J Exp Neurosci (2018) 12:1179069518789149. doi: 10.1177/1179069518789149
142. Nahman S, Belmaker RH, Azab AN. Effects of lithium on lipopolysaccharide-induced inflammation in rat primary glia cells. Innate Immun (2012) 18:447–58. doi: 10.1177/1753425911421512
143. Dong H, Zhang X, Dai X, Lu S, Gui B, Jin W, et al. Lithium ameliorates lipopolysaccharide-induced microglial activation via inhibition of toll-like receptor 4 expression by activating the PI3K/Akt/FoxO1 pathway. J Neuroinflammation (2014) 11:140. doi: 10.1186/s12974-014-0140-4
144. Singh V, Bhatia HS, Kumar A, de Oliveira AC, Fiebich BL. Histone deacetylase inhibitors valproic acid and sodium butyrate enhance prostaglandins release in lipopolysaccharide-activated primary microglia. Neuroscience (2014) 265:147–57. doi: 10.1016/j.neuroscience.2014.01.037
145. Wang CC, Chen PS, Hsu CW, Wu SJ, Lin CT, Gean PW. Valproic acid mediates the synaptic excitatory/inhibitory balance through astrocytes–a preliminary study. Prog Neuropsychopharmacol Biol Psychiatry (2012) 37:111–20. doi: 10.1016/j.pnpbp.2012.01.017
146. Ramón y Cajal S. Algunas conjeturas sobre el mechanismoanatomico de la ideacion, asociacion y atencion. Madrid: Imprenta y Libreria de Nicolas Moya (1895).
147. Schleich CL. Schmerzlose Operationen: Örtliche Betäubung mit indiffrenten Flüssigkeiten. In: Psychophysik des natürlichen und künstlichen Schlafes. Berlin: Julius Springer (1894). p. 256.
148. Brancaccio M, Patton AP, Chesham JE, Maywood ES, Hastings MH. Astrocytes control circadian timekeeping in the suprachiasmatic nucleus via glutamatergic signaling. Neuron (2017) 93:1420–35 e1425. doi: 10.1016/j.neuron.2017.02.030
149. Halassa MM, Florian C, Fellin T, Munoz JR, Lee SY, Abel T, et al. Astrocytic modulation of sleep homeostasis and cognitive consequences of sleep loss. Neuron (2009) 61:213–9. doi: 10.1016/j.neuron.2008.11.024
150. Brown RE, Basheer R, McKenna JT, Strecker RE, McCarley RW. Control of sleep and wakefulness. Physiol Rev (2012) 92:1087–187. doi: 10.1152/physrev.00032.2011
151. Haydon PG. Astrocytes and the modulation of sleep. Curr Opin Neurobiol (2017) 44:28–33. doi: 10.1016/j.conb.2017.02.008
152. Iliff JJ, Wang M, Liao Y, Plogg BA, Peng W, Gundersen GA, et al. A paravascular pathway facilitates CSF flow through the brain parenchyma and the clearance of interstitial solutes, including amyloid beta. Sci Transl Med (2012) 4:147ra111. doi: 10.1126/scitranslmed.3003748
153. Rosenblat JD, McIntyre RS. Bipolar disorder and immune dysfunction: epidemiological findings, proposed pathophysiology and clinical implications. Brain Sci (2017) 7(11): E144. doi: 10.3390/brainsci7110144
154. Irwin MR, Olmstead R, Carroll JE. Sleep Disturbance, sleep duration, and inflammation: a systematic review and meta-analysis of cohort studies and experimental sleep deprivation. Biol Psychiatry (2016) 80:40–52. doi: 10.1016/j.biopsych.2015.05.014
155. McIntyre RS. Sleep and inflammation: implications for domain approach and treatment opportunities. Biol Psychiatry (2016) 80:9–11. doi: 10.1016/j.biopsych.2016.04.018
156. Dolsen MR, Soehner AM, Harvey AG. Proinflammatory cytokines, mood, and sleep in interepisode Bipolar Disorder and Insomnia: a pilot study with implications for psychosocial interventions. Psychosom Med (2018) 80:87–94. doi: 10.1097/PSY.0000000000000529
157. Mukherjee D, Krishnamurthy VB, Millett CE, Reider A, Can A, Groer M, et al. Total sleep time and kynurenine metabolism associated with mood symptom severity in bipolar disorder. Bipolar Disord (2018) 20:27–34. doi: 10.1111/bdi.12529
158. Haarman BC, Riemersma-Van der Lek RF, de Groot JC, Ruhe HG, Klein HC, Zandstra TE, et al. Neuroinflammation in bipolar disorder - a [11C]-(R)-PK11195 positron emission tomography study. Brain Behav Immun (2014) 40:219–25. doi: 10.1016/j.bbi.2014.03.016
159. Reus GZ, Fries GR, Stertz L, Badawy M, Passos IC, Barichello T, et al. The role of inflammation and microglial activation in the pathophysiology of psychiatric disorders. Neuroscience (2015) 300:141–54. doi: 10.1016/j.neuroscience.2015.05.018
160. Keshavarz M. Glial cells as key elements in the pathophysiology and treatment of bipolar disorder. Acta Neuropsychiatr (2017) 29:140–52. doi: 10.1017/neu.2016.56
Keywords: astroglia, microglia, oligodendroglia, bipolar disorder, depressive behavior
Citation: Steardo L Jr, de Filippis R, Carbone EA, Segura-Garcia C, Verkhratsky A and De Fazio P (2019) Sleep Disturbance in Bipolar Disorder: Neuroglia and Circadian Rhythms. Front. Psychiatry 10:501. doi: 10.3389/fpsyt.2019.00501
Received: 10 May 2019; Accepted: 25 June 2019;
Published: 18 July 2019.
Edited by:
Maurice M. Ohayon, Stanford University, United StatesReviewed by:
Timo Partonen, National Institute for Health and Welfare, FinlandHiroshi Kadotani, Shiga University of Medical Science, Japan
Copyright © 2019 Steardo, de Filippis, Carbone, Segura-Garcia, Verkhratsky and De Fazio. This is an open-access article distributed under the terms of the Creative Commons Attribution License (CC BY). The use, distribution or reproduction in other forums is permitted, provided the original author(s) and the copyright owner(s) are credited and that the original publication in this journal is cited, in accordance with accepted academic practice. No use, distribution or reproduction is permitted which does not comply with these terms.
*Correspondence: Luca Steardo Jr. c3RlYXJkb0B1bmljei5pdA==