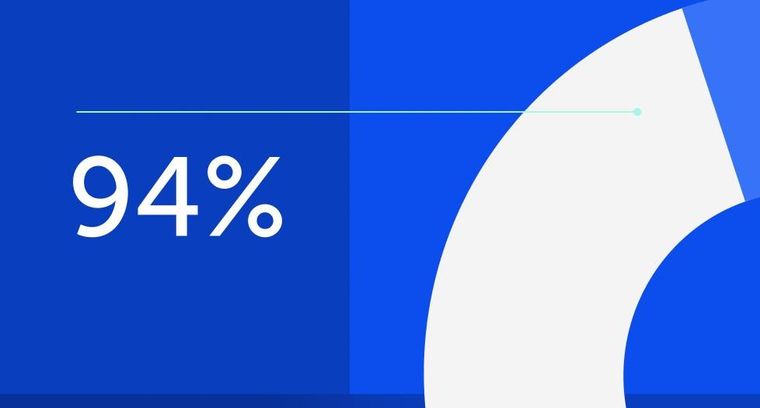
94% of researchers rate our articles as excellent or good
Learn more about the work of our research integrity team to safeguard the quality of each article we publish.
Find out more
MINI REVIEW article
Front. Psychiatry, 25 February 2016
Sec. Addictive Disorders
Volume 7 - 2016 | https://doi.org/10.3389/fpsyt.2016.00024
This article is part of the Research TopicMemory systems of the addicted brain: the underestimated role of drug-induced cognitive biases in addiction and its treatmentView all 14 articles
The view that anatomically distinct memory systems differentially contribute to the development of drug addiction and relapse has received extensive support. The present brief review revisits this hypothesis as it was originally proposed 20 years ago (1) and highlights several recent developments. Extensive research employing a variety of animal learning paradigms indicates that dissociable neural systems mediate distinct types of learning and memory. Each memory system potentially contributes unique components to the learned behavior supporting drug addiction and relapse. In particular, the shift from recreational drug use to compulsive drug abuse may reflect a neuroanatomical shift from cognitive control of behavior mediated by the hippocampus/dorsomedial striatum toward habitual control of behavior mediated by the dorsolateral striatum (DLS). In addition, stress/anxiety may constitute a cofactor that facilitates DLS-dependent memory, and this may serve as a neurobehavioral mechanism underlying the increased drug use and relapse in humans following stressful life events. Evidence supporting the multiple systems view of drug addiction comes predominantly from studies of learning and memory that have employed as reinforcers addictive substances often considered within the context of drug addiction research, including cocaine, alcohol, and amphetamines. In addition, recent evidence suggests that the memory systems approach may also be helpful for understanding topical sources of addiction that reflect emerging health concerns, including marijuana use, high-fat diet, and video game playing.
Investigators often look to mechanisms of learning and behavior to explain how human psychopathology is acquired and expressed. An example of such an application was provided by Norman M. White who employed tenets of classical learning theory and experimental evidence supporting the existence of multiple memory systems in the brain to provide a novel, influential approach to drug addiction (1). Specifically, White indicated that drugs can play the part of “reinforcers” that, like food or water in a learning task, strengthen associations among drug-related stimuli, context, and behavior to promote drug taking and, over time, addiction. White also incorporated the emerging hypothesis that there are different types of memory that are mediated by dissociable neural systems. According to this novel view, drugs can directly modulate multiple neural systems, and these neural systems go onto encode distinct components of the drug-related memory that, when expressed, promote further drug taking.
The year 2016 marks the 20th anniversary of the multiple memory systems view of drug addiction as described by White. The present review revisits this influential hypothesis, while highlighting some important recent developments that have not only substantiated the original hypothesis but have also produced additional insights into how multiple memory systems potentially support drug addiction.
Converging evidence from studies employing humans and lower animals indicates that mammalian memory is mediated by relatively independent neural systems [for reviews, see Ref. (2–4)]. The early experiments dissociating multiple memory systems were primarily conducted in the radial maze and indicated unique mnemonic functions for the hippocampus, dorsal striatum, and amygdala (5, 6). The hippocampus mediates a cognitive/spatial form of memory, whereas the dorsal striatum mediates stimulus–response (S–R) habit memory. The amygdala mediates Pavlovian and stimulus-affect-associative relationships (6, 7), while also subserving the modulatory role of emotional arousal on other types of memory (8–12).
Within the context of the multiple systems view of memory, White (1) suggested that the hippocampus, dorsal striatum, and amygdala encode unique components of drug-related memories (see Figure 1). The hippocampus encodes explicit knowledge pertaining to the relationship between cues and events (i.e., stimulus–stimulus associations) in the drug context. Importantly, the hippocampus does not encode behavioral responses, but rather the information acquired by the hippocampus can be used to generate the appropriate behavioral responses to receive drug reinforcement. On the other hand, the dorsal striatum encodes associations between drug-related stimuli and behavioral responses. This may allow the presentation of a drug-related cue to activate an automatic behavioral response that results in drug taking (e.g., running approach or instrumental lever press). The amygdala encodes Pavlovian-associative relationships, thus allowing neutral cues in the drug context to become associated with the drug reward. Animals later react to these conditioned cues similarly to how they originally reacted to the drug. Specifically, the conditioned cues activate conditioned emotional responses, including internal affective states and conditioned approach toward (or in some cases avoidance from) the conditioned cue. Another critical component of White’s hypothesis is that drugs can modulate memory function of each of these brain regions. Thus, drugs can potentially enhance their own self-administration via augmenting consolidation of the drug-related memories encoded by the hippocampus, amygdala, and dorsal striatum (see Figure 1).
Figure 1. White’s (1) multiple memory systems view of drug addiction. Like natural reinforcers, addictive drugs possess several “reinforcer actions,” including the ability to invoke positive/negative affect, approach, and modulation of memory systems. The amygdala, caudate–putamen (i.e., dorsal striatum), and hippocampus mediate dissociable memory systems, and each memory system presumably encodes unique components of drug-related memories. Given their memory modulatory properties, addictive drugs can potentially enhance their own self-administration by enhancing the function of these systems. (Reprinted from White with permission from John Wiley & Sons.)
Consistent with the multiple memory systems view of drug addiction, extensive evidence indicates critical roles for the hippocampus, dorsal striatum, and amygdala in drug addiction and relapse for a variety of abused substances [for review, see Ref. (13)]. The dorsal hippocampus appears to have a role in the contextual control of drug seeking for cocaine (14–16). The lateral region of the dorsal striatum (DLS) mediates S–R habitual lever pressing for cocaine and alcohol (17, 18), and the basolateral amygdala (BLA) mediates conditioned drug seeking for cocaine, alcohol, and heroin (19–22). Also consistent with White’s hypothesis, substances of abuse can modulate the mnemonic functions of the hippocampus, dorsal striatum, and amygdala (23–31).
Recent studies have contributed novel amendments to the multiple memory systems approach to drug addiction. Key features of this contemporary view include (1) a neuroanatomical shift over time to DLS-dependent habit memory, (2) competitive interactions between memory systems, (3) the role of stress and anxiety in enhancing habitual drug seeking, and (4) the application of this hypothesis to new emerging sources of addiction.
In experimental learning situations, subjects typically employ purposeful behavior when initially solving a task. However, following extensive training, behavior becomes autonomous and can be performed with little attention, intention, or cognitive effort, constituting a “habit” [for review, see Ref. (32)]. In early demonstrations of this shift from cognitive control of behavior to habit, rodents were trained using food reward in a dual-solution plus-maze task (33–35). In this task, rats were released from the same starting position (e.g., the south arm) and had to make a consistent body-turn at the maze intersection to receive food reward always located in the same goal arm (e.g., always make a left turn to find food in the west arm). Rats could solve this task by either learning a consistent body-turn response or by making whatever response necessary to go the same spatial location. To determine which strategy the rats employed, investigators implemented a probe test in which animals were released from the opposite start arm (e.g., the north arm). If animals made the opposite body-turn to go the original goal location, they were identified as place learners. If animals made the same body-turn as during training (i.e., going to the arm opposite to the original goal location), animals were identified as response learners. Evidence indicates that after some training, most animals display place learning, whereas after extensive training, animals shift to habitual response learning (34–36). Interestingly, this shift from place learning to response learning may reflect a neuroanatomical shift. The initial use of place learning in this task is mediated by the hippocampus and dorsomedial striatum [DMS (36, 37)], whereas the use of response learning after extended training is mediated by the DLS (36).
In addition to early demonstrations using the plus-maze (34, 35), the behavioral shift to habit memory was later demonstrated using operant lever pressing paradigms (38–42). In these instrumental learning tasks, animals initially lever press purposefully in order to obtain the outcome and will cease lever pressing once the food outcome is devalued. However, following extensive training animals will shift to habitual responding and will continue pressing the lever even after the food outcome has been devalued (40). As originally demonstrated in the plus-maze (36), the transition from cognition to habit in instrumental learning tasks might also be attributed to a neuroanatomical shift. The initial cognitive control of behavior in these instrumental learning tasks is mediated by the hippocampus and DMS (43, 44), whereas later habitual responding is mediated by the DLS (18, 45, 46).
Numerous investigators have suggested that the neuroanatomical shift to habit memory demonstrated in maze and instrumental learning tasks might also underlie the shift from recreational drug use to compulsive drug abuse (13, 47–50). Consistent with this hypothesis, investigators have demonstrated for a variety of abused substances that the DMS mediates goal-directed responding for drug reinforcement and the DLS mediates habitual responding for drug reinforcement (18, 31, 51–53).
Considering the high abuse potential of some drugs, investigators have suggested that addictive drugs might enhance DLS-dependent habit memory function and thereby accelerate the shift from cognitive to habitual control of behavior. Consistent with this hypothesis, repeated exposure to amphetamine or cocaine facilitates the shift from goal-directed to habitual responding for food reinforcement in instrumental lever pressing tasks (31, 54–59). In addition, lever pressing for addictive substances (e.g., alcohol or cocaine) versus food reward has been associated with greater habitual responding versus goal-directed responding (24, 60, 61). In humans, alcohol-dependent individuals show greater habitual responding in an instrumental learning task, relative to non-dependent control individuals (62). This enhancement of DLS-dependent habit memory by addictive drugs has also been observed in rodent maze learning tasks. Cocaine, amphetamine, and alcohol exposure have been associated with enhanced learning in DLS-dependent maze tasks or greater use of DLS-dependent response strategies in dual-solution versions of the maze (25, 63, 64). In humans, the use of abused substances, including alcohol and tobacco, has been correlated to the greater use of dorsal striatum-dependent navigational strategies in a virtual maze (65). Thus, some drugs of abuse might enhance DLS-dependent habit memory, and this heightened engagement of the DLS memory system might accelerate the transition from recreational drug use to habitual drug abuse. This proposed mechanism is consistent with White’s (1) original contention that drugs of abuse might sometimes facilitate their own self-administration by enhancing function of memory systems.
Although it is possible that addictive drugs enhance habit memory directly by enhancing function of the DLS [e.g., Ref. (29)], another possibility is that drugs of abuse enhance habit memory indirectly via modulation of other memory systems. This alternative mechanism invokes the hypothesis that in some learning situations, memory systems compete for control of learning and that by impairing the function of one memory system, function of another intact system might be enhanced (11, 66). Notably, the hippocampus and DLS might sometimes compete for control of learning, whereby lesion of the hippocampus enhances DLS-dependent memory function (5, 6, 67, 68). Competitive interactions can also be demonstrated in dual-solution tasks, when impairing one memory system results in the use of a strategy mediated by another intact system. For instance, animals given DMS lesions display DLS-dependent habitual responding for food reward in instrumental learning tasks (44).
Considering the competitive interactions that sometimes arise between memory systems, one possibility is that some drugs of abuse might enhance DLS-dependent habit memory indirectly by impairing cognitive memory mechanisms mediated by the DMS and hippocampus. As noted previously, alcohol is associated with greater use of DLS-dependent habit memory in maze and operant lever pressing paradigms (24, 61, 62, 64, 65). Evidence also indicates that alcohol impairs learning in hippocampus-dependent spatial memory tasks [(64, 69–72); for review, see Ref. (73)], as well as in DMS-dependent reversal learning tasks (74–77). Consistent with a competitive interaction between memory systems, it has been hypothesized that alcohol may facilitate DLS-dependent habit memory indirectly via impairing cognitive memory mechanisms (78).
It should be noted that aside from alcohol, numerous drugs have been associated with cognitive memory deficits. Exposure to morphine, heroin, methamphetamine, MDMA (ecstasy), or chronic cocaine similarly produces hippocampus-dependent spatial memory impairments across a variety of tasks (79–89). It is tempting to speculate that, as suggested for alcohol, cognitive memory impairments produced by addictive drugs might indirectly enhance DLS-dependent habit memory, and that this might be one mechanism allowing drug self-administration to become habitual in human drug abusers. On the other hand, it is also possible that spatial learning deficits produced by addictive drugs might occur indirectly via enhancement of DLS-dependent memory processes. Consistent with this hypothesis, stimulating CREB activity in the DLS impairs hippocampus-dependent spatial memory (90), whereas inhibition of CREB activity in the DLS reverses the spatial memory impairments produced by morphine (91).
An additional consideration regarding the multiple memory systems approach to drug addiction is the role of stress. Converging evidence indicates that robust emotional arousal facilitates DLS-dependent habit memory in rodents and humans [for reviews, see Ref. (9–12)]. Administration of anxiogenic drugs enhances DLS-dependent response learning in the water plus-maze (92–97). This enhancement of DLS-dependent habit memory is also observed following exposure to unconditioned behavioral stressors [e.g., chronic restraint, tail shock, predator odor, etc. (98–101)] and exposure to fear-conditioned stimuli [tone previously paired with shock (102, 103)]. Although originally demonstrated in rodents (92), this enhancement of habit memory induced by robust emotional arousal has also been demonstrated extensively in humans (99, 104–110).
The mechanisms allowing stress/anxiety to facilitate habit memory remain largely unknown; however, evidence indicates a critical modulatory role of the BLA (93–95, 100). Consistent with a competitive interaction between memory systems, some evidence also suggests that stress/anxiety might enhance DLS-dependent habit memory indirectly by impairing hippocampal function (94, 95).
Enhancement of habit memory following stress or anxiety may be relevant to understanding some prominent factors leading to drug abuse. Namely, stressful life events or chronic prolonged periods of stress/anxiety are associated with increased vulnerability to drug addiction and relapse in humans (111–117), and similar observations have been made in animal models of drug self-administration [for review, see Ref. (118)]. Investigators have suggested that consistent with the influence of emotional arousal on multiple memory systems (10), acute or chronic stress may enhance drug addiction and relapse in humans by engaging DLS-dependent habit memory processes (9, 49, 119). Consistent with this suggestion, stress in cocaine-dependent individuals is associated with decreased blood-oxygen-level-dependent (BOLD) activity in the hippocampus and increased activity in the dorsal striatum, and these BOLD activity changes are associated with stress-induced cocaine cravings (120).
Aside from drugs of abuse, the multiple memory systems hypothesis has also been recently employed for understanding other emerging sources of addiction. For instance, the rise in obesity over the past few decades has led to a comparable surge in experimental interest, with many investigators drawing parallels between drug addiction and overeating [for review, see Ref. (121–123)]. Some recent evidence has suggested that like drug addiction, food addiction might be partially attributed to heightened engagement of DLS-dependent habit memory. In rats, binge-like food consumption facilitates the shift from cognitive to habitual control of behavior (124, 125). Moreover, habitual behavior in bingeing animals is associated with increased DLS activity and may be prevented by blocking AMPA or dopamine D1 receptors in the DLS (125). Diet-induced obesity has also been recently associated with the use of habit memory in a Y-maze task (126).
Another emerging behavioral disorder that parallels some features of drug addiction is pathological video game playing or video game addiction [for review, see Ref. (127)]. Like drug addiction, long-term excessive video game playing has been associated with reduced dopamine D2 receptor binding in the dorsal striatum (128). Videogame playing is also correlated to increased activation of the dorsal striatum (129, 130), and greater dorsal striatal volumes predict higher levels of video game skill (131). People who regularly play action video games are more likely to use dorsal striatum-dependent habit memory in a virtual maze (132), and pre-training video game playing leads to habitual responding over goal-directed responding in a two-stage decision-making task (133). Thus, as proposed for drugs of abuse, playing video games might enhance video game addiction via engaging the DLS-dependent habit memory system.
Finally, the multiple memory systems approach might also be useful for understanding marijuana addiction. Although marijuana may have lower abuse potential than other illicit substances classically considered within the context of drug addiction research (e.g., cocaine, morphine, heroin, etc.), heavy cannabis use can nevertheless promote drug dependence and withdrawal symptoms as observed with other drugs of abuse (134–137). It has recently been suggested that marijuana addiction might be partially attributed to increased engagement of DLS-dependent habit memory (138). Whereas acute cannabinoid exposure impairs DLS-dependent memory function (139, 140), repeated cannabinoid exposure leads to greater DLS-dependent habitual responding in an instrumental learning task (141). In addition, heavy cannabis users display greater activation of the dorsal striatum, relative to non-users, when performing a marijuana version of the implicit association task (142), and participants with a history of cannabis use are more likely to use dorsal striatum-dependent habit memory in the virtual maze (65).
Given the successful application of the memory systems approach to emerging sources of addiction, it is reasonable to hypothesize that multiple memory systems might also be implicated in other behavioral pathologies associated with addiction, such as compulsive shopping, Internet addiction, and sex addiction. Indeed, whether the memory systems approach might be useful for understanding pathological gambling has also received some attention (143, 144).
Twenty years of experimental evidence has largely corroborated White’s (1) multiple memory systems approach to drug addiction. Evidence indicates that the hippocampus mediates contextual control of drug self-administration, the DLS mediates S–R habitual responding for drug reinforcement, and the amygdala mediates conditioned drug seeking. In addition, subsequent research has led to additional insights regarding the multiple memory systems view of drug addiction including the shift to habit memory, competition between memory systems, and the role of stress and anxiety.
Future research should attempt to integrate the memory systems approach with other theories of addiction, such as opponent motivational processes (145). It would also be useful to incorporate into the memory systems view additional features of addiction, such as drug dependence, tolerance, and withdrawal. Although the present review predominantly focused on the brain regions originally considered by White (i.e., the hippocampus, dorsal striatum, and amygdala), it should be noted that additional brain regions related to learning and memory have also been critically implicated in drug addiction and relapse, including the medial prefrontal cortex and nucleus accumbens [for review, see Ref. (13)]. Finally, although beyond the scope of the present review, it should be acknowledged that extensive evidence suggests that cellular and molecular changes in the midbrain dopaminergic system also contribute to addiction (146).
Although habit memories might be especially difficult to control, some evidence indicates that DLS-dependent memory, once acquired, can in some circumstances be suppressed (147) or even reversed (148, 149). Thus, it is possible that the pharmacological manipulations and behavioral procedures leading to the reversal or suppression of habit memory in animal models of learning might potentially be adapted to treat drug addiction and relapse in humans.
JG and MP both contributed ideas and writing of the present mini-review.
The authors declare that the research was conducted in the absence of any commercial or financial relationships that could be construed as a potential conflict of interest.
1. White NM. Addictive drugs as reinforcers: multiple partial actions on memory systems. Addiction (1996) 91(7):921–50. doi: 10.1111/j.1360-0443.1996.tb03586.x
2. White NM, McDonald RJ. Multiple parallel memory systems in the brain of the rat. Neurobiol Learn Mem (2002) 77(2):125–84. doi:10.1006/nlme.2001.4008
3. Squire LR. Memory systems of the brain: a brief history and current perspective. Neurobiol Learn Mem (2004) 82(3):171–7. doi:10.1016/j.nlm.2004.06.005
4. White NM, Packard MG, McDonald RJ. Dissociation of memory systems: the story unfolds. Behav Neurosci (2013) 127(6):813–34. doi:10.1037/a0034859
5. Packard MG, Hirsh R, White NM. Differential effects of fornix and caudate nucleus lesions on two radial maze tasks: evidence for multiple memory systems. J Neurosci (1989) 9(5):1465–72.
6. McDonald RJ, White NM. A triple dissociation of memory systems: hippocampus, amygdala, and dorsal striatum. Behav Neurosci (1993) 107(1):3–22. doi:10.1037/0735-7044.107.1.3
7. Maren S. Neurobiology of Pavlovian fear conditioning. Annu Rev Neurosci (2001) 24(1):897–931. doi:10.1146/annurev.neuro.24.1.897
8. McGaugh JL. The amygdala modulates the consolidation of memories of emotionally arousing experiences. Annu Rev Neurosci (2004) 27:1–28. doi:10.1146/annurev.neuro.27.070203.144157
9. Packard MG. Anxiety, cognition, and habit: a multiple memory systems perspective. Brain Res (2009) 1293:121–8. doi:10.1016/j.brainres.2009.03.029
10. Packard MG, Goodman J. Emotional arousal and multiple memory systems in the mammalian brain. Front Behav Neurosci (2012) 6:14. doi:10.3389/fnbeh.2012.00014
11. Packard MG, Goodman J. Factors that influence the relative use of multiple memory systems. Hippocampus (2013) 23(11):1044–52. doi:10.1002/hipo.22178
12. Schwabe L. Stress and the engagement of multiple memory systems: integration of animal and human studies. Hippocampus (2013) 23(11):1035–43. doi:10.1002/hipo.22175
13. Everitt BJ, Robbins TW. Neural systems of reinforcement for drug addiction: from actions to habits to compulsion. Nat Neurosci (2005) 8(11):1481–9. doi:10.1038/nn1579
14. Fuchs RA, Evans KA, Ledford CC, Parker MP, Case JM, Mehta RH, et al. The role of the dorsomedial prefrontal cortex, basolateral amygdala, and dorsal hippocampus in contextual reinstatement of cocaine seeking in rats. Neuropsychopharmacology (2005) 30(2):296–309. doi:10.1038/sj.npp.1300579
15. Fuchs RA, Eaddy JL, Su ZI, Bell GH. Interactions of the basolateral amygdala with the dorsal hippocampus and dorsomedial prefrontal cortex regulate drug context-induced reinstatement of cocaine-seeking in rats. Eur J Neurosci (2007) 26(2):487–98. doi:10.1111/j.1460-9568.2007.05674.x
16. Kramar CP, Barbano MF, Medina JH. Dopamine D1/D5 receptors in the dorsal hippocampus are required for the acquisition and expression of a single trial cocaine-associated memory. Neurobiol Learn Mem (2014) 116:172–80. doi:10.1016/j.nlm.2014.10.004
17. Zapata A, Minney VL, Shippenberg TS. Shift from goal-directed to habitual cocaine seeking after prolonged experience in rats. J Neurosci (2010) 30(46):15457–63. doi:10.1523/JNEUROSCI.4072-10.2010
18. Corbit LH, Nie H, Janak PH. Habitual alcohol seeking: time course and the contribution of subregions of the dorsal striatum. Biol Psychiatry (2012) 72(5):389–95. doi:10.1016/j.biopsych.2012.02.024
19. Whitelaw RB, Markou A, Robbins TW, Everitt BJ. Excitotoxic lesions of the basolateral amygdala impair the acquisition of cocaine-seeking behaviour under a second-order schedule of reinforcement. Psychopharmacology (1996) 127(1–2):213–24. doi:10.1007/BF02805996
20. Alderson HL, Robbins TW, Everitt BJ. The effects of excitotoxic lesions of the basolateral amygdala on the acquisition of heroin-seeking behaviour in rats. Psychopharmacology (2000) 153(1):111–9. doi:10.1007/s002130000527
21. Gabriele A, See RE. Reversible inactivation of the basolateral amygdala, but not the dorsolateral caudate putamen, attenuates consolidation of cocaine-cue associative learning in a reinstatement model of drug-seeking. Eur J Neurosci (2010) 32(6):1024–9. doi:10.1111/j.1460-9568.2010.07394.x
22. Sciascia JM, Reese RM, Janak PH, Chaudhri N. Alcohol-seeking triggered by discrete Pavlovian cues is invigorated by alcohol contexts and mediated by glutamate signaling in the basolateral amygdala. Neuropsychopharmacology (2015) 40:2801–12. doi:10.1038/npp.2015.130
23. Packard MG, Teather LA. Amygdala modulation of multiple memory systems: hippocampus and caudate-putamen. Neurobiol Learn Mem (1998) 69(2):163–203. doi:10.1006/nlme.1997.3815
24. Dickinson A, Wood N, Smith JW. Alcohol seeking by rats: action or habit? Q J Exp Psychol B (2002) 55(4):331–48. doi:10.1080/0272499024400016
25. Udo T, Ugalde F, DiPietro N, Eichenbaum HB, Kantak KM. Effects of persistent cocaine self-administration on amygdala-dependent and dorsal striatum-dependent learning in rats. Psychopharmacology (2004) 174(2):237–45. doi:10.1007/s00213-003-1734-1
26. Wood SC, Fay J, Sage JR, Anagnostaras SG. Cocaine and Pavlovian fear conditioning: dose-effect analysis. Behav Brain Res (2007) 176(2):244–50. doi:10.1016/j.bbr.2006.10.008
27. Wood SC, Anagnostaras SG. Memory and psychostimulants: modulation of Pavlovian fear conditioning by amphetamine in C57BL/6 mice. Psychopharmacology (2009) 202(1–3):197–206. doi:10.1007/s00213-008-1185-9
28. Iñiguez SD, Charntikov S, Baella SA, Herbert MS, Bolaños-Guzmán CA, Crawford CA. Post-training cocaine exposure facilitates spatial memory consolidation in C57BL/6 mice. Hippocampus (2012) 22(4):802–13. doi:10.1002/hipo.20941
29. DePoy L, Daut R, Brigman JL, MacPherson K, Crowley N, Gunduz-Cinar O, et al. Chronic alcohol produces neuroadaptations to prime dorsal striatal learning. Proc Natl Acad Sci U S A (2013) 110(36):14783–8. doi:10.1073/pnas.1308198110
30. Leri F, Nahas E, Henderson K, Limebeer CL, Parker LA, White NM. Effects of post-training heroin and d-amphetamine on consolidation of win-stay learning and fear conditioning. J Psychopharmacol (2013) 27(3):292–301. doi:10.1177/0269881112472566
31. Schmitzer-Torbert N, Apostolidis S, Amoa R, O’Rear C, Kaster M, Stowers J, et al. Post-training cocaine administration facilitates habit learning and requires the infralimbic cortex and dorsolateral striatum. Neurobiol Learn Mem (2015) 118:105–12. doi:10.1016/j.nlm.2014.11.007
32. Knowlton BJ. Basal ganglia: habit formation. In: Jaeger D, Jung R, editors. Encyclopedia of Computational Neuroscience. New York: Springer (2014). p. 1–17.
33. Tolman EC, Ritchie BF, Kalish D. Studies in spatial learning. IV. The transfer of place learning to other starting paths. J Exp Psychol (1947) 37(1):39–47. doi:10.1037/h0062061
34. Ritchie BF, Aeschliman B, Pierce P. Studies in spatial learning. VIII. Place performance and the acquisition of place dispositions. J Comp Physiol Psychol (1950) 43(2):73–85. doi:10.1037/h0055224
35. Hicks LH. Effects of overtraining on acquisition and reversal of place and response learning. Psychol Rep (1964) 15(2):459–62. doi:10.2466/pr0.1964.15.2.459
36. Packard MG, McGaugh JL. Inactivation of hippocampus or caudate nucleus with lidocaine differentially affects expression of place and response learning. Neurobiol Learn Mem (1996) 65(1):65–72. doi:10.1006/nlme.1996.0007
37. Yin HH, Knowlton BJ. Contributions of striatal subregions to place and response learning. Learn Mem (2004) 11(4):459–63. doi:10.1101/lm.81004
38. Adams CD, Dickinson A. Instrumental responding following reinforcer devaluation. Q J Exp Psychol (1981) 33B:109–12. doi:10.1080/14640748108400816
39. Adams CD, Dickinson A. Actions and habits: variations in associative representations during instrumental learning. In: Spear NE, Miller RR, editors. Information Processing in Animals: Memory Mechanisms. Hillsdale, NJ: Erlbaum (1981). p. 143–65.
40. Adams CD. Variations in the sensitivity of instrumental responding to reinforcer devaluation. Q J Exp Psychol (1982) 34B:77–98. doi:10.1080/14640748208400878
41. Dickinson A, Nicholas DJ. Irrelevant incentive learning during instrumental conditioning: the role of the drive-reinforcer and response-reinforcer relationships. Q J Exp Psychol (1983) 35B:249–63. doi:10.1080/14640748308400909
42. Dickinson A, Nicholas DJ, Adams CD. The effects of the instrumental contingency on susceptibility to reinforcer devaluation. Q J Exp Psychol (1983) 35B:35–51. doi:10.1080/14640748308400912
43. Corbit LH, Balleine BW. The role of the hippocampus in instrumental conditioning. J Neurosci (2000) 20(11):4233–9.
44. Yin HH, Ostlund SB, Knowlton BJ, Balleine BW. The role of the dorsomedial striatum in instrumental conditioning. Eur J Neurosci (2005) 22:513–23. doi:10.1111/j.1460-9568.2005.04218.x
45. Yin HH, Knowlton BJ, Balleine BW. Lesions of dorsolateral striatum preserve outcome expectancy but disrupt habit formation in instrumental learning. Eur J Neurosci (2004) 19:181–9. doi:10.1111/j.1460-9568.2004.03095.x
46. Quinn JJ, Pittenger C, Lee AS, Pierson JL, Taylor JR. Striatum-dependent habits are insensitive to both increases and decreases in reinforcer value in mice. Eur J Neurosci (2013) 37:1012–21. doi:10.1111/ejn.12106
47. Yin HH. From actions to habits: neuroadaptations leading to dependence. Alcohol Res Health (2008) 31(4):340–4.
48. Belin D, Jonkman S, Dickinson A, Robbins TW, Everitt BJ. Parallel and interactive learning processes within the basal ganglia: relevance for the understanding of addiction. Behav Brain Res (2009) 199(1):89–102. doi:10.1016/j.bbr.2008.09.027
49. Schwabe L, Dickinson A, Wolf OT. Stress, habits, and drug addiction: a psychoneuroendocrinological perspective. Exp Clin Psychopharmacol (2011) 19(1):53–63. doi:10.1037/a0022212
50. Hogarth L, Balleine BW, Corbit LH, Killcross S. Associative learning mechanisms underpinning the transition from recreational drug use to addiction. Ann N Y Acad Sci (2013) 1282(1):12–24. doi:10.1111/j.1749-6632.2012.06768.x
51. Murray JE, Belin D, Everitt BJ. Double dissociation of the dorsomedial and dorsolateral striatal control over the acquisition and performance of cocaine seeking. Neuropsychopharmacology (2012) 37(11):2456–66. doi:10.1038/npp.2012.104
52. Clemens KJ, Castino MR, Cornish JL, Goodchild AK, Holmes NM. Behavioral and neural substrates of habit formation in rats intravenously self-administering nicotine. Neuropsychopharmacology (2014) 39:2584–93. doi:10.1038/npp.2014.111
53. Corbit LH, Nie H, Janak PH. Habitual responding for alcohol depends upon both AMPA and D2 receptor signaling in the dorsolateral striatum. Front Behav Neurosci (2014) 8:301. doi:10.3389/fnbeh.2014.00301
54. Schoenbaum G, Setlow B. Cocaine makes actions insensitive to outcomes but not extinction: implications for altered orbitofrontal-amygdalar function. Cereb Cortex (2005) 15(8):1162–9. doi:10.1093/cercor/bhh216
55. Nelson A, Killcross S. Amphetamine exposure enhances habit formation. J Neurosci (2006) 26(14):3805–12. doi:10.1523/JNEUROSCI.4305-05.2006
56. Nordquist RE, Voorn P, De Mooij-van Malsen JG, Joosten RNJMA, Pennartz CMA, Vanderschuren LJMJ. Augmented reinforcer value and accelerated habit formation after repeated amphetamine treatment. Eur Neuropsychopharmacol (2007) 17(8):532–40. doi:10.1016/j.euroneuro.2006.12.005
57. LeBlanc KH, Maidment NT, Ostlund SB. Repeated cocaine exposure facilitates the expression of incentive motivation and induces habitual control in rats. PLoS One (2013) 8:e61355. doi:10.1371/journal.pone.0061355
58. Nelson AJ, Killcross S. Accelerated habit formation following amphetamine exposure is reversed by D1, but enhanced by D2, receptor antagonists. Front Neurosci (2013) 7:76. doi:10.3389/fnins.2013.00076
59. Corbit LH, Chieng BC, Balleine BW. Effects of repeated cocaine exposure on habit learning and reversal by N-acetylcysteine. Neuropsychopharmacology (2014) 39(8):1893–901. doi:10.1038/npp.2014.37
60. Miles FJ, Everitt BJ, Dickinson A. Oral cocaine seeking by rats: action or habit? Behav Neurosci (2003) 117(5):927–38. doi:10.1037/0735-7044.117.5.927
61. Mangieri RA, Cofresí RU, Gonzales RA. Ethanol seeking by Long Evans rats is not always a goal-directed behavior. PLoS One (2012) 7:e42886. doi:10.1371/journal.pone.0042886
62. Sjoerds Z, De Wit S, Van Den Brink W, Robbins TW, Beekman ATF, Penninx BWJH, et al. Behavioral and neuroimaging evidence for overreliance on habit learning in alcohol-dependent patients. Transl Psychiatry (2013) 3(12):e337. doi:10.1038/tp.2013.107
63. Packard MG, McGaugh JL. Quinpirole and d-amphetamine administration posttraining enhances memory on spatial and cued discriminations in a water maze. Psychobiology (1994) 22(1):54–60.
64. Matthews DB, Ilgen M, White AM, Best PJ. Acute ethanol administration impairs spatial performance while facilitating nonspatial performance in rats. Neurobiol Learn Mem (1999) 72(3):169–79. doi:10.1006/nlme.1998.3900
65. Bohbot VD, Balso D, Conrad K, Konishi K, Leyton M. Caudate nucleus-dependent navigational strategies are associated with increased use of addictive drugs. Hippocampus (2013) 23(11):973–84. doi:10.1002/hipo.22187
66. Poldrack RA, Packard MG. Competition among multiple memory systems: converging evidence from animal and human brain studies. Neuropsychologia (2003) 41(3):245–51. doi:10.1016/S0028-3932(02)00157-4
67. Matthews DB, Best PJ. Fimbria/fornix lesions facilitate the learning of a nonspatial response task. Psychon Bull Rev (1995) 2(1):113–6. doi:10.3758/BF03214415
68. Schroeder JP, Wingard JC, Packard MG. Post-training reversible inactivation of hippocampus reveals interference between memory systems. Hippocampus (2002) 12(2):280–4. doi:10.1002/hipo.10024
69. Matthews DB, Simson PE, Best PJ. Acute ethanol impairs spatial memory but not stimulus/response memory in the rat. Alcohol Clin Exp Res (1995) 19(4):902–9. doi:10.1111/j.1530-0277.1995.tb00965.x
70. White AM, Elek TM, Beltz TL, Best PJ. Spatial performance is more sensitive to ethanol than nonspatial performance regardless of cue proximity. Alcohol Clin Exp Res (1998) 22(9):2102–7. doi:10.1111/j.1530-0277.1998.tb05922.x
71. Matthews DB, Morrow AL, Tokunaga S, McDaniel JR. Acute ethanol administration and acute allopregnanolone administration impair spatial memory in the Morris water task. Alcohol Clin Exp Res (2002) 26(11):1747–51. doi:10.1111/j.1530-0277.2002.tb02479.x
72. Berry RB, Matthews DB. Acute ethanol administration selectively impairs spatial memory in C57BL/6J mice. Alcohol (2004) 32(1):9–18. doi:10.1016/j.alcohol.2003.09.005
73. Silvers JM, Tokunaga S, Berry RB, White AM, Matthews DB. Impairments in spatial learning and memory: ethanol, allopregnanolone, and the hippocampus. Brain Res Rev (2003) 43(3):275–84. doi:10.1016/j.brainresrev.2003.09.002
74. Badanich KA, Becker HC, Woodward JJ. Effects of chronic intermittent ethanol exposure on orbitofrontal and medial prefrontal cortex-dependent behaviors in mice. Behav Neurosci (2011) 125(6):879–91. doi:10.1037/a0025922
75. Coleman LG Jr, He J, Lee J, Styner M, Crews FT. Adolescent binge drinking alters adult brain neurotransmitter gene expression, behavior, brain regional volumes, and neurochemistry in mice. Alcohol Clin Exp Res (2011) 35(4):671–88. doi:10.1111/j.1530-0277.2010.01385.x
76. Kuzmin A, Liljequist S, Meis J, Chefer V, Shippenberg T, Bakalkin G. Repeated moderate-dose ethanol bouts impair cognitive function in Wistar rats. Addict Biol (2012) 17(1):132–40. doi:10.1111/j.1369-1600.2010.00224.x
77. Coleman LG, Liu W, Oguz I, Styner M, Crews FT. Adolescent binge ethanol treatment alters adult brain regional volumes, cortical extracellular matrix protein and behavioral flexibility. Pharmacol Biochem Behav (2014) 116:142–51. doi:10.1016/j.pbb.2013.11.021
78. Matthews DB, Silvers JR. The use of acute ethanol administration as a tool to investigate multiple memory systems. Neurobiol Learn Mem (2004) 82(3):299–308. doi:10.1016/j.nlm.2004.06.007
79. Broening HW, Morford LL, Inman-Wood SL, Fukumura M, Vorhees CV. 3, 4-methylenedioxymethamphetamine (ecstasy)-induced learning and memory impairments depend on the age of exposure during early development. J Neurosci (2001) 21(9):3228–35.
80. Williams MT, Morford LL, Wood SL, Wallace TL, Fukumura M, Broening HW, et al. Developmental d-methamphetamine treatment selectively induces spatial navigation impairments in reference memory in the Morris water maze while sparing working memory. Synapse (2003) 48(3):138–48. doi:10.1002/syn.10159
81. Vorhees CV, Reed TM, Skelton MR, Williams MT. Exposure to 3, 4-methylenedioxymethamphetamine (MDMA) on postnatal days 11-20 induces reference but not working memory deficits in the Morris water maze in rats: implications of prior learning. Int J Dev Neurosci (2004) 22(5):247–59. doi:10.1016/j.ijdevneu.2004.06.003
82. Cohen MA, Skelton MR, Schaefer TL, Gudelsky GA, Vorhees CV, Williams MT. Learning and memory after neonatal exposure to 3, 4-methylenedioxymethamphetamine (ecstasy) in rats: interaction with exposure in adulthood. Synapse (2005) 57(3):148–59. doi:10.1002/syn.20166
83. Skelton MR, Williams MT, Vorhees CV. Treatment with MDMA from P11-20 disrupts spatial learning and path integration learning in adolescent rats but only spatial learning in older rats. Psychopharmacology (2006) 189(3):307–18. doi:10.1007/s00213-006-0563-4
84. Ma MX, Chen YM, He J, Zeng T, Wang JH. Effects of morphine and its withdrawal on Y-maze spatial recognition memory in mice. Neuroscience (2007) 147(4):1059–65. doi:10.1016/j.neuroscience.2007.05.020
85. Belcher AM, Feinstein EM, O’Dell SJ, Marshall JF. Methamphetamine influences on recognition memory: comparison of escalating and single-day dosing regimens. Neuropsychopharmacology (2008) 33(6):1453–63. doi:10.1038/sj.npp.1301510
86. Tramullas M, Martínez-Cué C, Hurlé MA. Chronic administration of heroin to mice produces up-regulation of brain apoptosis-related proteins and impairs spatial learning and memory. Neuropharmacology (2008) 54(4):640–52. doi:10.1016/j.neuropharm.2007.11.018
87. North A, Swant J, Salvatore MF, Gamble-George J, Prins P, Butler B, et al. Chronic methamphetamine exposure produces a delayed, long-lasting memory deficit. Synapse (2013) 67(5):245–57. doi:10.1002/syn.21635
88. Fole A, Martin M, Morales L, Del Olmo N. Effects of chronic cocaine treatment during adolescence in Lewis and Fischer-344 rats: novel location recognition impairment and changes in synaptic plasticity in adulthood. Neurobiol Learn Mem (2015) 123:179–86. doi:10.1016/j.nlm.2015.06.001
89. Zhou M, Luo P, Lu Y, Li CJ, Wang DS, Lu Q, et al. Imbalance of HCN1 and HCN2 expression in hippocampal CA1 area impairs spatial learning and memory in rats with chronic morphine exposure. Prog Neuropsychopharmacol Biol Psychiatry (2015) 56:207–14. doi:10.1016/j.pnpbp.2014.09.010
90. Kathirvelu B, Colombo PJ. Effects of lentivirus-mediated CREB expression in the dorsolateral striatum: memory enhancement and evidence for competitive and cooperative interactions with the hippocampus. Hippocampus (2013) 23(11):1066–74. doi:10.1002/hipo.22188
91. Baudonnat M, Guillou JL, Husson M, Vandesquille M, Corio M, Decorte L, et al. Disrupting effect of drug-induced reward on spatial but not cue-guided learning: implication of striatal protein kinase A/cAMP response element-binding protein pathway. J Neurosci (2011) 31:16517–28. doi:10.1523/JNEUROSCI.1787-11.2011
92. Packard MG, Wingard JC. Amygdala and “emotional” modulation of the relative use of multiple memory systems. Neurobiol Learn Mem (2004) 82(3):243–52. doi:10.1016/j.nlm.2004.06.008
93. Elliott AE, Packard MG. Intra-amygdala anxiogenic drug infusion prior to retrieval biases rats towards the use of habit memory. Neurobiol Learn Mem (2008) 90(4):616–23. doi:10.1016/j.nlm.2008.06.012
94. Wingard JC, Packard MG. The amygdala and emotional modulation of competition between cognitive and habit memory. Behav Brain Res (2008) 193(1):126–31. doi:10.1016/j.bbr.2008.05.002
95. Packard MG, Gabriele A. Peripheral anxiogenic drug injections differentially affect cognitive and habit memory: role of basolateral amygdala. Neuroscience (2009) 164(2):457–62. doi:10.1016/j.neuroscience.2009.07.054
96. Leong KC, Goodman J, Packard MG. Buspirone blocks the enhancing effect of the anxiogenic drug RS 79948-197 on consolidation of habit memory. Behav Brain Res (2012) 234(2):299–302. doi:10.1016/j.bbr.2012.07.009
97. Goodman J, Leong KC, Packard MG. Glucocorticoid enhancement of dorsolateral striatum-dependent habit memory requires concurrent noradrenergic activity. Neuroscience (2015) 311:1–8. doi:10.1016/j.neuroscience.2015.10.014
98. Kim JJ, Lee HJ, Han JS, Packard MG. Amygdala is critical for stress-induced modulation of hippocampal long-term potentiation and learning. J Neurosci (2001) 21(14):5222–8.
99. Schwabe L, Dalm S, Schächinger H, Oitzl MS. Chronic stress modulates the use of spatial and stimulus-response learning strategies in mice and man. Neurobiol Learn Mem (2008) 90(3):495–503. doi:10.1016/j.nlm.2008.07.015
100. Leong KC, Packard MG. Exposure to predator odor influences the relative use of multiple memory systems: role of basolateral amygdala. Neurobiol Learn Mem (2014) 109:56–61. doi:10.1016/j.nlm.2013.11.015
101. Taylor SB, Anglin JM, Paode PR, Riggert AG, Olive MF, Conrad CD. Chronic stress may facilitate the recruitment of habit-and addiction-related neurocircuitries through neuronal restructuring of the striatum. Neuroscience (2014) 280:231–42. doi:10.1016/j.neuroscience.2014.09.029
102. Leong KC, Goodman J, Packard MG. Post-training re-exposure to fear conditioned stimuli enhances memory consolidation and biases rats toward the use of dorsolateral striatum-dependent response learning. Behav Brain Res (2015) 291:195–200. doi:10.1016/j.bbr.2015.05.022
103. Goode TE, Leong KC, Goodman J, Maren S, Packard MG. Enhancement of striatum-dependent memory by conditioned fear is mediated by beta-adrenergic receptors in the basolateral amygdala. Neurobiology of Stress (in press). doi:10.1016/j.ynstr.2016.02.004
104. Schwabe L, Oitzl MS, Philippsen C, Richter S, Bohringer A, Wippich W, et al. Stress modulates the use of spatial versus stimulus-response learning strategies in humans. Learn Mem (2007) 14(1–2):109–16. doi:10.1101/lm.435807
105. Schwabe L, Schächinger H, de Kloet ER, Oitzl MS. Corticosteroids operate as a switch between memory systems. J Cogn Neurosci (2010) 22(7):1362–72. doi:10.1162/jocn.2009.21278
106. Schwabe L, Tegenthoff M, Höffken O, Wolf OT. Concurrent glucocorticoid and noradrenergic activity shifts instrumental behavior from goal-directed to habitual control. J Neurosci (2010) 30(24):8190–6. doi:10.1523/JNEUROSCI.0734-10.2010
107. Schwabe L, Tegenthoff M, Höffken O, Wolf OT. Mineralocorticoid receptor blockade prevents stress-induced modulation of multiple memory systems in the human brain. Biol Psychiatry (2013) 74(11):801–8. doi:10.1016/j.biopsych.2013.06.001
108. Schwabe L, Wolf OT. Stress prompts habit behavior in humans. J Neurosci (2009) 29(22):7191–8. doi:10.1523/JNEUROSCI.0979-09.2009
109. Schwabe L, Wolf OT. Socially evaluated cold pressor stress after instrumental learning favors habits over goal-directed action. Psychoneuroendocrinology (2010) 35(7):977–86. doi:10.1016/j.psyneuen.2009.12.010
110. Guenzel FM, Wolf OT, Schwabe L. Glucocorticoids boost stimulus-response memory formation in humans. Psychoneuroendocrinology (2014) 45:21–30. doi:10.1016/j.psyneuen.2014.02.015
111. Higgins RL, Marlatt GA. Fear of interpersonal evaluation as a determinant of alcohol consumption in male social drinkers. J Abnorm Psychol (1975) 84(6):644–51. doi:10.1037/0021-843X.84.6.644
112. Marlatt GA, Gordon JR. Determinants of relapse: implications for the maintenance of behavior change. In: Davidson PO, Davidson SM, editors. Behavioral Medicine: Changing Lifestyles. New York: Brunne/Mazel (1980). p. 410–52.
113. Newcomb MD, Bentler PM. Impact of adolescent drug use and social support on problems of young adults: a longitudinal study. J Abnorm Psychol (1988) 97:64–75. doi:10.1037/0021-843X.97.1.64
114. Wallace BC. Psychological and environmental determinants of relapse in crack cocaine smokers. J Subst Abuse Treat (1989) 6(2):95–106. doi:10.1016/0740-5472(89)90047-0
115. Kaplan HB, Johnson RJ. Relationships between circumstances surrounding initial illicit drug use and escalation of drug use: moderating effects of gender and early adolescent experiences. In: Glantz M, Pickens R, editors. Vulnerability to Drug Abuse. Washington, DC: American Psychological Association (1992). p. 200–358.
116. Harrison PA, Fulkerson JA, Beebe TJ. Multiple substance use among adolescent physical and sexual abuse victims. Child Abuse Neglect (1997) 21:529–39. doi:10.1016/S0145-2134(97)00013-6
117. Chilcoat HD, Breslau N. Posttraumatic stress disorder and drug disorders: testing causal pathways. Arch Gen Psychiatry (1998) 55(10):913–7. doi:10.1001/archpsyc.55.10.913
118. Piazza PV, Le Moal M. The role of stress in drug self-administration. Trends Pharmacol Sci (1998) 19(2):67–74. doi:10.1016/S0165-6147(97)01115-2
119. Goodman J, Leong KC, Packard MG. Emotional modulation of multiple memory systems: implications for the neurobiology of post-traumatic stress disorder. Rev Neurosci (2012) 23(5–6):627–43. doi:10.1515/revneuro-2012-0049
120. Sinha R, Lacadie C, Skudlarski P, Fulbright RK, Rounsaville BJ, Kosten TR, et al. Neural activity associated with stress-induced cocaine craving: a functional magnetic resonance imaging study. Psychopharmacology (2005) 183(2):171–80. doi:10.1007/s00213-005-0147-8
121. Avena NM, Rada P, Hoebel BG. Evidence for sugar addiction: behavioral and neurochemical effects of intermittent, excessive sugar intake. Neurosci Biobehav Rev (2008) 32(1):20–39. doi:10.1016/j.neubiorev.2007.04.019
122. Gearhardt AN, Corbin WR, Brownell KD. Food addiction: an examination of the diagnostic criteria for dependence. J Addict Med (2009) 3(1):1–7. doi:10.1097/ADM.0b013e318193c993
123. Smith DG, Robbins TW. The neurobiological underpinnings of obesity and binge eating: a rationale for adopting the food addiction model. Biol Psychiatry (2013) 73(9):804–10. doi:10.1016/j.biopsych.2012.08.026
124. de Jong JW, Meijboom KE, Vanderschuren LJ, Adan RA. Low control over palatable food intake in rats is associated with habitual behavior and relapse vulnerability: individual differences. PLoS One (2013) 8(9):e74645. doi:10.1371/journal.pone.0074645
125. Furlong TM, Jayaweera HK, Balleine BW, Corbit LH. Binge-like consumption of a palatable food accelerates habitual control of behavior and is dependent on activation of the dorsolateral striatum. J Neurosci (2014) 34(14):5012–22. doi:10.1523/JNEUROSCI.3707-13.2014
126. Hargrave SL, Davidson TL, Zheng W, Kinzig KP. Western diets induce blood-brain barrier leakage and alter spatial strategies in rats. Behav Neurosci (2016) 130(1):123–35. doi:10.1037/bne0000110
127. Smith KL, Hummer TA, Hulvershorn LA. Pathological video gaming and its relationship to substance use disorders. Curr Addict Rep (2015) 2(4):302–9. doi:10.1007/s40429-015-0075-6
128. Weinstein AM. Computer and video game addiction – a comparison between game users and non-game users. Am J Drug Alcohol Abuse (2010) 36(5):268–76. doi:10.3109/00952990.2010.491879
129. Kätsyri J, Hari R, Ravaja N, Nummenmaa L. The opponent matters: elevated fMRI reward responses to winning against a human versus a computer opponent during interactive video game playing. Cereb Cortex (2013) 23(12):2829–39. doi:10.1093/cercor/bhs259
130. Kätsyri J, Hari R, Ravaja N, Nummenmaa L. Just watching the game ain’t enough: striatal fMRI reward responses to successes and failures in a video game during active and vicarious playing. Front Hum Neurosci (2013) 7:278. doi:10.3389/fnhum.2013.00278
131. Erickson KI, Boot WR, Basak C, Neider MB, Prakash RS, Voss MW, et al. Striatal volume predicts level of video game skill acquisition. Cereb Cortex (2010) 20:2522–30. doi:10.1093/cercor/bhp293
132. West GL, Drisdelle BL, Konishi K, Jackson J, Jolicoeur P, Bohbot VD. Habitual action video game playing is associated with caudate nucleus-dependent navigational strategies. Proc R Soc B (2015) 282(1808). doi:10.1098/rspb.2014.2952
133. Liu S, Schad DJ, Kuschpel MS, Rapp MA, Heinz A. Music and video gaming during breaks: influence of habitual versus goal-directed decision making. Paper Presented at 45th Annual Meeting of the Society for Neuroscience. Chicago, IL: Society for Neuroscience (2015).
134. de Fonseca FR, Carrera MRA, Navarro M, Koob GF, Weiss F. Activation of corticotropin-releasing factor in the limbic system during cannabinoid withdrawal. Science (1997) 276(5321):2050–4. doi:10.1126/science.276.5321.2050
135. Cornelius JR, Chung T, Martin C, Wood DS, Clark DB. Cannabis withdrawal is common among treatment-seeking adolescents with cannabis dependence and major depression, and is associated with rapid relapse to dependence. Addict Behav (2008) 33(11):1500–5. doi:10.1016/j.addbeh.2008.02.001
136. Greene MC, Kelly JF. The prevalence of cannabis withdrawal and its influence on adolescents’ treatment response and outcomes: a 12-month prospective investigation. J Addict Med (2014) 8:359–67. doi:10.1097/ADM.0000000000000064
137. Wagner FA, Anthony JC. From first drug use to drug dependence; developmental periods of risk for dependence upon marijuana, cocaine, and alcohol. Neuropsychopharmacology (2002) 26:479–88. doi:10.1016/S0893-133X(01)00367-0
138. Goodman J, Packard MG. The influence of cannabinoids on learning and memory processes of the dorsal striatum. Neurobiol Learn Mem (2015) 125:1–14. doi:10.1016/j.nlm.2015.06.008
139. Rueda-Orozco PE, Soria-Gomez E, Montes-Rodriguez CJ, Martínez-Vargas M, Galicia O, Navarro L, et al. A potential function of endocannabinoids in the selection of a navigation strategy by rats. Psychopharmacology (2008) 198(4):565–76. doi:10.1007/s00213-007-0911-z
140. Goodman J, Packard MG. Peripheral and intra-dorsolateral striatum injections of the cannabinoid receptor agonist WIN 55,212-2 impair consolidation of stimulus-response memory. Neuroscience (2014) 274:128–37. doi:10.1016/j.neuroscience.2014.05.007
141. Nazzaro C, Greco B, Cerovic M, Baxter P, Rubino T, Trusel M, et al. SK channel modulation rescues striatal plasticity and control over habit in cannabinoid tolerance. Nat Neurosci (2012) 15:284–93. doi:10.1038/nn.3022
142. Ames SL, Grenard JL, Stacy AW, Xiao L, He Q, Wong SW, et al. Functional imaging of implicit marijuana associations during performance on an implicit association test (IAT). Behav Brain Res (2013) 256:494–502. doi:10.1016/j.bbr.2013.09.013
143. Redish AD, Jensen S, Johnson A. A unified framework for addiction: vulnerabilities in the decision process. Behav Brain Sci (2008) 31(04):415–37. doi:10.1017/S0140525X0800472X
144. Brevers D, Bechara A, Cleeremans A, Noël X. Iowa Gambling Task (IGT): twenty years after – gambling disorder and IGT. Front Psychol (2013) 4:665. doi:10.3389/fpsyg.2013.00665
145. Koob GF, Le Moal M. Neurobiological mechanisms for opponent motivational processes in addiction. Philos Trans R Soc B Biol Sci (2008) 363(1507):3113–23. doi:10.1098/rstb.2008.0094
146. Hyman SE, Malenka RC, Nestler EJ. Neural mechanisms of addiction: the role of reward-related learning and memory. Annu Rev Neurosci (2006) 29:565–98. doi:10.1146/annurev.neuro.29.051605.113009
147. Goodman J, Packard M. The memory system engaged during acquisition determines the effectiveness of different extinction protocols. Front Behav Neurosci (2015) 9:314. doi:10.3389/fnbeh.2015.00314
148. Palencia CA, Ragozzino ME. The contribution of NMDA receptors in the dorsolateral striatum to egocentric response learning. Behav Neurosci (2005) 119(4):953–60. doi:10.1037/0735-7044.119.4.953
149. Rueda-Orozco PE, Montes-Rodriguez CJ, Soria-Gomez E, Méndez-Díaz M, Prospéro-García O. Impairment of endocannabinoids activity in the dorsolateral striatum delays extinction of behavior in a procedural memory task in rats. Neuropharmacology (2008) 55(1):55–62. doi:10.1016/j.neuropharm.2008.04.013
Keywords: memory, drug addiction, hippocampus, striatum, amygdala, stress, anxiety
Citation: Goodman J and Packard MG (2016) Memory Systems and the Addicted Brain. Front. Psychiatry 7:24. doi: 10.3389/fpsyt.2016.00024
Received: 01 December 2015; Accepted: 11 February 2016;
Published: 25 February 2016
Edited by:
Vincent David, Centre National de la Recherche Scientifique (CNRS), FranceReviewed by:
Jacques Micheau, University of Bordeaux 1, FranceCopyright: © 2016 Goodman and Packard. This is an open-access article distributed under the terms of the Creative Commons Attribution License (CC BY). The use, distribution or reproduction in other forums is permitted, provided the original author(s) or licensor are credited and that the original publication in this journal is cited, in accordance with accepted academic practice. No use, distribution or reproduction is permitted which does not comply with these terms.
*Correspondence: Mark G. Packard, bWFya3BhY2thcmRAdGFtdS5lZHU=
Disclaimer: All claims expressed in this article are solely those of the authors and do not necessarily represent those of their affiliated organizations, or those of the publisher, the editors and the reviewers. Any product that may be evaluated in this article or claim that may be made by its manufacturer is not guaranteed or endorsed by the publisher.
Research integrity at Frontiers
Learn more about the work of our research integrity team to safeguard the quality of each article we publish.