- 1Limnological Station, Department of Plant and Microbial Biology, University of Zurich, Kilchberg, Switzerland
- 2Physics of Aquatic Systems Laboratory, Margaretha Kamprad Chair, Institute of Environmental Engineering, École Polytechnique Fédérale de Lausanne, Lausanne, Switzerland
- 3Eawag, Swiss Federal Institute of Aquatic Science and Technology, Surface Waters – Research and Management, Kastanienbaum, Switzerland
- 4Zurich Water Supply, Zurich, Switzerland
After years of partial winter mixing in Lake Zurich (Switzerland), a complete turnover of the water column reoccurred during winter/spring 2021. It was favored by a cold, windy winter and a small difference of water temperatures between the surface zone and a hypolimnion (deep water zone) that had gradually warmed during the previous years. The trend of declining phytoplankton spring blooms due to incomplete winter mixing was interrupted by mass development of algae due to the upwelling of nutrients accumulated in the hypolimnion. The effects of this singular deep mixing on the microbial food web during spring were studied in a high-frequency sampling campaign and compared with data from two years of partial winter mixing (2020 and 2022). A particular focus was put on the quantitative composition of the ciliate assemblage. Our results showed that not all organisms reacted equally to the nutrient (phosphorus) boost in the surface zone. Centric diatoms and cryptophytes profited most directly from the deep mixing, outcompeting the otherwise dominant cyanobacterium Planktothrix rubescens. Heterotrophic bacteria and their top predators, the ‘heterotrophic nanoflagellates’ trophic guild, were less affected by the nutrient supply and showed only short-lived increases of maximal biomass. The assemblage composition of ciliate morphotypes was highly resilient over the three years, presumably due to the range of acceptable food items of the predominant omnivorous species. However, numerous ciliate morphotypes showed brief mass development in 2021, and Balanion planctonicum, small Urotricha species and tintinnids were significantly more frequent than in 2020/2022. Small interception-feeding morphotypes apparently profited from the rich supply of their cryptomonad food, and tintinnid morphotypes additionally benefited from the availability of building material (e.g., centric diatom shells) for their loricae. In summary, we show that effects of lake warming in deep stratifying lakes are not as unidirectional as previously presumed, and we reveal resilience of the pelagic ciliate morphotype assemblage to lake warming related interannual variability in Lake Zurich.
1 Introduction
Lakes around the world are affected by warming. Surface water temperatures have risen steadily over the past century in response to increasing air temperature (Woolway et al., 2020; Dokulil et al., 2021) and global radiation (Schmid and Köster, 2016), and lake warming is expected to even aggravate in the future (Råman Vinnå et al., 2021; Desgué-Itier et al., 2023). However, the extent of lake warming varies; it depends on the prevailing local air temperature, wind conditions (O’Reilly et al., 2015) and lake morphology (Toffolon et al., 2014). Lakes in the mid and high latitudes of the northern hemisphere have undergone greater warming than those in low latitudes and the southern hemisphere (Schneider and Hook, 2010). In Central Europe, both warming of surface water in spring and summer (Posch et al., 2012; Yankova et al., 2016) and reduced cooling in winter have been observed (Dokulil, 2014). The resulting discrepancy in heat content between surface and deep water leads to a strengthening of the thermal stratification and water column stability and thus to an earlier onset and prolongation of seasonal stratification (Shimoda et al., 2011; Dokulil, 2014). This development has a negative impact on the intensity and duration of winter mixing in deep lakes (Woolway and Merchant, 2019), which in turn has profound consequences for nutrient-poor (oligotrophic and oligo-mesotrophic) systems: It directly affects the oxygenation of the deep water zone (hypolimnion) (Schwefel et al., 2016) and the upwelling of nutrient-rich deep water into the surface zone (epilimnion) (Salmaso et al., 2018; Schwefel et al., 2019), which is essential for lake productivity (O’Reilly et al., 2003; Yankova et al., 2017).
Lake Zurich, a deep oligo-mesotrophic Swiss lake (Peeters et al., 2002; Knapp et al., 2021) is a representative example for documented changes in thermal stratification and mixing regime due to climate warming. Since the 1970s, surface temperatures in Lake Zurich have increased steadily by 0.39°C per decade as to data collected by Zurich Water Supply (Knapp et al., 2021). The number of winters with shallow mixing (not affecting the deep hypolimnion) has steadily increased since the 2000s (Posch et al., 2012), and since 2013, holomixis (complete water turnover affecting the entire water body) seems to be the exception rather than the rule (Yankova et al., 2017). The resulting decrease in epilimnetic nutrients due to diminished replenishment from the deep hypolimnion has persistently affected phytoplankton blooms in early spring. Based on a long-term dataset (since 1975) of the total phytoplankton community (monthly samples analyzed according to Utermöhl, 1958), it was shown that both, centric diatoms and cryptophytes, are the first and quantitative dominant eukaryotic algae reacting on vernal nutrient replenishment in Lake Zurich. However, low epilimnetic phosphorous concentrations likely have hampered algal growth, especially of centric diatoms, but also of cryptophytes (Yankova et al., 2017). On the other hand, nutrients, especially orthophosphate (PO4), has accumulated in the deep hypolimnion during periods with incomplete water turnover. In contrast, the cyanobacterium Planktothrix rubescens has benefited from prolonged thermal stratification and weak winter mixing, promoting its mass development in summer (Posch et al., 2012; Knapp et al., 2021).
Shifts in phytoplankton abundance and composition determine the occurrence and growth of primary consumers and their predators within aquatic food webs (Sommer et al., 2012). Accordingly, numbers of zooplankton (Phyllopoda and Copepoda) in Lake Zurich have also been declining for decades (Yankova et al., 2017). However, it is not known what effect the altered mixing regime and epilimnetic nutrient availability have on other important grazers of phytoplankton. Ciliates (Ciliophora) form a monophyletic group of morphologically very divers unicellular eukaryotes and are key elements of the planktonic food web. As the first and most effective grazers of phytoplankton, they play a particularly important role during the spring bloom (Posch et al., 2022). Furthermore, ciliate assemblages affect several trophic levels, as they also harbor omnivorous, bacterivorous, and even predatory species (Weisse, 2017). They thus act as central energy pathway by channeling carbon and nutrients from their food source to higher trophic levels, i.e., to their main consumers such as rotifers, cladocerans, and copepods (Sanders and Wickham, 1993).
The most recent climate scenarios predict further warming of Lake Zurich in the coming decades (Råman Vinnå et al., 2021). While the effects of the resulting incomplete winter turnover have already been studied (Yankova et al., 2017), the consequences of a sporadically reoccurring holomixis after a long period of incomplete winter mixing have not been explored (Holzner et al., 2009). Such a scenario would lead to intense nutrient enrichment of the upper water layer and consequently strongly favor the phytoplankton (Lepori et al., 2018).
During high-frequency spring bloom sampling campaigns in Lake Zurich, effects of a nearly complete turnover was studied as deep mixing reoccurred in winter 2021, within a multi-annual long period of incomplete winter mixing. Here we present in detail three aspects of this nutrient pulse: (i) We analyzed physicochemical parameters to describe the functioning of complete winter mixing and the effects on oxygen and nutrient concentrations in the water column (including a total phosphorus budget of Lake Zurich) during spring. (ii) We quantified the vernal succession of microbial food web components in the seasons after deep winter mixing and compared this dataset with observed dynamics during two years with only partial winter mixing. We analyzed the differences in the quantity of centric diatoms and cryptophytes, bacteria, heterotrophic nanoflagellates (HNF), ciliates and rotifers for three consecutive years, i.e., 2020 (partial mixing), 2021 (deep mixing) and 2022 (partial mixing). (iii) Particular emphasis was put on the composition of the pelagic ciliates assemblage and their role in the food web, i.e., as link between lower (phytoplankton, bacteria, HNF) and higher (rotifers) trophic levels.
2 Material and methods
2.1 Lake Zurich
Lake Zurich is an oligo-mesotrophic, prealpine lake situated in a densely populated, economically important region in the northern part of Switzerland (Bossard et al., 2001). The lake is separated by a natural dam in two major basins: an upper, shallow part (zmax = 48 m, 21.7 km2, 0.4 km3), fed by the Linth River, and a lower, much deeper part (zmax = 136 m, 66.6 km2, 3.3 km3), herein referred to as Lake Zurich. This part of the lake is monomictic, with meanwhile irregular holomixis between February and March, typically followed by a summer-long period of thermal stratification.
2.2 Sampling and measurements of in situ parameters
Sampling of the Lake Zurich water column was conducted biweekly over four consecutive years (September 2019 until December 2022) at the long-term monitoring site, located close to the deepest point of the lake (47°18’16’’N, 8°34’31’’E, zmax = 136 m). In spring 2020–2022 (February–May), sampling was carried out in shorter intervals: biweekly to weekly in 2020 at the long-term monitoring site and three times per week in 2021 and 2022 in the vicinity of the Limnological Station Kilchberg (47°19’31’’ N, 8°33’81’’ E, zmax = 100 m). During the spring campaigns, samples for the analysis of bacteria, HNF, phytoplankton, ciliates and rotifers were collected from 1 to 8 m depth with an integrating water sampler (IWS 3, Hydro-Bios Apparatebau, Germany). Samples were stored in a 10 L container and brought directly to the laboratory, where they were immediately processed according to the workflow of Figure 1. Physicochemical and biological in situ parameters, i.e., water temperature (°C), oxygen concentration (mg O2 L−1) and in vivo P. rubescens-related chlorophyll a concentration (µg Chl a L−1; Beutler et al., 2002; Hofmann and Peeters, 2013) were measured throughout the entire water column using a multiparameter probe (6600 V2, YSI Incorp., United States) and a TS-16-12 fluoroprobe (bbe Moldaenke GmbH, Germany), respectively. A specific calibration via optical fingerprints for the detection of P. rubescens was first described by Leboulanger et al. (2002) and is meanwhile included in the basic setup of the commercially available bbe fluoroprobes. We could show in a long-term (12 years) comparison of both parameters (see Supplementary Figure S2 in Knapp et al., 2021) that in vivo related chlorophyll a measurement is a good proxy for P. rubescens biovolume. The detection of P. rubescens via the phycoerythrin-specific sensor of the multiparameter probe proofed as inadequate as this sensor does not differentiate between signals originating from P. rubescens and cryptophytes. Profiles of temperature and oxygen were used to determine the mixing depth in early spring. Maximal mixing depth was defined as the depth, where oxygen concentration and temperature abruptly increased (Supplementary Figure 1).
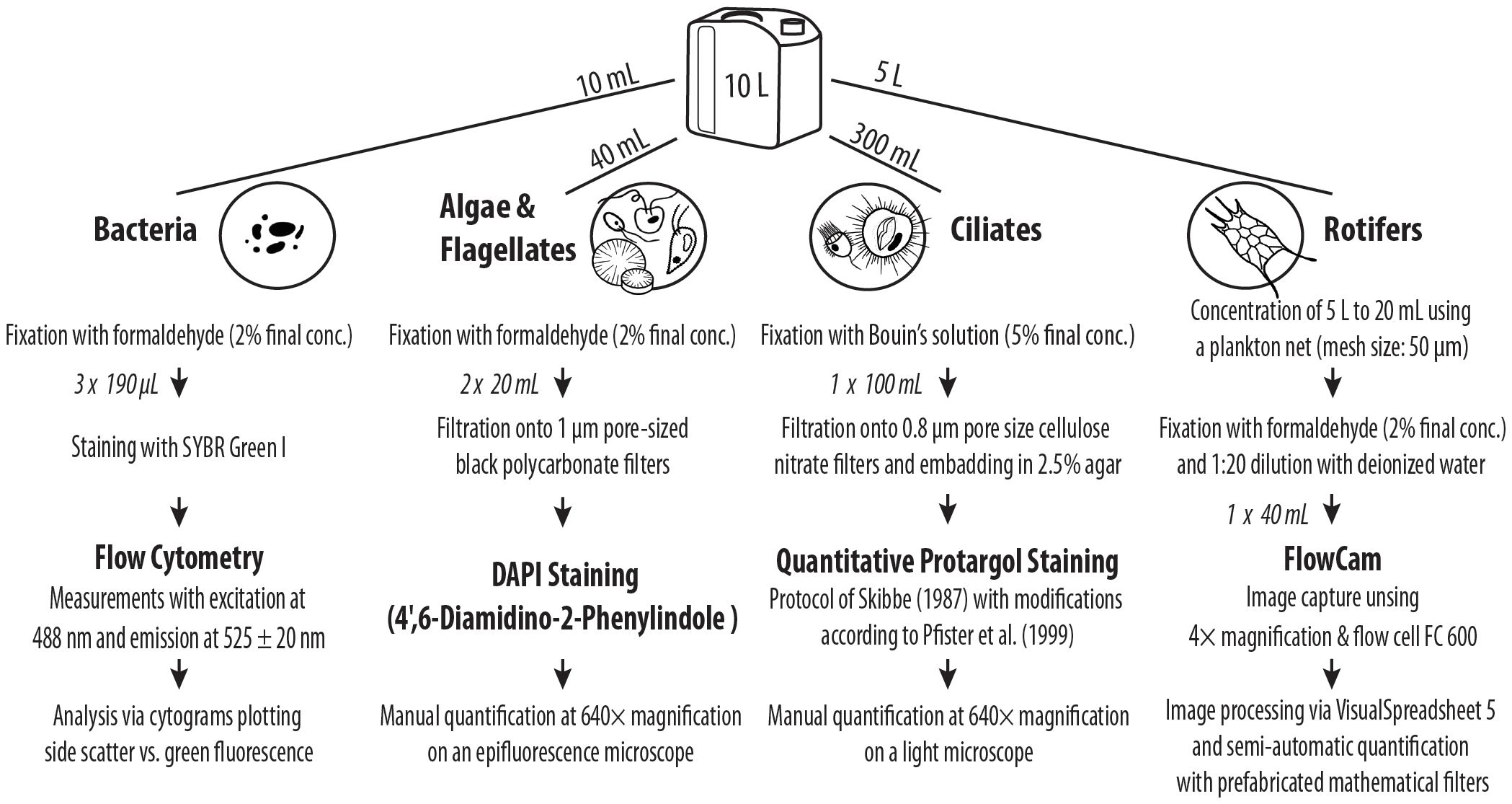
Figure 1. Workflow for the quantification of bacteria, phytoplankton (Cryptomonas spp., Rhodomonas spp. and centric diatoms) and heterotrophic nanoflagellates (HNF), ciliates and rotifers.
Concentrations of total phosphorus (Ptot), PO4 and silica were measured at 17 distinct depths (0–136 m) monthly throughout the sampling period and additionally biweekly in March and April (0–20 m) by Zurich Water Supply. Seasonal phosphorus inventories were established by volume-integration of the Ptot concentration profile, assuming homogeneity within topographically defined horizontal layers. The phosphorus discharge via the Limmat River outflow was calculated using the average Ptot content in the uppermost 5 m of Lake Zurich and the water outflow rate from https://www.hydrodaten.admin.ch. Daily values of air temperature were obtained from the Mythenquai Meteorological Station managed by the Zurich Water Police (https://tecson.ch).
2.2.1 Flow cytometric analysis of bacteria
For bacterial counts, 10 mL lake water was fixed with formaldehyde (2% final conc.) and cells were stained with SYBR Green I (Merck Sigma Aldrich, Germany) before bacterial abundances were evaluated in triplicates of 190 µL subsamples via flow cytometry (Cytoflex S, Beckman Coulter, United States). The excitation was set at 488 nm and the emission was measured at 525 ± 20 nm. Bacterial counts included both, heterotrophic bacteria and coccoid cyanobacteria, the latter with an average percentage of 0.13% (maximum 0.52%) on total counts for all years of investigation. Bacteria with high and low nucleic acid content were quantified from cytograms plotting side scatter vs. green fluorescence.
2.2.2 Quantification of selected phytoplankton groups and HNF
Abundances of HNF and selected phytoplankton groups, i.e., cryptophytes (Cryptomonas spp., Rhodomonas spp.), and centric diatoms (small diatoms: Ø 5–10 µm and large diatoms: Ø 10–25 µm), were determined via fluorescence labelling. Therefore, 40 mL lake water was fixed with formaldehyde (2% final conc.), filtered in duplicates (each 20 mL) onto 1 µm pore-sized, black polycarbonate filters (Whatman, Germany) and stained with 4’,6-Diamidino-2-Phenylindole (DAPI; Porter and Feig, 1980). Organisms were manually counted at 640× magnification with an Axio Imager M1 epifluorescence microscope (Zeiss, Germany) applying UV-excitation (365 nm) and green-excitation (546 nm) to check for the presence of chlorophyll, respectively. At least 50 counting fields were evaluated per filter, corresponding to an average of 450 (130–2,065) counted cells per sample.
2.2.3 Quantification of ciliates
For the quantification of ciliates, 300 mL of water samples were fixed with Bouin’s solution (5% final conc.; containing 10.71 mL picric acid (100%), 3.57 mL formaldehyde (37%) and 720 µL glacial acetic acid (15%); Skibbe, 1994). Subsamples of 100 mL were filtered onto 0.8 µm pore size cellulose nitrate filters (Sartorius, Germany) with counting grids of 9 mm2 per square and embedded in 2.5% agar (Agar Noble, DifcoTM, Becton Dickinson, United States). Subsequently, Quantitative Protargol Staining was performed following the protocol of Skibbe (1994) with modifications according to Pfister et al. (1999). Finally, the filters were preserved in Canada Balsam (Merck Millipore, Germany). Ciliate abundances were determined at 640× magnification on a Zeiss Axio Imager M1 microscope. At least six squares or a minimum of 250 ciliates per filter were counted. Finally, to record the full diversity of ciliates, the entire filter was re-examined for the presence of rare morphotypes. Morphological identification and description of feeding types was done according to the taxonomy keys by volumes I–IV of Foissner et al. (1991, 1992, 1994, 1995) and Foissner et al. (1999). The classification systems by Gao et al. (2016) and Lynn (2008) were consulted for the taxonomic affiliation of the detected species. Net growth rate was determined for ciliate morphotypes that contributed to the maxima of total ciliate abundance in 2021 (9th April–16th April). For this purpose, exponential growth rates were estimated as r = ln(Nt/N0) t−1, where r is the rate of population growth (d−1), N0 and Nt are the initial and final population densities and t the time since sampling day 0, respectively.
2.2.4 Quantification of rotifers
Five liters of lake water were concentrated to 20 mL using a plankton net with a mesh-size of 50 µm and fixed with formaldehyde (2% final conc.). Quantification and identification of rotifers were performed with an image flow cytometer (FlowCam 8000; Yokogawa Fluid Imaging Technologies, USA), a combination of flow cytometer and light microscope, equipped with a camera. To avoid clogging, the samples were diluted with deionized water in a ratio of 1:20. Of these, subsamples of 40 mL (corresponding to an original volume of 500 mL) were analyzed using 4× magnification and a flow cell FC600. Particles in the range of 15–1,000 µm were captured in the auto-image mode. Image processing and analysis was conducted with the provided software VisualSpreadsheet 5. By gathering around fifty images of each rotifer morphospecies from different perspectives, image libraries were created manually for the most abundant morphospecies: Asplanchna spp., Kellicottia longispina, Polyarthra vulgaris, Keratella quadrata and Keratella cochlearis. Based on these libraries, mathematical filters were created that allowed the automatic assignment of images to the respective species. The resulting assignments were manually reviewed, and the remaining images were examined for undetected rotifers. The software thus allowed for a semi-automatic quantification of the most common morphotypes.
2.2.5 Carbon content
The cellular carbon content (C-content) was calculated from volumetric measurements or, in the case of rotifers, based on values from the literature (Table 1). The total C-content (biomass) was calculated by multiplying the cellular C-content (organismic carbon in case of rotifers) times the respective total abundances of the organismic entities. Average cell volume of bacteria was determined according to Posch et al. (2009) using size measurements from samples taken in spring 2019 for Lake Zurich. We measured the average cell parameters of DAPI-stained HNF (n=60 cells) and calculated the average cell volume based on geometric formulae. Average cell volume was converted to cellular carbon content via the formula published by Børsheim and Bratbak (1987), using the approach for fixed cells (Table 1). Cell volumes of algal groups were based on live measurements and were provided by Zurich Water Supply. These values were used to determine the cellular C-content (Table 1) of diatoms and cryptophytes separately (Montagnes et al., 1994). Ciliate cell volume was calculated for each species separately, using a geometric formula that most closely resembled the body shape of the species (Supplementary Table 1). A general conversion factor for protists (Table 1) was applied to determine the cellular C-content of each ciliate species (Menden-Deuer and Lessard, 2000).
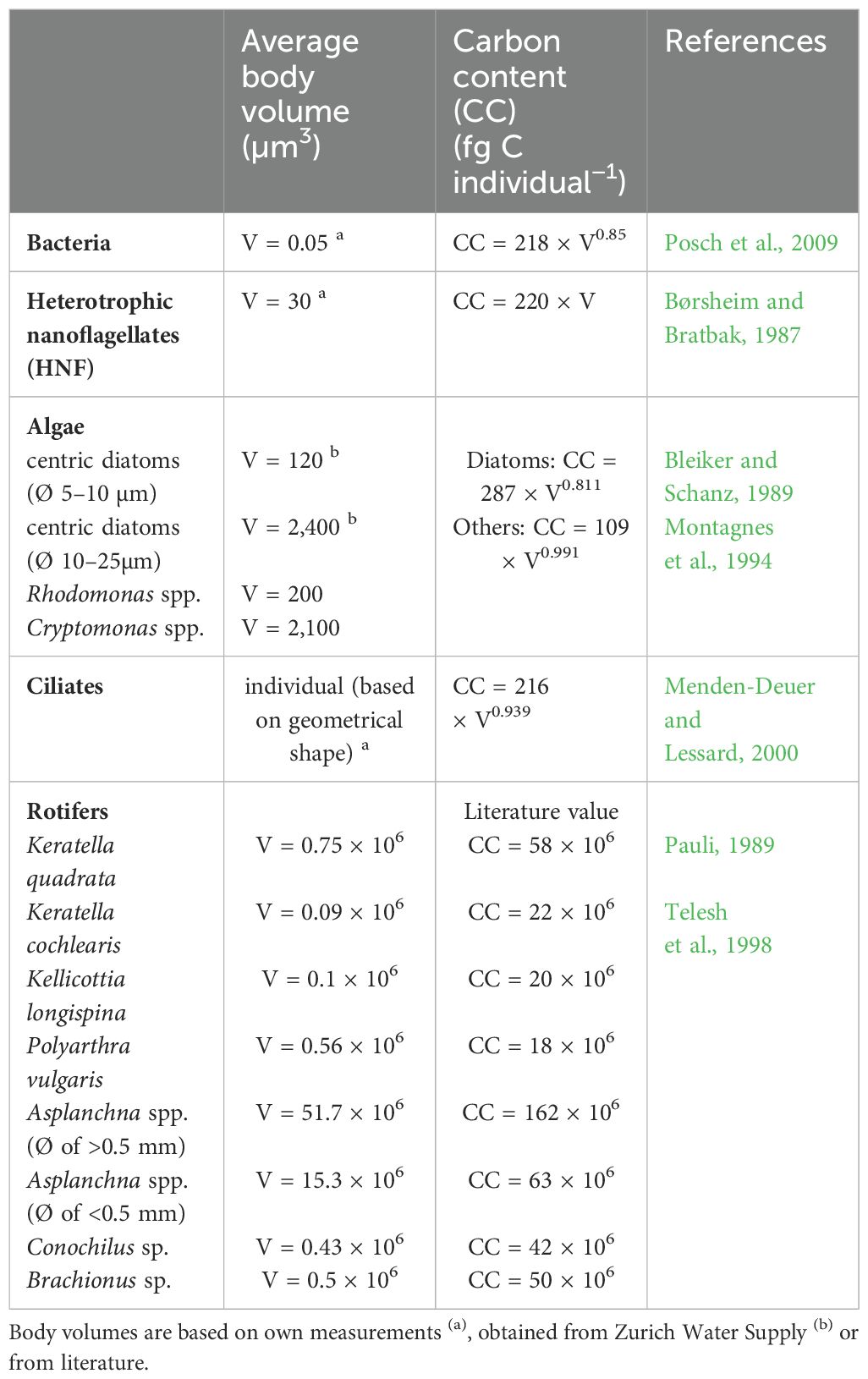
Table 1. Values of the average body volume (µm3) of the investigated organismic groups, formulae used to calculate the cellular carbon content (fg C cell−1), and the carbon content of one individual in case of rotifers and corresponding references.
For inter-annual comparison of the carbon transfer within the microbial food, daily values of biomass were calculated based on interpolation between the sampling days, and finally, the average of all daily values was determined. The same was conducted for P. rubescens, using the average concentration of P. rubescens-related Chl a between 1 and 8 m depths. Results were visualized in a simplified microbial food web according to Fenchel (1987).
2.3 Statistical analysis
Statistical analyses were carried out with the software R (version 4.0.5). Differences between the three years, regarding the vernal biomass of cryptophytes, diatoms, bacteria, HNF, total ciliates and selected ciliate morphospecies, and regarding the chlorophyll content of P. rubescens were tested using Mann–Whitney U tests (package stats) followed by a Dunn’s tests (package FSA). To differentiate between the high and less productive phase of diatoms and ciliate morphospecies, their biomass dynamics were split into an early (end-February until mid-April) and a late phase before statistical analyses were conducted. False discovery rate of multiple testing was corrected using the Benjamini–Hochberg method.
3 Results
3.1 Physical and chemical parameters
At the beginning of February 2020, temperature differences between surface and deep water were still high (6°C vs. 4.8°C). Due to the still existing density gradient, convective mixing reached only ~80 m depth on February 13th (Supplementary Figure 1). This deep-water replacement caused a temporary increase in oxygen concentration in the hypolimnion and a short upwelling of PO4 (maximum of 3.5 µg PO4‐P L−1) into the euphotic zone from mid-February to mid-March (Figures 2B, C).
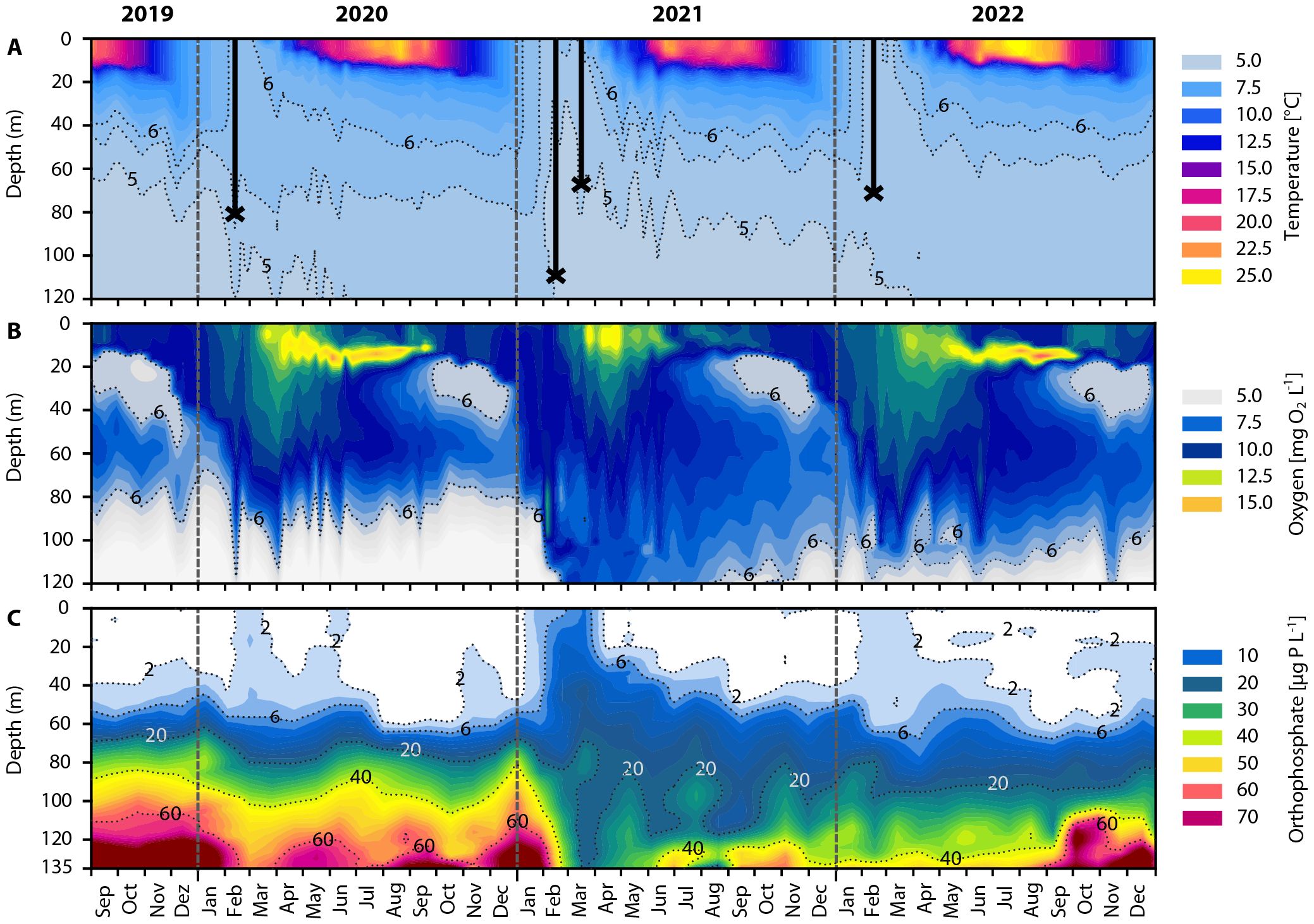
Figure 2. Physicochemical parameter contour plot of Lake Zurich from September to December 2019, and for the entire years 2020, 2021 and 2022. (A) Water temperature (°C). Black lines with crosses indicate maximal winter mixing depth (see also Supplementary Figure 1). (B) Oxygen concentration (mg O2 L−1). Black dotted line displays the 6 mg O2 L−1 threshold. (C) Orthophosphate (PO4) concentration (µg PO4-P L−1). Black dotted lines and corresponding values indicate distinct PO4 isolines. Temperature and oxygen profiles (A, B) were measured between 0 and 100/120 m, PO4 concentration (C) between 0 and 136 m. Grey dashed vertical lines represent the beginning of the year.
In contrast, in February 2021 two phenomena caused isothermal conditions favoring much deeper mixing: i) unusually high deep-water temperature combined with ii) strong lake surface cooling (Figure 2A). i) In addition to the long-term trend of increasing hypolimnetic temperatures, another substantial warming occurred in spring 2020. As a result, in January 2021, at the end of the stratified period, the temperature at 120 m depth reached 5.2°C (Figure 2A). ii) Low air temperatures, which averaged at 3.1°C (below TLake) from mid-November 2020 to mid-February 2021, led to a constant strong cooling of the surface water. After one week of average air temperatures of −2°C, the lowest heat content was reached on February 17th, 2021. The cold epilimnetic temperature (~5°C) in combination with the warm hypolimnetic water supported deep convective mixing down to 110 m. Below that depth, the last ten meters above the lake bottom remained stably density-stratified by dissolved solids (Supplementary Figure 1). Deep mixing continued due to relatively long periods of barely present warming until late March. During this time, conditions in the lake were characterized by short stratification episodes interrupted by short cooling and shallow mixing events. This led to a continuous homogenization of the water column that resulted in a second unusual deep mixing to ~60 m depth on March 17th (Figure 2A). Deep convective mixing together with recurring turbulences increased the downwelling of oxygen-rich surface water, leading to hypolimnetic oxygen replenishment (Figure 2B). During this period, oxygen concentration at great depth exceeded the 6 mg O2 L−1 threshold, which can be used as a rough proxy for mixing depth (Örn et al., 1981). Hypolimnetic oxygen concentrations remained high until the end of summer 2021. Simultaneously, PO4 was transported multiple times to the euphotic zone, causing finally a three-fold rise in concentration compared to 2020 (maximum of 8.5 µg PO4‐P L−1 in mid-March 2021). A drastic decline in hypolimnetic PO4 concentrations was observed during that time, which remained at low level until September 2022. In addition, Ptot content (including PO4 as well as particulate and organismic bound phosphorus) of Lake Zurich decreased continuously during the entire year 2021. At the end of 2021 and throughout 2022, Ptot was 20 metric tons P lower compared to 2020 (Supplementary Figure 2). Heavy rain falls lasting from May to July in 2021 (MeteoSwiss, 2021) led to a high outflow of the River Limmat and thus to an increase in Ptot discharge to 37 tons P year−1. In comparison, the calculated annual Ptot discharge was only 33 tons P year−1 in 2020 and 23 tons P year−1 in 2022 (Supplementary Figure 3).
The deep and intense water turnover in 2021 again led to a cooling of the deep water layers (below 5°C, Figure 2A). Together with the relatively warm lake surface at the end of the stratification period in 2021, temperature conditions in 2022 were similar to those in winter 2020. Thus, a partial water turnover to a depth of ~70 m was observed in mid-February 2022 (Supplementary Figure 1) and PO4 concentrations in the euphotic epilimnion increased only to ~2.7 µg PO4‐P L−1.
3.2 Successions during the spring phytoplankton bloom
An exceptional increase of maximal cell numbers and biomass was observed for nearly all studied organisms (Figure 3A; Supplementary Figure 4). In particular, centric diatoms (mainly Cyclotella spp. and Stephanodiscus spp.) increased in abundance and thus in biomass already in early spring during the turbulent phase and after the second deep mixing in mid-March. The numbers of large centric diatoms rose to a greater extent than that of small ones, exceeding the maximal biomass of the 2020 population by a factor of six. In 2022, the numbers of centric diatoms remained extremely low throughout the spring period. Also, biomass of cryptophytes (Cryptomonas and Rhodomonas spp.) increased by fivefold within one week in April 2021, clearly exceeding the peaks of the years 2020 and 2022 (Figure 3A). In general, peak values of cryptophytes and diatoms were followed by maximum abundances and biomass of bacteria, HNF and ciliates (Figure 3A; Supplementary Figure 4). Towards the end of April 2021, the maxima of these microorganisms were at least twice as high as in 2020 and 2022. Additionally, the proportion of active bacteria (with high nucleic acid content) was also higher in 2021 compared to values observed in the other years (Supplementary Figure 4).
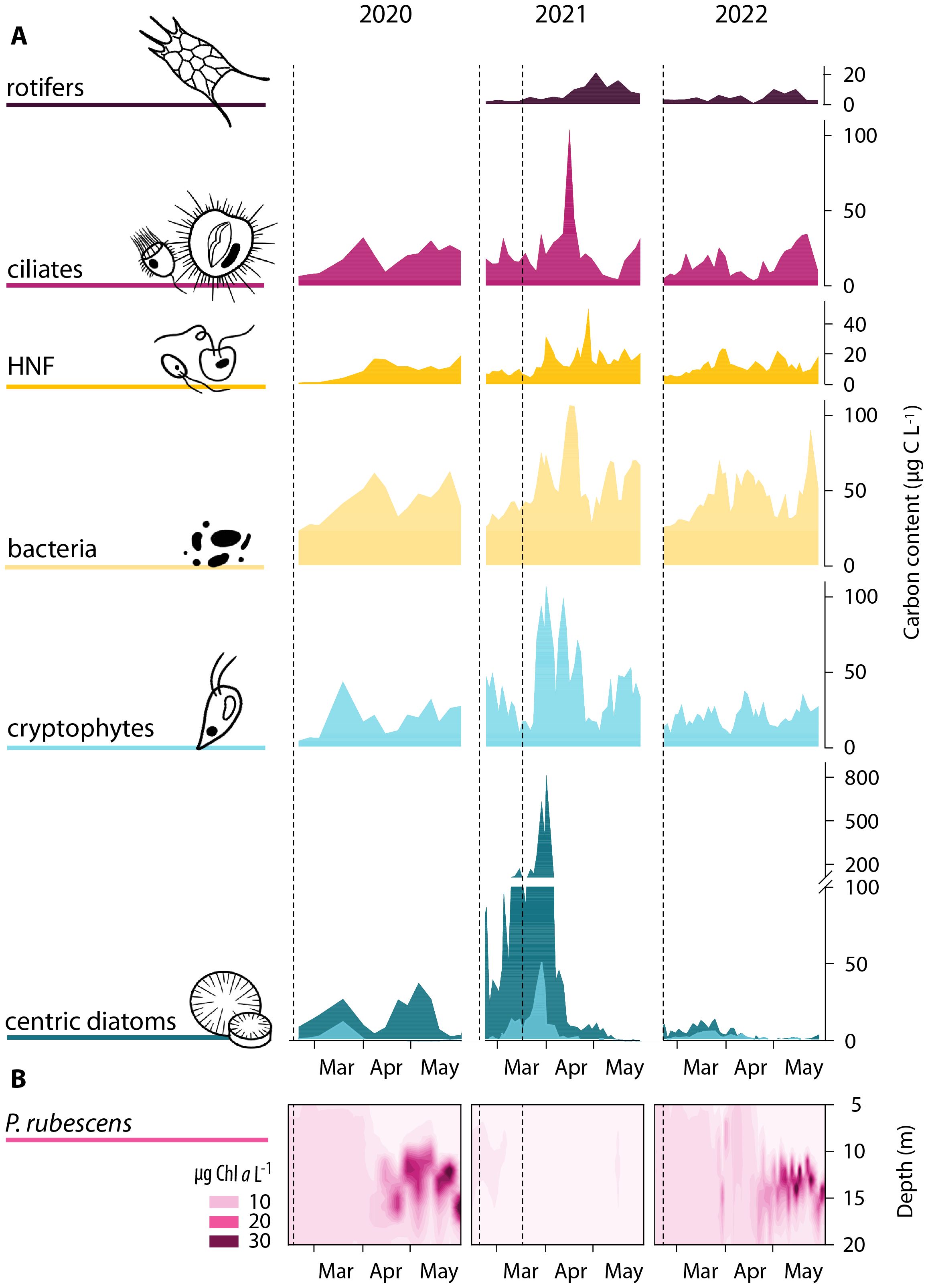
Figure 3. Succession patterns of the investigated organisms in Lake Zurich from mid-February to end-May 2020 (left), 2021 (middle) and 2022 (right). (A) Biomass (carbon content in µg C L−1) calculated from cell counts and cellular/individual carbon content (Table 1) of rotifers, ciliates, heterotrophic nanoflagellates (HNF), bacteria, cryptophytes and centric diatoms found at a depth between 1 and 8 m. Different size of centric diatoms is indicated by colors, light blue: Ø 5–10 µm, dark blue: Ø 10–25 µm. Biomass of rotifers was analyzed in 2021 and 2022 only. (B) P. rubescens-related Chl a values from 5 to 20 m depth. Dashed lines in (A, B) represent times of deep mixing.
The numbers and biomass of rotifers were analyzed in 2021 and 2022. In both years, rotifer biomass increased towards the second half of spring, with highest values recorded in late April and May (Figure 3A; Supplementary Figure 4). Maximum biomass in 2021, dominated by the species Keratella quadrata, Asplanchna spp., Kellicotia longispina and Brachionus sp., exceeded that of 2022 by a factor of two, in the case of Keratella cochlearis and Polyarthra vulgaris by a factor of five.
Although, after winter, the cyanobacterium P. rubescens started with low population densities in all three years, its development during spring varied greatly (Figure 3B). P. rubescens-derived Chl a in the metalimnion increased towards end-April in the years 2020/22. In contrast, no growth was observed in spring 2021, and the cyanobacterium remained nearly absent for the rest of the stratified season.
3.3 Ciliate succession
In spring 2020, 2021 and 2022, a total of 47 different ciliate morphotypes were identified, 28 at species and 19 at genus level. Of these, 39 morphotypes were observed in all three years (Figure 4A). The proportion of functional groups, i.e., algivorous, bacterivorous, mixotrophic, omnivorous and raptorial ciliates, was similar in all years (Figure 4B). The share of mixotrophs was slightly lower in 2021, compared to the adjacent years, while algivores were more represented in 2021/2022.
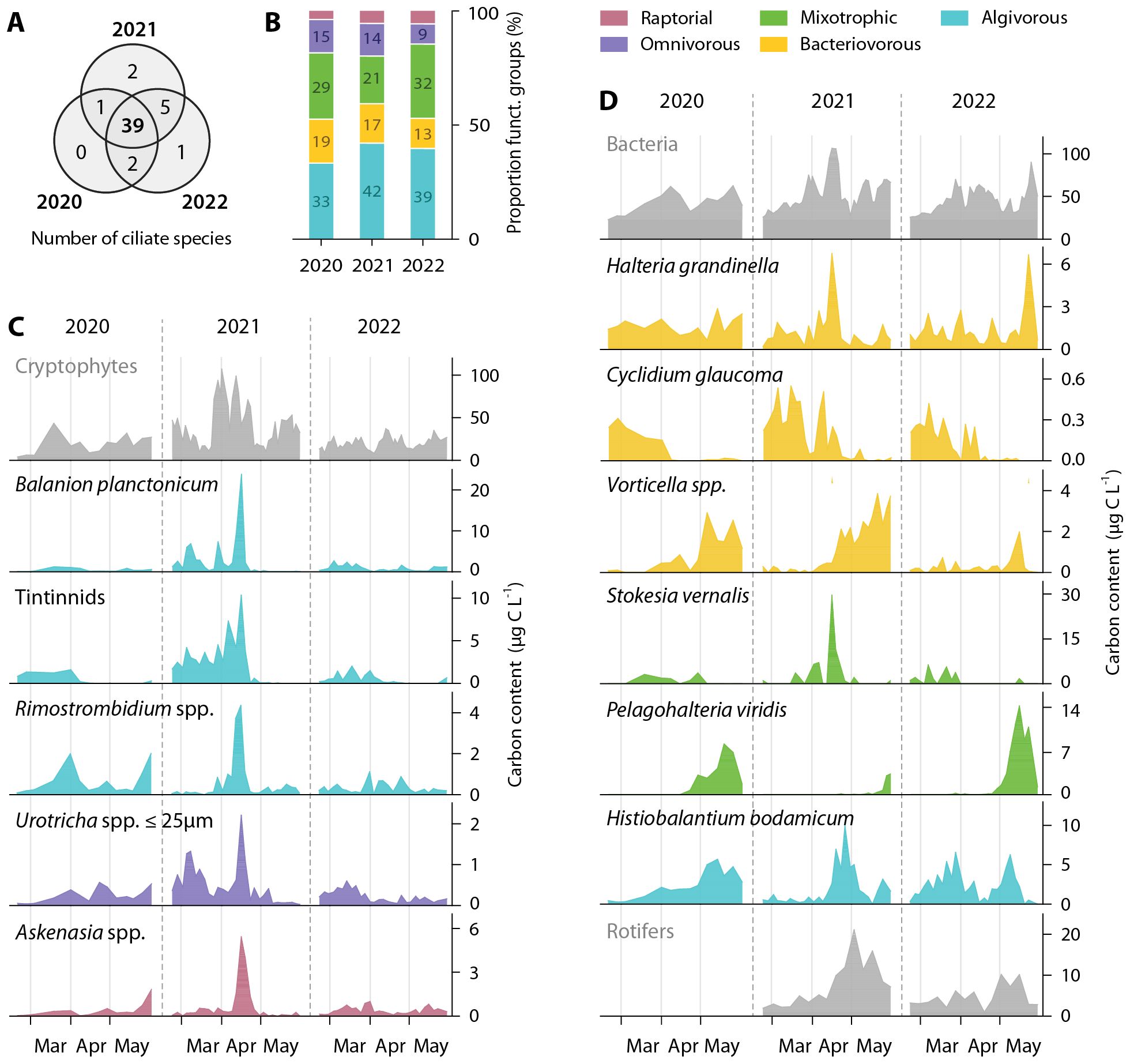
Figure 4. Number of ciliate morphotypes (A), their proportion to functional groups (B) and succession patterns (C, D) from a depth of 1–8 m in Lake Zurich from mid-February to end-May 2020, 2021 and 2022. (C, D) Succession patterns showing the biomass (carbon content in µg C L−1) of 11 selected, abundant ciliate morphotypes (see color code at top-right) in combination with potential food sources (cryptophytes and bacteria) and predators/competitors (rotifers), all depicted in grey. Note the different scales for biomass among the different morphotypes/organismic groups. Light-grey lines indicate beginning of the months, grey dashed lines separate the three years. Different colors in (B–D) reflect distinct functional groups, shown at the top-right of the figure.
Overall, 2021 was an exceptional year regarding ciliate dynamics. Numerous ciliate species had distinctly higher maximal abundances, contributing to peak densities of ~140,000 ciliates L−1 on 16th April 2021 (max. 2020: ~33,000 cells L−1; 2022: ~23,000 cells L−1; see also Supplementary Table 2 for maxima of selected ciliate morphotypes). This corresponded to 104 µg C L−1, about three times higher than the maxima in 2020/22 (Figure 3A). This short-lasting peak was dominated by the small algivorous Balanion planctonicum (20.3 µg C L−1) and the large mixotrophic Stokesia vernalis (29.5 µg C L−1, Figures 4C, D). B. planctonicum was also the most abundant morphospecies (75,000 cells L−1) with respect to total ciliate counts, while S. vernalis was represented by very low cell numbers (634 cells L−1). Further, tintinnids (Tintinnidium pusillum and Tintinnopsis cylindrata) were highly abundant in spring 2021 (Figure 4C). Contrary to 2020/2022, maximum values were not observed in early spring but tintinnids further increased until 16th April, resulting in a fourfold higher amount of total biomass (total from mid-February to end-May). Simultaneously, other ciliates like the algivorous small Rimostrombidium spp. (mainly R. hyalinum and R. branchykinetum), omnivorous Urotricha spp. (Ø <25 µm), the bacterivorous Halteria grandinella as well as raptorial Askenasia spp. reached highest abundances and biomass (Figures 4C, D). At this time B. planctonicum, Rimostrombidium spp. and Askenasia spp. reached high net growth rates of 0.5 d−1, followed by tintinnids, Urotricha spp. (Ø <25 µm), Halteria grandinella with net growth rates of 0.3 d−1. The bacterivorous ciliate Cyclidium glaucoma showed similar growth dynamics in all three years, with decreasing biomass towards mid-spring (Figure 4D). Again, biomass peaks in 2021 were slightly higher.
After the short-lasting peak in mid-April, the ciliate population declined. Total ciliate biomass decreased to a minimum of ~45 µg C L−1 on 17th May 2021 (Figure 3A). Many ciliate morphotypes remained at very low abundances thereafter until the end of the sampling campaign at the end of May. One exception was the large, mainly algivorous ciliate Histiobalantium bodamicum (Figure 4D). At the beginning of spring 2021, its biomass was lower than in 2020 and 2022. In late April and early May, however, peak values were 1.5 times higher than those of the other two years. In the second half of spring 2021, also peritrichs of the genus Vorticella increased in abundance, exceeding the total biomass of the neighboring years (Figure 4D). In the following year, the Vorticella populations decreased drastically and reached only one third of the maximum of 2021. During the study period, the mixotrophic Pelagohalteria viridis was the latest representative in the ciliate succession to reach maximum biomass (Figure 4D). In 2021, with a delay of three weeks compared to 2020 and 2022, the peak was even further behind.
3.4 Consequences for the microbial food web
In all three years, the vernal PO4 enrichment in the epilimnion promoted a bloom of centric diatoms and cryptophytes, which served as nutritional basis for many consumers within the microbial food web. In addition to single high abundance peaks in 2021, also averaged population densities and biomass of the studied autotrophic organisms differed significantly between 2021 and 2020/2022 (Dunn’s test, p<0.05). Significant differences between the three years were found for diatoms until mid of April, for cryptophytes during the entire spring period. In 2021, total biomass of diatoms increased by fourfold and doubled for cryptophytes relative to the previous year. In contrast, the population density of P. rubescens dropped significantly, with 75% less total Chl a than in 2020/22 (Figure 5). The effects of phosphorus availability in 2021 on the heterotrophic components of the microbial food web were less pronounced than on phototrophs and no significant difference in biomass was observed for bacteria, HNF and total ciliates during spring (Dunn’s test, p>0.05). However, biomass of three ciliate morphotypes (Balanion planctonicum, Urotricha spp. <25µm and tintinnids) increased significantly (Dunn’s test, p<0.05) in the first half of spring 2021 compared to 2020/2022, whereas other morphotypes showed only short-term maxima in otherwise similar trends to 2020/2022 (Supplementary Table 3). In the second half of spring 2021, the biomass of the mixotrophic species Pelagohalteria viridis was significantly lower than in the two other years.
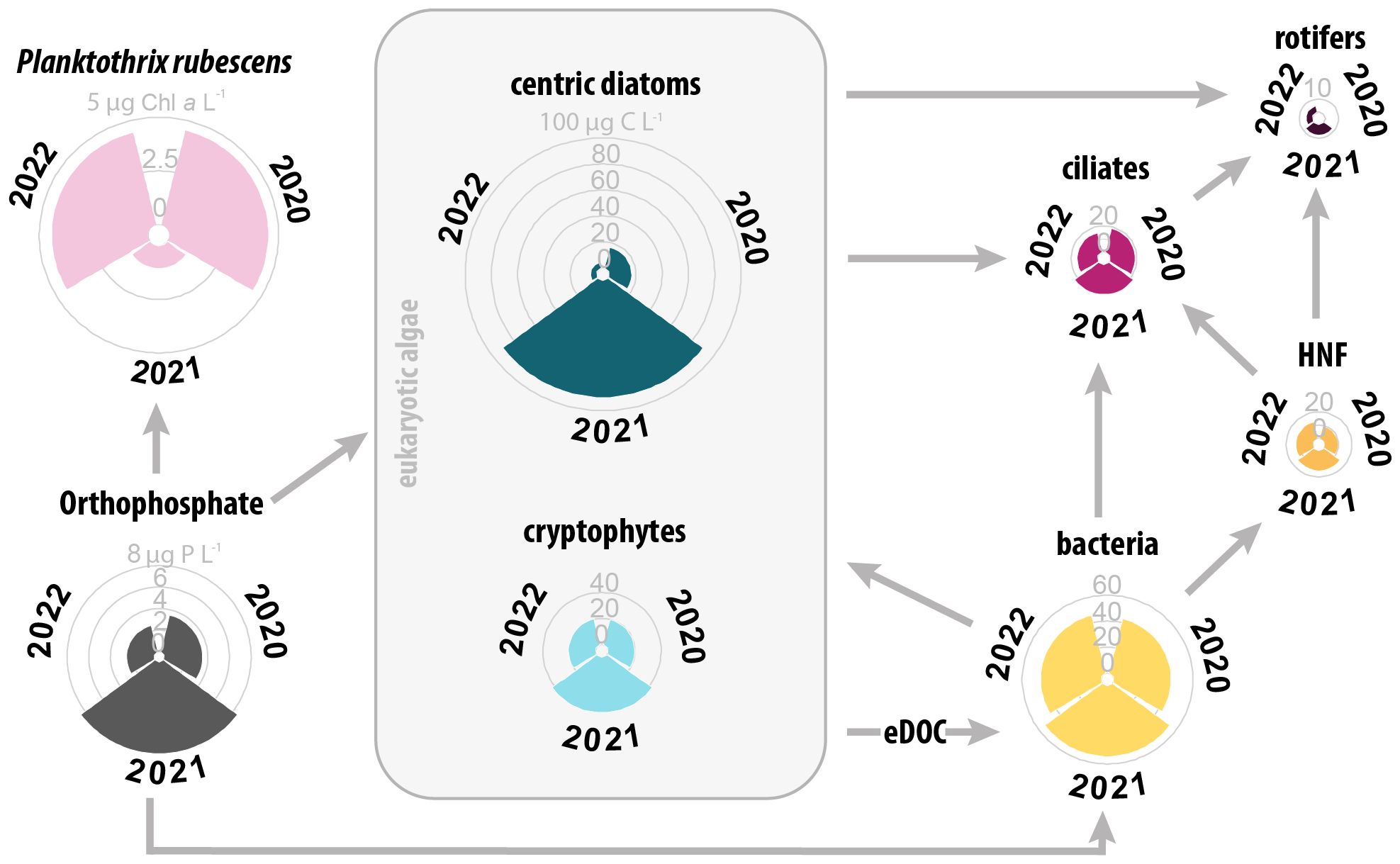
Figure 5. Schematic illustration of estimated energy transfer pathways in a simplified microbial food web of Lake Zurich during springtime. Comparison of initial epilimnetic orthophosphate concentration (PO4‐P, in µg P L−1) after winter mixing (average from 1 to 8 m), average daily biomass (µg C L−1) and average Chl a value (P. rubescens, in µg Chl a L−1) during spring (end-February until end-May) of the years 2020, 2021 and 2022. Therefore, arrows represent possible energy fluxes within the food web according to the scheme published by Fenchel (1987).
4 Discussion
4.1 Functioning of deep water turnover
While convective seasonal mixing occurred in all three winters, the deeper mixing in 2021 caused a three-fold higher concentration of epilimnetic PO4 compared to 2020/2022. In February 2021, convective mixing to 110 m depth was favored by isothermal conditions over the water column due to strong surface cooling in combination with relatively warm water at great depth. In contrast, temperature differences between the surface and the hypolimnetic water were too high to support deep winter mixing in February 2020 and 2022. As an effect, the hypolimnetic water temperatures were unusually high at the end of the subsequent stratified period. Besides a long-term trend of increasing temperatures at depth (Knapp et al., 2021), another pronounced warming occurred already in spring 2020. The stepwise warming was most probably induced by lateral inflow of warmer water from the shallow eastern lake domain. Cold nights in January 2020 caused a cooling of these shallow waters to ~5.2°C (alplakes.eawag.ch). Subsequently, these heavier water masses, which were cooler than the central surface but warmer than the deepest waters, flowed westwards to greater depths (Doda et al., 2022; Doda et al, 2023), where these intrusions finally led to warming of the deepest reaches, which remained weakly stratified.
4.2 Loss of total phosphorus through washout
Despite similar winter mixing conditions and phytoplankton bloom dynamics, the Ptot content of the pelagic zone and hypolimnetic PO4 concentrations were lower in 2022 than in 2020 (Supplementary Figure 2). A reason for this discrepancy can be found in the physico-chemical processes observed in the previous year. Deep mixing in combination with recurring turbulences in 2021 did not only result in a striking rise of epilimnetic PO4 concentrations, but also in a removal of hypolimnetic PO4. In addition, the high oxygen concentration in the deep zone (Figure 2B) potentially resulted in reduced PO4 release from the sediment surface (Einsele, 1936; Mortimer, 1942). However, this is not sufficient to explain the overall decrease in Ptot in the pelagic zone over the year 2021 (Supplementary Figure 2). An additional important reason might be the persistent heavy rainfalls lasting from May to July in 2021 (MeteoSwiss, 2021). To prevent flooding of the lakeshore, the outflow rate at the Limmat River weir was increased, especially during July (Supplementary Figure 3; https://www.hydrodaten.admin.ch). The higher discharge led to washout of PO4 and phytoplankton from Lake Zurich, resulting in an overall decrease in Ptot. Another reason could be the sedimentation of part of the algal biomass, especially diatoms (Smetacek, 1985), thereby transporting the bound phosphate to the sediment surface, where it was not yet released. In addition, the high Ptot export from the productive surface layer in 2021 explains why O2 consumption by decomposers was not enhanced even though production was highest in this year. This resulted in a persistent high oxygen concentration of the hypolimnion throughout 2021.
4.3 Consequences for the microbial food web
The vernal phytoplankton spring bloom is a recurring phenomenon in Lake Zurich. However, its intensity varies over the years and is influenced by mixing depth and subsequent upwelling of nutrients from the autochthonous pool in the hypolimnion (Yankova et al., 2017). In sum, the maximal abundances of the observed groups in 2021 were exceptional, even compared to dynamics observed during the last 15 years (Table 2). Although similarly high PO4 pulses have been observed before (2006, 2009, 2012 and 2018), the turbulent mixing episode in March 2021 caused a long-lasting nutrient enrichment of the epilimnion and a striking decline in P. rubescens (a major competitor for eukaryotic algae, see below).
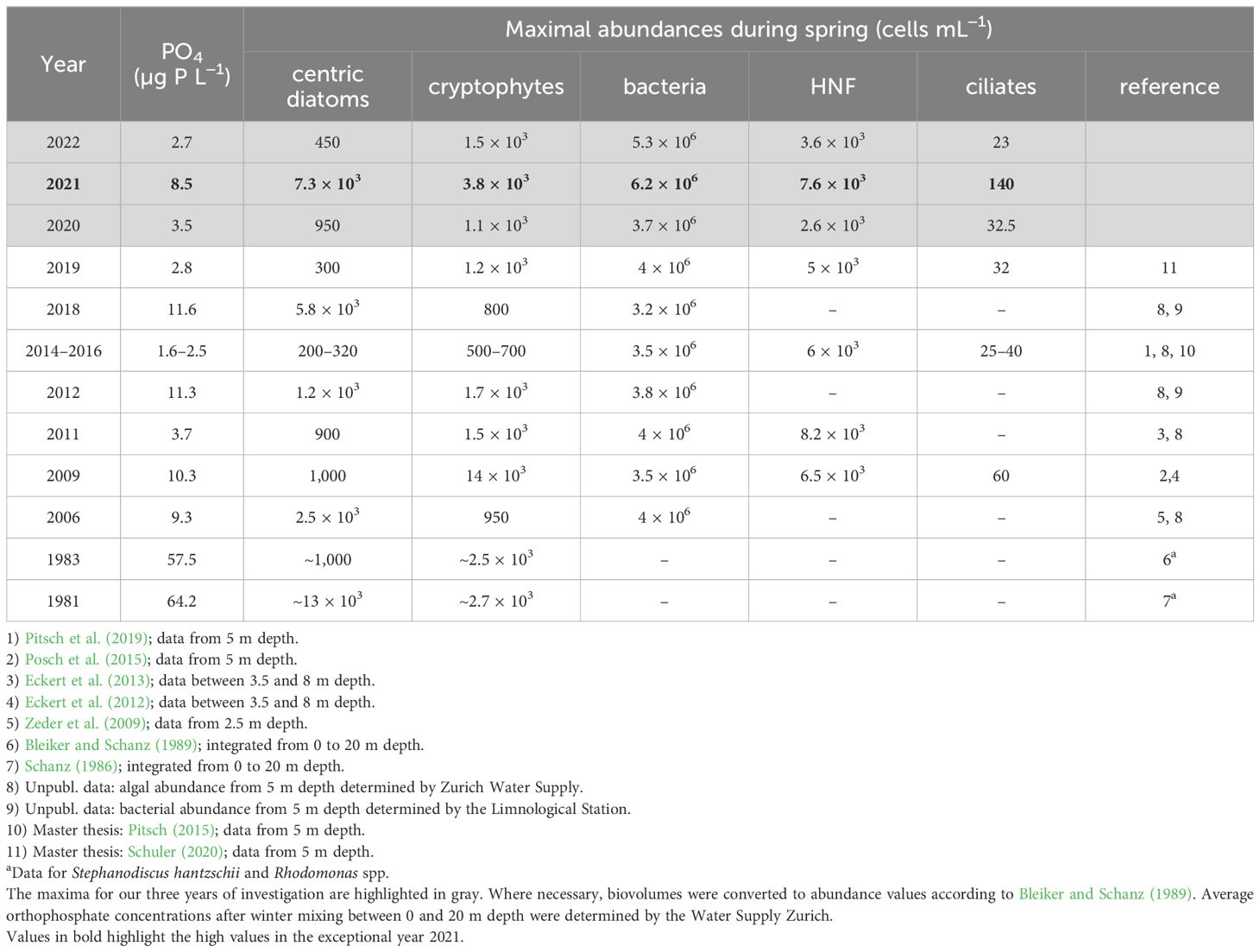
Table 2. Reported maximal abundances (cells mL−1) of the investigated organismic groups during spring period of the last 10 to 50 years from Lake Zurich.
4.3.1 Specific phytoplankton groups as profiteers
We focused on two algal groups (centric diatoms and cryptophytes) known to respond faster to nutrient replenishment in spring than other members of the phytoplankton community (Yankova et al., 2017). Additionally, owing to their unambiguous morphologies, both groups could be readily quantified by epifluorescence microscopy. This allowed for a relatively quick evaluation of many samples. However, we are aware that our approach cannot replace the classic quantification of algae by the Utermöhl technique (Utermöhl, 1958) if the entire phytoplankton community is to be analyzed.
Since the restoration of Lake Zurich starting in the 1960s, Ptot level have declined, whereas epilimnetic nitrogen concentrations have remained high (Posch et al., 2012). In the early 2000s, phosphorus became the limiting growth factor of phytoplankton in spring owing to reoccurring shallow winter mixing and the resulting insufficient replenishment of nutrients in the epilimnetic zone (Yankova et al., 2017). Despite rising phosphorus availability in 2021, not all studied autotrophic organisms have benefited equally, and centric diatoms were the primary profiteers (Figures 3, 5). These small-sized algae can have even higher growth rates than flagellated algae in the same size range (e.g., cryptophytes) under non-limiting conditions (Reynolds, 2006). Besides phosphorus, the growth of diatoms depends on the availability of silica, an essential component of frustules. However, while epilimnetic PO4 nearly disappeared in times of partial turnover during the early 2000s, silica slightly accumulated in the euphotic water layer due to reduced consumption (Yankova et al., 2017) and never fell below the critical threshold for diatom proliferation (Supplementary Figure 5; Egge and Aksnes, 1992). Furthermore, along with the favorable nutrient conditions, centric diatoms profited from the turbulent mixing episode in March 2021, allowing them to remain suspended in the euphotic zone for a longer period of time (Huisman et al., 2004). The second profiteers were cryptophytes. These typical r-strategists exhibited high growth rates at end of March, probably as response to high epilimnetic P levels. However, later abundance peaks in April and May hint at a different nutritional strategy less dependent on high P values (Sommer et al., 1986): Some genera of cryptophytes can acquire essential nutrients via mixotrophy by feeding on bacteria (Urabe et al., 2000; Yoo et al., 2017).
4.3.2 Planktothrix rubescens and mixing dynamics
Among autotrophic organisms, it was mainly P. rubescens (Figures 3, 5) that did not profit from environmental conditions in spring 2021. This filamentous cyanobacterium is usually the dominant phototrophic organism in Lake Zurich and develops massive annual blooms since the 1970s (Posch et al., 2012). Owing to its high toxicity to most eukaryotes (Blom et al., 2003; Kurmayer et al., 2016), predation on P. rubescens is very low and it is thought to be a sink rather than a link for nutrients within the food web. The physical downwelling of the filaments below 100 m depth is a limiting factor for P. rubescens. High hydrostatic pressure causes the collapse of their gas vesicles needed for buoyancy, thereby trapping filaments in the deep dark zone during winter mixing (Walsby et al., 1998). This was also the case in winter 2021, where deep convective turnover to ~110 m significantly reduced its population size. However, P. rubescens is not only influenced by downwelling of filaments, but also by its successful reestablishment in the metalimnion after spring. This is tightly associated with the vertical positioning of filaments in the water column, as triggered by irradiance (Knapp et al., 2021). From mid-February until end-March 2021, short stratification episodes were repeatedly interrupted by shallow mixing events, likely preventing the formation of a stable water column and thus the regrowth of the cyanobacterium during the nutrient-rich period. The absence of P. rubescens thus resulted in a competitive advantage for eukaryotic phytoplankton by reducing the dominant nutrient competitor.
4.3.3 Short lasting maxima of bacteria and HNF
The bacterial abundance peak in 2021 exceeded the observed maxima of the last 15 years by a factor of 1.5 (Table 2). Their growth was probably promoted by increased phosphorus availability (Mindl et al., 2005), as well as by the excretions of extracellular dissolved organic carbon (eDOC) from algae (Esteban and Fenchel, 2020). Nevertheless, total bacterial biomass differed marginally between the three years of our investigation (Dunn’s test, p>0.05; Figure 5) which could be linked to an increased predation pressure regulating the size of the bacterial standing stock. Microcosm experiments showed a similar weak response in bacterial abundance after nutrient addition (Yankova et al., 2017), where phosphorus (20 µg P L−1) was added to epilimnetic water, notable even three times higher concentrations than observed during deep mixing 2021. However, the slightly higher proportion of bacteria with high nucleic acid content in 2021 points to an increased bacterial activity compared to dynamics observed in the neighboring years (Supplementary Figure 4). Bacterial carbon maxima were followed by heterotrophic nanoflagellates as main predators of bacterial biomass. Like bacteria, they had only a temporary higher maximum in otherwise similar trends to 2020/2022 and thus no significant difference between years was observed (Dunn’s test, p>0.05; Figure 5).
4.4 Consequences for ciliates
By using the classical QPS approach to quantify ciliate morphotypes, we obtained reliable abundance and biomass estimates of the ciliate assemblage, as well as a data set that could be readily compared with previous studies (Table 2). Earlier attempts to apply next generation sequencing for the quantification of ciliates in Lake Zurich (Pitsch et al., 2019) further confirmed the known problems in ciliate abundance determination by this approach (Santoferrara et al., 2014). Alternatively, fluorescence in situ hybridization represents a powerful potential tool for reliably quantifying planktonic ciliate genotypes, as recently demonstrated for Lake Zurich (Dirren-Pitsch et al., 2022). However, the currently available repertoire of specific oligonucleotide probes is still too limited for analyzing the entire ciliate assemblage as presented in this study.
Although environmental conditions differed remarkably between the three years, there was clear resilience in ciliate morphotype composition (Figures 4A, B). The co-occurrence of a wide range of ciliate morphotypes may be due to their highly diversified ecological niches and foraging strategy: numerous ciliate species do not feed exclusively on one type of prey but are rather omnivorous (Posch et al., 2022). However, this study reports the highest ciliate numbers ever observed in Lake Zurich (Table 2).
4.4.1 Ciliates profiting from increasing food sources
Planktonic ciliates have fast doubling rates of <1 to 2 d−1, allowing for immediate response to rising prey availability (Macek et al., 1996). In Lake Zurich, particularly small fast-growing r-strategists benefited from increasing food amounts, including B. planctonicum and small Urotricha species which reached significantly higher numbers in early spring 2021 than in 2020/2022 (Dunn’s test, p<0.05).
In spring, B. planctonicum is a highly efficient grazer of small algae with preferences for cryptophytes (Tirok and Gaedke, 2007; Posch et al., 2015). It can have high growth rates resulting in up to 2 doublings d−1 at 18°C and 1 doubling d−1 at 9°C, respectively (Müller and Geller, 1993). These values are slightly higher than our observed net growth rate of 0.5 d−1 at ~7°C (1 doubling per 31 h) during the ciliate peak in 2021. There is a niche separation with equally sized, small Urotricha species, which feed on similar sized food items but are omnivores that also accept other prey types (Weisse et al., 2001). Small sized Urotricha species (e.g., U. furcata) are also characterized as r-strategists with slightly lower growth rates than B. planctonicum at low to moderate temperatures (5–18°C, Müller and Geller, 1993). Similar observations were made in Lake Zurich, where their net growth rate (0.3 d−1) was below that of B. planctonicum, which may explain the observed differences in maximal cell numbers. In our study, small-sized species of the genus Rimostrombidium (e.g., R. humile and brachykinetum) also responded quickly to the phytoplankton bloom with equal growth rates as B. planctonicum, and thus represented other efficient r-strategists within the ciliate assemblage. These species prefer small algae with a strong positive selection for cryptophytes (Posch et al., 2015), but can also ingest small centric diatoms (Müller and Schlegel, 1999). However, they were not as abundant as the two morphotypes mentioned above, and no significant differences between the three years could be observed for small Rimostrombidium species.
Despite the high biomass of centric diatoms in spring 2021, only a small number of ciliates profited from the rich food supply, because most ciliates cannot grow on an exclusive diet of diatoms (Skogstad et al., 1987). Ciliated interception feeders (e.g., B. planctonicum) select against immotile diatoms as a food source, whereas some filter feeders (e.g., Rimostrombidium and tintinnids) do not (Müller and Schlegel, 1999). In Lake Zurich, numbers of tintinnids increased significantly in early spring 2021. They benefitted from the high food availability, but also from diatom frustules as construction material for their loricae (Agatha et al., 2012). Remarkably, tintinnids built their loricae exclusively from centric diatom frustules after the large diatom bloom in 2021, whereas it consisted of various detrital particles in other years (Supplementary Figure 6).
Not all r-strategists increased to a similar extent. The fast-growing (up to 2.4 doublings d−1) Cyclidium glaucoma was just slightly more abundant in 2021 compared to the neighboring years. The strictly bacterivorous species has moderate ingestion rates in comparison to other bacterivorous ciliate species like H. grandinella (Šimek et al., 2019), potentially resulting in exploitation competition between the two species. In addition, abundance peaks of C. glaucoma and HNF were temporally shifted, suggesting that C. glaucoma was also in competition with flagellates for bacterial prey.
4.4.2 Top-down effects and consequences for the ciliate assemblage
With the proliferation of rotifers and prior to the peak of cladocerans, most ciliates species dropped in abundance towards the end of the investigation period, particularly in 2021. Ciliates are, besides phytoplankton and fine organic aggregates, within the accepted food size range of many planktonic rotifers. Species within the genera Asplanchna and Keratella were highly abundant in late spring 2021 and are known to prey efficiently on ciliates (Weisse and Frahm, 2002; Gilbert, 2022). Beside predation, food niches of planktonic rotifers overlap with many ciliates leading to an exploitative competition between the two groups (Gilbert, 2022).
However, a few ciliate species, like Histiobalantium bodamicum, seemed to benefit from the decline of total ciliate numbers and the concomitantly reduced competition. This large slow-growing species is an inferior competitor to ciliated r-strategists (Müller and Schlegel, 1999), and was significantly less abundant in the first half of spring 2021 than in the second half. In contrast to many other ciliates, it has a jumping like flight behavior similar to the jumping swim style of Halteria (Gilbert, 1994), allowing it to escape from rotifer predation.
Peritrichous ciliates of the genus Vorticella, mainly as epiphytes on the colonial diatom Fragilaria, also increased in abundance at the end of the spring periods 2020 and 2021. On the one hand, attachment is as grazing protection strategy against larger predators, as colonial pennate diatoms are outside the prey size range of most rotifers (Sonntag et al., 2006). On the other hand, sessile peritrichs can generate filtration currents and thus reach higher ingestion rates than their freely swimming competitors (Fenchel, 1987; Esteban and Fenchel, 2020), with grazing rates of up to 8,000 bacteria ciliate−1 h−1 (Šimek et al., 2019).
4.4.3 Delayed clear water phase
The renewed increase in phytoplankton productivity in the second half of April 2021 resulted in a delayed onset of the clear water phase (period with highest water transparency). The clear water phase goes hand in hand with low primary production and a decline in bacterial and HNF abundance. In this time of low food supply, mixotrophic ciliates benefit from their dual nutrition strategy, i.e., by feeding on particles and by using algal endosymbionts at the same time (Posch et al., 2022). The mixotrophic ciliate Pelagohalteria viridis typically becomes abundant at the onset of this period in Lake Zurich (Posch et al., 2015). Due to the delayed onset of the clear water phase in 2021, P. viridis occurred two weeks later than in the two other years of investigation, reflecting the significant discrepancy between the years (Dunn’s test). By contrast, other mixotrophic ciliates, e.g., Stokesia vernalis, already increased during early spring in parallel with r-strategists. This large slowly growing ciliate consumes a wide size range of food particles in addition to harboring endosymbiotic algae and thus probably benefitted from increased prey availability during that time of the year.
5 Conclusion
Despite climate warming, aperiodic deep winter turnover still occurs in Lake Zurich. Deep-water warming can facilitate deep mixing events, as an increasing heat content of hypolimnetic water allows homogenization of the water column at higher temperatures. Consequently, the strong epilimnetic enrichment by PO4 that has accumulated in the deep zone during previous periods of incomplete mixing, can promote intense blooms of centric diatoms and cryptophytes. Only a few ciliate species (Balanion planctonicum, Urotricha spp. <25µm and tintinnids) significantly increased their abundances during the first half of spring 2021 (deep mixing), compared to the adjacent years, whereas others showed only short-term abundance peaks and otherwise similar trends than in 2020/2022. Thus, the total ciliate assemblage showed a surprisingly high resilience in terms of morphotype composition. However, most planktonic ciliate species have been taxonomically described based on microscopy. Current research focuses on the potential cryptic genetic diversity within ciliate morphospecies, and which diagnostic features need to be examined to define it (Rajter et al., 2022; Smith et al., 2022). Therefore, apparent resilience of the composition of ciliate morphotypes may mask genotypic shifts within distinct morphotypes. While knowledge about the cryptic diversity of planktonic ciliate species is still in its infancy, new methodological tools are emerging (Dirren-Pitsch et al., 2022), that will open new perspectives on studying this topic.
Data availability statement
The original contributions presented in the study are included in the article/Supplementary Material. Further inquiries can be directed to the corresponding author.
Author contributions
MS-S: Writing – review & editing, Writing – original draft, Visualization, Validation, Supervision, Methodology, Investigation, Formal analysis, Data curation, Conceptualization. AW: Writing – review & editing, Visualization, Validation, Methodology, Formal analysis. GD-P: Writing – review & editing, Visualization, Validation, Supervision, Methodology, Investigation, Formal analysis. RN: Writing – review & editing, Methodology, Investigation, Data curation. BB: Writing – review & editing, Methodology, Investigation, Data curation. OK: Writing – review & editing, Data curation. JP: Writing – review & editing, Supervision, Resources. TP: Writing – review & editing, Validation, Supervision, Resources, Project administration, Methodology, Investigation, Funding acquisition, Formal analysis, Data curation, Conceptualization.
Funding
The author(s) declare financial support was received for the research, authorship, and/or publication of this article. This study was supported by the Swiss National Science Foundation grants 31003A_182489 and 31003A_182336.
Acknowledgments
We thank E. Loher and D. Marty for all the help during the intensive sampling campaign. We thank the Water Police Zurich for providing meteorological data and the federal government (BAFU) for discharge values of Lake Zurich.
Conflict of interest
The authors declare that the research was conducted in the absence of any commercial or financial relationships that could be construed as a potential conflict of interest.
Publisher’s note
All claims expressed in this article are solely those of the authors and do not necessarily represent those of their affiliated organizations, or those of the publisher, the editors and the reviewers. Any product that may be evaluated in this article, or claim that may be made by its manufacturer, is not guaranteed or endorsed by the publisher.
Supplementary material
The Supplementary Material for this article can be found online at: https://www.frontiersin.org/articles/10.3389/frpro.2024.1428985/full#supplementary-material
References
Agatha S., Laval-Peuto M., Simon P. (2012). “The tintinnid lorica,” in The biology and ecology of tintinnid ciliates. Eds. Dolan J. R., Montagnes D. J. S., Agatha S., Coats D. W., Stoecker D. K. (Wiley-Blackwell, United State), 17–41.
Beutler M., Wiltshire K. H., Meyer B., Moldaenke C., Lüring C., Meyerhöfer M., et al. (2002). A fluorometric method for the differentiation of algal populations in vivo and in situ. Photosynth. Res. 72, 39–53. doi: 10.1023/A:1016026607048
Bleiker W., Schanz F. (1989). Influence of environmental factors on the phytoplankton spring bloom in Lake Zürich. Aquat. Sci. 51, 47–58. doi: 10.1007/BF00877780
Blom J. F., Bister B., Bischoff D., Nicholson G., Jung G., Süssmuth R. D., et al. (2003). Oscillapeptin J, a new grazer toxin of the freshwater cyanobacterium Planktothrix rubescens. J. Nat. Prod. 66, 431–434. doi: 10.1021/np020397f
Børsheim K. Y., Bratbak G. (1987). Cell volume to cell carbon conversion factors for a bacterivorous Monas sp. enriched from seawater. Mar. Ecol. Prog. Ser. 36, 171–175. doi: 10.3354/meps036171
Bossard P., Gammeter S., Lehmann C., Schanz F., Bachofen R., Bürgi H.-R., et al (2001). Limnological description of the Lakes Zurich, Lucerne, and Cadagno. Aquat. Sci. 63, 225–249. doi: 1007/PL000013533
Desgué-Itier O., Melo Vieira Soares L., Anneville O., Bouffard D., Chanudet V., Danis P. A., et al. (2023). Past and future climate change effects on the thermal regime and oxygen solubility of four peri-alpine lakes. Hydrol. Earth Syst. Sci. 27, 837–859. doi: 10.5194/hess-27-837-2023
Dirren-Pitsch G., Bühler D., Salcher M. M., Bassin B., Le Moigne A., Schuler M., et al. (2022). FISHing for ciliates: Catalyzed reporter deposition fluorescence in situ hybridization for the detection of planktonic freshwater ciliates. Front. Microbiol. 13. doi: 10.3389/fmicb.2022.1070232
Doda T., Ramón C. L., Ulloa H. N., Wüest A., Bouffard D. (2022). Seasonality of density currents induced by differential cooling. Hydrol. Earth Syst. Sci. 26, 331–353. doi: 10.5194/hess-26-331-2022
Doda T., Ulloa H. N., Ramón C. L., Wüest A., Bouffard D. (2023). Penetrative convection modifies the dynamics of downslope gravity currents. Geophys. Res. Lett. 50, e2022GL100633. doi: 10.1029/2022GL100633
Dokulil M. T. (2014). Impact of climate warming on European inland waters. Inl. Waters 4, 27–40. doi: 10.5268/IW-4.1.705
Dokulil M. T., de Eyto E., Maberly S. C., May L., Weyhenmeyer G. A., Woolway R. I. (2021). Increasing maximum lake surface temperature under climate change. Clim. Change 165, 56. doi: 10.1007/s10584-021-03085-1
Eckert E. M., Baumgartner M., Huber I. M., Pernthaler J. (2013). Grazing resistant freshwater bacteria profit from chitin and cell-wall-derived organic carbon. Environ. Microbiol. 15, 2019–2030. doi: 10.1111/1462-2920.12083
Eckert E. M., Salcher M. M., Posch T., Eugster B., Pernthaler J. (2012). Rapid successions affect microbial N-acetyl-glucosamine uptake patterns during a lacustrine spring phytoplankton bloom. Environ. Microbiol. 14, 794–806. doi: 10.1111/j.1462-2920.2011.02639.x
Egge J. K., Aksnes D. L. (1992). Silicate as regulating nutrient in phytoplankton competition. Mar. Ecol. Prog. Ser. 83, 281–289. doi: 10.3354/meps083281
Einsele (1936). Über die Beziehungen des Eisenkreislaufs zum Phosphatkreislauf im eutrophen See. Arch. für Hydrobiol. 29, 664–686.
Esteban G. F., Fenchel T. M. (2020). Ecology of protozoa. 2nd ed (Berlin: Springer Verlag). doi: 10.1007/978-3-030-59979-9
Fenchel T. (1987). The biology of free-living phagotrophic protists (Berlin: Springer Verlag). doi: 10.1007/978-3-030-59979-9
Foissner W., Berger H., Blatterer H., Kohmann F. (1995). Taxonomische und ökologische Revision der Ciliaten des Saprobiensystems. Band IV: Gymnostomatea, Loxodes, Suctoria. Heft 1/95 (Munich: Informationsberichte des Bayerischen Landesamtes für Wasserwirtschaft).
Foissner W., Berger H., Kohmann F. (1994). Taxonomische und ökologische Revision der Ciliaten des Saprobiensystems – Band III: Hymenostomata, Prostomatida, Nassulida. Heft 1/94 (Munich: Informationsberichte des Bayerischen Landesamtes für Wasserwirtschaft).
Foissner W., Berger H., Schaumburg J. (1999). Identification and ecology of limnetic plankton ciliates. Heft 3/99 (Munich: Informationsberichte des Bayerischen Landesamtes für Wasserwirtschaft).
Foissner W., Blatterer H., Berger H., Kohmann F. (1992). Taxonomische und ökologische Revision der Ciliaten des Saprobiensystems. Band II: Peritrichia, Heterotrichida, Odontostomatida. Heft 5/92 (Munich: Informationsberichte des Bayerischen Landesamtes für Wasserwirtschaft).
Foissner W., Blatterer H., Kohmann F. (1991). Taxonomische und ökologische Revision der Ciliaten des Saprobiensystems – Band I: Cryptophorida, Oligotrichida, Hypotrichia, Colpodea. Heft 1/91 (Munich: Informationsberichte des Bayerischen Landesamtes für Wasserwirtschaft).
Gao F., Warren A., Zhang Q., Gong J., Miao M., Sun P., et al. (2016). The all-data-based evolutionary hypothesis of ciliated protists with a revised classification of the phylum Ciliophora (Eukaryota, Alveolata). Sci. Rep. 6, 24874. doi: 10.1038/srep24874
Gilbert J. J. (1994). Jumping behavior in the oligotrich ciliates Strobilidium velox and Halteria grandinella, and its significance as a defense against rotifer predators. Microb. Ecol. 27, 189–200. doi: 10.1007/BF00165817
Gilbert J. J. (2022). Food niches of planktonic rotifers: Diversification and implications. Limnol. Oceanogr. 67, 2218–2251. doi: 10.1002/lno.12199
Hofmann H., Peeters F. (2013). In-situ optical and acoustical measurements of the buoyant cyanobacterium P. rubescens: Spatial and temporal distribution patterns. PloS One 8, e80913. doi: 10.1371/journal.pone.0080913
Holzner C. P., Aeschbach-Hertig W., Simona M., Veronesi M., Imboden D. M., Kipfer R. (2009). Exceptional mixing events in meromictic Lake Lugano (Switzerland/Italy), studied using environmental tracers. Limnol. Oceanogr. 54, 1113–1124. doi: 10.4319/lo.2009.54.4.1113
Huisman J., Sharples J., Stroom J. M., Visser P. M., Kardinaal W. E. A., Verspagen J. M. H., et al. (2004). Changes in turbulent mixing shift competition for light between phytoplankton species. Ecology 85, 2960–2970. doi: 10.1890/03-0763
Knapp D., Fernández Castro B., Marty D., Loher E., Köster O., Wüest A., et al. (2021). The red harmful plague in times of climate change: Blooms of the cyanobacterium Planktothrix rubescens triggered by stratification dynamics and irradiance. Front. Microbiol. 12. doi: 10.3389/fmicb.2021.70591
Kurmayer R., Deng L., Entfellner E. (2016). Role of toxic and bioactive secondary metabolites in colonization and bloom formation by filamentous cyanobacteria Planktothrix. Harmful Algae 54, 69–86. doi: 10.1016/j.hal.2016.01.004
Leboulanger C., Dorigo U., Jacquet S., Le Berre B., Paolini G., Humbert J. F. (2002). Application of a submersible spectrofluorometer for rapid monitoring of freshwater cyanobacterial blooms: a case study. Aquat. Microb. Ecol. 30, 83–89. doi: 10.3354/ame030083
Lepori F., Bartosiewicz M., Simona M., Veronesi M. (2018). Effects of winter weather and mixing regime on the restoration of a deep perialpine lake (Lake Lugano, Switzerland and Italy). Hydrobiologia 824, 229–242. doi: 10.1007/s10750-018-3575-2
Lynn D. H. (2008). The ciliated protozoa. Characterization, classification, and guide to the literature. 3rd ed (Dordrecht: Springer Netherlands). doi: 10.1007/978-1-4020-8239-9
Macek M., Šimek K., Pernthaler J., Vyhnálek V., Psenner R. (1996). Growth rates of dominant planktonic ciliates in two freshwater bodies of different trophic degree. J. Plankton Res. 18, 463–481. doi: 10.1093/plankt/18.4.463
Menden-Deuer S., Lessard E. J. (2000). Carbon to volume relationships for dinoflagellates, diatoms, and other protist plankton. Limnol. Oceanogr. 45, 569–579. doi: 10.4319/lo.2000.45.3.0569
MeteoSwiss (2021). Klimabulletin sommer 2021 (Zürich). Available online at: https://www.meteoschweiz.admin.ch/service-und-publikationen/publikationen/berichte-und-bulletins/2021/klimabulletin-sommer-2021.html (Accessed January 19, 2024).
Mindl B., Sonntag B., Pernthaler J., Vrba J., Psenner R., Posch T. (2005). Effects of phosphorus loading on interactions of algae and bacteria: Reinvestigation of the “phytoplankton-bacteria paradox” in a continuous cultivation system. Aquat. Microb. Ecol. 38, 203–213. doi: 10.3354/ame038203
Montagnes D. J. S., Berges J. A., Harrison P. J., Taylor F. J. R. (1994). Estimating carbon, nitrogen, protein, and chlorophyll a from volume in marine phytoplankton. Limnol. Oceanogr. 39, 1044–1060. doi: 10.4319/lo.1994.39.5.1044
Mortimer C. H. (1942). The exchange of dissolved substances between mud and water in lakes. J. Ecol. 29, 147–201. doi: 10.2307/2256691
Müller H., Geller W. (1993). Maximum growth rates of aquatic ciliated protozoa: the dependence on body size and temperature reconsidered. Arch. für Hydrobiol. 126, 315–327. doi: 10.1127/archiv-hydrobiol/126/1993/315
Müller H., Schlegel A. (1999). Responses of three freshwater planktonic ciliates with different feeding modes to cryptophyte and diatom prey. Aquat. Microb. Ecol. 17, 49–60. doi: 10.3354/ame017049
O’Reilly C. M., Alin S. R., Piisnier P. D., Cohen A. S., McKee B. A. (2003). Climate change decreases aquatic ecosystem productivity of Lake Tanganyika, Africa. Nature 424, 766–768. doi: 10.1038/nature01833
O’Reilly R. R.J., Schneider P., Lenters J. D., Mcintyre P. B., Kraemer B. M. (2015). Rapid and highly variable warming of lake surface waters around the globe. Geophys. Res. Lett. 42, 10773–10781. doi: 10.1002/2015GL066235
Örn C. G., Schanz F., Thomas E. A. (1981). An empirical model relating wind action and hypolimnetic oxygenation during vernal circulation in Lake Zurich from 1950–1979. Verhandlungen Des. Int. Verein Limnol. 21, 109–114. doi: 10.1080/03680770.1980.11896965
Pauli H. R. (1989). A new method to estimate individual dry weights of rotifers. Hydrobiologia 186, 355–361. doi: 10.1007/BF00048932
Peeters F., Livingstone D. M., Goudsmit G. H., Kipfer R., Forster R. (2002). Modeling 50 years of historical temperature profiles in a large central European lake. Limnol. Oceanogr. 47, 186–197. doi: 10.4319/lo.2002.47.1.0186
Pfister G., Sonntag B., Posch T. (1999). Comparison of a direct live count and an improved quantitative protargol stain (QPS) in determining abundance and cell volumes of pelagic freshwater protozoa. Aquat. Microb. Ecol. 18, 95–103. doi: 10.3354/ame018095
Pitsch G. (2015). Morphological and molecular based analyses of planktonic ciliates in Lake Zurich. University of Zurich, Zurich (CH).
Pitsch G., Bruni E. P., Forster D., Qu Z., Sonntag B., Stoeck T., et al. (2019). Seasonality of planktonic freshwater ciliates: Are analyses based on V9 regions of the 18S rRNA gene correlated with morphospecies counts? Front. Microbiol. 10. doi: 10.3389/fmicb.2019.00248
Posch T., Eugster B., Pomati F., Pernthaler J., Pitsch G., Eckert E. M. (2015). Network of interactions between ciliates and phytoplankton during spring. Front. Microbiol. 6. doi: 10.3389/fmicb.2015.01289
Posch T., Franzoi J., Prader M., Salcher M. M. (2009). New image analysis tool to study biomass and morphotypes of three major bacterioplankton groups in an alpine lake. Aquat. Microb. Ecol. 54, 113–126. doi: 10.3354/ame01269
Posch T., Köster O., Salcher M. M., Pernthaler J. (2012). Harmful filamentous cyanobacteria favored by reduced water turnover with lake warming. Nat. Clim. Change 2, 809–813. doi: 10.1038/nclimate1581
Posch T., Pitsch G., Bruni E. P. (2022). “Protists: ciliates,” in Encyclopedia of inland waters (Elsevier, Oxford), 639–649. doi: 10.1016/B978-0-12-819166-8.00001-3
Porter K. G., Feig Y. S. (1980). The use of DAPI for identifying and counting aquatic microflora. Limnol. Oceanogr. 25, 943–948. doi: 10.4319/lo.1980.25.5.0943
Rajter Ľ., Lu B., Rassoshanska E., Dunthorn M. (2022). Future prospects for investigating ciliate biodiversity. Acta Protozool. 61, 35–46. doi: 10.4467/16890027AP.22.005.16236
Råman Vinnå L., Medhaug I., Schmid M., Bouffard D. (2021). The vulnerability of lakes to climate change along an altitudinal gradient. Commun. Earth Environ. 2, 35. doi: 10.1038/s43247-021-00106-w
Reynolds C. S. (2006). The ecology of phytoplankton (Cambridge: Cambridge University Press). doi: 10.1017/CBO9780511542145
Salmaso N., Boscaini A., Capelli C., Cerasino L. (2018). Ongoing ecological shifts in a large lake are driven by climate change and eutrophication: evidences from a three-decade study in Lake Garda. Hydrobiologia 824, 177–195. doi: 10.1007/s10750-017-3402-1
Sanders R., Wickham S. A. (1993). Planktonic protozoa and metazoa: Predation, food quality and population control. Mar. Microb. Food Webs 7, 197–223.
Santoferrara L. F., Grattepanche J.-D., Katz L. A., McManus G. B. (2014). Pyrosequencing for assessing diversity of eukaryotic microbes: analysis of data on marine planktonic ciliates and comparison with traditional methods. Environ. Microbiol. 16, 2752–2763. doi: 10.1111/1462-2920.12380
Schanz F. (1986). Depth distribution of phytoplankton and associated spectral changes in downward irradiance in Lake Züric/81). Hydrobiologia 134, 183–192. doi: 10.1007/BF00006740
Schmid M., Köster O. (2016). Excess warming of a Central European lake driven by solar brightening. Water Resour. Res. 52, 8103–8116. doi: 10.1002/2016WR018651
Schneider P., Hook S. (2010). Space observations of inland water bodies show rapid surface warming since 1985. J. Geophys. Res. Lett. 37, L22405. doi: 10.1029/2010GL045059
Schuler M. (2020). In situ versus artificial water turnover during spring : A comparison of microbial food web structures. University of Zurich, Zurich (CH).
Schwefel R., Gaudard A., Wüest A., Bouffard D. (2016). Effects of climate change on deepwater oxygen and winter mixing in a deep lake (Lake Geneva): Comparing observational findings and modeling. Water Resour. Res. 52, 8811–8826. doi: 10.1002/2016WR019194
Schwefel R., Müller B., Boisgontier H., Wüest A. (2019). Global warming affects nutrient upwelling in deep lakes. Aquat. Sci. 81, 50. doi: 10.1007/s00027-019-0637-0
Shimoda Y., Azim M. E., Perhar G., Ramin M., Kenney M. A., Sadraddini S., et al. (2011). Our current understanding of lake ecosystem response to climate change: What have we really learned from the north temperate deep lakes? J. Great Lakes Res. 37, 173–193. doi: 10.1016/j.jglr.2010.10.004
Šimek K., Grujčić V., Nedoma J., Jezberová J., Šorf M., Matoušů A., et al. (2019). Microbial food webs in hypertrophic fishponds: Omnivorous ciliate taxa are major protistan bacterivores. Limnol. Oceanogr. 64, 2295–2309. doi: 10.1002/lno.11260
Skibbe O. (1994). An improved quantitative protargol stain for ciliates and other planktonic protists. Arch. für Hydrobiol. 130, 339–347. doi: 10.1127/archiv-hydrobiol/130/1994/339
Skogstad A., Granskog L., Klaveness D. (1987). Growth of freshwater ciliates offered planktonic algae as food. J. Plankton Res. 9, 503–512. doi: 10.1093/plankt/9.3.503
Smetacek V. S. (1985). Role of sinking in diatom life-history cycles: ecological, evolutionary and geological significance. Mar. Biol. 84, 239–251. doi: 10.1007/BF00392493
Smith S. A., Santoferrara L. F., Katz L. A., McManus G. B. (2022). Genome architecture used to supplement species delineation in two cryptic marine ciliates. Mol. Ecol. Resour. 22, 2880–2896. doi: 10.1111/1755-0998.13664
Sommer U., Adrian R., De Senerpont Domis L., Elser J. J., Gaedke U., Ibelings B., et al. (2012). Beyond the plankton ecology group (PEG) model: Mechanisms driving plankton succession. Annu. Rev. Ecol. Evol. Syst. 43, 429–448. doi: 10.1146/annurev-ecolsys-110411-160251
Sommer U., Gliwicz Z. M., Lampert W. I., Duncan A. (1986). The PEG-model of seasonal succession of planktonic events in fresh waters. Arch. fur Hydrobiol. 106, 433–471. doi: 10.1127/archiv-hydrobiol/106/1986/433
Sonntag B., Posch T., Klammer S., Teubner K., Psenner R. (2006). Phagotrophic ciliates and flagellates in an oligotrophic, deep, alpine lake: Contrasting variability with seasons and depths. Aquat. Microb. Ecol. 43, 193–207. doi: 10.3354/ame043193
Telesh I. V., Rahkola M., Viljanen M. (1998). Carbon content of some freshwater rotifers. Hydrobiologia 388, 355–360. doi: 10.1007/978-94-011-4782-8_47
Tirok K., Gaedke U. (2007). Regulation of planktonic ciliate dynamics and functional composition during spring in Lake Constance. Aquat. Microb. Ecol. 49, 87–100. doi: 10.3354/ame01127
Toffolon M., Piccolroaz S., Majone B., Soja A. M., Peeters F., Schmid M., et al. (2014). Prediction of surface temperature in lakes with different morphology using air temperature. Limnol. Oceanogr. 59, 2185–2202. doi: 10.4319/lo.2014.59.6.2185
Urabe J., Gurung T. B., Yoshida T., Sekino T., Nakanishi M., Maruo M., et al. (2000). Diel changes in phagotrophy by Cryptomonas in Lake Biwa. Limnol. Oceanogr. 45, 1558–1563. doi: 10.4319/lo.2000.45.7.1558
Utermöhl H. (1958). Zur Vervollkommnung der quantitativen Phytoplankton-Methodik. Mitt. Int. Verein. Theor. Angew. Limnol. 9, 1–38.
Walsby A. E., Avery A., Schanz F. (1998). The critical pressures of gas vesicles in Planktothrix rubescens in relation to the depth of winter mixing in Lake Zurich, Switzerland. J. Plankton Res. 20, 1357–1375. doi: 10.1093/plankt/20.7.1357
Weisse T. (2017). Functional diversity of aquatic ciliates. Eur. J. Protistol. 61, 331–358. doi: 10.1016/j.ejop.2017.04.001
Weisse T., Frahm A. (2002). Direct and indirect impact of two common rotifer species (Keratella spp.) on two abundant ciliate species (Urotricha furcata, Balanion planctonicum). Freshw. Biol. 47, 53–64. doi: 10.1046/j.1365-2427.2002.00780.x
Weisse T., Karstens N., Meyer V. C. L., Janke L., Lettner S., Teichgräber K. (2001). Niche separation in common prostome freshwater ciliates: The effect of food and temperature. Aquat. Microb. Ecol. 26, 167–179. doi: 10.3354/ame026167
Woolway R. I., Kraemer B. M., Lenters J. D., Merchant C. J., O’Reilly C. M., Sharma S. (2020). Global lake responses to climate change. Nat. Rev. Earth Environ. 1, 388–403. doi: 10.1038/s43017-020-0067-5
Woolway R. I., Merchant C. J. (2019). Worldwide alteration of lake mixing regimes in response to climate change. Nat. Geosci. 12, 271–276. doi: 10.1038/s41561-019-0322-x
Yankova Y., Neuenschwander S., Köster O., Posch T. (2017). Abrupt stop of deep water turnover with lake warming: Drastic consequences for algal primary producers. Sci. Rep. 7, 13770. doi: 10.1038/s41598-017-13159-9
Yankova Y., Villiger J., Pernthaler J., Schanz F., Posch T. (2016). Prolongation, deepening and warming of the metalimnion change habitat conditions of the harmful filamentous cyanobacterium Planktothrix rubescens in a prealpine lake. Hydrobiologia 776, 125–138. doi: 10.1007/s10750-016-2745-3
Yoo Y., Seong K. A., Jeong H. J., Yih W., Rho J.-R., Nam S. W., et al. (2017). Mixotrophy in the marine red-tide cryptophyte Teleaulax amphioxeia and ingestion and grazing impact of cryptophytes on natural populations of bacteria in Korean coastal waters. Harmful Algae 68, 105–117. doi: 10.1016/j.hal.2017.07.012
Keywords: complete turnover, deep winter mixing, freshwater microbial food web, lake warming, Lake Zurich, pelagic ciliates, phytoplankton spring bloom, resilience of freshwater food webs
Citation: Schalch-Schuler M, Wüest A, Dirren-Pitsch G, Niedermann R, Bassin B, Köster O, Pernthaler J and Posch T (2024) Variability of winter cooling affects intensity of phytoplankton spring blooms – how resilient is the ciliate assemblage composition to changes in food availability? Front. Protistol. 2:1428985. doi: 10.3389/frpro.2024.1428985
Received: 07 May 2024; Accepted: 24 July 2024;
Published: 12 August 2024.
Edited by:
Jean-David Grattepanche, Temple University, United StatesReviewed by:
Marija Gligora Udovic, University of Zagreb, CroatiaWeiwei Liu, Chinese Academy of Sciences (CAS), China
Copyright © 2024 Schalch-Schuler, Wüest, Dirren-Pitsch, Niedermann, Bassin, Köster, Pernthaler and Posch. This is an open-access article distributed under the terms of the Creative Commons Attribution License (CC BY). The use, distribution or reproduction in other forums is permitted, provided the original author(s) and the copyright owner(s) are credited and that the original publication in this journal is cited, in accordance with accepted academic practice. No use, distribution or reproduction is permitted which does not comply with these terms.
*Correspondence: Thomas Posch, cG9zY2hAbGltbm9sLnV6aC5jaA==